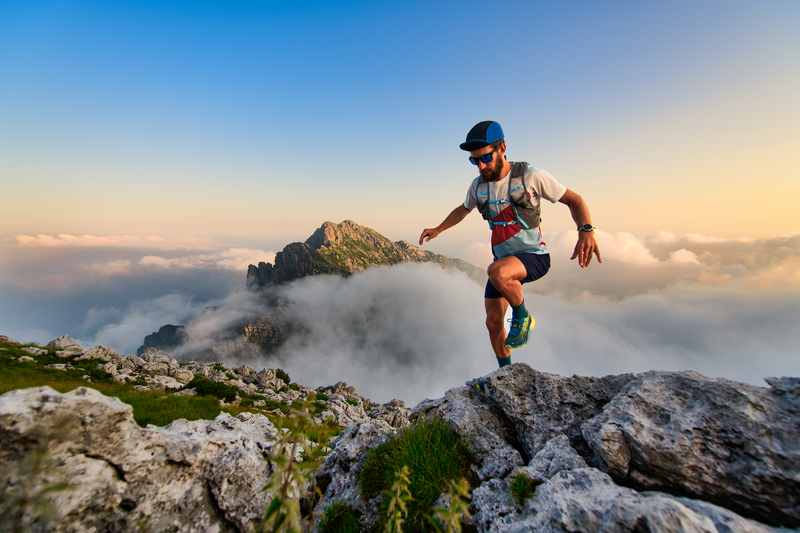
95% of researchers rate our articles as excellent or good
Learn more about the work of our research integrity team to safeguard the quality of each article we publish.
Find out more
ORIGINAL RESEARCH article
Front. Mar. Sci. , 18 May 2023
Sec. Marine Biotechnology and Bioproducts
Volume 10 - 2023 | https://doi.org/10.3389/fmars.2023.1048346
Introduction: The up-side down jellyfish Cassiopea andromeda represents a yet untapped marine species that could be targeted as a new source for functional ingredients, such as natural pigments and antioxidants. Since C. andromeda hosts endosymbiotic dinoflagellates, this jellyfish contains peridinin pigments, which are linked with high antioxidant capacities and many other health-promoting properties. This study investigates the potential to specifically increase the content of peridinin and overall antioxidant activity in C. andromeda, through the targeted application of different photosynthetic active radiation (PAR) intensities and ultraviolet radiation, cultured in fully controlled indoor aquaculture systems.
Materials and Methods: Indoor bred C. andromeda specimens were exposed to five different PAR intensities (50, 100, 200, 400 and 800 µmol photons m−2 s−1) and a combined treatment of narrow-band UVB (λ = 285 ± 10 nm) radiation and intermediate (200 µmol photons m−2 s−1) PAR intensity over a period of four weeks. Before the treatment and after two- and four-week treatment intervals, pigment concentrations and antioxidant activity levels were measured using high-performance liquid chromatography (HPLC) and the Trolox Equivalent AntioxidantCapacity (TEAC) assay, respectively. In addition, relative growth rate, umbrella pulsation and photosynthetic efficiency (measured by pulse amplitude modulated fluorometry) of C. andromeda individuals were also monitored throughout the experiment.
Results: Chlorophyll a (Chl a) and peridinin (Per) dominated overall pigment content in C. andromeda endosymbionts, chlorophyll c2 and diadinoxanthin were detected in minor amounts. Over the treatment time, Chl a and Per concentrations, measured as µg g−1 jellyfish dry weight and pg microalgae-cell−1, decreased sharply at higher PAR intensities (200 – 800 µmol photons m−2 s−1) compared to the control treatment (100 µmol photons m−2 s−1). After four weeks Chl a and Per concentrations were lowest at the highest PAR intensity (800 µmol photons m−2 s−1) and highest at the lowest PAR intensity (50 µmol photons m−2 s−1). Moreover, the ratio of Chl a and Per showed a relative decrease of Per with increasing PAR intensity. The combined treatment of narrow-band UVB (λ = 285 ± 10 nm) radiation and intermediate (200 µmol photons m−2 s−1) PAR intensity led to significantly elevated Chl a and Per concentrations compared to the 200 µmol photons m−2 s−1 PAR treatment without UVB. Significantly elevated antioxidant activity levels, measured as Trolox Equivalents mmol g−1 jellyfish dry weight, were only detected in UVB exposed C. andromeda, indicating that Chl a and Per did not determine overall antioxidant capacity. The photosynthetic efficiency of C. andromeda endosymbionts was not affected by elevated antioxidant activity. Opposing that, the jellyfish hosts that were exposed to the UVB treatment shrunk drastically, indicating a strong stress response.
Discussion: With this study, we demonstrate for the first time the application potential of PAR intensity manipulations and UVB irradiation, to increase the content of valuable pigments and antioxidants in C. andromeda jellyfish and their endosymbiotic dinoflagellates that live in hospite within the host tissue. Based on these findings, we propose the culture of C. andromeda under fully controlled and light-optimized conditions as new pathway to harness bioproducts and functional ingredients.
The interest in jellyfish biomass as potential resource for bioproducts such as functional ingredients to enhance food, feed, cosmetic and pharmaceutic items is globally on the rise. While sustainable practices for the utilization of wild jellyfish stocks are being initiated (Edelist et al., 2021), a vast array of culture techniques enable the controlled indoor aquaculture of many species (Duarte et al., 2022). However, the resources (i.e. labor and energy) required to cultivate jellyfish in land-based aquaculture systems narrows the scope for species that contain high-value products, such as functional bioactive compounds. Moreover, detailed knowledge about the content of target compounds is essential for the economic viability of controlled production systems. Recent studies on the nutritional and pharmacological properties of several jellyfish (Leone et al., 2013; Leone et al., 2015; De Domenico et al., 2019; Leone et al., 2019; De Rinaldis et al., 2021) showed promising characteristics and revealed that jellyfish, which host endosymbiotic microalgae exhibit particularly prospective functional assets, as the proteinaceous animal tissue is enriched with value-adding algae components (Leone et al., 2015). In this regard, the upside-down jellyfish Cassiopea andromeda is a particularly auspicious candidate species for controlled captivity culture, as members of the genus Cassiopea harbor densely packed microalgal symbionts belonging to the dinoflagellate family Symbiodiniaceae (Symbiodinium spp.) (Lampert et al., 2012). In C. andromeda, dinoflagellates are endosymbionts that reside in the jellyfish mantle tissue, primarily in the appendages. The resultant strongly interdependent organism-unit is referred to as a ‘holobiont’. As the C. andromeda holobiont is specialized to provide optimal growing conditions and protection for the dinoflagellates, these microalgae can readily proliferate in this host habitat (Lampert, 2016; Djeghri et al., 2019).
Dinoflagellates are a major source of bioactive molecules in marine environments, producing a wide range of chemically diverse compounds (Gallardo-Rodríguez et al., 2012; Camacho-Muñoz et al., 2021). Among others, microalgal dinoflagellates contain peridinin (Per), which is known as their signature pigment (Barlow et al., 1993; Begum et al., 2016; Jeffrey et al., 2011). In previous studies Per has shown antioxidant, antitumor, and anti-inflammatory activities (Sugawara et al., 2007; Onodera et al., 2014; Supasri et al., 2021a). Like the accessory pigment fucoxanthin, Per is a xanthophyll with unique allenic bond, which enables strong antioxidant properties (Miyashita et al., 2011; Torregrosa-Crespo et al., 2018). Due to its remarkable structure, Per is among the very few carotenoids that can exhibit high antioxidant potential, even under anoxic conditions (Nomura et al., 1997; Sachindra et al., 2007; Supasri et al., 2021a). As for most of the functional substances that are discovered in dinoflagellates, the sourcing of Per is challenged by limited natural availabilities and difficulties to culture target species in captivity. The targeted production of Per has been tested through the long-term culture of free-living dinoflagellates (Amphidinium carterae) (Fuentes-Grünewald et al., 2016) and isolated endosymbiotic dinoflagellates (Symbiodinium tridacnidorum CS-73) (Supasri et al., 2021a; Supasri et al., 2021b). However, targeting valuable compounds such as Per in endosymbiotic Symbiodinium spp., grown in hospite in a host species such as C. andromeda, has not been tested so far.
In dinoflagellates, the majority of Per occurs in two constellations: 1) in the thylakoid membrane-bound chlorophyll a-chlorophyll c2-peridinin-protein complex (acpPC) (Iglesias-Prieto et al., 1993) and 2) in a water-soluble light-harvesting complex, namely peridinin-chlorophyll a-protein (PCP) (Iglesias-Prieto et al., 1991), where Per belongs to the primary components for light-harvesting (LH). The unique structure and LH properties of PCP has been extensively studied (Song et al., 1976; Koka and Song, 1977; Polívka et al., 2007; Carbonera et al., 2014) and revealed the function of Per as carbonyl-containing carotenoid in the antenna complex, which captures light outside the absorption spectra of chlorophyll. Next to that Per plays a crucial role as photo-protectant, which prevents the formation of reactive oxygen species (ROS) by quickly and efficiently quenching the chlorophyll a triplet state that can form under photic stress (Carbonera et al., 2014). These functional characteristics make LH pigments like Per also valuable ingredients for natural healthcare items, such as food supplements (Leong et al., 2018). The growing global interest in such products requires sophisticated tools for the extraction and recovery of pigment-protein complexes (Chia et al., 2020) and makes the exploration of new sources for the sustainable supply of valuable pigments such as Per a prime interest. Like all photoactive organisms, dinoflagellates are capable to continuously optimize their LH capacity by altering the number and ratio of photosynthetic pigments (Hennige et al., 2011), in order to respond to ambient light changes. Next to LH optimization, pigment alterations can also reflect photic stress e.g. due to high intensities of photosynthetically active radiation (PAR) and ultraviolet radiation (UVR), which are both linked with the formation of cell-damaging molecules such as ROS. The targeted manipulation of algae photosystems, through controlled light changes, has been broadly studied. Several studies have suggested that lower PAR intensities lead to the increased synthesis of LH pigments (Wyman and Fay, 1987; Grossman et al., 1993), whereas higher PAR intensities result in the enhanced expression of phycocyanin, phycoerythrin (Madhyastha and Vatsala, 2007), astaxanthin (Imamoglu et al., 2009), and β-carotene (Pisal and Lele, 2005) in microalgae and cyanobacteria. In similar studies, the exposure of microalgae and cyanobacteria to varying light spectra and UVB radiation proved feasible to enhance the synthesis of phycobiliproteins (Fatma, 2009) and the production of vitamin D3 (Ljubic et al., 2020). In macroalgae, AOA levels could be significantly enhanced through elevated PAR intensities (Magnusson et al., 2015; Sommer et al., 2022). Such knowledge about the optimization of ambient light factors to enhance the production of target compounds in macroalgae and free-living microalgae, can also be considered as refinement key to enable the economic exploitation of photoactive holobionts, such as C. andromeda. Yet, information about the application of light manipulations to enhance the production of target compounds, such as Per, in C. andromeda endosymbionts cultured in hospite is scarce. Given that C. andromeda provides optimal growing conditions and protection for its endosymbionts, the in hospite culture approach may provide a more robust culture habitat compared to the culture of free-living dinoflagellates. However, a crucial aspect of the symbiosis between host species and endosymbiotic dinoflagellates is the capacity of the host tissue to optimize the incoming light through absorption and scattering. Since the quantity and quality of light that reaches the endosymbionts within the host tissue is crucial for photosynthetic activity, host species developed specific tissue characteristics, with permeability for beneficial light and impermeability for harmful light radiation, to enhance the habitat for the endosymbionts. The medusa tissue of the endosymbiotic jellyfish Cotylorhiza tuberculata showed strong properties to absorb UVR and to attenuate high light intensities (Enrique-Navarro et al., 2022). In studies with corals, different Symbiodinium strains showed adjustments of pigments, due to photic changes, only outside the host environment (Roth et al., 2010). This raises the question whether the concentration of light-harvesting pigments (LHP) can be specifically triggered in C. andromeda endosymbionts growing in hospite, through the application of LED lighting.
At present, considerable knowledge gaps remain regarding the impact of the targeted application of PAR intensity changes and UVR on the C. andromeda holobiont, particularly in the context of health-promoting properties such as Per content and AOA levels. Therefore, the aim of this study was to elucidate the effects of a broad range of PAR intensities (50–800 photons µmol m-2 s-1) and a low dose (1.3 KJ m-2 day-1) of narrow-band UVR within the UVB spectrum (λ = 285 ± 10 nm) in combination with a mild PAR intensity (200 photons µmol m-2 s-1) on (1) the content and ratio of the dominant LHPs in C. andromeda endosymbionts cultured in hospite and on (2) the AOA levels in the overall tissue of the C. andromeda holobiont cultured in land based, fully-controlled aquaculture systems.
Adult Cassiopea andromeda medusae were sourced from an established jellyfish culture bred from polyps (origin: Aquarium Berlin) within the aquaria facilities of the Leibniz Centre for Tropical Marine Research (ZMT), Bremen, Germany. Incubation experiments were conducted in experimental tanks (ETs) in the Marine Experimental Ecology unit (MAREE) of ZMT. The individual ETs function as recirculating aquaculture systems with a water volume of ~120 L, with an upper culture unit and a sump tank equipped with a biofilter system and a protein skimmer below. The temperature and salinity were set at 26°C and 35 SA (Red Sea Salt, Red Sea Fish, Israel), respectively, these conditions were controlled and regulated automatically through submerged sensors. The ETs were each illuminated with an Aquaillumination Hydra FiftyTwo HD (AI Hydra 52 HyperDrive, USA) lamp with seven types of LEDs, emitting the full spectrum (380–680 nm) of photoactive radiation (PAR) with a photon flux density of 100 µmol photons m-2 s-1.
In total, 52 visually healthy (i.e. no injured bells or lost oral arms etc.) Cassiopea andromeda specimens with initial body weights of 111.4 ± 35.7 g and diameters of 10.3 ± 1.3 cm were randomly allocated into 6 ETs. Within these ETs, the animals were individually housed in plastic containers (length 16 cm, width 12 cm, height 12 cm). These containers were fixed just below the water surface, to maintain the same horizontal position and vertical distance under the lamps. This setup allowed for the recognition of individual jellyfish and the precise control of PAR emission on a per-animal basis. Slits on the sides of these plastic containers allowed the exchange of water within the container and the surrounding tank. Over the acclimation and experimental phase, the ETs were cleaned once per week. This included the scratching off of biofilms and the siphoning of feed residues and other particles. During cleaning, approximately one-third of the water volume was exchanged with filtered seawater. In addition, the bacterial film that accumulated at the surface of the water was removed daily with a fine mesh, to prevent the refraction of light through this layer. C. andromeda individuals were target fed daily with 1 mL of dense freshly hatched brine shrimp Artemia nauplii solution using a plastic pipette. One hour after feeding, remaining food residues and any faecal matter were removed from the plastic containers via siphoning with a small plastic pipette. The small plastic boxes were regularly rotated in order to exclude any potential confounding effects associated with different positioning within these tanks. For acclimation purposes, the jellyfish were kept for three weeks in the ETs at a constant PAR intensity of 100 µmol photons m-2 s-1 with 12:12 h light/dark cycle. Light intensities (Li-250A, LI-COR, USA) and spectra (RAMSES ACC-VIS spectroradiometer, TriOS, Germany) were determined at the bottom of the plastic containers.
After the acclimation phase, four animals (n = 4) were collected for initial sampling. Subsequently, the PAR intensities in five ETs were changed in steps of no more than 100 µmol photons m-2 s-1 per day, until the desired PAR intensities of 50, 200, 400, and 800 µmol photons m-2 s-1 were reached. For the UVR-treatment, UVB-LEDs (λ=285 ± 10 nm) emitting a dose of 1.3 KJ m-2 day-1 were installed above second ET that reached a final PAR intensity of 200 µmol photons m-2 s-1. Once the target treatment conditions had been reached, these five different light manipulations remained constant over a four-week period. The sixth ET kept a constant PAR intensity of 100 µmol photons m-2 s-1 throughout the acclimation and experimental phase and served as control treatment.
The umbrella pulsation rate, chlorophyll a fluorescence and wet biomass were quantified for each individual C. andromeda medusa initially, after the acclimation phase (day 0) and at the end (day 28) of the different PAR intensity treatments and the exposure to UVB radiation. Umbrella pulsations were counted over 15 s, this number was then extrapolated to determine the number of umbrella pulses per minute and served as proxy for overall organism activity. To exclude the effect of potential handling stress, umbrella pulsations were counted before taking the organisms out of the tanks for further analyses. Chlorophyll a fluorescence was measured using a portable pulse amplitude modulated fluorometer (Diving-PAM, Walz, Effeltrich, Germany). Immediately before the fluorescence measurements, randomly selected C. andromeda specimens (n = 4 per treatment) were collected from the ETs and placed into small glass containers filled with seawater derived from their tanks. In these containers, the animals were kept in darkness for 8 min, to dark-adapt the endosymbiotic dinoflagellates. The subsequent fluorescence measurements included the following steps: 1) a probe with a low-intensity modulated measuring light was placed in close distance to the oral arms and tentacles of each C. andromeda specimen to detect minimal fluorescence (F0) of the dark-adapted endosymbionts. 2) A saturating pulse of light (2700 µmol photons m-2 s-1) was applied (0.8 s) to obtain the maximum fluorescence (Fm) of the endosymbiotic dinoflagellates. On the basis of F0 and Fm the ratio Fv/Fm was calculated using the formula (Schreiber et al., 1995; Maxwell and Johnson, 2000):
The ratio Fv/Fm quantifies the maximum quantum efficiency of photosystem II and can serve as a proxy for photosynthetic performance. Right after the fluorescence measurements, the C. andromeda medusa were placed on absorbent tissues for 5 s to remove excess water before determining the wet weight of the jellyfish on a digital scale (Sartorius, Germany). The relative growth rate (RGR) was calculated based on wet biomass weight using the formula (Connolly and Wayne, 1996):
Where ln is the natural log, W1 is the starting wet weight of one individual jellyfish, W2 is the total jellyfish wet weight after four weeks experimental time and ΔT is the length of the experiment.
For the analyses of pigments and AOA, four C. andromeda were sampled after the acclimation phase, after two weeks, and after four weeks (at the end of the experimental period). At each of these time points, whole animals were snap-frozen in liquid N2 and stored at -80°C. Prior to lab-based analyses, the sampled organisms were lyophilized for 72 h at 1 mbar (ALPHA 1-4 LD plus; Christ GmbH, Osterode, Germany). After lyophilization the dry weight of individual organisms were documented before the whole jellyfish were ground to powder for 20 s, using a benchtop homogenizer (FastPrep-24, MP Biomedicals, Germany). For the counting of endosymbiotic algae cells, the protocol from Mammone et al. (2021) was adapted and applied. From each sample, a quantity of ~20 mg lyophilized powder was resuspended in 500 µl of distilled water. For homogenization, the suspension was shaken for 16 h at 60 rpm (Intelli-Mixer RM-2L; ELMI SIA, Riga, Latvia). To prevent cellular clumping, resuspended sample solutions were ultrasonicated twice for 30 s (HD 2070.2; Brandelin electronic GmbH & Co KG, Berlin, Germany) using a Sonotrode (MS 72; 20 KHz, 70 Watt, 285µm = 100%) with a low amplitude of 20% prior to cell counting. The sample solutions were then pipetted into a Neubauer Hemocytometer (0.1 mm depth), to count the microalgae cells in triplicate under an optical microscope (20x). The total amount of microalgae cells per individual jellyfish, was calculated based on the cell concentration per dry weight of the resuspended jellyfish sub-sample.
For pigment analyses, 140 mg of lyophilized sample material was weighed into Eppendorf tubes, after which the pigments therein were extracted in 1 mL of cold 90% acetone for 24 h at 4°C in the dark. After centrifugation (2500g, 4°C, 5 min) and filtration (0.45 µm nylon syringe filters, Nalgene, USA), pigment analyses were performed using reversed-phase high-performance liquid chromatography (HPLC). Pigments (chlorophyll a, peridinin, chlorophyll c2, diadinoxanthin, and β-carotene) were separated on a LaChromElite system equipped with a chilled autosampler L-2200 and a DAD detector L-2450 (VWR-Hitachi, Germany) with a LiChropher 100-RP-18 guard cartridge, applying a gradient according to Wright et al. (1991). Peaks were detected at 440 nm, identified, and quantified via co-chromatography with appropriate standards (obtained from DHI Lab Products, Denmark). Pigment concentrations were expressed as µg g-1 C. andromeda dry weight and as pg cell-1 of endosymbiotic microalgae.
To measure AOA, 200 mg of lyophilized sample was dissolved in 1 mL ethanol (70%) and extracted in a water bath (47°C) for 4 h, vortexing hourly. Prior to this analysis, samples were centrifuged (2500 g, 20°C) for 5 min. The AOA was determined using a modified version of the ABTS+ assay described by Re et al. (1999), also known as the Trolox Equivalent Antioxidant Capacity (TEAC) assay, with Trolox (6-hydroxy-2,5,7,8-tetramethylchroman-2-carboxylic acid) serving as a standard. A 2.45 mM ABTS+ stock solution was obtained by oxidizing 7.0 mM of ABTS+ with potassium disulfate (K2S2O8) for 16 h. A working solution with a consistent photometrically measured absorption of 0.7 ± 0.02 at a wavelength of 734 nm (UV/VIS-spectrophotometer, Thermo Scientific Genesys 140/150, Fisher Scientific GmbH, Schwerte, Germany) was obtained via dilution with absolute ethanol. For the AOA analysis, 1 mL of this ABTS+ working solution was added to 10 μL of sample extract, and deradicalization was measured after 6 min. AOA of the samples was expressed as Trolox Equivalents (mmol TE 100 g-1 DW) after adjusting for the appropriate dilution factor. All chemicals were purchased from Sigma (Aldrich/Merck KGaA, Darmstadt, Germany).
To compare potential changes in the measured parameters over time, the four C. andromeda specimens that were analyzed and sampled initially (following the acclimation phase) served as a reference. To determine the effects of the different PAR intensities and UVB radiation, the different treatments (50–800 µmol photons m-2 s-1 and 200 µmol photons m-2 s-1 + UVB) were compared with one another (n = 4 per treatment), with the treatment that was the continuation of the acclimation condition (100 µmol photons m-2 s-1) serving as the control for these analyses. Statistical analyses were conducted using R (version 3.4.3; R Core Team, 2019). After confirming that data conformed to a normal distribution, differences over time and between treatments were compared using analyses of variance (ANOVAs). A p-value< 0.05 was the threshold for statistical significance. Post-hoc Tukey tests were used for pairwise comparisons among treatment groups.
Initially, the relative growth rate (RGR), umbrella pulsation rates, and photosynthetic efficiency (Fv/Fm) values for the medusae in different treatment groups were analyzed (Table 1). No significant differences in RGR were observed for C. andromeda treated with varying levels of PAR intensity (50–800 µmol photons m-2 s-1). In contrast, 200 µmol photons m-2 s-1 PAR intensity with additional UVB (λ=285 ± 10 nm) radiation (1.3 KJ m-2 day-1) exposure resulted in a significant reduction in RGR (p< 0.01; n = 4). The umbrella pulsation rate of C. andromeda revealed a clear positive correlation with PAR intensities. Compared to the initial values the pulsation rates increased significantly (p< 0.05; n = 4) for all specimens that were exposed to elevated PAR intensities (200–800 µmol photons m-2 s-1) for four weeks. C. andromeda specimens that adapted to 800 µmol photons m-2 s-1 almost doubled their pulsation rates (p< 0.01; n = 4) relative to the specimens raised under the lowest PAR intensity (50 µmol photons m-2 s-1). The UVB radiation did not affect umbrella pulsation rates. After four weeks, the photosynthetic performance, measured as Fv/Fm remained in a very narrow range (mean values: 0.66–0.67) for C. andromeda endosymbionts treated with relatively low PAR intensities (50–200 µmol photons m-2 s-1) and with additional UVB radiation, exhibiting no significant changes from the mean initial Fv/Fm values (0.68 ± 0.02). In contrast, at relatively high PAR intensities of 400–800 µmol photons m-2 s-1, Fv/Fm tended to decrease after a four-week exposure period. At 400 µmol photons m-2 s-1 the mean Fv/Fm dropped to 0.62 ± 0.02, while the lowest mean Fv/Fm value (0.58 ± 0.02) was reached at the highest PAR intensity of 800 µmol photons m-2 s-1. This latter Fv/Fm value was significantly lower (p< 0.05; n = 4) compared to the initial mean and the values in all other treatment groups other than the 400 µmol photons m-2 s-1 group, following a four-week treatment period.
Table 1 Relative growth rate (RGR), umbrella pulsation rate, and photosynthetic efficiency (Fv/Fm) values for Cassiopea andromeda specimens treated with different levels of PAR intensities (50–800 µmol photons m-2 s-1) and UVB radiation (1.3 KJ m-2 day-1).
Analyses of light-harvesting pigments (LHP) in C. andromeda endosymbionts revealed the presence of chlorophyll a, (Chl a) chlorophyll c2, and the two carotenoids peridinin (Per) and diadinoxanthin (Table 2). β-carotene and diatoxanthin were also detected, but only in negligible amounts. Pigment quantification revealed Chl a and Per as the dominant LHPs in C. andromeda endosymbionts, both on a per jellyfish dry weight and a per microalgal cell basis.
Table 2 Concentrations of chlorophyll a, peridinin, chlorophyll c2, and diadinoxanthin detected in initially sampled Cassiopea andromeda specimens.
Relative to the initial Chl a concentrations (per jellyfish dry weight), these levels only rose in C. andromeda endosymbionts exposed to the 50 µmol photons m-2 s-1 or UVB + 200 µmol photons m-2 s-1 after a two-week exposure period (Figure 1). Chl a levels in the other treatment groups decreased below the initial values with increasing PAR intensity. After two weeks, the control (100 µmol photons m-2 s-1) and elevated PAR intensity treatments (200–800 µmol photons m-2 s-1) exhibited significantly lower Chl a levels compared to the 50 µmol photons m-2 s-1 and UVB + 200 µmol photons m-2 s-1 treatment groups (p< 0.01). After four weeks, the mean Chl a concentration had more than doubled at the lowest level of PAR intensity as compared to initial levels, with this group exhibiting the highest Chl a content (p< 0.01) compared to all other treatments with the exception of UVB + 200 µmol photons m-2 s-1. Chl a concentrations in all elevated PAR intensity groups (200–800 µmol photons m-2 s-1) dropped significantly (p< 0.01) below initial and control levels after four weeks, reaching the lowest concentrations at the highest levels of PAR intensity (800 µmol photons m-2 s-1). Throughout the experiment, jellyfish endosymbionts that were exposed to UVB + 200 µmol photons m-2 s-1 exhibited significantly higher (p< 0.01) Chl a concentrations relative to endosymbionts in the 200 µmol photons m-2 s-1 treatment group. The comparison of Chl a concentrations per cell of endosymbiotic microalgae revealed a very similar pattern as compared to the changes in Chl a content per jellyfish dry weight. But, the increase in Chl a was less pronounced on a per microalgal cell basis. An overall decrease (p< 0.01-0.05) in Chl a concentrations per microalgal cell was, however, measured at PAR levels above the control intensity (100 µmol photons m-2 s-1). Moreover, a significant (p< 0.01) increase in Chl a content per microalgae cell was detected in the endosymbionts treated with UVB + 200 µmol photons m-2 s-1, compared to the endosymbionts that were treated only with the lowest PAR intensity elevation (200 µmol photons m-2 s-1).
Figure 1 Chlorophyll a concentrations in Cassiopea andromeda specimens treated with different PAR intensities (50–800 µmol photons m-2 s-1) and 200 µmol photons m-2 s-1 + UVB (1.3 KJ m-2 day-1). White boxes show the values upon initial sampling, while colored boxes show the values for these different light treatments after an exposure time of two weeks (left) and four weeks (right). Chlorophyll a concentrations are presented on a (A) per jellyfish dry weight and (B) per endosymbiotic microalgal cell basis. Boxes represent the interquartile range with lowest and highest percentiles (lines), while dots indicate outliers. Lowercase letters indicate significant differences between treatments and asterisk denote significant differences over time (between 2 and 4 weeks) within treatments. Significant differences are based on one-way ANOVAs (n = 4; p< 0.05) followed by Tukey’s HSD test.
The overall changes in Per concentrations strongly paralleled those for Chl a concentrations, both on a per jellyfish dry weight and per microalgal cell basis (Figure 2). Specifically, Per concentrations dropped after two weeks (p< 0.01) in those jellyfish endosymbionts that were treated with elevated PAR intensities (200 – 800 µmol photons m-2 s-1), compared to all other treatment groups. After four weeks, Per concentrations under elevated PAR intensities remained significantly lower (p< 0.01), continuously decreasing with rising PAR intensity. Conversely, Per levels increased (p< 0.01) at the lowest PAR intensity (50 µmol photons m-2 s-1), reaching significantly higher levels as compared to the control treatment. Throughout the experiment (after 2 and 4 weeks), the Per concentrations (per jellyfish dry weight and per microalgal cell) were significantly higher (p< 0.01) in C. andromeda endosymbionts treated with 200 µmol photons m-2 s-1 + UVB as compared to those treated with 200 µmol photons m-2 s-1 in the absence of UVB exposure.
Figure 2 Peridinin concentrations in Cassiopea andromeda specimens treated with different PAR intensities (50–800 µmol photons m-2 s-1) and 200 µmol photons m-2 s-1 + UVB (1.3 KJ m-2 day-1). White boxes show the values upon initial sampling, while colored boxes show the values for the different light treatments after two weeks (left) and four weeks (right) of exposure. Peridinin concentrations are presented on a (A) per jellyfish dry weight and (B) per endosymbiotic microalgae cell basis. Boxes represent the interquartile range with lowest and highest percentiles (lines), while dots indicate outliers. Lowercase letters indicate significant differences between treatments and asterisk denote significant differences over time (between 2 and 4 weeks) within treatments. Significant differences are based on one-way ANOVAs (n = 4; p< 0.05) followed by Tukey’s HSD test.
The Chl a to Per ratio (CPR) trended upwards when PAR intensities exceeded the control level of 100 µmol photons m-2 s-1 (Figure 3A). After two weeks, the CPR was significantly higher in the endosymbiotic microalgae receiving 400 and 800 µmol photons m-2 s-1 compared to those receiving 50 µmol photons m-2 s-1. After four weeks, C. andromeda endosymbionts treated with the highest PAR intensity exhibited a peaking CPR level, with significant (p< 0.01) differences compared to the initial, control, and lowest PAR treatment. UVB radiation did not affect CPR. No significant changes in overall AOA, as measured in Trolox equivalents (TE; mmol per 100 g dried C. andromeda), were observed between any of the tested PAR intensities (50–800 µmol photons m-2 s-1) nor over time (Figure 3B). Only those jellyfish that were exposed to 200 µmol photons m-2 s-1 + UVB exhibited significantly elevated AOA after two and after four weeks.
Figure 3 (A) The ratio of chlorophyll a/peridinin based on the concentrations per microalgal cells (pg cell-1) and (B) antioxidant activity expressed in Trolox Equivalents (TE mmol g-1 dry weight) in Cassiopea andromeda treated with different levels of PAR intensity (50–800 µmol photons m-2 s-1) and 200 µmol photons m-2 s-1 + UVB (1.3 KJ m-2 day-1). White boxes show the values upon initial sampling, while colored boxes display the values for the different light treatments after two weeks (left) and four weeks (right) of exposure. Boxes represent interquartile ranges with lowest and highest percentiles (lines), dots indicate outliers. Lowercase letters indicate significant differences between treatments. Significant differences are based on one-way ANOVAs (n = 4; p< 0.05) followed by Tukey’s HSD test.
In this study, the photosynthetic efficiency quantified as Fv/Fm was used as indicator for photosynthetic fitness of C. andromeda endosymbionts, which can also be considered as important vitality marker for the overall holobiont (Arossa et al., 2021). Based on this approach, the Fv/Fm ratio was measured to evaluate potential stress effects caused by the different PAR intensity treatments (50–800 µmol photons m-2 s-1) and the exposure to narrow-band UVB (λ=285 ± 10 nm) radiation (1.3 KJ m-2 day-1). Although the Fv/Fm values dropped clearly at elevated PAR intensities of 400 and 800 µmol photons m-2 s-1, the maximum quantum yield of photosystem II was always within a photosynthetically active range (Fv/Fm = 0.58–0.68). This suggests that the endosymbionts of these indoor-raised C. andromeda holobionts were readily able to cope with changing PAR conditions. Similarly, wild C. andromeda holobionts exhibited a high degree of photosynthetic plasticity, with photosynthetic saturation reportedly being reached at 800 and 400 µmol photons m-2 s-1, and photosynthetic compensation occurring around 200 and 50 µmol photons m-2 s-1 PAR in studies published by Mammone et al. (2021) and Welsh et al. (2009), respectively. With respect to umbrella pulsation, C. andromeda showed a clear trend towards increased activity with rising PAR intensity. Faster bell contractions may indicate a growing energy demand of C. andromeda with increasing PAR intensities. This view is supported by a study from Battista et al. (2022), in which the jet stream generated by C. andromeda umbrella pulsation was linked to feeding, nutrient and gas exchange as well as with the removal of excreta. While RGR would be expected to reflect changes in energy turnover, the RGR of the jellyfish exposed to 50–800 µmol photons m-2 s-1 did not differ. Overall, the mean RGRs observed in the tested range of PAR intensity manipulations were rather low and exhibited very large standard deviations. The poor growth performance of the medusa during this experiment may be a repercussion of spatial containment in the culture system. Hence, the measured RGRs cannot be considered as representative parameter for potential light effects, due to the applied PAR intensity changes. However, compared to all other treatments, the jellyfish that were exposed to narrow-band UVB radiation in addition to 200 µmol photons m-2 s-1 shrank significantly over the experimental period. These UVB-treated jellyfish produced notably more mucus than the jellyfish that were only exposed to PAR intensity changes, suggesting that the applied UVB dose led to harmful effects on the C. andromeda host tissue, causing the disintegration of mantle tissue.
In this study, the primary photosynthetic pigments detected in C. andromeda endosymbionts were chlorophyll a (Chl a) and c2 as well as the carotenoids peridinin (Per) and diadinoxanthin. This finding is consistent with the pigment profile reported in other endosymbiotic dinoflagellates (Hennige et al., 2009; Roth, 2014) and has also been identified in other jellyfish holobionts, including Cotylorhiza tuberculata (Enrique-Navarro et al., 2022). Overall, the pigments Chl a and Per dominated the light-harvesting complex (LHC) of C. andromeda endosymbionts. Under control conditions (100 µmol photons m-2 s-1) the mean concentration of Chl a and Per in the endosymbiotic microalgae ranged from 2–2.7 pg cell-1 and 1–1.5 pg cell-1, respectively. Chl a levels measured in other jellyfish holobionts were slightly lower than those reported in this study, including 1.33 pg cell-1 in C. tuberculata (Enrique-Navarro et al., 2022), 1–2.21 pg cell-1 in Cassiopea xamachana (Vodenichar, 1995; Verde and McCloskey, 1998; Estes et al., 2003), 2–2.1 pg cell-1 in Linuche unguiculata (Kremer et al., 1990; Wilkerson and Kremer, 1993), and 2 pg cell-1 in Mastigias sp. (McCloskey et al., 1994). When calculated as pigment concentration per C. andromeda dry weight, mean Chl a and Per contents ranged from 71–127 µg g-1 dry weight (DW) and from 38–78 µg g-1 DW, respectively, under control conditions in this study. Leone et al. (2013) reported much higher Per levels of 385 ± 49.6 µg g-1 DW in the C. tuberculata holobiont. However, it should be considered that comparisons of total pigment concentrations are difficult because differences in sample processing and handling can lead to marked variations in the final data.
Overall, C. andromeda proved to be a robust medium for the biosynthesis and accumulation of health-promoting pigments through its endosymbiotic microalgae, implying strong utilization potential. Pigments are exclusively synthesized by plants and algae, this means that sufficient levels of pigments such as carotenoids need to be obtained exogenously for conversion into functional metabolites that are indispensable for human cells (Chuyen and Eun, 2017). With respect to AOA, C. andromeda exhibited considerable mean levels thereof under control conditions (from 92–94 TE mmol 100 g-1 DW). The AOA levels measured in this study are substantially higher than the mean AOA levels of 1.63 ± 0.125 and 2.94 ± 0.28 TE mmol 100 g-1 measured recently by De Rinaldis et al. (2021) in dried C. andromeda umbrella and oral arms, respectively. However, the AOA levels of C. andromeda found in the current study are in a similar range to the AOA levels reported for different microalgae such as Haematococcus pluvialis (up to 197.4 TE mmol 100 g-1 dried supercritical H2O extract) (Rodríguez-Meizoso et al., 2010), Dunaliella salina (up to 111.8 TE mmol 100 g-1 dried hexane extract) (Herrero et al., 2006), and Chaetoceros sp. (102.9 TE mmol 100 g-1 dried dichloromethane extract) in terms of superoxide radical neutralization capacity (Guzman et al., 2001). The present data highlight the great potential of C. andromeda as a novel source of antioxidants for biofunctional purposes. Given that antioxidants are key mediators of endogenous ROS removal, a diet rich in antioxidants has been linked with many health benefits (Halliwell, 2000; Zampelas and Micha, 2015; Coulombier et al., 2021).
This study was developed to explore whether the synthesis of pigments, in particular Per can be specifically triggered in C. andromeda endosymbionts through ambient light manipulations, including PAR intensity changes and UVB exposure. Moreover, it was tested whether the concentration of pigments in the endosymbionts correlate positively with AOA in the overall tissue of C. andromeda holobionts. The results of these analyses indicated that the endosymbiotic microalgae, growing in hospite in adult C. andromeda medusae exhibited significantly higher concentrations of the dominant light-harvesting pigments Chl a and Per at lower PAR intensities (50-100 µmol photons m-2 s-1) compared to elevated PAR intensities (200-800 µmol photons m-2 s-1). Similar results were also found in a study by Supasri et al. (2021b), in which the synthesis of the valuable Per-chlorophyll a-protein (PCP) complex was brought to a peak, in an isolated culture of endosymbiotic Symbiodinium (S. tridacnidorum CS-73), through the lowest PAR intensity treatment (30 µmol photons m-2 s-1). The significantly highest concentrations of Per and Chl a pigments that were measured at the lowest PAR intensity (50 µmol photons m-2 s-1) in the present study, provide first evidence that pigment content can be triggered also in endosymbionts that grow in hospite in C. andromeda. Also low-dose UVB exposure (1.3 KJ m-2 day-1) in combination with 200 µmol photons m-2 s-1 led to significantly increased pigment levels in C. andromeda endosymbionts, compared to the control treatment without UVB. This discovery contradicts the generally accepted assumption that UVR exposure leads to photoinhibition in endosymbiotic cnidaria as a result of damage to the photosynthetic apparatus (e.g. Weis, 2008; Enrique-Navarro et al., 2022). Similarly, in the microalgae Nannochloropsis oceanica the concentrations of chlorophylls and carotenoids decreased significantly with increasing UVB (312 nm) dose, ranging from 3–22 KJ m-2 day-1 (Ljubic et al., 2020).
To clarify whether the measured changes in µg pigment per C. andromeda dry weight were triggered via PAR intensity- and UVB-induced pigment synthesis, within individual endosymbiont-cells, and not by variations in microalgae cell density in the C. andromeda tissue, the measured concentration of Chl a and Per were also quantified on a per microalgal-cell basis (pg microalgal cell-1). Although less pronounced, the effects of PAR intensity and UVB on the amounts of Chl a and Per per microalgae-cell, followed the same significant trend of increasing pigment concentration with decreasing PAR intensity and UVB exposure, which was also detectable in total pigment quantities per jellyfish dry weight. Thus, it can be concluded that the measured differences in pigment quantities resulted from LHC adjustments within the endosymbiotic microalgae, in response to the targeted PAR and UVB manipulations, growing in hospite in the C. andromeda mantle tissue.
Moreover, a significant shift in the ratio of Chl a and Per in the endosymbiotic microalgae due to PAR intensity changes was observed, which indicated that targeted PAR intensity exposure changed not only total pigment concentrations but also relative pigment composition. Overall, the present study provides new significant insights, which demonstrates for the first time that the synthesis of LHC pigments can be drastically manipulated in endosymbiotic dinoflagellates, growing in hospite, through PAR intensity changes and UVB exposure. These pioneering results shed new light on the debate regarding the extent to which ambient light changes can affect Symbiodiniaceae in cnidaria holobionts, despite protection through the host tissue. Previous studies have provided evidence for the assumption that optical properties of cnidarian tissue, in terms of scattering and absorption of the ambient light, shelter the endosymbionts from sub-optimal light conditions (Kühl et al., 1995; Roth, 2014). In these studies, only isolated endosymbiotic microalgae that were cultured ex hospite showed responses at the level of LHC pigment adjustments, due to ambient light changes. On the contrary, the present study shows that in hospite growing C. andromeda endosymbionts exhibited a clear pattern to adjust the concentration and composition of LHC pigments according to ambient PAR intensity changes. The decreased pigment synthesis at high PAR intensities observed in the current study might be a response of the microalgae to reduce the light-energy yield. For the same reason, the decrease of the Per/Chl a ratio with increasing PAR intensity may indicate a reduction of the overall LHC absorption spectrum, to prevent harmful effects due to excessive light energy. This assumption is strengthened by the measured Fv/Fm values, which indicated decreasing photosynthetic efficiency with increasing PAR intensity. The significantly enhanced pigment synthesis observed in response to UVB exposure presumably reflects the initiation of a protective response. However, given that the UVB-treated C. andromeda endosymbionts exhibited no photoinhibition in form of decreased Fv/Fm values, it can be assumed that the increased pigment synthesis was not yet a sign for photic stress, but rather a triggered form of UVB protection as has been demonstrated in terrestrial plants (Schreiner et al., 2017). Recent studies of UVB exposure in various terrestrial plant species have highlighted the regulatory properties of ecologically-relevant UVB radiation, which can trigger distinct changes in the levels of secondary plant metabolites such as carotenoids, chlorophylls, and flavonoids, ultimately leading to the desirable accumulation of these protective compounds for human dietary intake (Schreiner et al., 2009; Schreiner et al., 2012). In this line of research, the results of the present work are highly significant as they give a first indication that targeted low-dose UVB radiation can be used as an emerging technology to generate pigment-enriched food sources not only in terrestrial organisms but also in phototrophic marine organisms. Opposed to the mild UVB effects on C. andromeda endosymbionts, the UVB-exposed jellyfish host exhibited a strong stress response in the form of mucus production and drastic reduction of overall biomass. This suggests that most of the severe UVB stress was absorbed by the jellyfish tissue, raising the question to what extent the emitted UVB dose actually reached the endosymbionts in the jellyfish host tissue. The tissue of cnidarian holobionts is known to possess UVR-absorbing properties that can protect the endosymbionts therein (Higuchi et al., 2010; Enrique-Navarro et al., 2022). Moreover, mycosporine-like amino acids (MAA), which can be considered as functional UVR screen, were found in cnidarian holobionts (Banaszak and Lesser, 2009). In the endosymbiotic jellyfish Cassiopea xamachana, MAAs were synthesized by its own symbionts under UV (280–400 nm) exposure (Banaszak and Trench, 1995). Accounting for the different UVR-protection strategies of cnidaria holobionts, it can be assumed that only a fraction of the actual 1.3 KJ m-2 day-1 UVB dose reached the endosymbionts in this experiment. This underlines that only a very mild UVB exposure is required to create pigment-promoting conditions in the studied C. andromeda endosymbionts. Future studies should determine the optimal UVB dose for the C. andromeda holobiont, which increases the synthesis of Per without harmfully affecting the host tissue.
Since only the jellyfish host showed significant stress symptoms in response to UVB exposure, whereas Fv/Fm values indicated no sign of photoinhibition in the endosymbionts, it can be assumed that the significantly enhanced AOA levels in UVB-treated C. andromeda primarily reflect damage to the host tissue. The fact that overall AOA levels did not correlate positively with changes in light-harvesting pigment concentrations suggests that the LHC of the endosymbionts was not the dominant driver of AOA levels in the C. andromeda holobiont.
This is the first study, which reveals that C. andromeda endosymbionts growing in hospite adjust the total content and relative composition of light-harvesting pigments (LHP) as response to ambient PAR intensity changes. Moreover, we provided first evidence that narrow-band UVB exposure leads to elevated LHP content and increased antioxidant activity (AOA) in the C. andromeda holobiont. With these new findings, we also provide practical information to systematically valorize the yet underutilized C. andromeda biomass by specifically enhancing the content of LHPs and AOA. The so enriched C. andromeda jellyfish, with increased functional and health-promoting properties possesses diverse utilization potentials for new food and healthcare items. Given that the inclusion of antioxidants, chlorophyll, and carotenoid-based formulations in human diets is emerging as a new focus for the promotion of a healthy lifestyle, C. andromeda enriched in peridinin, chlorophyll a, and overall AOA may represent a promising source of antioxidant and anti-lipoperoxidant compounds with utilization potential as innovative food ingredients to supplement dietary formulations such as functional foods and nutraceuticals. Moreover, the implementation of LED-light optimized, modular C. andromeda cultivation systems may present an innovative and resource-saving opportunity to exploit this marine biomass also in urban and suburban surroundings. However, the current results represent only the very first insights into the potential of C. andromeda as prolific source of high-value bioproducts. In future studies the ambient light conditions, which trigger the optimal balance between C. andromeda biomass yield, LHP content (mainly peridinin) and overall AOA needs to be narrowed down further. This is particularly relevant for the treatment with UVB, which led in the current study to significantly increased LHP and AOA levels, but caused also a major reduction in overall C. andromeda biomass. Next to that, protocols for the extraction of pigment-protein complexes need to be established and evaluated for C. andromeda, to generate practical information on total LHP yields from light-optimized cultures.
The datasets presented in this study can be found in online repositories. The names of the repository/repositories and accession number(s) can be found below: https://issues.pangaea.de/browse/PDI-32852.
HK planned and conducted the experiment. This included the experimental setup, the design of light treatments, monitoring/measuring of physiological parameters and sampling of the organisms. Moreover, HK did the analysis and statistical exploration of the data. Finally, HK wrote the manuscript and directed its continuous revisions, consisting of the remarks from the co-authors, until he submitted the final version. MS provided crucial expertise in terms of the application of UVB radiation as tool to enhance health-promoting properties in photoactive organisms. MS was also taking the lead with revising the manuscript content and structure. AK secured the funding for this work and contributed proof-reading of the final manuscript. KS assisted HK with the design of the light treatments and gave guidance, concerning methods to determine photosynthetic efficiency. KS took also full responsibility of sample processing and analyses, with regards to the measurement of pigments and antioxidants. During the writing phase KS assisted HK with the interpretation of the obtained results. All authors contributed to the article and approved the submitted version.
This work is part of the research project “food4future” (F4F) funded by the Federal Ministry of Education and Research (BMBF) under the umbrella of the strategic research line Agricultural Systems of the Future (#FKZ 031B0730B).
The authors are grateful for the technical support from Silvia Hardenberg, Nico Steinel und José Garcia from the ZMT aquaculture unit. Great support in terms of expertise on the captivity culture of C. andromeda was also provided by Jana Wohlfahrt and Jens Bohn from the public aquarium of the Zoo in Rostock, Germany.
The authors declare that the research was conducted in the absence of any commercial or financial relationships that could be construed as a potential conflict of interest.
All claims expressed in this article are solely those of the authors and do not necessarily represent those of their affiliated organizations, or those of the publisher, the editors and the reviewers. Any product that may be evaluated in this article, or claim that may be made by its manufacturer, is not guaranteed or endorsed by the publisher.
The Supplementary Material for this article can be found online at: https://www.frontiersin.org/articles/10.3389/fmars.2023.1048346/full#supplementary-material
Arossa S., Barozzi A., Callegari M., Klein S. G., Parry A. J., Hung S. H., et al. (2021). The internal microenvironment of the symbiotic jellyfish cassiopea sp. from the red Sea. Front. Mar. Sci. 8, 705915. doi: 10.3389/fmars.2021.705915
Banaszak A. T., Lesser M. P. (2009). Effects of solar ultraviolet radiation on coral reef organisms. Photochem. Photobiological Sci. 8, 1276–1294. doi: 10.1039/b902763g
Banaszak A. T., Trench R. K. (1995). Effects of ultraviolet (UV) radiation on marine microalgal-invertebrate symbioses. II. the synthesis of mycosporine-like amino acids in response to exposure to UV in Anthopleura elegantissima and Cassiopeia xamachana. J. Exp. Mar. Biol. Ecol. 194, 233–250. doi: 10.1016/0022-0981(95)00073-9
Barlow R. G., Mantoura R. F. C., Gough M. A., Fileman T. W. (1993). Pigment signatures of the phytoplankton composition in the northeastern Atlantic during the 1990 spring bloom. Deep Sea Res. Part II: Topical Stud. Oceanogr. 40 (1-2), 459–477. doi: 10.1016/0967-0645(93)90027-K
Battista N., Gaddam M. G., Hamlet C. L., Hoover A. P., Miller L. A., Santhanakrishnan A. (2022). The presence of a substrate strengthens the jet generated by upside-down jellyfish. Front. Mar. Sci. 9, 847061. doi: 10.3389/fmars.2022.847061
Begum H., Yusoff F. M., Banerjee S., Khatoon H., Shariff M. (2016). Availability and utilization of pigments from microalgae. Crit. Rev. Food Sci. Nutr. 56 (13), 2209–2222. doi: 10.1080/10408398.2013.764841
Camacho-Muñoz D., Praptiwi R. A., Lawton L. A., Edwards C. (2021). High value phycotoxins from the dinoflagellate Prorocentrum. Front. Mar. Sci. 8, 638739. doi: 10.3389/fmars.2021.638739
Carbonera D., Di Valentin M., Spezia R., Mezzetti A. (2014). The unique photophysical properties of the peridinin-Chlorophyll-a-Protein. Curr. Protein Pept. Sci. 15, 332–350. doi: 10.2174/1389203715666140327111139
Chia S. R., Chew K. W., Leong H. Y., Manickam S., Show P. L., Nguyen T. H. P. (2020). Sonoprocessing-assisted solvent extraction for the recovery of pigment-protein complex from spirulina platensis. Chem. Eng. J. 398, 125613. doi: 10.1016/j.cej.2020.125613
Chuyen H. V., Eun J. B. (2017). Marine carotenoids: bioactivities and potential benefits to human health. Crit. Rev. Food Sci. Nutr. 57 (12), 2600–2610. doi: 10.1080/10408398.2015.1063477
Connolly J., Wayne P. (1996). Asymmetric competition between plant species. Oecologia 108, 311–320. doi: 10.1007/BF00334656
Coulombier N., Jauffrais T., Lebouvier N. (2021). Antioxidant compounds from microalgae: a review. Mar. Drugs 19 (10), 549. doi: 10.3390/md19100549
De Domenico S., De Rinaldis G., Paulmery M., Piraino S., Leone A. (2019). Barrel jellyfish (Rhizostoma pulmo) as source of antioxidant peptides. Mar. Drugs 17, 134. doi: 10.3390/md17020134
De Rinaldis G., Leone A., De Domenico S., Bosch-Belmar M., Slizyte R., Milisenda G., et al. (2021). Biochemical characterization of Cassiopea andromeda (Forsskål 1775), another red Sea jellyfish in the Western Mediterranean Sea. Mar. Drugs 19 (9), 498. doi: 10.3390/md1909498
Djeghri N., Pondaven P., Stibor H., Dawson M. N. (2019). Review of the diversity, traits, and ecology of zooxanthellate jellyfishes. Mar. Biol. 166, 1–19. doi: 10.1007/s00227-019-3581-6
Duarte I. M., Marques S. C., Leandro S. M., Calado R. (2022). An overview of jellyfish aquaculture: for food, feed, pharma and fun. Rev. Aquaculture 14 (1), 265–287. doi: 10.1111/raq.12597
Edelist D., Angel D. L., Canning-Clode J., Gueroun S. K., Aberle N., Javidpour J., et al. (2021). Jellyfishing in Europe: current status, knowledge gaps, and future directions towards a sustainable practice. Sustainability 13 (22), 12445. doi: 10.3390/su132212445
Enrique-Navarro A., Huertas E., Flander-Putrle V., Bartual A., Navarro G., Ruiz J., et al. (2022). Living inside a jellyfish: the symbiosis case study of host-specialized dinoflagellates, “Zooxanthellae”, and the scyphozoan Cotylorhiza tuberculata. Front. Mar. Sci. 9, 817312. doi: 10.3389/fmars.2022.817312
Estes A. M., Kempf S. C., Henry R. P. (2003). Localization and quantification of carbonic anhydrase activity in the symbiotic scyphozoan Cassiopea xamachana. Biol. Bull. 204, 278–289. doi: 10.2307/1543599
Fatma T. (2009). Screening of cyanobacteria for phycobiliproteins and effect of different environmental stress on its yield. Bull. Environ. Contamination Toxicol. 83 (4), 509–515. doi: 10.1007/s00128-009-9837-y
Fuentes-Grünewald C., Bayliss C., Fonlut F., Chapuli E. (2016). Long-term dinoflagellate culture performance in a commercial photobioreactor: Amphidinium carterae case. Bioresource Technol. 218, 533–540. doi: 10.1016/j.biortech.2016.06.128
Gallardo-Rodríguez J., Sánchez-Mirón A., García-Camacho F., López-Rosales L., Chisti Y., Molina-Grima E. (2012). Bioactives from microalgal dinoflagellates. Biotechnol. Adv. 30 (6), 1673–1684. doi: 10.1016/j.biotechadv.2012.07.005
Grossman A. R., Schaefer M. R., Chiang G. G., Collier J. L. (1993). Environmental effects on the light-harvesting complex of cyanobacteria. J. Bacteriol. 175 (3), 575–582. doi: 10.1128/jb.175.3.575-582.1993
Guzman S., Gato A., Calleja J. M. (2001). Antiinflammatory, analgesic and free radical scavenging activities of the marine microalgae Chlorella stigmatophora and Phaeodactylum tricornutum. Phytother. Res.: Int. J. Devoted to Pharmacol. Toxicological Eval. Natural Product Derivatives 15 (3), 224–230. doi: 10.1002/ptr.715
Halliwell B. (2000). The antioxidant paradox. Lancet 355 (9210), 1179–11180. doi: 10.1016/S0140-6736(00)02075-4
Hennige S. J., McGinley M. P., Grottoli A. G., Warner M. E. (2011). Photoinhibition of symbiodinium spp. within the reef corals Montastraea faveolata and Porites astreoides: implications for coral bleaching. Mar. Biol. 158 (11), 2515–2526. doi: 10.1007/s00227-011-1752-1
Hennige S. J., Suggett D. J., Warner M. E., McDougall K. E., Smith D. J. (2009). Photobiology of symbiodinium revisited: bio-physical and bio-optical signatures. Coral Reefs 28, 179–195. doi: 10.1007/s00338-008-0444-x
Herrero M., Jaime L., Martín-Álvarez P. J., Cifuentes A., Ibáñez E. (2006). Optimization of the extraction of antioxidants from Dunaliella salina microalga by pressurized liquids. J. Agric. Food Chem. 54 (15), 5597–5603. doi: 10.1021/jf060546q
Higuchi T., Fujimura H., Hitomi Y., Arakaki T., Oomori T., Suzuki Y. (2010). Photochemical formation of hydroxyl radicals in tissue extracts of the coral Galaxea fascicularis. Photochem. Photobiological Sci. 86, 1421–1426. doi: 10.1111/j.1751-1097.2010.00802.x
Iglesias-Prieto R., Govind N. S., Trench R. K. (1991). Apoprotein composition and spectroscopic characterization of the water-soluble peridinin–chlorophyll a–proteins from three symbiotic dinoflagellates. Proc. R. Soc. London Ser. B: Biol. Sci. 246 (1317), 275–283. doi: 10.1098/rspb.1991.0155
Iglesias-Prieto R., Govind N. S., Trench R. K. (1993). Isolation and characterization of three membranebound chlorophyll-protein complexes from four dinoflagellate species. Philos. Trans. R. Soc. London Ser. B: Biol. Sci. 340 (1294), 381–392. doi: 10.1098/rstb.1993.0080
Imamoglu E., Dalay M. C., Sukan F. V. (2009). Influences of different stress media and high light intensities on accumulation of astaxanthin in the green alga Haematococcus pluvialis. New Biotechnol. 26 (3–4), 199–204. doi: 10.1016/j.nbt.2009.08.007
Jeffrey S., Wright S., Zapata M. (2011). “Microalgal classes and their signature pigments,” in phytoplankton pigments,”. Eds. Roy S., Llewellyn C. A., Egeland E. S., Geir J. Phytoplankton Pigments Characterization, Chemotaxonomy and Applications in Oceaonagraphy (Cambridge: Cambridge University Press), 3–77.
Kühl M., Cohen Y., Dalsgaard T., Jørgensen B. B., Revsbech N. P. (1995). Microenvironment and photosynthesis of zooxanthellae in scleractinian corals studied with microsensors for O2, pH and light. Mar. Ecol. Progrss Ser. 117, 159–172. doi: 10.3354/meps117159
Koka P., Song P. S. (1977). The chromophore topography and binding environment of perididin. chlorophyll a. protein complexes from marine dinoflagellate algae. Biochim. Biophys. Acta 495 (2), 220–231. doi: 10.1016/0005-2795(77)90379-8
Kremer P., Costello J., Kremer J., Canino M. (1990). Significance of photosynthetic endosymbionts to the carbon budget of the scyphomedusa Linuche unguiculata. Limnol. Oceanogr. 35, 609–624. doi: 10.4319/lo.1990.35.3.0609
Lampert K. P. (2016). “Cassiopea and its zooxanthellae,” in The cnidaria, past, present and future (Cham: Springer), 415–423.
Lampert K. P., Bürger P., Striewski S., Tollrian R. (2012). Lack of association between color morphs of the jellyfish Cassiopea andromeda and zooxanthella clade. Mar. Ecol. 33, 364–369. doi: 10.1111/j.1439-0485.2011.00488.x
Leone A., Lecci R., Durante M., Meli F., Piraino S. (2015). The bright side of gelatinous blooms: nutraceutical value and antioxidant properties of three mediterranean jellyfish (Scyphozoa). Mar. Drugs 13, 4654–4681. doi: 10.3390/md13084654
Leone A., Lecci R. M., Durante M., Piraino S. (2013). Extract from the zooxanthellatae jellyfish Cotylorhiza tuberculata modulates gap junction intercellular communication in human cell cultures. Mar. Drugs 11, 1728–1762. doi: 10.3390/md11051728
Leone A., Lecci R. M., Milisenda G., Piraino S. (2019). Mediterranean Jellyfish as novel food: effects of thermal processing on antioxidant, phenolic, and protein contents. Eur. Food Res. Technol. 245, 1611–1627. doi: 10.1007/s00217-019-03248-6
Leong H. Y., Show P. L., Lim M. H., Ooi C. W., Ling T. C. (2018). Natural red pigments from plants and their health benefits: a review. Food Rev. Int. 34 (5), 463–482. doi: 10.1080/87559129.2017.1326935
Ljubic A., Jacobsen C., Holdt S. L., Jakobsen J. (2020). Microalgae Nannochloropsis oceanica as a future new natural source of vitamin D3. Food Chem. 320, 126627. doi: 10.1016/j.foodchem.2020.126627
Madhyastha H. K., Vatsala T. M. (2007). Pigment production in Spirulina fusiformis in different photophysical conditions. Biomol. Eng. 24 (3), 301–305. doi: 10.1016/j.bioeng.2007.04.001
Magnusson M., Mata L., Wang N., Zhao J., de Nys R., Paul N. A. (2015). Manipulating antioxidant content in macroalgae in intensive land-based cultivation systems for functional food applications. Algal Res. 8, 153–160. doi: 10.1016/j.algal.2015.02.007
Mammone M., Lavorano S., Rizzo L., Piraino S., Rossi S. (2021). High photosynthetic plasticity may reinforce invasiveness of upside-down zooxanthellate jellyfish in Mediterranean coastal waters. PloS One 16 (3), e0248814. doi: 10.1371/journal.pone.0248814
Maxwell K., Johnson G. N. (2000). Chlorophyll fluorescence–a practical guide. J. Exp. Bot. 51 (345), 659–668. doi: 10.1093/jexbot/51.345.659
McCloskey L. R., Muscatine L., Wilkerson F. P. (1994). Daily photosynthesis, respiration, and carbon budgets in a tropical marine jellyfish (Mastigias sp.). Mar. Biol. 119, 13–22. doi: 10.1007/BF00350101
Miyashita K., Nishikawa S., Beppu F., Tsukui T., Abe M., Hosokawa M. (2011). The allenic carotenoid fucoxanthin, a novel marine nutraceutical from brown seaweeds. J. Sci. Food Agric. 91 (7), 1166–1174. doi: 10.1002/jsfa.4353
Nomura T., Kikuchi M., Kubodera A., Kawakami Y. (1997). Proton-donative antioxidant activity of fucoxanthin with 1, 1-diphenyl-2-picrylhydrazyl (DPPH). IUBMB Life 42 (2), 361–370. doi: 10.1080/15216549700202761
Onodera K. I., Konishi Y., Taguchi T., Kiyoto S., Tominaga A. (2014). Peridinin from the marine symbiotic dinoflagellate, symbiodinium sp., regulates eosinophilia in mice. Mar. Drugs 12 (4), 1773–1787. doi: 10.3390/md12041773
Pisal D. S., Lele S. S. (2005). Carotenoid production from microalga, Dunaliella salina. Indian J. Biotechnol. 4, 476–483. Available at: http://nopr.niscpr.res.in/handle/123456789/5775
Polívka T., Hiller R. G., Frank H. A. (2007). Spectroscopy of the peridinin–chlorophyll-a protein: insight into light-harvesting strategy of marine algae. Arch. Biochem. biophys. 458 (2), 111–120. doi: 10.1016/j.abb.2006.10.006
R Core Team (2019). R: a language and environment for statistical computing (Vienna, Austria: R Foundation for Statistical Computing). Available at: http://www.r-project.org/.
Re R., Pellegrini N., Proteggente A., Pannala A., Yang M., Rice-Evans C. (1999). Antioxidant activity applying an improved ABTS radical cation decolorization assay. Free Radical Biol. Med. 26 (9-10), 1231–1237. doi: 10.1016/S0891-5849(98)00315-3
Rodríguez-Meizoso I., Jaime L., Santoyo S., Señoráns F. J., Cifuentes A., Ibáñez E. (2010). Subcritical water extraction and characterization of bioactive compounds from Haematococcus pluvialis microalga. J. Pharm. Biomed. Anal. 51 (2), 456–463. doi: 10.1016/j.jpba.2009.03.014
Roth M. S. (2014). The engine of the reef: photobiology of the coral-algal symbiosis. Front. Microbiol. 5, 422. doi: 10.3389/fmicb.2014.00422
Roth M. S., Latz M. I., Goericke R., Deheyn D. D. (2010). Green fluorescent protein regulation in the coral Acropora yongei during photoacclimation. J. Exp. Biol. 213, 3644–3655. doi: 10.1242/jeb.040881
Sachindra N. M., Sato E., Maeda H., Hosokawa M., Niwano Y., Kohno M., et al. (2007). Radical scavenging and singlet oxygen quenching activity of marine carotenoid fucoxanthin and its metabolites. J. Agric. Food Chem. 55 (21), 8516–8522. doi: 10.1021/jf071848a
Schreiber U. B. W. N., Bilger W., Neubauer C. (1995). “Chlorophyll fluorescence as a nonintrusive indicator for rapid assessment of in vivo photosynthesis,” in Ecophysiology of photosynthesis (Berlin, Heidelberg: Springer), 49–70.
Schreiner M., Krumbein A., Mewis I., Ulrichs C., Huyskens-Keil S. (2009). Short-term and moderate UV-b radiation effects on secondary plant metabolism in different organs of nasturtium (Tropaeolum majus l.). Innovative Food Sci. Emerging Technol. 10 (1), 93–96. doi: 10.1016/j.ifset.2008.10.001
Schreiner M. A., Mewis I., Huyskens-Keil S., Jansen M. A. K., Zrenner R., Winkler J. B., et al. (2012). UV-B induced secondary plant metabolites - potential benefits for plant and human health. Crit. Rev. Plant Sci. 31, 229–240. doi: 10.1080/07352689.2012.664979
Schreiner M. A., Wiesner M., Baldermann S., Hanschen F. S., Neugart S. (2017). UV-B induced changes in secondary plant metabolites. in: the role of UV-b radiation in plant growth and development. Ed. Jordan B. R. (Oxford, UK: CABI press), 39–57.
Sommer J., Kunzmann A., Stuthmann L. E., Springer K. (2022). The antioxidative potential of sea grapes (Caulerpa lentillifera, Chlorophyta) can be triggered by light to reach comparable values of pomegranate and other highly nutritious fruits. Plant Physiol. Rep. 27(1), 186–191. doi: 10.1007/s40502-021-00637-6
Song P. S., Koka P., Prezelin B. B., Haxo F. T. (1976). Molecular topology of the photosynthetic light-harvesting pigment complex, peridinin-chlorophyll a-protein, from marine dinoflagellates. Biochemistry 15 (20), 4422–4427. doi: 10.1021/bi00665a012
Sugawara T., Yamashita K., Sakai S., Asai A., Nagao A., Shiraishi T., et al. (2007). Induction of apoptosis in DLD-1 human colon cancer cells by peridinin isolated from the dinoflagellate, Heterocapsa triquetra. Biosci. biotechnol. Biochem. 71 (4), 1069–1072. doi: 10.1271/bbb.60597
Supasri K. M., Kumar M., Mathew M. J., Signal B., Padula M. P., Suggett D. J., et al. (2021b). Evaluation of filter, paramagnetic, and STAGETips aided workflows for proteome profiling of symbiodiniaceae dinoflagellate. Processes 9 (6), 983. doi: 10.3390/pr9060983
Supasri K. M., Kumar M., Segečová A., McCauley J. I., Herdean A., Padula M. P., et al. (2021a). Characterisation and bioactivity analysis of peridinin-chlorophyll a-protein (PCP) isolated from Symbiodinium tridacnidorum CS-73. J. Mar. Sci. Eng. 9 (12), 1387. doi: 10.3390/jmse9121387
Torregrosa-Crespo J., Montero Z., Fuentes J. L., Reig García-Galbis M., Garbayo I., Vílchez C., et al. (2018). Exploring the valuable carotenoids for the large-scale production by marine microorganisms. Mar. Drugs 16 (6), 203. doi: 10.3390/md16060203
Verde E. A., McCloskey L. R. (1998). Production, respiration, and photophysiology of the mangrove jellyfish Cassiopea xamachana symbiotic with zooxanthellae: effect of jellyfish size and season. Mar. Ecol. Prog. Ser. 168, 147–162. doi: 10.3354/meps168147
Vodenichar J. S. (1995). Ecological physiology of the scyphozoan cassiopea xamachana (Athens, USA: University of Georgia).
Weis V. M. (2008). Cellular mechanisms of cnidarian bleaching: stress causes the collapse of symbiosis. J. Exp. Biol. 211, 3059–3066. doi: 10.1242/jeb.009597
Welsh D. T., Dunn R. J. K., Meziane T. (2009). Oxygen and nutrient dynamics of the upside- down jellyfish (Cassiopea sp.) and its influence on benthic nutrient exchanges and primary production. Hydrobiologia 635, 351–362. doi: 10.1007/s10750-009-9928-0
Wilkerson F. P., Kremer P. (1993). DIN, DON and PO∼4 flux by a medusa with algal symbionts. Mar. Ecol. Prog. Ser. 90, 237–250. doi: 10.3354/meps090237
Wright S. W., Jeffrey S. W., Mantoura R. F. C., Llewellyn C. A., Bjørnland T., Repeta D., et al. (1991). Improved HPLC method for the analysis of chlorophylls and carotenoids from marine phytoplankton. Mar. Ecol. Prog. Ser., 183–196. doi: 10.3354/meps077183
Wyman M., Fay P. (1987). “Acclimation to the natural light climate,” in The cyanobacteria. Eds. Fay P., van Baalen C. (Amsterdam: Elsevier), 347–376.
Keywords: Light-harvesting pigments, carotenoids, endosymbiotic dinoflagellates, symbiodiniaceae, up-side down jellyfish, ultraviolet radiation (UVR) LEDs, health-promoting ingredients, new marine food
Citation: Kühnhold H, Schreiner M, Kunzmann A and Springer K (2023) Light intensity changes and UVB radiation affect peridinin content and antioxidant activity in the Cassiopea andromeda holobiont. Front. Mar. Sci. 10:1048346. doi: 10.3389/fmars.2023.1048346
Received: 19 September 2022; Accepted: 03 May 2023;
Published: 18 May 2023.
Edited by:
Christophe Brunet, Anton Dohrn Zoological Station Naples, ItalyReviewed by:
Milan Szabo, Eötvös Loránd Research Network (ELKH), HungaryCopyright © 2023 Kühnhold, Schreiner, Kunzmann and Springer. This is an open-access article distributed under the terms of the Creative Commons Attribution License (CC BY). The use, distribution or reproduction in other forums is permitted, provided the original author(s) and the copyright owner(s) are credited and that the original publication in this journal is cited, in accordance with accepted academic practice. No use, distribution or reproduction is permitted which does not comply with these terms.
*Correspondence: Holger Kühnhold, aG9sZ2VyLmt1ZWhuaG9sZEBsZWlibml6LXptdC5kZQ==
Disclaimer: All claims expressed in this article are solely those of the authors and do not necessarily represent those of their affiliated organizations, or those of the publisher, the editors and the reviewers. Any product that may be evaluated in this article or claim that may be made by its manufacturer is not guaranteed or endorsed by the publisher.
Research integrity at Frontiers
Learn more about the work of our research integrity team to safeguard the quality of each article we publish.