- 1Department of Ecology, Evolution, and Marine Biology, University of California, Santa Barbara, CA, United States
- 2Marine Science Institute, University of California, Santa Barbara, CA, United States
Introduction: Changes in temperature can fundamentally transform how species interact, causing wholesale shifts in ecosystem dynamics and stability. Yet we still have a limited understanding of how temperature-dependence in physiology drives temperature-dependence in species-interactions. For predator-prey interactions, theory predicts that increases in temperature drive increases in metabolism and that animals respond to this increased energy expenditure by ramping up their food consumption to meet their metabolic demand. However, if consumption does not increase as rapidly with temperature as metabolism, increases in temperature can ultimately cause a reduction in consumer fitness and biomass via starvation.
Methods: Here we test the hypothesis that increases in temperature cause more rapid increases in metabolism than increases in consumption using the California spiny lobster (Panulirus interruptus) as a model system. We acclimated individual lobsters to temperatures they experience sacross their biogeographic range (11, 16, 21, or 26°C), then measured whether lobster consumption rates are able to meet the increased metabolic demands of rising temperatures.
Results and discussion: We show positive effects of temperature on metabolism and predation, but in contrast to our hypothesis, rising temperature caused lobster consumption rates to increase at a faster rate than increases in metabolic demand, suggesting that for the mid-range of temperatures, lobsters are capable of ramping up consumption rates to increase their caloric demand. However, at the extreme ends of the simulated temperatures, lobster biology broke down. At the coldest temperature, lobsters had almost no metabolic activity and at the highest temperature, 33% of lobsters died. Our results suggest that temperature plays a key role in driving the geographic range of spiny lobsters and that spatial and temporal shifts in temperature can play a critical role in driving the strength of species interactions for a key predator in temperate reef ecosystems.
Introduction
To predict the ecological consequences of global change, we require a mechanistic understanding of how changes in temperature cascade from changes in animal physiology to changes in animal ecology. However, our mechanistic understanding of temperature-dependence in ecology remains incomplete. One way to measure the effects of temperature in ecology is to measure how the strength of interactions between two species changes as temperature changes. Here we consider temperature dependence in predator-prey interactions and define interaction strength as the the effect of one individual of a predator has on one individual of a prey species per unit time (Laska and Wootton, 1998). It is well established that increases in temperature typically increase the strength of predator-prey interactions (Rall et al., 2010; Kordas et al., 2011), which has the potential alter ecosystem dynamics and stability (Vasseur and McCann, 2005; Vucic-Pestic et al., 2011; e.g., Gilbert et al., 2014). However we are still unpacking the physiological mechanisms underlying temperature-dependent predation (Biro and Stamps, 2010; Warne et al., 2019). Resolving this incomplete link between temperature-dependence in physiology and predation can allow us to better predict how natural and human-induced shifts in temperature are likely to alter the strength of interactions between species to shift ecosystem stability, function, and services (Chiabai et al., 2018).
For ectotherms, the leading hypothesis for why predation rates increase with temperature is that rising temperatures increase an animal’s basal metabolism, and that this increase in metabolism with temperature creates a caloric demand that an animal can only meet by increasing their consumption rates (Brown et al., 2004). However, metabolism and consumption may not increase at the same rate with with temperature. If metabolism increases more rapidly with temperature than consumption increases, animals may spend the majority of the calories they consume maintaining homeostasis rather than allocating new calories to growth or egg production as temperatures rise (Vucic-Pestic et al., 2011; Rall et al., 2012; Huey and Kingsolver, 2019). Such a calorie deficit would be a mechanistic explanation for potential negative consequences of global warming on natural ecosystems (Huey and Kingsolver, 2019). Yet very few studies have simultaneously studied the effects of temperature on animal metabolism and predation rates to explicitly link metabolism to predation and resolve whether predation rates change sufficiently rapidly to meet metabolic demand at temperature extremes (Sohlström et al., 2021).
Much of what ecologists know about the link between temperature, metabolism, and predation comes from tests of the metabolic theory of ecology (MTE), which predicts that predation rates should increase with temperature at the same rate as basal metabolism increases with temperature (Gillooly et al., 2001; Brown et al., 2004; Clarke, 2004). Meta-analysis testing the prediction suggests that while predation rates do systematically increase with temperature, the rate at which predation increases with temperature differs substantially from the predictions of metabolic theory (Englund et al., 2011; Rall et al., 2012; Bruno et al., 2015; Lindmark et al., 2022). These tests further our understanding of scaling relationships, but do not directly correlate an animal’s metabolism with its predation rate and thus offer little insight into whether rising temperatures cause calorie deficits. Instead, they examine how predation rates scale across taxa that live in different temperatures and compare this scaling to the rate at which metabolism scales across taxa that live in different temperatures (but see Lindmark et al., 2022). To understand whether and how metabolism and predation are connected we require studies that carefully measure animal bioenergetics and link an individual predator’s metabolism to its predation rate.
Ecologists thinking about temperature-dependence in predator metabolism typically focus on standard metabolic rate as a predictor of predation rates (Vucic-Pestic et al., 2011). Standard metabolic rate, which describes an organism’s baseline energy expenditure at rest excluding digestion, reproduction, and growth (Hulbert and Else, 2000), should explain some of an animal’s demand for food because animals must at minimum meet these demands to survive. In practice, resting metabolic rates are commonly measured in lieu of standard metabolic rates because of the logistical challenges of measuring animals over several days (Eliason and Farrell, 2016). However, additional components of animal metabolism are also important, because the act of predation must feed the calories required for an animal’s basal metabolism as well as the energy need to grow, reproduce, forage and digest food (predation) (Biro and Stamps, 2010). Individuals with higher standard or resting metabolic rates also tend to have higher maximum metabolic rates (Killen et al., 2016), which expands their energetic capacity that can be invested in prior listed vital performances. Therefore, measuring standard or resting metabolic rate alone may underestimate the bioenergetic demands that fuel predation intensity and behavior. Instead, we predict an animal’s absolute aerobic scope (AAS) – the difference between the maximum metabolic rate (MMR) that sets the upper limit for AAS and resting metabolic rate (RMR)– to predict predator consumption rates (e.g., Auer et al., 2015). Absolute aerobic scope represents the overall capacity for the cardio-respiratory system to supply oxygen for activities beyond resting metabolic rate, and can be positively correlated with a variety of performance measures such as locomotion (Killen et al., 2007), feeding capacity (Auer et al., 2015), and ability to recover from exercise or stress events (Marras et al., 2010).
In this study we link temperature-dependence in physiological responses to temperature-dependence in predator-prey interactions in a model predator – the California spiny lobster, (Panulirus interruptus, hereafter spiny lobster). On the west coast of North America, spiny lobsters are both an important predator in nearshore southern California ecosystems (Tegner and Levin, 1983; Robles, 1987; Eurich et al., 2014) and an economically valuable fisheries species (California Spiny Lobster Fisheries Management Plan, 2016). Spiny lobsters occupy a thermally heterogeneous environment, with temperatures ranging from approximately 9 to 30°C across their geographic range (effectively Point Conception, CA to Magdalena Bay, Baja California, Mexico) and seasonal fluctuations as large as 11.5°C in our focal region the Santa Barbara Channel (Aristizábal et al., 2016). To link physiology to predation, we measured a suite of physiological traits to explore possible drivers of predation rates that extend beyond resting metabolic rate. Specifically, we aimed to address the following questions: (i) How does temperature alter lobster resting metabolic rate and aerobic scope? (ii) How does temperature alter lobster predation rates? and (iii) How effectively does variation in metabolism predict variation in predation?
Materials and methods
We examined how temperature affects metabolic and predation rates of lobster using the following four steps: (i) we acclimated lobsters to one of four ecologically relevant temperatures (11, 16, 21, 26°C), (ii) we measured lobster resting and maximum metabolic rates using aquatic respirometry, and (iii) we measured lobster consumption rates of a mussel prey, Mytilus californianus.
Animal collection, husbandry, and acclimation
We collected 24 male lobsters (carapace length = 70.00 - 81.04 mm, wet mass = 302 – 466 g; Table S1) using Self Contained Underwater Breathing Aparatus (SCUBA) from nearshore kelp forests and rocky reefs in Santa Barbara county, CA. We maintained lobsters under a natural photoperiod (12 light:12 dark cycle) in tanks (76 cm L x 75 cm W x 30 cm H) divided by a perforated barrier with one lobster per half tank, each of which we stocked with one 6x8x16” and one 6x8x8” cinder block for shelter. We kept lobsters at ambient temperature (11°C) with flow-through filtered seawater for at least six days, during which we fed each individual with mussels (Mytilus spp.) ad libitum.
Our goal was to test the relationship between metabolism and predation at a range of temperatures consistent with the geographic distribution of spiny lobster. We therefore selected four experimental temperatures at which to acclimate lobsters: 11, 16, 21, and 26°C. The 11, 16, and 21°C treatments represent seasonal minimum, mean, and maximum temperatures in the coastal waters of the Santa Barbara Channel (Figure 1), while 26°C represents the near-maximum temperature approaching the southern end of the species range in Mexico (Table S2).
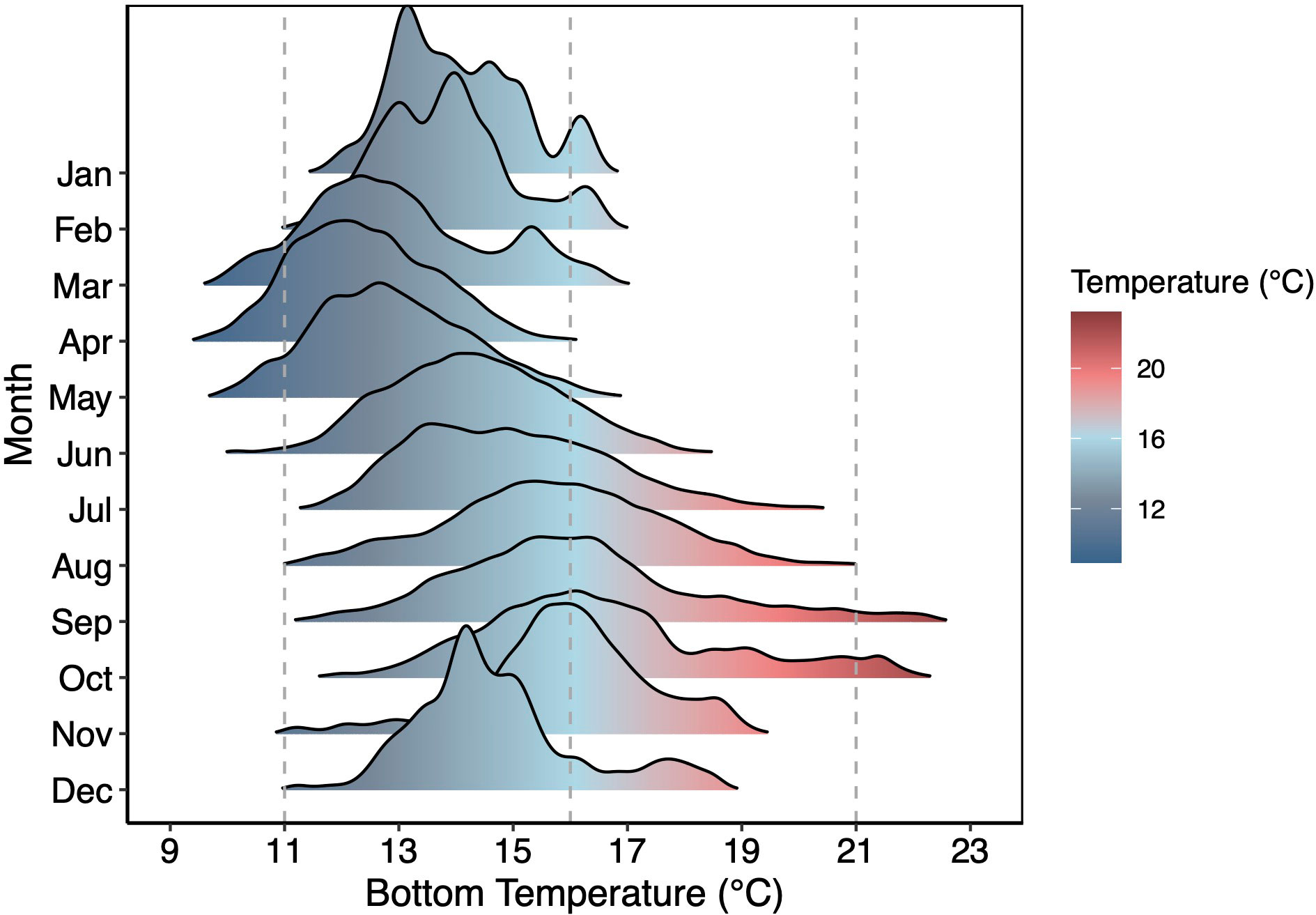
Figure 1 Temporal Variation in Bottom Temperature. Monthly bottom (4.5 m depth) temperatures at Mohawk Reef (34.396290, -119.731297) in Santa Barbara, CA compiled from 2005-2017. Vertical dashed lines represent three of four treatment temperatures (11, 16, 21°C). Data Source: Santa Barbara Coastal Long-Term Ecological Research group.
For treatments above ambient temperature (16, 21, 26°C), we heated flow-through seawater using Titanium heating tubes (Finnex, Chicago, IL, 500W) to maintain steady temperature at 16, 21, and 26°C in flow-through aquaria. For the one treatment consistently below ambient temperature (11°C), we chilled seawater to 11°C using a titanium chiller (AquaEuro Systems, Gardena, CA, ½ HP Max-Chill Titanium Chiller), which fed into an 80-gallon header tank. We distributed chilled water throughout experimental tanks via a partial recirculating system. We recorded temperature and sustained temperature using thermostats on regulating devices (chiller for 11°C, INKBIRD ITC-308 temperature Seabird controllers, Shenzhen, China). We recorded temperature at least twice a day using secondary measurements with a digital aquarium thermometer with a submersible probe. To manipulate temperature, we gradually acclimated lobsters by modifying the temperature by no more than 2°C day-1, well within daily temperature fluctuations experienced by lobsters in natural conditions. Once the target temperature was achieved, we held lobsters at stable focal treatment temperatures for 18-28 days. Aquarium water was fed from the salt water flow through conditions maintained by the Marine Science Institute of UC Santa Barbara. Due to aquaria space limitations, we ran temperature treatments in two separate rounds: (i) June 2018 – December 2018 (11 & 21°C treatments), and (ii) January 2019 – June 2019 (16 & 26°C treatments).
Measuring temperature-dependence in lobster metabolism
We used intermittent-flow respirometry (Svendsen et al., 2016) to measure oxygen consumption rates (MO2; mg O2 L-1 min-1) of temperature-acclimated lobsters. We submerged respirometers in a filtered seawater bath which was either heated or chilled to our experimental temperatures. To measure oxygen consumption rates, we placed lobsters in 17.9 L watertight plastic respirometers connected via tubes to two submersible pumps (Eheim, Deizisau, Germany, Universal 600 Aquarium Pump), which created both a flushing loop and a recirculation loop. We measured dissolved oxygen (DO) continuously in the recirculation loop using a fiber optic oxygen sensor (Firesting, PyroScience, Aachen, Germany). To limit movement throughout the trial, we provided lobsters with footholds (polystyrene eggcrate) at the bottoms of the respirometers and covered the seawater bath with shade cloth. We measured DO continuously in 23 min long cycles for the 16, 21, and 26°C treatments (8 min flush + 15 min measurement) and in 33 min long cycles for 11°C treatment (8 min flush + 25 min measurement). We calibrated our oxygen sensors before each trial using a 2 point calibration (0 was generated using sodium sulfite, 100% air-saturated water was generated using seawater vigorously aerated with an airstone). It was necessary to increase the measurement phase at the coldest temperature to capture sufficient declines in DO such that estimating MO2 was possible. DO within the respirometers did not drop below 80% air saturation for all experiments. To account for any background microbial respiration, we recorded at least one blank cycle (2 min flush + 10 min measurement) both before and after each trial.
To avoid increases in metabolism due to digestion (McCue, 2006), we fasted lobsters for 72 h prior to respirometry trials. We measured maximum metabolic rate and resting metabolic rate for each individual by first performing a standardized chase procedure to induce exhaustion. Here, we removed lobsters from their holding tanks for a 30 s air exposure, then hand-chased lobsters underwater for 30 s, repeating the process three times. This chase procedure was followed by a one min air exposure to ensure exhaustion (Norin and Clark, 2016) which we considered to be the point at which the animal ceased tail flapping, a common escape response (Bouwma & Herrnkind, 2009). We then transferred lobsters to the respirometers and quickly sealed them inside (<30 s). Lobsters were left in the respirometers overnight for 24 h, allowing them to return to resting levels of MO2.
Estimating lobster metabolic rates
We quality-controlled our metabolism data by visually examining all slopes of oxygen concentration over time. During some measurements, lobsters displayed periods of apnea, particularly at 11°C, discernable as extended periods with no change in oxygen concentration (McGaw et al., 2018). We excluded these periods from the slope measurement for MO2 (see Table S3). Only measurements that exhibit linear declines in mg O2 L-1 min-1 with an r2 > 0.85 for at least two minutes were used in the analysis.
We then calculated mass-specific and background respiration-corrected MO2 values using the equation:
where m1 and m2 are the rates of O2 decline (mg O2 L-1 min-1) over time during the measurement phase when a lobster is present and absent, respectively, V1 and V2 are the water volumes in the respirometer (L) when the lobster is present and absent, respectively, and M is the wet body mass of the lobster (kg). Body size (mass) of lobsters was constrained within a narrow range and did not have significant influence on metabolic rates. Therefore, using isometric metabolic scaling to express mass-specific metabolism is appropriate in our study. We calculated the rate of O2 decline attributable to background microbial respiration (m2) by averaging the MO2 values from blank measurements conducted both before and after an individual lobster’s trial. We estimated resting metabolic rate (mg O2 kg-1 min-1) as the lowest 15th percentile of measurements taken throughout the 24 h trial (Fitzgibbon, 2010; Fitzgibbon et al., 2014; Chabot et al., 2016; Twiname et al., 2020). Within the 24 h measurement period, the lobsters reached low and stable MO2 levels. While others have estimated standard metabolic rate in crustaceans off of<24 hours of MO2 measurements (Fitzgibbon et al., 2014; Twiname et al., 2020), it is possible that additional time in the respirometer would reveal even lower basal MO2 levels. As such, we termed this resting metabolic rate (RMR), not standard metabolic rate (SMR), though it is likely our estimates of RMR are only slightly elevated compared to true SMR. We estimated maximum metabolic rate as the fastest rate of linear O2 decline over a 60 s interval (Little et al., 2020), with an r2 greater than 0.90. We calculated absolute aerobic scope (AAS) as the difference between maximum metabolic rate and resting metabolic rate (AAS = MMR – RMR) for each lobster and factorial aerobic scope (FAS) as MMR/RMR.
Measuring temperature-dependence in lobster predation rates
The importance of prey density in modifying predator foraging behavior has a rich history in ecology (Holling, 1959). For example, shifts in a predator’s foraging behavior can affect the stability of predator–prey dynamics (DeAngelis et al., 1975), spatial distribution of predators (Meer and Ens, 1997), food chain length (Schmitz, 1992), and the strength of species interactions in complex food webs (Novak and Wootton, 2008). The functional response describes how the number of prey a predator eats increases as the density of prey increases (Stier et al., 2013); it represents mechanisms underlying the predator-prey interaction; thus, quantifying changes in the functional response with temperature is a natural way to test hypotheses and develop insight into how variation in temperature drives variation in predator-prey interactions. Estimating the functional response rather than simply measuring predation rates at a single prey density allows researchers to compare and model temperature-dependence in predator feeding rates across species and ecosystems.
To determine how increases in temperature altered lobster predation, we estimated the functional responses of temperature-acclimated lobsters by measuring the predation rates of each individual lobster on a common prey, Mytilus californianus (Robles, 1987) at five densities presented in sequential trials (5, 10, 20, 30, 60 mussels). We collected all mussels from shore-level rocks at Arroyo Burro Beach, in Santa Barbara, CA (34.403942°N, -119.742992°W). We constrained mussel sizes to 3.0-4.5 cm shell lengths, which is a preferred size for lobsters within our experimental size range (Robles et al., 1990). To standardize initial hunger levels of each individual, we fed lobsters ad libitum for 24 h then fasted them for 72 h prior to each trial. We introduced mussels to lobster holding tanks between 12:00 and 15:00, then removed and counted remaining intact mussels 24 h later. We replicated feeding assays three times per lobster at each of the five prey densities. Five lobsters (one at 11°C, one at 16°C, three at 21°C) molted during the feeding assays and stopped eating for 15-27 days during this period, as is common behavior in spiny lobsters (Lindberg, 1955). We maintained these individuals on the same feeding assay schedule and later repeated trials in which they did not feed. These repeated trials were substituted for any trials in which lobsters naturally fasted pre- and post-molt.
We modeled a type II functional response to estimate the per capita lobster feeding rate, F, as a function of prey density, N, F = αN/(1+αhN), where α and h describe a predator’s attack rate and handling time, respectively (Holling, 1959). In this model lobster consumption increases with prey density at lower densities, then eventually saturates. To estimate the functional response parameters (α and h) we used a Bayesian hierarchical model. Specifically, our model simultaneously estimated the relationship between mussel density and consumption rate at the population level (e.g. across all lobsters in the experiment), at the level of each temperature treatment (11, 16, 21, 26 °C), and for each individual lobster across replicated trials.
We assumed that the number of prey consumed (C) in trial (i) was binomially distributed given the number of prey offered (Ri) and the proportion of prey consumed (Pi). We estimated the proportion of prey consumed according to a type II functional response (Bolker, 2008),
Ci ~ Binomial (Ni, Pi)
where αL,k is the attack rate (predator-1 hr-1) of lobster L in temperature treatment T, and hL,T is the handling time (hours) of lobster L in temperature treatment T. Throughout the model hierarchy, we used diffuse, non-informative, normally distributed priors on the attack rate and handling time parameters and implemented the model in JAGS (Plummer, 2003). For more details on our Bayesian modeling approach see Text S1.
Estimating temperature sensitivity of metabolism and consumption
To assess changes in the thermal sensitivity (e.g. temperature dependence) of measured rates between the experimental temperatures, we calculated Q10, the factor by which a reaction rate changes over 10°C, as,
where R is a reaction rate (e.g., resting metabolic rate) and T is temperature (°C). However previous work has shown that Q10 may be temperature dependent (Gillooly et al., 2001). Therefore, we also fit phenomenological and mechanistic models to our rate data to provide direct comparison to theoretical predictions.
The metabolic theory of ecology predicts that biological rates, such as RMR, should increase exponential with temperature according to the Arrhenius equation. Furthermore, MTE hypothesizes that consumption rates should scale with metabolic demand, such that consumption increases with temperature at the same rate as metabolism (~0.6-0.7 eV (Gillooly et al., 2006; Allen and Gillooly, 2007). Following previous work (Gillooly et al., 2001; Rall et al., 2012), we therefore fit the Arrhenius equation to both the data on lobster RMR and max consumption rates, such that
Where k is Boltzmann’s constant (8.617 x 10-5 eV K-1), T is temperature (Kelvin), T0 is 273.15 and sets the intercept of the temperature relationship, and Ea is the activation energy of the reaction. To express all temperatures in °C, we allowed , where Tc is temperate in °C (Gillooly et al., 2001). We linearized eq. 4 and estimated the parameters (i0, Ea) using linear regression. In the case of Cmax, we resampled with replacement 50 draws from the posterior estimate for 1/h 1000 times to bootstrap the median and 95% CI’s of the relationship.
Unlike resting metabolic rate, previous work reveals that MMR should increase with temperature to a maximum before declining. Similarly, since RMR increases with temperature exponentially, absolute aerobic scope (MMR – RMR) should also follow a unimodal relationship with temperature. Therefore, to estimate the relationship between MMR or AAS and temperature we fit quadratic functions using regression approaches. We used simple linear regression to estimate the relationship between FAS and temperature.
To determine whether increasing predation rates could keep pace with increasing metabolic demand at higher temperatures, we then asked whether the effects of temperature on metabolism and the effects of temperature on predation scaled similarly.
Data analysis
We performed all analyses using R v3.6.3 and the following packages: nlme, tidybayes, R2jags, and rjags.
Results
As we increased temperature, lobster metabolism and predation rates increased. At the three lower temperature treatments (11, 16, 21°C) all lobsters survived, but acclimation to the hottest temperature (26°C) caused 33% mortality. Metabolism, and predation rate were correlated, but metabolism increased with temperature at a slower rate than predation. Below we detail how temperature affected lobster metabolism, and predation.
Temperature increased lobster metabolism
Increases in temperature had positive effects on lobster metabolism. Overall, resting metabolic rate increased by approximately 330% from the lowest to the highest temperature ( Q10SMR(11−26°C) = 2.65, Figure 2A; Table S4). Maximum metabolic rate and absolute aerobic scope increased to maxima at 21°C and declined to 26°C (Figures 2B, C; Table S5). Factorial aerobic scope (FAS) decreased with increasing temperature ranging from 14.5 ± 7.60 at 11°C to 4.80 ± 0.67 at 26°C (mean ± SE) (Figure 2D). However, there was substantial among individual variation in how lobsters’ metabolism responded to increases in temperature, with some animals exhibiting the capacity to maintain high aerobic scope at the lowest and highest temperatures while others had almost no aerobic scope.
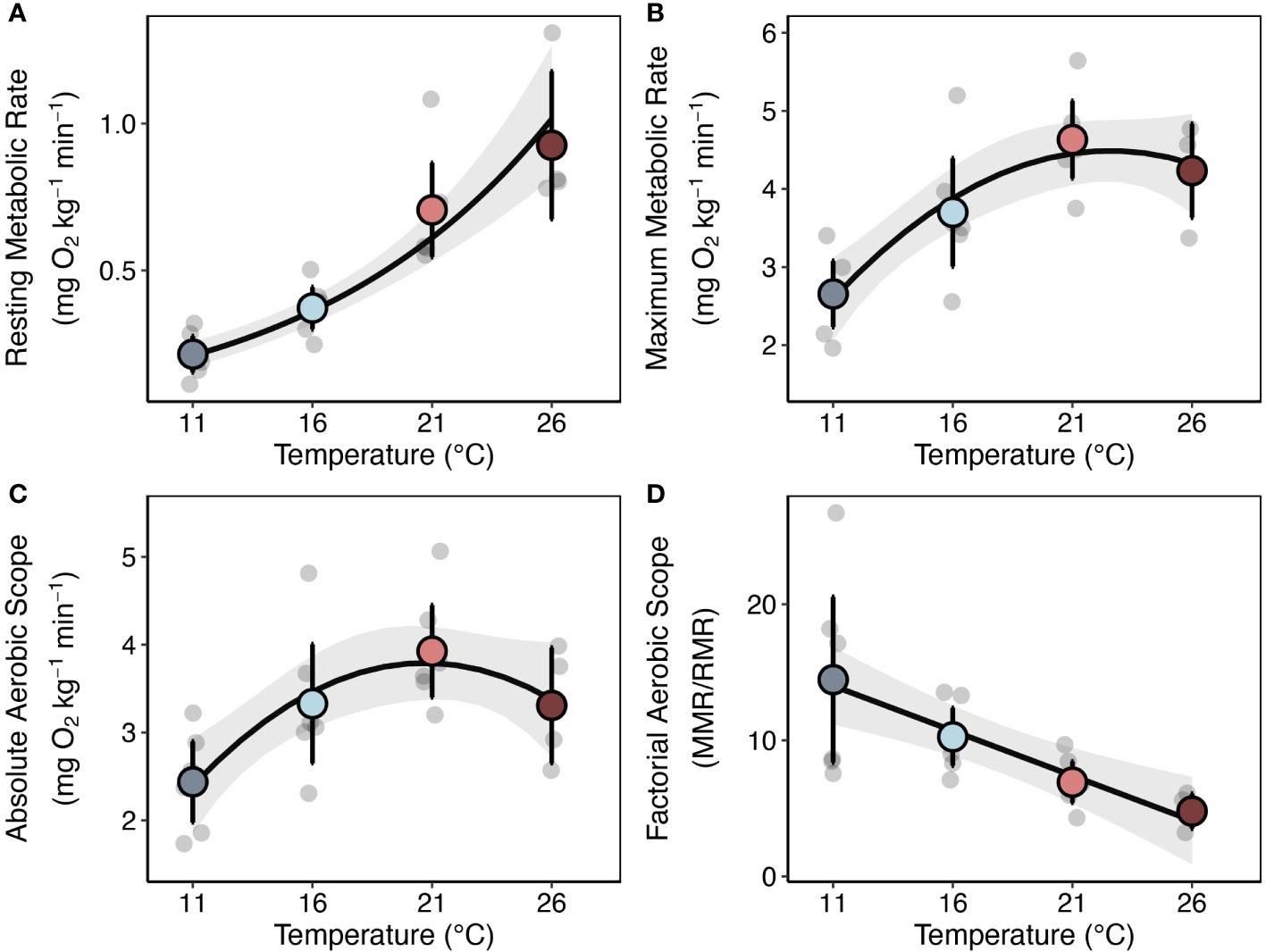
Figure 2 Temperature Effects on Lobster Metabolism. (A) Resting metabolic rates (RMR), (B) maximum metabolic rates (MMR), (C) absolute aerobic scopes (AAS = MMR – RMR), and (D) factorial aerobic scopes (FAS = MMR/RMR) measured for individual lobsters (light gray points) at 11, 16, 21, and 26°C (n = 6 for all treatments except 26°C, where n = 4). Colored points represent treatment means and bars are equal to 95% confidence intervals. Gray dots represent jittered raw data. Lines and gray shading are the mean ± 95% CI’s for fitted models. See Methods for details on the models fit to each rate.
Warming temperatures increase predation rates
Increases in temperature caused increases in lobster consumption rates across mussel densities (Figure 3). When mussels were at their maximum density, consumption rates increased 9.6-fold from the lowest (11°C) to the highest (26°C) temperature. Lobster predation was near zero in many of the trials in the coldest temperature treatment. In contrast, no fewer than two mussels were ever consumed by lobsters across all other temperature treatments when mussels were at maximum density.
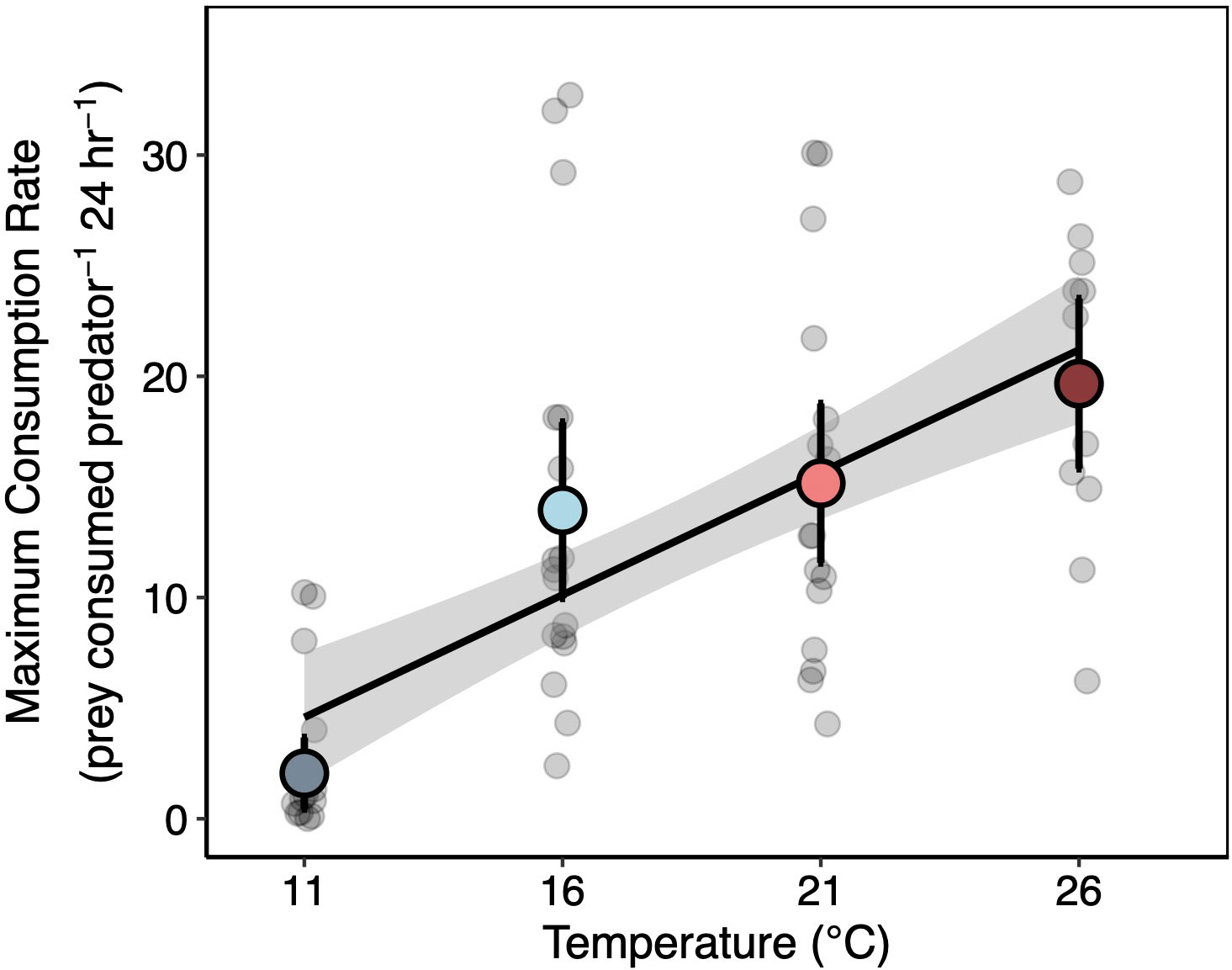
Figure 3 Temperature Effects on Lobster Functional Responses. Type II functional responses fit using a Bayesian hierarchical framework. Gray curves represent individual lobsters and colored curves represent treatment means (left to right: light blue = 11°C, dark blue = 16°C, light red = 21°C, dark red = 26°C). Solid gray regions highlight the 95% credible intervals estimated at the treatment level.
Similar to lobster metabolic responses to temperature, consumption rates varied widely among individuals within a given temperature treatment. For example, some individual lobsters ate more than twice as much as other lobsters within the same temperature treatment (Figure 3; Figure S1). One lobster individual in the 16°C exhibited anomalously high consumption rates that may have been linked to molting. Therefore, this lobster individual was dropped from the primary analysis (see Figure S3 for more details).
The differences in the lobster functional response with temperature were largely driven by increases in maximum consumption rates (e.g. 1/h; Figure 4). On average, the maximum consumption rate of lobsters was lowest at 11°C, similar at 16 and 21°C, and reached a maximum at 26°C (Figure 4). Attack rates were lower at the 11°C treatment that the other temperatures (Figures S1, S2). However, we found little evidence for variation in attack rates between 16 and 26°C.
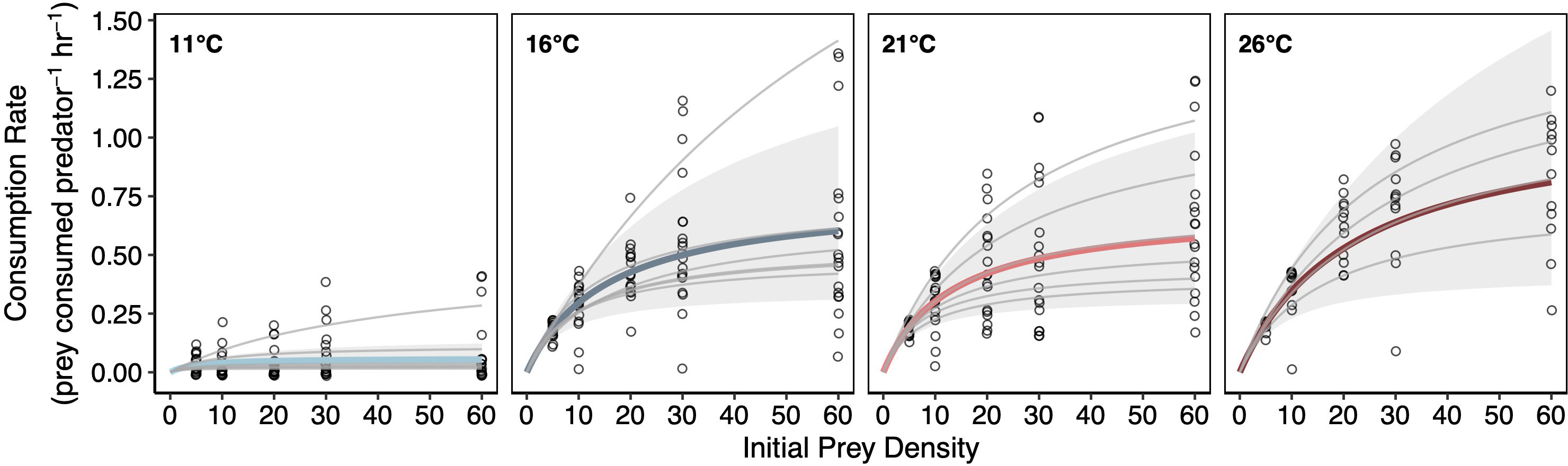
Figure 4 Temperature Effects on Lobster Maximum Consumption Rates. Median estimates of maximum consumption rates (Cmax) for each individual lobster (gray points in background) and lobsters in each temperature treatment (colored points). Colored points represent treatment medians ± 95% CI’s. Black line and surrounding shading are the median ± 95% CI of the bootstrapped regression relationship between temperature and maximum consumption rate fit to the treatment-level posterior estimates. Individual median estimates (gray points) are jittered for clarity.
Variation in predator metabolism predicts variation in predator feeding
Nearly all metabolic rates were positively correlated with predation rates. Lobster resting metabolic rate, maximum metabolic rate, and absolute aerobic scope exhibited positive relationships with maximum consumption rates across temperature (Figure 5). The 11°C-acclimated lobsters had consistently low resting metabolic rates and maximum metabolic rates, small absolute aerobic scopes, and low maximum consumption rates (Figure 5). However, for the other temperature treatments, lobsters exhibited substantial among individual variation in temperature dependence (Figure 5). For example, one 11°C-acclimated lobster exhibited a below-average resting metabolic rate, but an above-average maximum metabolic rate and absolute aerobic scope as compared to other individuals within that treatment. With low maintenance costs (resting metabolic rates) and a large remaining energy budget (absolute aerobic scope, factorial aerobic scope), this individual was able to maintain consumption rates similar to those observed in warmer temperature treatments (Figure 5, denoted by arrows). On the contrary, one 26°C-acclimated lobster had the highest resting metabolic rates, but a below average maximum metabolic rate, absolute aerobic scope, and maximum consumption rate compared to other individuals within the 26°C treatment (Figure 5, denoted by arrows).
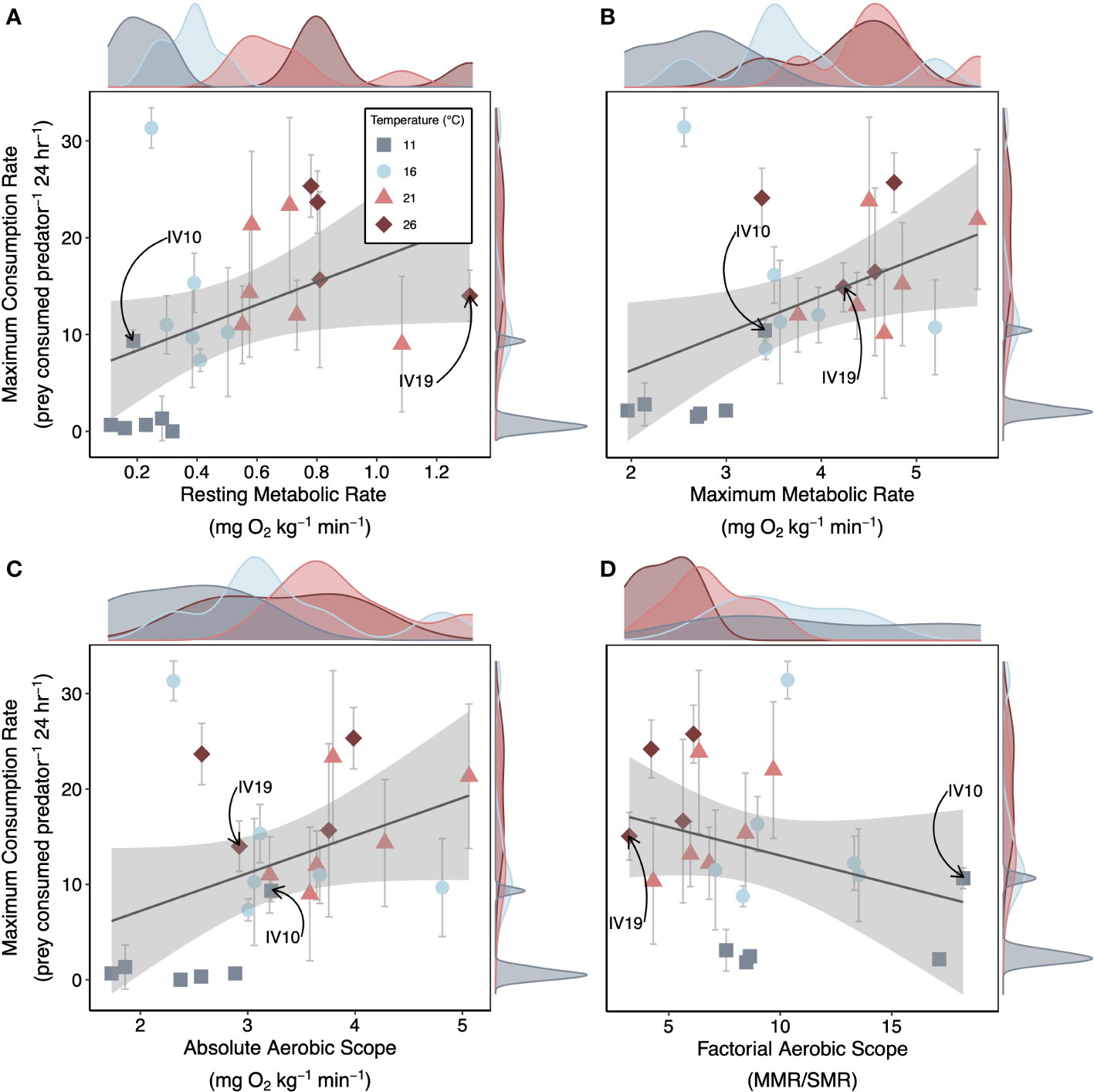
Figure 5 Metabolism Effects on Foraging Across Temperatures. Median posterior estimates for the maximum consumption rate (Cmax) of each individual lobster plotted as a function of the individuals (A) resting metabolic rate, (B) maximum metabolic rate, (C) absolute aerobic scope and (D) factorial aerobic scope across temperatures. Gray error bars are the 95% CI’s of the median estimate from the posterior distribution. Lines and gray shading are mean linear trends and 95% CI’s from simple linear regressions fit to the median estimates of Cmax. Density plots show the distribution of metabolic traits (x-axis) and Cmax (y-axis) for each treatment. We provide an example of an individual we characterized as an overperformer (IV10; 11°C treatment) and as an underperformer (IV19; 26°C treatment) as compared to treatment means.
Activation energies fall within the range predicted by metabolic theory of ecology
While metabolism and predation were both affected by temperature, the effects of temperature on metabolism were weaker than the effects of temperature on predation. The scaling exponent of lobster resting metabolic rates fell at the upper end of the range predicted by metabolic theory of ecology (MTE-predicted: 0.6-0.7 eV; Observed: EaSMR = 0.77 ± 0.09 (Gillooly et al., 2006; Allen and Gillooly, 2007). However, the scaling exponent of maximum consumption rates was substantially higher than measured in resting metabolic rate or predicted by the metabolic theory of ecology (EaC = 1.33 [1.26 – 1.42], X̅ [95% CI], Table S4). Thus, the resting metabolic rate of lobsters increased with temperature ~60% slower than maximum consumption rate increased with temperature, challenging the theoretical prediction that temperature drives proportional increases in resting metabolic rate and predation.
Discussion
Whether temperature-dependence in feeding rates match or mismatch with temperature-dependence in metabolic rates is key to anticipating the impacts of warming on ecosystem stability and dynamics (Lindmark et al., 2019). Here we offer one of the few integrative studies that explicitly link temperature-dependence in metabolism with temperature-dependence in predator-prey interactions. Our results indicate that metabolic, and predation rates increase as functions of temperature, but show that predation rates increased at a faster rate with temperature than metabolism. This capacity to maintain high foraging rates as temperatures increased suggests that some species may not immediately suffer from a bioenergetic meltdown caused by declining energy intake and increased energy expenditure. Yet at the coldest temperatures lobsters were barely ate, and at the hottest temperatures lobsters had high mortality, which highlights the existence of thermal limits. Below we discuss the similarities and differences of our results from existing research on temperature-dependence, speculate further on the link between metabolism and predation, and offer insights into how our findings may have implications for lobster biogeography and ecology.
Linking temperature-dependence in spiny lobsters to temperature-dependence in other systems
Temperature-dependence of spiny lobsters has both similarities and differences with existing theoretical and empirical literature on temperature-dependence in other systems. For example our study adds to a plethora of evidence that have shown increases in temperature cause increases in consumption rates (Englund et al., 2011; Rall et al., 2012; Sohlström et al., 2021; Lindmark et al., 2022). Overall, increases in maximum consumption rates as temperatures increase typically increase the capacity of predators to limit prey populations (Vasseur and McCann, 2005), though the population dynamic consequences in this system remain unclear without a better understanding of the relative impacts of temperature on other key demographic parameters for both lobsters and their mussel prey (e.g., population growth rate or dispersal).
Temperature-dependence in predation rates in other systems are usually driven by simultaneous decreases in handling times and increases in attack rates as temperatures warm. However, in spiny lobsters we found that increases in temperature had very limited effect on attack rate, but that increases in temperature caused large increases in maximum consumption rates. In other words, increases in temperature decreased the time it took lobsters to manipulate, consume, or digest mussels allowing lobsters to consume more per unit time, but temperature did not affect how long it took for lobsters to search for mussel prey. Two potential explanations exist for the lack of temperature-sensitivity in attack rate. First, thermal sensitivity can depend on prey mobility, with lower thermal sensitivity for sedentary prey (Vucic-Pestic et al., 2011). Second, searching for prey was relatively simple inside the experimental arena. This may bias laboratory attack rates, but is not particularly different from field conditions where prey such as urchins and mussels are readily available and nearby lobsters in many sites.
Should metabolism and predation be more strongly correlated?
All measures of metabolism were positively correlated with maximum consumption rates, but maximum metabolic rate was the strongest predictor. This suggests that baseline maintenance requirements may not be solely driving consumption, instead maximum capacity may also be driving consumption. Physiologists studying other processes have similarly found utility in metabolic measurements beyond resting metabolic rate (e.g. Brownscombe et al., 2017; Prystay et al., 2017; Little et al., 2020), and ecologists interested in physiological mechanisms of behavior will continue to benefit from similar diverse measurements of animal metabolism.
An outstanding question is what underlies the unexplained variation in maximum consumption rates that is not explained by variation in metabolism. In this study, the greatest source of variation in the metabolism-predation relationship was among individual variation. Such variation in temperature dependence makes it difficult to detect mean effects of temperature and suggests that in addition to environmental temperature, phenotypic plasticity, genetics (Somero, 2009; Razgour et al., 2019), behavioral diversity, or even maternal effects (e.g., egg size Régnier et al., 2012) may explain significant variation in lobster consumption rates. Future work should examine the high inter-individual variability in decapod physiological rates, particularly via longer measurements (>24 h) and ideally measuring free-ranging animals using loggers and/or telemetry (e.g., heart rate and accelerometery; McGaw and Nancollas, 2018; McGaw et al., 2018; Steell et al., 2020; McGaw and Nancollas, 2021).
Insights from temperature dependence for effects of seasonality and lobster biogeographic range
Our results also suggest that temperature plays a key role in limiting lobster foraging activity throughout seasonal temperature shifts. Such temperature-dependence helps us better understand lobster biogeography, with different mechanisms limiting lobsters at low and high temperatures. Low temperatures appear to impose an inflexible floor for lobsters, suppressing physiological function and constraining foraging activity, despite their presumed history withstanding bottom temperatures that frequently approach and even drop below 11°C (Figure 1) in the Santa Barbara Channel. These physiological limits are linked to long pauses in lobster breathing and significant reductions in metabolism. Although we did not observe mortality associated with our coldest temperature treatment, it is likely that with any further decreases in resting metabolic rate driven by decreases in temperature, intake through any prey source would be unlikely to be able to sustain tissue function and mortality or reproductive failure would ensue.
Spiny lobsters in the Santa Barbara Channel seem to be surviving near their physiological lower thermal limits from March to May in the Santa Barbara channel (Figure 1), during which they may have a low or negligible impact on prey communities. Similarly, seasonal low temperature periods, coastal upwelling, or La Niña events should then drive reductions in lobster-mussel interaction strengths at a local scale (sensu Sanford, 1999). At larger spatial scales, the per capita impact of spiny lobster on prey may decrease with increasing latitude due to decreasing mean annual sea surface temperature. Constraints of low temperature on lobster metabolic and feeding rates likely sets the spiny lobster northern range which typically stops at Point Conception where coastal regions experience longer and more frequent upwelling events than in the Santa Barbara Channel, often experiencing sustained periods below 11°C (Huyer, 1983; Watson et al., 2011). Lobsters limited function in cold water likely explains the infrequent sightings of large spiny lobster populations in the significantly cooler waters north of Point Conception, CA, with the exception of during warming events (Leising et al., 2015). As ocean water continues to warm, it is possible we will observe a persistent northward expansion of the range of generalist spiny lobsters.
Similar insights into seasonality and biogeography emerge from consideration of lobster physiology and feeding behavior at warmer temperatures. By maximizing the thermal sensitivity (Q10) of metabolism at the lowest end of their ambient temperature range lobsters have reduced maintenance costs when temperatures are too low to support active foraging but also rapidly capitalize on increases in temperature that promote sustained activity, a pattern familiar from studies in other taxa (Naya et al., 2009; Kreiman et al., 2019). As temperatures warm, lobsters are able to recover 91.4% of their maximum consumption capacity when temperatures increase from 11 to 16°C. This increase in lobster maximum consumption rates with temperature reflect faster biochemical reaction rates and increased oxygen demands.
During the spring and summer months, or high temperature periods such as El Niños, lobsters are likely to have their highest metabolic demands and will therefore also exert their strongest effects on prey populations (sensu Carr and Bruno, 2013). Although these high foraging rates at high temperatures are like coupled with disproportionate physiological costs of homeostasis. While the population genetics of lobsters is an active area of research, previous studies suggest that lobster populations are relatively well-mixed on the west coast of California and Mexico (Iacchei et al., 2013), which may influence capacity for local adaptation. We can therefore speculate that if lobsters living in the southern range respond similarly to the hottest 26°C treatment in this study, that temperatures that are persistently 26°C or higher are likely to leave lobsters with little energetic capacity to perform other critical fitness-enhancing processes, such as escaping predators, growth and reproduction. Therefore, a deeper understanding of the link between physiology and ecology of lobsters throughout their range as well as consideration of local adaptation and plasticity, will offer important insight into the likelihood of future range contractions or shifts in the ecological role of lobsters as waters warm.
Conclusion
The better we understand why animals experience temperature dependence in their physiology or ecology, the better we will be able to predict how natural and human-induced changes in temperature are likely to affect the stability and function of ecosystems. Our study provides evidence that at least some species can maintain high feeding rates to meet the higher energetic demands associated with higher temperatures. We also show how cold constraints can fully stop predators from functioning physiologically and ecologically, suggesting that the strength of predation is likely highly seasonal. Lastly, while we link temperature, metabolism, and predation, we also demonstrate that, at least for lobsters, a significant amount of temperature-dependence in predation is explained by individual variation driven by other non-metabolic biological processes that are currently unknown. Identifying these unknown forces is critical to evaluate and fully predict how temperature alters the strength species interactions, drives range shifts, and alters animal bioenergetics.
Data availability statement
The datasets presented in this study can be found in online repositories. The names of the repository/repositories and accession number(s) can be found below: https://github.com/stier-lab/Csik-etal-Functional-Ecology.
Author contributions
SC, AS, and EE conceived the ideas; SC, KK, JC, EH, AS, and EE designed methodology; SC collected the data; SC, KK, BD, and JC analyzed the data; SC and AS led the writing of the manuscript. All authors contributed to the article and approved the submitted version.
Funding
This work was funded by the California Sea Grant Prop 84 grant program (R/OPCOAH-2), the NSF GRFP, and the Santa Barbara Coastal Long-Term Ecological Research program (NSF OCE 1831937).
Acknowledgments
We thank numerous undergraduate researchers who cared for the lobsters and mussels used in this study, and C. Nelson, S. Sampson, S. Harrer, and the rest of the SBC LTER research team for their commitment in collecting long-term ecological data.
Conflict of interest
The authors declare that the research was conducted in the absence of any commercial or financial relationships that could be construed as a potential conflict of interest.
Publisher’s note
All claims expressed in this article are solely those of the authors and do not necessarily represent those of their affiliated organizations, or those of the publisher, the editors and the reviewers. Any product that may be evaluated in this article, or claim that may be made by its manufacturer, is not guaranteed or endorsed by the publisher.
Supplementary material
The Supplementary Material for this article can be found online at: https://www.frontiersin.org/articles/10.3389/fmars.2023.1072807/full#supplementary-material
References
Allen A. P., Gillooly J. F. (2007). The mechanistic basis of the metabolic theory of ecology. Oikos 116, 1073–1077. doi: 10.1111/j.2007.0030-1299.16079.x
Aristizábal M. F., Fewings M. R., Washburn L. (2016). Contrasting spatial patterns in the diurnal and semidiurnal temperature variability in the Santa Barbara channel, California. J. Geophys. Res. Oceans 121, 427–440. doi: 10.1002/2015JC011239
Auer S. K., Salin K., Anderson G. J., Metcalfe N. B. (2015). Aerobic scope explains individual variation in feeding capacity. Biol. Lett. 11, 20150793. doi: 10.1098/rsbl.2015.0793
Biro P. A., Stamps J. A. (2010). Do consistent individual differences in metabolic rate promote consistent individual differences in behavior? Trends Ecol. Evol. 25, 653–659. doi: 10.1016/j.tree.2010.08.003
Bouwma P. E., Herrnkind W. F. (2009). Sound production in Caribbean spiny lobster Panulirus argus and its role in escape during predatory attack by Octopus briareus. New Zealand Journal of Marine and Freshwater Research 43, 3–13. doi: 10.1080/00288330909509977
Brown J. H., Gillooly J. F., Allen A. P., Savage V. M., West G. B. (2004). Toward a metabolic theory of ecology. Ecology 85, 1771–1789. doi: 10.1890/03-9000
Brownscombe J. W., Cooke S. J., Danylchuk A. J. (2017). Spatiotemporal drivers of energy expenditure in a coastal marine fish. Oecologia 183, 689–699. doi: 10.1007/s00442-016-3800-5
Bruno J. F., Carr L. A., O’Connor M. I. (2015). Exploring the role of temperature in the ocean through metabolic scaling. Ecology 96, 3126–3140. doi: 10.1890/14-1954.1
California Spiny lobster fisheries management plan. (2016). Available at: https://wildlife.ca.gov/Conservation/Marine/Lobster-FMP.
Carr L. A., Bruno J. F. (2013). Warming increases the top-down effects and metabolism of a subtidal herbivore. PeerJ 1, e109. doi: 10.7717/peerj.109
Chabot D., Steffensen J. F., Farrell A. P. (2016). The determination of standard metabolic rate in fishes. J. Fish Biol. 88, 81–121. doi: 10.1111/jfb.12845
Chiabai A., Quiroga S., Martinez-Juarez P., Higgins S., Taylor T. (2018). The nexus between climate change, ecosystem services and human health: Towards a conceptual framework. Sci. Total Environ. 635, 1191–1204. doi: 10.1016/j.scitotenv.2018.03.323
Clarke A. (2004). Is there a universal temperature dependence of metabolism? Funct. Ecol. 18, 252–256. doi: 10.1111/j.0269-8463.2004.00842.x
DeAngelis D. L., Goldstein R. A., O’Neill R. V. (1975). A model for tropic interaction. Ecology 56, 881–892. doi: 10.2307/1936298
Eliason E. J., Farrell A. P. (2016). Oxygen uptake in pacific salmon oncorhynchus spp.: when ecology and physiology meet. J. Fish Biol. 88, 359–388. doi: 10.1111/jfb.12790
Englund G., Öhlund G., Hein C. L., Diehl S. (2011). Temperature dependence of the functional response: temperature dependence of consumption. Ecol. Lett. 14, 914–921. doi: 10.1111/j.1461-0248.2011.01661.x
Eurich J. G., Selden R. L., Warner R. R. (2014). California Spiny lobster preference for urchins from kelp forests: implications for urchin barren persistence. Mar. Ecol. Prog. Ser. 498, 217–225. doi: 10.3354/meps10643
Fitzgibbon Q. P. (2010). Standard metabolic rate of spiny lobster (Sagmariasus verreauxi) pueruli determined by intermittent flow-through respirometry. J. Mar. Biol. Assoc. India 52(2):264–273.
Fitzgibbon Q. P., Ruff N., Tracey S. R., Battaglene S. C. (2014). Thermal tolerance of the nektonic puerulus stage of spiny lobsters and implications of ocean warming. Mar. Ecol. Prog. Ser. 515, 173–186. doi: 10.3354/meps10979
Gilbert B., Tunney T. D., McCann K. S., DeLong J. P., Vasseur D. A., Savage V., et al. (2014). A bioenergetic framework for the temperature dependence of trophic interactions. Ecol. Lett. 17, 902–914. doi: 10.1111/ele.12307
Gillooly J. F., Allen A. P., Savage V. M., Charnov E. L., West G. B., Brown J. H. (2006). Response to Clarke and Fraser: effects of temperature on metabolic rate. Funct. Ecol. 20, 400–404. doi: 10.1111/j.1365-2435.2006.01110.x
Gillooly J. F., Brown J. H., West G. B., Savage V. M., Charnov E. L. (2001). Effects of size and temperature on metabolic rate. Science 293, 2248–2251. doi: 10.1126/science.1061967
Holling C. S. (1959). The components of predation as revealed by a study of small-mammal predation of the European pine sawfly. Can. Entomologist 91, 293–320. doi: 10.4039/Ent91293-5
Huey R. B., Kingsolver J. G. (2019). Climate warming, resource availability, and the metabolic meltdown of ectotherms. Am. Nat. 194, E140–E150. doi: 10.1086/705679
Hulbert A. J., Else P. L. (2000). Mechanisms underlying the cost of living in animals. Annu. Rev. Physiol. 62, 207–235. doi: 10.1146/annurev.physiol.62.1.207
Huyer A. (1983). Coastal upwelling in the California current system. Prog. Oceanography 12, 259–284. doi: 10.1016/0079-6611(83)90010-1
Iacchei M., Ben-Horin T., Selkoe K. A., Bird C. E., García-Rodríguez F. J., Toonen R. J. (2013). Combined analyses of kinship and FST suggest potential drivers of chaotic genetic patchiness in high gene-flow populations. Mol. Ecol. 22, 3476–3494. doi: 10.1111/mec.12341
Killen S. S., Brown J. A., Gamperl A. K. (2007). The effect of prey density on foraging mode selection in juvenile lumpfish: Balancing food intake with the metabolic cost of foraging. J. Anim. Ecol. 76, 814–825. doi: 10.1111/j.1365-2656.2007.01237.x
Killen S. S., Glazier D. S., Rezende E. L., Clark T. D., Atkinson D., Willener A. S. T., et al. (2016). Ecological influences and morphological correlates of resting and maximal metabolic rates across teleost fish species. Am. Nat. 187, 592–606. doi: 10.1086/685893
Kordas R. L., Harley C. D. G., O’Connor M. I. (2011). Community ecology in a warming world: The influence of temperature on interspecific interactions in marine systems. J. Exp. Mar. Biol. Ecol. 400, 218–226. doi: 10.1016/j.jembe.2011.02.029
Kreiman L. E., Solano-Iguaran J. J., Bacigalupe L. D., Naya D. E. (2019). Testing the metabolic homeostasis hypothesis in amphibians. Phil. Trans. R. Soc B 374, 20180544. doi: 10.1098/rstb.2018.0544
Laska M. S., Wootton J. T. (1998). Theoretical concepts and empirical approaches to measuring interaction strength. Ecology 79, 461–476. doi: 10.1890/0012-9658(1998)079[0461:TCAEAT]2.0.CO;2
Leising A. W., Schroeder I. D., Bograd S. J., Abell J., Durazo R., Gaxiola-Castro G., et al. (2015). State of the California current 2014-15: Impacts of the warm-water “Blob” (California: California Cooperative Oceanic Fisheries Investigations Reports) 56, 31–68.
Lindberg R. G. (1955). Growth, population dynamics, and field behavior in the spiny lobster, panulirus interruptus (Randall). Univ. California Publications Zoölogy 59, 157–248.
Lindmark M., Ohlberger J., Gårdmark A. (2022). Optimum growth temperature declines with body size within fish species. Global Change Biol. 28, 2259–2271. doi: 10.1111/gcb.16067
Lindmark M., Ohlberger J., Huss M., Gårdmark A. (2019). Size-based ecological interactions drive food web responses to climate warming. Ecol. Lett. 22, 778–786. doi: 10.1111/ele.13235
Little A. G., Dressler T., Kraskura K., Hardison E., Hendriks B., Prystay T., et al. (2020). Maxed out: optimizing accuracy, precision, and power for field measures of maximum metabolic rate in fshes. Physiol. Biochem. Zoology 93, 243–254. doi: 10.1086/708673
Marras S., Claireaux G., McKenzie D. J., Nelson J. A. (2010). Individual variation and repeatability in aerobic and anaerobic swimming performance of European sea bass, dicentrarchus labrax. J. Exp. Biol. 213, 26–32. doi: 10.1242/jeb.032136
McCue M. D. (2006). Specific dynamic action: A century of investigation. Comp. Biochem. Physiol. Part A: Mol. Integr. Physiol. 144, 381–394. doi: 10.1016/j.cbpa.2006.03.011
McGaw I. J., Nancollas S. J. (2018). Experimental setup influences the cardiovascular responses of decapod crustaceans to environmental change. Can. J. Zool. 96, 1043–1052. doi: 10.1139/cjz-2017-0252
McGaw I. J., Nancollas S. J. (2021). Patterns of heart rate and cardiac pausing in unrestrained resting decapod crustaceans. J. Exp. Zoology Part A: Ecol. Integr. Physiol. 335, 678–690. doi: 10.1002/jez.2533
McGaw I. J., Steell S. C., Leeuwen T. E. V., Eliason E. J., Cooke S. J. (2018). Application of miniature heart rate data loggers for use in Large free-moving decapod crustaceans: Method development and validation. Physiol. Biochem. Zoology 91, 731–739. doi: 10.1086/695839
Meer J. V. D., Ens B. J. (1997). Models of interference and their consequences for the spatial distribution of ideal and free predators. J. Anim. Ecol. 66, 846–858. doi: 10.2307/6000
Naya D. E., Veloso C., Sabat P., Bozinovic F. (2009). The effect of short- and long-term fasting on digestive and metabolic flexibility in the Andean toad, bufo spinulosus. J. Exp. Biol. 212, 2167–2175. doi: 10.1242/jeb.030650
Norin T., Clark T. D. (2016). Measurement and relevance of maximum metabolic rate in fishes: maximum metabolic rate in fishes. J. Fish Biol. 88, 122–151. doi: 10.1111/jfb.12796
Novak M., Wootton J. T. (2008). Estimating nonlinear interaction strengths: An observation-based method for species-rich food webs. Ecology 89, 2083–2089. doi: 10.1890/08-0033.1
Plummer M. (2003). “JAGS: A program for analysis of Bayesian graphical models using Gibbs sampling,” in Proceedings of the 3rd International Workshop on Distributed Statistical Computing (Vienna) Vol. 8, 1–10.
Prystay T. S., Eliason E. J., Lawrence M. J., Dick M., Brownscombe J. W., Patterson D. A., et al. (2017). The influence of water temperature on sockeye salmon heart rate recovery following simulated fisheries interactions. Conserv. Physiol. 5 1–12. doi: 10.1093/conphys/cox050
Rall B. C., Brose U., Hartvig M., Kalinkat G., Schwarzmüller F., Vucic-Pestic O., et al. (2012). Universal temperature and body-mass scaling of feeding rates. Phil. Trans. R. Soc B 367, 2923–2934. doi: 10.1098/rstb.2012.0242
Rall B. C., Vucic-Pestic O., Ehnes R. B., Emmerson M., Brose U. (2010). Temperature, predator-prey interaction strength and population stability. Global Change Biol. 16, 2145–2157. doi: 10.1111/j.1365-2486.2009.02124.x
Razgour O., Forester B., Taggart J. B., Bekaert M., Juste J., Ibáñez C., et al. (2019). Considering adaptive genetic variation in climate change vulnerability assessment reduces species range loss projections. Proc. Natl. Acad. Sci. U.S.A. 116, 10418–10423. doi: 10.1073/pnas.1820663116
Régnier T., Bolliet V., Gaudin P., Labonne J. (2012). Female effects on offspring energetic status and consequences on early development in yolk feeding brown trout (Salmo trutta). J. Exp. Zool. 317, 347–358. doi: 10.1002/jez.1728
Robles C. (1987). Predator foraging characteristics and prey population structure on a sheltered shore. Ecology 68, 1502–1514. doi: 10.2307/1939234
Robles C., Sweetnam D., Eminike J. (1990). Lobster predation on mussels: shore-level differences in prey vulnerability and predator preference. Ecology 71, 1564–1577. doi: 10.2307/1938292
Sanford E. (1999). Regulation of keystone predation by small changes in ocean temperature. Science 283, 2095–2097. doi: 10.1126/science.283.5410.2095
Schmitz O. J. (1992). Exploitation in model food chains with mechanistic consumer-resource dynamics. Theor. Population Biol. 41, 161–183. doi: 10.1016/0040-5809(92)90042-R
Sohlström E. H., Archer L. C., Gallo B., Jochum M., Kordas R. L., Rall B. C., et al. (2021). Thermal acclimation increases the stability of a predator–prey interaction in warmer environments. Global Change Biol. 27, 3765–3778. doi: 10.1111/gcb.15715
Somero G. N. (2009). The physiology of climate change: how potentials for acclimatization and genetic adaptation will determine “winners” and “losers”. J. Exp. Biol. 213, 912–920. doi: 10.1242/jeb.037473
Steell S. C., Cooke S. J., Eliason E. J. (2020). Artificial light at night does not alter heart rate or locomotor behaviour in Caribbean spiny lobster (Panulirus argus): Insights into light pollution and physiological disturbance using biologgers. Conserv. Physiol. 8, coaa097. doi: 10.1093/conphys/coaa097
Stier A. C., Geange S. W., Bolker B. M. (2013). Predator density and competition modify the benefits of group formation in a shoaling reef fish. Oikos 122, 171–178. doi: 10.1111/j.1600-0706.2012.20726.x
Svendsen M. B. S., Bushnell P. G., Christensen E. A. F., Steffensen J. F. (2016). Sources of variation in oxygen consumption of aquatic animals demonstrated by simulated constant oxygen consumption and respirometers of different sizes. J. Fish Biol. 88, 51–64. doi: 10.1111/jfb.12851
Tegner M. J., Levin L. A. (1983). Spiny lobsters and sea urchins: analysis of a predator-prey interaction. J. Exp. Mar. Biol. Ecol. 73, 125–150. doi: 10.1016/0022-0981(83)90079-5
Twiname S., Fitzgibbon Q. P., Hobday A. J., Carter C. G., Oellermann M., Pecl G. T. (2020). Mismatch of thermal optima between performance measures, life stages and species of spiny lobster. Sci. Rep. 10, 21235. doi: 10.1038/s41598-020-78052-4
Vasseur D. A., McCann K. S. (2005). A mechanistic approach for modeling temperature-dependent consumer-resource dynamics. Am. Nat. 166, 184–198. doi: 10.1086/431285
Vucic-Pestic O., Ehnes R. B., Rall B. C., Brose U. (2011). Warming up the system: higher predator feeding rates but lower energetic efficiencies. Global Change Biol. 17, 1301–1310. doi: 10.1111/j.1365-2486.2010.02329.x
Warne R. W., Baer S. G., Boyles J. G. (2019). Community physiological ecology. Trends Ecol. Evol. 34, 510–518. doi: 10.1016/j.tree.2019.02.002
Keywords: functional response, predator-prey interactions, metabolic theory of ecology, Panulirus interruptus, temperature dependence, metabolism
Citation: Csik SR, DiFiore BP, Kraskura K, Hardison EA, Curtis JS, Eliason EJ and Stier AC (2023) The metabolic underpinnings of temperature-dependent predation in a key marine predator. Front. Mar. Sci. 10:1072807. doi: 10.3389/fmars.2023.1072807
Received: 17 October 2022; Accepted: 12 January 2023;
Published: 07 February 2023.
Edited by:
Gisela Lannig, Alfred Wegener Institute Helmholtz Centre for Polar and Marine Research (AWI), GermanyReviewed by:
Bastian Maus, University Hospital Münster, GermanyYouji Wang, Shanghai Ocean University, China
Copyright © 2023 Csik, DiFiore, Kraskura, Hardison, Curtis, Eliason and Stier. This is an open-access article distributed under the terms of the Creative Commons Attribution License (CC BY). The use, distribution or reproduction in other forums is permitted, provided the original author(s) and the copyright owner(s) are credited and that the original publication in this journal is cited, in accordance with accepted academic practice. No use, distribution or reproduction is permitted which does not comply with these terms.
*Correspondence: Adrian C. Stier, YXN0aWVyQHVjc2IuZWR1