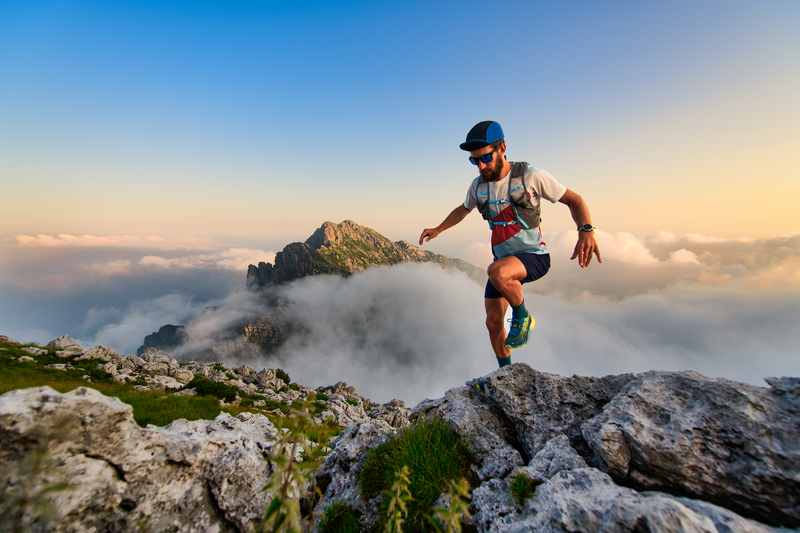
95% of researchers rate our articles as excellent or good
Learn more about the work of our research integrity team to safeguard the quality of each article we publish.
Find out more
MINI REVIEW article
Front. Mar. Sci. , 20 January 2023
Sec. Aquatic Physiology
Volume 10 - 2023 | https://doi.org/10.3389/fmars.2023.1066929
This article is part of the Research Topic Insights in Aquatic Physiology: 2021 View all 16 articles
How teleosts take up Na+ from the surrounding freshwater (FW) as well as the underlying mechanisms associated with this process have received considerable attention over the past 85 years. Owing to an enormous ion gradient between hypotonic FW and fish body fluids, teleosts gills have to actively absorb Na+ (via ionocytes) to compensate for the passive loss of Na+. To date, three models have been proposed for Na+ uptake in teleost ionocytes, including Na+/H+ exchanger (NHE)-mediated, acid-sensing ion channel (ASIC)-mediated, Na+-Cl- co-transporter (NCC)-mediated pathways. However, some debates regarding these models and unclear mechanisms still remain. To better understand how teleosts take up Na+ from FW, this mini-review summarizes the main progress and related regulatory mechanisms of Na+ uptake, and discusses some of the challenges to the current models.
Body fluid Na+ homeostasis is pivotal for maintaining proper cell activities and physiological processes. In teleosts, the principal organs for ion exchange are the gills (and larval skin), which function via the large surface of the epithelium that is directly exposed to water. Regulation of ion transport functions and the epithelial permeability is key for precisely controlling internal osmolality and ion concentrations within a narrow range. In hypotonic freshwater (FW), teleosts actively take up Na+ via ionocytes and reduce passive Na+ loss by regulating epithelial permeability (Evans et al., 2005). To date, Na+ uptake mechanisms have become a highly discussed issue in osmoregulatory and evolutionary physiology (Wichmann and Althaus, 2020; Tseng et al., 2022). Although they have been widely studied in different species, several unclear mechanisms and controversial models still need to be clarified in FW teleosts.
Compared to salt excreting pathways in seawater (SW) teleosts, Na+ uptake mechanisms in FW ones are more diverse and sophisticated in terms of ionocyte subtypes and related transporters (Evans et al., 2005; Yan and Hwang, 2019). Currently, there are three proposed pathways for Na+ uptake in FW teleosts, including Na+/H+ exchanger (NHE)-mediated, acid-sensing ion channel (ASIC)-mediated, and Na+-Cl- co-transporter (NCC)-mediated Na+ absorption models. Among these, the NHE and NCC models were established with solid and convincing molecular/physiological evidence in FW teleosts, and therefore have become widely accepted concepts about Na+ uptake (Evans, 2011; Guh and Hwang, 2017; Lewis and Kwong, 2018). However, these models were recently challenged, and an alternative pathway was proposed (Zimmer and Perry, 2020; Zimmer et al., 2020; Clifford et al., 2022). To better understand how teleosts absorb Na+, this mini-review not only summarizes the major progress in the studies of the three models and the related regulatory mechanisms, but also describes and clarifies the debates on the current models.
Krogh’s pioneering exchange idea was the first concept proposed for fish Na+ uptake and was based on the correlation of decreasing Na+ and increasing in the water containing fish (Krogh, 1938). Several decades later, it was indicated that Na+ is actually exchanged for H+, not , via Na+/H+ exchangers (NHEs) (Kerstetter et al., 1970; Kirschner et al., 1973). Thus, until 2009, an idea of metabolon was proposed that apical Rhcg and NHE in ionocytes function together to achieve (Wright and Wood, 2009). In zebrafish and medaka, knockdown/pharmacological experiments and in situ proximity ligation assays demonstrated a coupling function of apical Na+/H+ exchange (via NHE3) and NH3 excretion (via Rhcg2) in ionocytes (Wu et al., 2010; Shih et al., 2012; Ito et al., 2013). Intracellular H+ and NH3 ( deprotonated by Rhcg2) respectively facilitate apical Na+/H+ exchange and NH3 excretion, and excreted H+ and NH3 further convert into in the external water. Soon after these experiments, zebrafish NHE3b was surprisingly reported to exhibit activity (even under ion-poor conditions). NHE3b-expressing Xenopus oocytes exposed to -containing medium showed decreased intracellular Na+ and increased intracellular activities (Ito et al., 2014). Together, in the current model of NHE3-expressing ionocytes (Figure 1), basolateral Rhbg transports (and NKA probably transports ) from the interstitial fluid to the cytosol (Nakada et al., 2007; Wu et al., 2010). The could either be deprotonated by apical Rhcg for the Na+/H+ activity, or directly provide a chemical gradient for the activity of apical NHE3. In addition, carbonic anhydrases (CAs) are involved in extracellular regeneration and intracellular deprotonation of CO2, which also elevates Na+/H+ activity of NHE3 (Ito et al., 2013; Ito et al., 2014; Yan and Hwang, 2019).
Figure 1 General model of ionocytes for teleost Na+ uptake. Details refer to the text. AE1, anion exchanger 1; ASIC, acid-sensing ion channel; CA15, membrane-bound carbonic anhydrase 15; CA2, cytosolic carbonic anhydrase 2; CLC, Cl- channel; NBC, Na+-HCO3- co-transporter; NCC, Na+-Cl- co-transporter; NCC cell, NCC-expressing ionocyte; NHE3, Na+/H+ exchanger 3; NHE cell, NHE3-expressing ionocyte; NKA, Na+/K+-ATPase; Rhbg, rhesus B glycoprotein; Rhcg, rhesus C glycoprotein; VHA, vacuolar-type H+-ATPase; question mark (?), uptake function with controversial evidence; triangle mark (△), apical localization only in zebrafish and limited species; cross mark (✝), unclear driving force for NCC. Rhombus mark (⋄), transporter expressed in ionocytes or in pavement cells/keratinocytes (Nakada et al., 2007; Wu et al., 2010; Shih et al., 2013). Model size does not represent a relative cell size for NHE and NCC cells.
On the other hand, a model focusing on the epithelial Na+ channel (ENaC) coupled vacuolar-type H+-ATPase (VHA), derived from the model in frog skin (Harvey, 1992), was proposed as an alternative pathway for fish Na+ uptake (Avella and Bornancin, 1989; Bury and Wood, 1999). In fact, teleosts have lost ENaC genes and thus lack the trait of VHA-driven ENaC that is needed to absorb Na+ (Waldmann and Lazdunski, 1998). However, several studies have provided functional evidence that bafilomycin (a VHA inhibitor) decreased Na+ uptake in FW tilapia, carp, zebrafish, and trout (Fenwick et al., 1999; Reid et al., 2003; Esaki et al., 2007), raising the possibility of other VHA-driven Na+ channels. The long-sought after candidate turned out to be the acid-sensing ion channel (ASIC, belonging to ENaC/degenerin superfamily) which was found in teleost genomes (Paukert et al., 2004; Holzer, 2009). ASIC4b was found to be expressed in trout ionocytes and zebrafish H+-ATPase-rich (HR) ionocytes (Dymowska et al., 2014; Dymowska et al., 2015). Unfortunately, the ASIC model may not fit all FW teleosts. Medaka express VHA in the basolateral membrane of ionocytes, and tilapia did not show VHA expressed in ionocytes at all (Hiroi et al., 1998; Hsu et al., 2014). Actually, only zebrafish and very limited stenohaline FW species were reported to show apical VHA in gill ionocytes (Tseng et al., 2020). Functionally, it does not seem possible to take up Na+ from FW via ASIC, owing to its gating kinetics. ASIC is constitutively inactivated and only opens transiently when encountering external acidification, but prolonged acidification desensitizes ASIC and makes it closed (Gründer and Pusch, 2015; Yoder et al., 2018; Wichmann and Althaus, 2020). Altogether, it seems that ASIC may not play a role in Na+ uptake of ionocytes. This is probably the reason why ASIC inhibitor treatments or knockdown of ASIC4b did not decrease Na+ influxes in zebrafish larvae (Zimmer et al., 2018). Overall, whether the ASIC model is applicable to teleost ionocytes remains controversial.
An early concept that stood for almost 70 years suggested that the Na+ uptake pathway was uncoupled with Cl- transport in fish (Krogh, 1937; Maetz and Garcia Romeu, 1964). However, a direct linkage between Na+ and Cl- uptake was functionally observed in tilapia and goldfish (Chang et al., 2003; Preest et al., 2005). Subsequently, the Na+-Cl- co-transporter (NCC) was discovered to apically localize in gill ionocytes of FW tilapia (Hiroi et al., 2005; Hiroi et al., 2008). Na+ and Cl- uptake functions of NCC-expressing ionocytes were also examined using metolazone (a NCC inhibitor) or specific morpholino knockdown in the larvae of tilapia and zebrafish (Horng et al., 2009; Wang et al., 2009). In the current model of NCC-expressing ionocytes (Figure 1), apical uptake of Na+ and Cl- is achieved through NCC, and basolateral absorptions of Na+ and Cl- are considered to be achieved through the co-transporter (NBC)/NKA and Cl- channel (CLC), respectively (Evans, 2011; Wang et al., 2015; Yan and Hwang, 2019).
Thermodynamic principles and the driving force behind Na+ uptake are the pressing issues yet to be addressed in membrane ion transport. In the NHE model, NHE3, an electroneutral transporter, extrudes to bring Na+ into ionocytes across the apical membrane down the chemical gradient between the environment and the cytosol. The Na+ concentration (< 1 mM) in FW is much lower than the intracellular concentration of Na+ in gill ionocytes (6.4-15 mM, data from opercular ionocytes in tilapia), suggesting that NHE must rely on H+ and/or gradients against unfavorable Na+ gradients. Indeed, the intracellular concentration in teleost gill cells (626-963 μM) is much higher than that in FW (<0.6 μM) (Li et al., 1997; Tseng et al., 2022). High intracellular could provide a great chemical gradient of (or H+, dissociated from ) to inwardly drive Na+ transport. Although short-term acid (pH< 5) or low-Na+ (Na+< 0.1 mM) exposure may suddenly increase the thermodynamic constraint (Parks et al., 2008), most FW teleosts are able to increase NHE3/Rhcg/Rhbg expression and the number of NHE3-expressing ionocytes after long-term acclimation, as well as elevate excretion (Hirata et al., 2003; Wu et al., 2010; Furukawa et al., 2011; Lin et al., 2012; Tseng et al., 2020). Taken together, the contribution of intracellular and apical NHE-mediated exchange are key factors to be reckoned with. Interestingly, during the evolution of FW adaptation, the NHE model may have developed as the dominant way for teleosts to take up Na+ (see our next section).
On the other hand, in the NCC model, basolateral NBC, CLC, and NKA of ionocytes do not seem capable of inwardly driving Na+ and Cl- uptake via apical NCC, owing to high Na+ and Cl- concentrations in teleost blood (130 mM and 125mM respectively) (Evans et al., 2005). Although the transport function of NCC has been examined in vivo using morpholinos and inhibitors (Horng et al., 2009; Wang et al., 2009), the driving force for apical uptake via NCC is still an open question.
As ammonotelic animals, teleosts mainly produce ammonia as nitrogen wastes and directly excrete ammonia (including ~2% NH3 and ~98% under normal physiological pH) into the surrounding water, which saves more energy than further converting ammonia into urea or uric acid before excretion. It is physiologically reasonable that NHE-mediated exchange would be an efficient and energy-saving pathway for excreting acid (H+) and nitrogen wastes (ammonia), as well as taking up Na+ from FW. Because most FW teleosts show a high NHE3 expression in a specific subtype of gill/skin ionocytes (NHE3-expressing ionocytes), an evolutionary hypothesis has been recently proposed. During the evolution of FW adaptation, teleosts likely relied on NHE-mediated exchange for a large amount of Na+ uptake (Tseng et al., 2020; Tseng et al., 2022). Of note, teleosts generally exhibit a relative high excretion rate up to almost 2500 µmole/kg/h in FW, compared to that of non-teleost fishes such as stenohaline lamprey (Cyclostomata) (50-100 µmole/kg/h in FW), skate (Chondrichthyes) (~130 µmole/kg/h in SW), and sturgeon (Condrostei) (208-724 µmole/kg/h in FW) (Gershanovich and Pototskij, 1995; Altinok and Grizzle, 2004; Steele et al., 2005; Tseng et al., 2022). Moreover, convincing physiological evidence was also found in two model species of teleosts, euryhaline medaka and stenohaline zebrafish. Acute exposure to high ammonia FW decreased Na+ uptake in skin ionocytes of larval medaka by around 70%; a treatment of NHE inhibitor (5-ethylisopropyl amiloride, EIPA) caused similar declines (65-70%) in Na+ uptake and excretion (Tseng et al., 2022). Similarly in larval skin of zebrafish acclimated to low-Na+ FW, both high ammonia exposure and knockdown of NHE3b impaired over 50% of Na+ uptake and excretion (Shih et al., 2012). In the gills of zebrafish and medaka, NHE3 expression was also stimulated by Na+-deficient FW (Shih et al., 2012; Tseng et al., 2022). These findings reinforce the notion of a considerable reliance on NHE-mediated exchange by FW teleosts.
Differentially expressed in two subtypes of ionocyte, NHE3 and NCC work in collaboration to take up Na+ (Yan and Hwang, 2019; Inokuchi et al., 2022). It is widely accepted that NHE3 is a major transporter and NCC is a minor transporter for Na+ uptake in FW teleosts, based on the evidence that Na+ is mainly accumulated in zebrafish HR ionocytes (NHE3b-expressing cells), and the density of NHE3-expressing ionocytes is higher than that of NCC-expressing ionocytes in larval skin (Esaki et al., 2007; Hiroi et al., 2008; Shih et al., 2021). Besides, compensatory regulation on Na+ uptake by NHE3b and NCC was also revealed in larval zebrafish (Chang et al., 2013).
Several reviews have comprehensively summarized how hormones act on Na+ uptake regulation in teleosts (Guh and Hwang, 2017; Lewis and Kwong, 2018; Yan and Hwang, 2019). Here, we focused on describing the cases in which the regulation of Na+ uptake is also dependent on water chemistry. Acidic or low-Na+ FW results in the alteration of transporter expression and ionocyte number. Long-term exposure to acidic FW triggers the expression of NHE3 (and Rhcg) in most FW teleosts such as dace, tilapia, medaka, carp, and goldfish (Hirata et al., 2003; Tseng et al., 2020). They adopt NHE3 to excrete more against acidic environments and simultaneously absorb Na+. Meanwhile, very few teleosts (zebrafish, for example) mainly up-regulate apical VHA instead of NHE3 for the enhancement of acid excretion (Yan et al., 2007; Tseng et al., 2020). Zebrafish gills showed a down-regulated NHE3b expression with an increased number of NCC2b-expressing ionocytes after acid acclimation for 7 days (Chang et al., 2013). That is, zebrafish utilize NCC2b as a backup transporter for maintaining Na+ homeostasis under acidic FW, although this fact still cannot exclude the possibility that other NHE isoforms may compensate for the loss of NHE3b. Long-term exposure to Na+-deficient FW stimulates mRNA expression of NHE3 (and Rhcg) and the number of NHE3-expressing ionocytes in FW teleosts (Inokuchi et al., 2009; Wu et al., 2010; Shih et al., 2012; Tseng et al., 2022). However, studies from zebrafish and medaka revealed that branchial mRNA expression of NCC was down-regulated in low-Na+ FW (with low-Cl-) (Wang et al., 2009; Hsu et al., 2014). In tilapia gills, low-Na+ FW (with normal- or low-Cl-) did not affect the mRNA expression of NCC, while low-Cl- FW (with normal Na+) increased the mRNA expression of NCC and the density of NCC-expressing ionocytes (Inokuchi et al., 2009). These findings suggest that up-regulation of NHE3 is the major pathway for functional enhancement of Na+ uptake under Na+-deficient situations, but the regulation of NCC is depending on both Na+/Cl- levels in FW and probably varies in different species.
Debates on the current models of Na+ uptake pathways originated from the thermodynamic considerations for NHE and NCC. Some studies have proposed that the Na+ uptake function of NHEs is only favored when a ratio of intracellular and FW concentration of Na+ is smaller than that of a ratio of H+, which is not feasible under acidic or Na+-poor situations (Dymowska et al., 2014; Dymowska et al., 2015; Clifford et al., 2022). Obviously, their concern probably neglected the activity of NHEs. As we described above, apical exchange of NHEs could be driven down the gradients in ionocytes. But for NCC, how to drive Na+/Cl- into ionocytes against the thermodynamic limitations indeed remains a mystery. Based on these debates, recent studies generated nhe3b- and rhcg2-knockout zebrafish (using CRISPR/Cas9) to reassess the contribution of NHE3b, Rhcg2, and NCC to Na+ uptake in larvae (Zimmer and Perry, 2020; Zimmer et al., 2020). They found that knockout of nhe3b or rhcg2 did not reduce whole-body Na+ uptake and Na+ content, and Na+ or Cl- influxes were not respectively affected by Cl–-free or low-Na+ FW in NHE3b mutants, thereby concluding that larval zebrafish do not require NHE3b and Rhcg2 to sustain whole-body Na+ uptake, nor do they adopt an NCC-mediated pathway to compensate for the loss of NHE3b function. The finding that zebrafish could survive even lacking the major transporter (NHE3b) for Na+ uptake is unexpected and does suggest the possibility of unknown back-up pathways for Na+ compensatory regulation in teleosts. However, the knockout results are not necessary to overrule the previous knockdown/pharmacological evidence that supported the crucial role of NHE3b and NCC in Na+ uptake (Esaki et al., 2007; Wang et al., 2009; Shih et al., 2012; Chang et al., 2013; Ito et al., 2014). In fact, it is quite reasonable to observe different results among gene knockout and knockdown experiments. Knockdown and knockout probably induced distinct compensatory mechanisms and thereby resulted in inconsistent phenotypes (Rossi et al., 2015). That said, further and more comprehensive explorations of the compensatory mechanisms activated in those knockout mutants (Zimmer and Perry, 2020; Zimmer et al., 2020) are awaited. Loss- (or gain-) of-function experiments using pharmacology, knockdown, or knockout approaches are powerful, but could link misleading information to related issues without the appropriate and careful characterizations of the methodology effectiveness and related compensatory mechanisms.
A new pathway for Na+ uptake, derived from the same debates around thermodynamics, was recently proposed in adult zebrafish. Clifford and his colleagues found that Na+ uptake was constitutively lower at 0 h of acid exposure but recovered after 8-10 h of acid exposure. They considered this recovery of Na+ uptake to be linked to the environmental K+ concentration, not the NHE- and NCC-mediated pathways (Clifford et al., 2022), and thus proposed an alternative pathway for zebrafish coping with short-term acidification. However, inconsistent results in a previous study reported that acute acid exposure (0 h) did not reduce Na+ uptake in adult zebrafish (Kumai et al., 2011), which implies further confirmation of the methodology or a detailed description of experimental designs would be necessary in advance. Furthermore, Na+ uptake did not change during the initial 96 h acid exposure, and instead, a great degree of increase in Na+ uptake was observed after 120 h acid exposure (Kumai et al., 2011). These results highlight the variable physiological responses that could be observed during the acclimation period. A reasonable comparison of mechanisms or hypothetical differences between studies should base on a similar or comparable experimental time period. On the other hand, Clifford proposed K+-dependent Na+/Ca2+ exchangers (NCKXs) to be the candidates that mediate the K+-associated Na+ uptake function (Clifford et al., 2022). It has been noted that NCKX3 was found to localize to the basolateral layer of mice DCT and involved in Ca2+ transport (Lee et al., 2009), but how NCKXs work and even cellular localization of NCKXs in teleost gills are unknown. Further characterization of the molecular identity of the newly-proposed transport pathway is needed.
FW teleosts absorb Na+ via NHE-mediated and NCC-mediated Na+ uptake pathways in gill/skin ionocytes, and they rely on NHE for a majority of Na+ uptake probably due to a powerful force ( gradient) that efficiently drives NHE (Figure 1). Although some unclear mechanisms still remain, powerful techniques (e.g., single cell transcriptome analysis and a scanning ion-selective electrode technique) have been developed and recently applied to fish gills (Pan et al., 2022; Shih et al., 2022), which may shed some light on fish osmoregulation and the transport mechanisms of Na+ and other ions.
S-WS and P-PH conceived the idea. J-JY and M-YC provided suggestions to this review. S-WS wrote the manuscript draft. P-PH supervised and finalized the manuscript. All authors approved the manuscript for publication.
This review was financially supported by grants to P-PH from the Ministry of Science and Technology (MOST 109-2326-B-001-007), Taiwan.
We thank Ya-Ting Chuang (Institute of Cellular and Organismic Biology, Academia Sinica) for drawing Figure 1.
The authors declare that the research was conducted in the absence of any commercial or financial relationships that could be construed as a potential conflict of interest.
All claims expressed in this article are solely those of the authors and do not necessarily represent those of their affiliated organizations, or those of the publisher, the editors and the reviewers. Any product that may be evaluated in this article, or claim that may be made by its manufacturer, is not guaranteed or endorsed by the publisher.
Altinok I., Grizzle J. M. (2004). Excretion of ammonia and urea by phylogenetically diverse fish species in low salinities. Aquaculture 238, 499–507. doi: 10.1016/j.aquaculture.2004.06.020
Avella M., Bornancin M. (1989). A new analysis of ammonia and sodium transport through the gills of the freshwater rainbow trout (Salmo gairdneri). J. Exp. Biol. 142, 155–175. doi: 10.1242/jeb.142.1.155
Bury N. R., Wood C. M. (1999). Mechanism of branchial apical silver uptake by rainbow trout is via the proton-coupled na+ channel. Am. J. Physiol. Regul. Integr. Comp. Physiol. 277, R1385–R1391. doi: 10.1152/ajpregu.1999.277.5.R1385
Chang W.-J., Wang Y.-F., Hu H.-J., Wang J.-H., Lee T.-H., Hwang P.-P. (2013). Compensatory regulation of na+ absorption by Na+/H+ exchanger and na+-cl- cotransporter in zebrafish (Danio rerio). Front. zoology 10, 1–12. doi: 10.1186/1742-9994-10-46
Chang I.-C., Wei Y.-Y., Chou F.-I., Hwang P.-P. (2003). Stimulation of cl– uptake and morphological changes in gill mitochondria-rich cells in freshwater tilapia (Oreochromis mossambicus). Physiol. Biochem. Zoology 76, 544–552. doi: 10.1086/375438
Clifford A. M., Tresguerres M., Goss G. G., Wood C. M. (2022). A novel k+-dependent na+ uptake mechanism during low pH exposure in adult zebrafish (Danio rerio): New tricks for old dogma. Acta Physiologica 234, e13777. doi: 10.1111/apha.13777
Dymowska A. K., Boyle D., Schultz A. G., Goss G. G. (2015). The role of acid-sensing ion channels in epithelial na+ uptake in adult zebrafish (Danio rerio). J. Exp. Biol. 218, 1244–1251. doi: 10.1242/jeb.113118
Dymowska A. K., Schultz A. G., Blair S. D., Chamot D., Goss G. G. (2014). Acid-sensing ion channels are involved in epithelial na+ uptake in the rainbow trout Oncorhynchus mykiss. Am. J. Physiology-Cell P hysiology 307, C255–C265. doi: 10.1152/ajpcell.00398.2013
Esaki M., Hoshijima K., Kobayashi S., Fukuda H., Kawakami K., Hirose S. (2007). Visualization in zebrafish larvae of na+ uptake in mitochondria-rich cells whose differentiation is dependent on foxi3a. Am. J. Physiology-Regulatory Integr. Comp. Physiol. 292, R470–R480. doi: 10.1152/ajpregu.00200.2006
Evans D. (2011). Freshwater fish gill ion transport: August krogh to morpholinos and microprobes. Acta Physiologica 202, 349–359. doi: 10.1111/j.1748-1716.2010.02186.x
Evans D. H., Piermarini P. M., Choe K. P. (2005). The multifunctional fish gill: dominant site of gas exchange, osmoregulation, acid-base regulation, and excretion of nitrogenous waste. Physiol. Rev. 85, 97–177. doi: 10.1152/physrev.00050.2003
Fenwick J., Wendelaar Bonga S., Flik G. (1999). In vivo bafilomycin-sensitive na+ uptake in young freshwater fish. J. Exp. Biol. 202, 3659–3666. doi: 10.1242/jeb.202.24.3659
Furukawa F., Watanabe S., Inokuchi M., Kaneko T. (2011). Responses of gill mitochondria-rich cells in Mozambique tilapia exposed to acidic environments (pH 4.0) in combination with different salinities. Comp. Biochem. Physiol. Part A: Mol. Integr. Physiol. 158, 468–476. doi: 10.1016/j.cbpa.2010.12.003
Gershanovich A., Pototskij I. (1995). The peculiarities of non-faecal nitrogen excretion in sturgeons (Pisces; acipenseridae)–2. effects of water temperature, salinity and pH. Comp. Biochem. Physiol. Part A: Physiol. 111, 313–317. doi: 10.1016/0300-9629(94)00201-4
Gründer S., Pusch M. (2015). Biophysical properties of acid-sensing ion channels (ASICs). Neuropharmacology 94, 9–18. doi: 10.1016/j.neuropharm.2014.12.016
Guh Y.-J., Hwang P.-P. (2017). Insights into molecular and cellular mechanisms of hormonal actions on fish ion regulation derived from the zebrafish model. Gen. Comp. Endocrinol. 251, 12–20. doi: 10.1016/j.ygcen.2016.08.009
Harvey B. J. (1992). Energization of sodium absorption by the h+-ATPase pump in mitochondria-rich cells of frog skin. J. Exp. Biol. 172, 289–309. doi: 10.1242/jeb.172.1.289
Hirata T., Kaneko T., Ono T., Nakazato T., Furukawa N., Hasegawa S., et al. (2003). Mechanism of acid adaptation of a fish living in a pH 3.5 lake. Am. J. Physiology-Regulatory Integr. Comp. Physiol. 284, R1199–R1212. doi: 10.1152/ajpregu.00267.2002
Hiroi J., Kaneko T., Uchida K., Hasegawa S., Tanaka M. (1998). Immunolocalization of vacuolar-type h-ATPase in the yolk-sac membrane of tilapia (Oreochromis mossambicus) larvae. Zoological Sci. 15, 447–453. doi: 10.2108/0289-0003(1998)15[447:IOVHIT]2.0.CO;2
Hiroi J., McCormick S. D., Ohtani-Kaneko R., Kaneko T. (2005). Functional classification of mitochondrion-rich cells in euryhaline Mozambique tilapia (Oreochromis mossambicus) embryos, by means of triple immunofluorescence staining for Na+/K+-ATPase, Na+/K+/2Cl- cotransporter and CFTR anion channel. J. Exp. Biol. 208, 2023–2036. doi: 10.1242/jeb.01611
Hiroi J., Yasumasu S., McCormick S. D., Hwang P.-P., Kaneko T. (2008). Evidence for an apical Na–cl cotransporter involved in ion uptake in a teleost fish. J. Exp. Biol. 211, 2584–2599. doi: 10.1242/jeb.018663
Holzer P. (2009). Acid-sensitive ion channels and receptors. Sensory nerves 194, 283–332. doi: 10.1007/978-3-540-79090-7_9
Horng J.-L., Hwang P.-P., Shih T.-H., Wen Z.-H., Lin C.-S., Lin L.-Y. (2009). Chloride transport in mitochondrion-rich cells of euryhaline tilapia (Oreochromis mossambicus) larvae. Am. J. Physiology-Cell Physiol. 297, C845–C854. doi: 10.1152/ajpcell.00218.2009
Hsu H.-H., Lin L.-Y., Tseng Y.-C., Horng J.-L., Hwang P.-P. (2014). A new model for fish ion regulation: identification of ionocytes in freshwater-and seawater-acclimated medaka (Oryzias latipes). Cell Tissue Res. 357, 225–243. doi: 10.1007/s00441-014-1883-z
Inokuchi M., Hiroi J., Kaneko T. (2022). Why can Mozambique tilapia acclimate to both freshwater and seawater? insights from the plasticity of ionocyte functions in the euryhaline teleost. Front. Physiol. 13. doi: 10.3389/fphys.2022.914277
Inokuchi M., Hiroi J., Watanabe S., Hwang P.-P., Kaneko T. (2009). Morphological and functional classification of ion-absorbing mitochondria-rich cells in the gills of Mozambique tilapia. J. Exp. Biol. 212, 1003–1010. doi: 10.1242/jeb.025957
Ito Y., Kato A., Hirata T., Hirose S., Romero M. F. (2014). Na+/H+ and Na+/NH4+ exchange activities of zebrafish NHE3b expressed in xenopus oocytes. Am. J. Physiology-Regulatory Integr. Comp. Physiol. 306, R315–R327. doi: 10.1152/ajpregu.00363.2013
Ito Y., Kobayashi S., Nakamura N., Miyagi H., Esaki M., Hoshijima K., et al. (2013). Close association of carbonic anhydrase (CA2a and CA15a), Na+/H+ exchanger (Nhe3b), and ammonia transporter Rhcg1 in zebrafish ionocytes responsible for na+ uptake. Front. Physiol. 4, 59. doi: 10.3389/fphys.2013.00059
Kerstetter T. H., Kirschner L. B., Rafuse D. D. (1970). On the mechanisms of sodium ion transport by the irrigated gills of rainbow trout (Salmo gairdneri). J. Gen. Physiol. 56, 342–359. doi: 10.1085/jgp.56.3.342
Kirschner L. B., Greenwald L., Kerstetter T. H. (1973). Effect of amiloride on sodium transport across body surfaces of freshwater animals. Am. J. Physiology-Legacy Content 224, 832–837. doi: 10.1152/ajplegacy.1973.224.4.832
Krogh A. (1937). Osmotic regulation in fresh water fishes by active absorption of chloride ions. Z. für vergleichende Physiologie 24, 656–666. doi: 10.1007/BF00592303
Krogh A. (1938). The active absorption of ions in some freshwater animals. Z. für vergleichende Physiologie 25, 335–350. doi: 10.1007/BF00339641
Kumai Y., Bahubeshi A., Steele S., Perry S. F. (2011). Strategies for maintaining na+ balance in zebrafish (Danio rerio) during prolonged exposure to acidic water. Comp. Biochem. Physiol. Part A: Mol. Integr. Physiol. 160, 52–62. doi: 10.1016/j.cbpa.2011.05.001
Lee G.-S., Choi K.-C., Jeung E.-B. (2009). K+-dependent Na+/Ca2+ exchanger 3 is involved in renal active calcium transport and is differentially expressed in the mouse kidney. Am. J. Physiology-Renal Physiol. 297, F371–F379. doi: 10.1152/ajprenal.90615.2008
Lewis L., Kwong R. W. (2018). Zebrafish as a model system for investigating the compensatory regulation of ionic balance during metabolic acidosis. Int. J. Mol. Sci. 19, 1087. doi: 10.3390/ijms19041087
Li J., Eygensteyn J., Lock R., Bonga S., Flik G. (1997). Na+ and Ca2+ homeostatic mechanisms in isolated chloride cells of the teleost oreochromis mossambicus analysed by confocal laser scanning microscopy. J. Exp. Biol. 200, 1499–1508. doi: 10.1242/jeb.200.10.1499
Lin C.-C., Lin L.-Y., Hsu H.-H., Thermes V., Prunet P., Horng J.-L., et al. (2012). Acid secretion by mitochondrion-rich cells of medaka (Oryzias latipes) acclimated to acidic freshwater. Am. J. Physiology-Regulatory Integr. Comp. Physiol. 302, R283–R291. doi: 10.1152/ajpregu.00483.2011
Maetz J., Garcia Romeu F. (1964). The mechanism of sodium and chloride uptake by the gills of a fresh-water fish, carassius auratus: II. evidence for NH4+/Na+ and HCO 3-/Cl- exchanges. J. Gen. Physiol. 47, 1209–1227. doi: 10.1085/jgp.47.6.1209
Nakada T., Westhoff C. M., Kato A., Hirose S. (2007). Ammonia secretion from fish gill depends on a set of Rh glycoproteins. FASEB J. 21, 1067–1074. doi: 10.1096/fj.06-6834com
Pan W., Godoy R. S., Cook D. P., Scott A. L., Nurse C. A., Jonz M. G. (2022). Single-cell transcriptomic analysis of neuroepithelial cells and other cell types of the gills of zebrafish (Danio rerio) exposed to hypoxia. Sci. Rep. 12, 1–17. doi: 10.1038/s41598-022-13693-1
Parks S. K., Tresguerres M., Goss G. G. (2008). Theoretical considerations underlying na+ uptake mechanisms in freshwater fishes. Comp. Biochem. Physiol. Part C: Toxicol. Pharmacol. 148, 411–418. doi: 10.1016/j.cbpc.2008.03.002
Paukert M., Sidi S., Russell C., Siba M., Wilson S. W., Nicolson T., et al. (2004). A family of acid-sensing ion channels from the zebrafish: widespread expression in the central nervous system suggests a conserved role in neuronal communication. J. Biol. Chem. 279, 18783–18791. doi: 10.1074/jbc.M401477200
Preest M. R., Gonzalez R. J., Wilson R. W. (2005). A pharmacological examination of na+ and cl– transport in two species of freshwater fish. Physiol. Biochem. Zoology 78, 259–272. doi: 10.1086/427058
Reid S. D., Hawkings G., Galvez F., Goss G. (2003). Localization and characterization of phenamil-sensitive na+ influx in isolated rainbow trout gill epithelial cells. J. Exp. Biol. 206, 551–559. doi: 10.1242/jeb.00109
Rossi A., Kontarakis Z., Gerri C., Nolte H., Hölper S., Krüger M., et al. (2015). Genetic compensation induced by deleterious mutations but not gene knockdowns. Nature 524, 230–233. doi: 10.1038/nature14580
Shih T.-H., Horng J.-L., Lai Y.-T., Lin L.-Y. (2013). Rhcg1 and rhbg mediate ammonia excretion by ionocytes and keratinocytes in the skin of zebrafish larvae: H+-ATPase-linked active ammonia excretion by ionocytes. Am. J. Physiology-Regulatory Integr. Comp. Physiol. 304, R1130–R1138. doi: 10.1152/ajpregu.00550.2012
Shih T.-H., Horng J.-L., Liu S.-T., Hwang P.-P., Lin L.-Y. (2012). Rhcg1 and NHE3b are involved in ammonium-dependent sodium uptake by zebrafish larvae acclimated to low-sodium water. Am. J. Physiology-Regulatory Integr. Comp. Physiol. 302, R84–R93. doi: 10.1152/ajpregu.00318.2011
Shih S.-W., Yan J.-J., Tsou Y.-L., Lu S.-W., Wang M.-C., Chou M.-Y., et al. (2022). In vivo functional assay in fish gills: Exploring branchial acid-excreting mechanisms in zebrafish. Int. J. Mol. Sci. 23, 4419. doi: 10.3390/ijms23084419
Shih S.-W., Yan J.-J., Wang Y.-H., Tsou Y.-L., Chiu L., Tseng Y.-C., et al. (2021). Estrogen-related receptor γ2 controls NaCl uptake to maintain ionic homeostasis. J. Endocrinol. 251, 149–159. doi: 10.1530/JOE-21-0112
Steele S. L., Yancey P. H., Wright P. A. (2005). The little skate raja erinacea exhibits an extrahepatic ornithine urea cycle in the muscle and modulates nitrogen metabolism during low-salinity challenge. Physiol. Biochem. Zoology 78, 216–226. doi: 10.1086/427052
Tseng Y. C., Yan J. J., Furukawa F., Chen R. D., Lee J. R., Tsou Y. L., et al. (2022). Teleostean fishes may have developed an efficient na+ uptake for adaptation to the freshwater system. Front. Physiol. 13, 947958. doi: 10.3389/fphys.2022.947958
Tseng Y. C., Yan J. J., Furukawa F., Hwang P. P. (2020). Did acidic stress resistance in vertebrates evolve as Na+/H+ exchanger-mediated ammonia excretion in fish? BioEssays 42, 1900161. doi: 10.1002/bies.201900161
Waldmann R., Lazdunski M. (1998). H+-gated cation channels: neuronal acid sensors in the NaC/DEG family of ion channels. Curr. Opin. Neurobiol. 8, 418–424. doi: 10.1016/S0959-4388(98)80070-6
Wang Y.-F., Tseng Y.-C., Yan J.-J., Hiroi J., Hwang P.-P. (2009). Role of SLC12A10. 2, a Na-cl cotransporter-like protein, in a cl uptake mechanism in zebrafish (Danio rerio). Am. J. Physiology-Regulatory Integr. Comp. Physiol. 296, R1650–R1660. doi: 10.1152/ajpregu.00119.2009
Wang Y.-F., Yan J.-J., Tseng Y.-C., Chen R.-D., Hwang P.-P. (2015). Molecular physiology of an extra-renal cl- uptake mechanism for body fluid cl- homeostasis. Int. J. Biol. Sci. 11, 1190. doi: 10.7150/ijbs.11737
Wichmann L., Althaus M. (2020). Evolution of epithelial sodium channels: current concepts and hypotheses. Am. J. Physiol. Regul. Integr. Comp. Physiol. 319, R387–R400. doi: 10.1152/ajpregu.00144.2020
Wright P. A., Wood C. M. (2009). A new paradigm for ammonia excretion in aquatic animals: role of rhesus (Rh) glycoproteins. J. Exp. Biol. 212, 2303–2312. doi: 10.1242/jeb.023085
Wu S.-C., Horng J.-L., Liu S.-T., Hwang P.-P., Wen Z.-H., Lin C.-S., et al. (2010). Ammonium-dependent sodium uptake in mitochondrion-rich cells of medaka (Oryzias latipes) larvae. Am. J. Physiology-Cell Physiol. 298, C237–C250. doi: 10.1152/ajpcell.00373.2009
Yan J.-J., Chou M.-Y., Kaneko T., Hwang P.-P. (2007). Gene expression of Na+/H+ exchanger in zebrafish h+-ATPase-rich cells during acclimation to low-na+ and acidic environments. Am. J. Physiology-Cell Physiol. 293, C1814–C1823. doi: 10.1152/ajpcell.00358.2007
Yan J.-J., Hwang P.-P. (2019). Novel discoveries in acid-base regulation and osmoregulation: a review of selected hormonal actions in zebrafish and medaka. Gen. Comp. Endocrinol. 277, 20–29. doi: 10.1016/j.ygcen.2019.03.007
Yoder N., Yoshioka C., Gouaux E. (2018). Gating mechanisms of acid-sensing ion channels. Nature 555, 397–401. doi: 10.1038/nature25782
Zimmer A. M., Dymowska A. K., Kumai Y., Goss G. G., Perry S. F., Kwong R. W. (2018). Assessing the role of the acid-sensing ion channel ASIC4b in sodium uptake by larval zebrafish. Comp. Biochem. Physiol. Part A: Mol. Integr. Physiol. 226, 1–10. doi: 10.1016/j.cbpa.2018.06.012
Zimmer A. M., Perry S. F. (2020). The rhesus glycoprotein rhcgb is expendable for ammonia excretion and na+ uptake in zebrafish (Danio rerio). Comp. Biochem. Physiol. Part A: Mol. Integr. Physiol. 247, 110722. doi: 10.1016/j.cbpa.2020.110722
Keywords: ionocyte, NHE, ASIC, NCC, Na+ uptake, teleost
Citation: Shih S-W, Yan J-J, Chou M-Y and Hwang P-P (2023) Recent progress and debates in molecular physiology of Na+ uptake in teleosts. Front. Mar. Sci. 10:1066929. doi: 10.3389/fmars.2023.1066929
Received: 11 October 2022; Accepted: 04 January 2023;
Published: 20 January 2023.
Edited by:
Leonardo Julián Magnoni, Plant and Food Research, New ZealandReviewed by:
Susumu Hyodo, The University of Tokyo, JapanCopyright © 2023 Shih, Yan, Chou and Hwang. This is an open-access article distributed under the terms of the Creative Commons Attribution License (CC BY). The use, distribution or reproduction in other forums is permitted, provided the original author(s) and the copyright owner(s) are credited and that the original publication in this journal is cited, in accordance with accepted academic practice. No use, distribution or reproduction is permitted which does not comply with these terms.
*Correspondence: Pung-Pung Hwang, cHBod2FuZ0BnYXRlLnNpbmljYS5lZHUudHc=
Disclaimer: All claims expressed in this article are solely those of the authors and do not necessarily represent those of their affiliated organizations, or those of the publisher, the editors and the reviewers. Any product that may be evaluated in this article or claim that may be made by its manufacturer is not guaranteed or endorsed by the publisher.
Research integrity at Frontiers
Learn more about the work of our research integrity team to safeguard the quality of each article we publish.