- 1Fisheries and Oceans Canada, Institute of Ocean Sciences, Sidney, BC, Canada
- 2Swire Institute of Marine Science and Area of Ecology and Biodiversity, School of Biological Sciences, The University of Hong Kong, Hong Kong, Hong Kong SAR, China
- 3Fisheries and Oceans Canada, Pacific Biological Station, Nanaimo, BC, Canada
- 4Department of Biology, University of Victoria, Victoria, BC, Canada
Euphausiids form a critical component of oceanic food chains and individual species vary in their responses to perturbation events. We aimed to establish if patterns of fine scale oceanographic variability and larger-scale climate events such as marine heatwaves (MHWs) could be linked with spring variability in the biomass of two key forage species in the northeast Pacific Ocean, Euphausia pacifica and Thysanoessa spinifera. To achieve this, we used long-term datasets from the west coast of Vancouver Island Canada, an important commercial fishing area, to first quantify interannual signals of variability in fine-scale oceanographic conditions using multivariate analysis. We then used geostatistical spatiotemporal modelling to quantify the effects on species-specific euphausiid biomass. Oceanographic data showed that the effects of warm events are not always observable, and effects vary across small spatial scales. Warming due to the 2014-2016 MHW was relatively mild on the continental shelf during spring (<1°C above climatology). Spring biomass of euphausiids, particularly E. pacifica, peaked in 2015, and all euphausiid groups analysed (E. pacifica, T. spinifera and total euphausiids) exhibited significant correlations with positive phases of the Pacific Decadal Oscillation. These results have implications for marine predators as euphausiids may act as system stabilisers in the northeast Pacific, thereby potentially increasing ecosystem resilience during extreme events.
1. Introduction
Understanding how lower trophic levels respond to environmental change is an important component of ecosystem-based management. In the northeast Pacific Ocean, euphausiids are important prey for a wide range of marine predators, including whales and seabirds (Abraham and Sydeman, 2004; Hipfner, 2009; Nickels et al., 2019), and an array of commercially important fish species such as Pacific hake (Merluccius productus), Pacific herring (Clupea pallasii), sablefish (Anoplopoma fimbria) and Pacific salmon (Tanasichuk et al., 1991; Robinson, 2000; Beamish et al., 2004). Euphausiids form dense aggregations which are inherently patchily distributed through the marine environment. These patches usually co-occur with areas of elevated primary production, and their distribution can be linked at various scales to local changes in currents and upwelling, and to basin-scale indices such as El Niño and the Pacific Decadal Oscillation (PDO) (e.g. Ressler et al., 2005; Swartzman et al., 2005; Santora et al., 2011; Fiechter et al., 2020).
Over the last few decades, marine ecosystems in the northeast Pacific have undergone numerous climate-related shifts, and the frequency of anomalous warm events such as El Niño and marine heatwaves have increased (McGowan, 1998; Sydeman et al., 2013). Fluctuations between the different climate modes of the El Niño Southern Oscillation (ENSO), the Pacific Decadal Oscillation (PDO) and the North Pacific Gyre Oscillation (NPGO) can alter alongshore wind direction and strength, in turn affecting the timing of the spring transition, upwelling intensity, and therefore nutrient delivery to the coastal zone (Mantua and Hare, 2002; Peterson and Schwing, 2003; Di Lorenzo et al., 2008). Alternative modes of these indices can alter the biomass, distribution and availability of prey, leading to direct implications for foraging marine predators, such as changes in distribution (Sprogis et al., 2018), diet (Gladics et al., 2014; Hipfner et al., 2020), and breeding success (Sydeman et al., 2006; Hipfner, 2008; Wolf et al., 2009).
Several marine heatwaves (MHWs) have been documented in the northeast Pacific in recent years (Cheung and Frölicher, 2020; Scannell et al., 2020). The MHW that persisted in the northeast Pacific Ocean through 2014-2016, known as the ‘blob’, was one of the most extreme on record in terms of intensity and duration, and led to widespread food web disruption (Di Lorenzo and Mantua, 2016; Sen Gupta et al., 2020). This event has been followed by several other MHWs which were less intense in terms of duration and ecosystem responses (Amaya et al., 2020). During the 2014-2016 event, marine predator mortality occurred from Alaska to southern California, thought to be associated with a reduction in the biomass, size and energy content of colder-water associated macro-zooplankton (Brodeur et al., 2019; Robertson and Bjorkstedt, 2020; Arimitsu et al., 2021). In the California Current, the effects of these MHW events can vary by latitude due to changes in the strength and direction of currents. In the tropics, reduced local abundance and biodiversity often occurs while the opposite is observed at higher latitudes due to an influx of more tropical species following warmer waters northward, such as the subtropical euphausiid N. simplex. This species usual northern extent is off northern California, but during the 1958, 1983, and 1998 El Niño events it was detected to Oregon and even northwest Vancouver Island (Brinton, 1960; Brodeur, 1986; Mackas and Galbraith, 2002; Santora et al., 2017).
The two most abundant species of euphausiids in the northern California Current, Thysanoessa spinifera and Euphausia pacifica, have exhibited varying responses in abundance and distribution to variability in ocean conditions and climate modes. Following the El Niño warm event of 1997-1998 and a shift in PDO sign from positive to negative, T. spinifera biomass off Vancouver Island increased, while E. pacifica biomass decreased (Mackas et al., 2001; Lu et al., 2003). Results off central California suggest that the 2014-2016 MHW may have more negatively affected T. spinifera biomass than E. pacifica, but that T. spinifera biomass recovered more quickly than E. pacifica in the years following the event, with peak abundance in 2017 (while peak of E. pacifica abundance occurred in 2008; Cimino et al., 2020). T. spinifera is slightly larger and has a higher energy content than E. pacifica, and there is evidence of species-selectivity by some euphausiid predators in the California Current (Abraham and Sydeman, 2006; Wells et al., 2012; Nickels et al., 2018). Variability in the abundance and availability of these two euphausiid species through different climate cycles is therefore likely to affect foraging conditions for marine predators. However, comparatively few studies in the northeast Pacific have looked at euphausiid-environment interactions at species level (but see Mackas et al., 2013; Sydeman et al., 2013; Kalyn, 2014; Qualls, 2019; Cimino et al., 2020).
The west coast of Vancouver Island encompasses both the northern terminus of the equator-ward flowing California Current and the transition zone with the poleward-flowing Alaska Current to the north (see Figure 1). This area is one of the most commercially important fishing regions in Canada (McFarlane et al., 1997; Ware and Thomson, 2005). The upwelling system along the continental slope west of Vancouver Island (southern shelf bioregion, hereafter south bioregion) is separated from the area to the north of Vancouver Island where downwelling is dominant (northern shelf bioregion, hereafter north bioregion). The offshore region encompasses the deeper part of the continental slope and Pacific Ocean proper and is less affected by upwelling activity, but perhaps more affected by large-scale events such as MHWs. Large spatial differences exist in local oceanographic conditions within the transition zone of the California and Alaska Currents off the northwest coast. Studies linking euphausiid distribution with either bottom up or top down pressures have focused on the highly productive southwest region encompassing the La Perouse Bank and the Juan de Fuca Eddy and canyon system (e.g. McFarlane et al., 1997; Robinson, 2000; Allen et al., 2001; Mackas et al., 2001). Few studies have examined euphausiid dynamics across the whole region during periods of extreme ocean warming (but see Lu et al., 2003; Mackas et al., 2004).
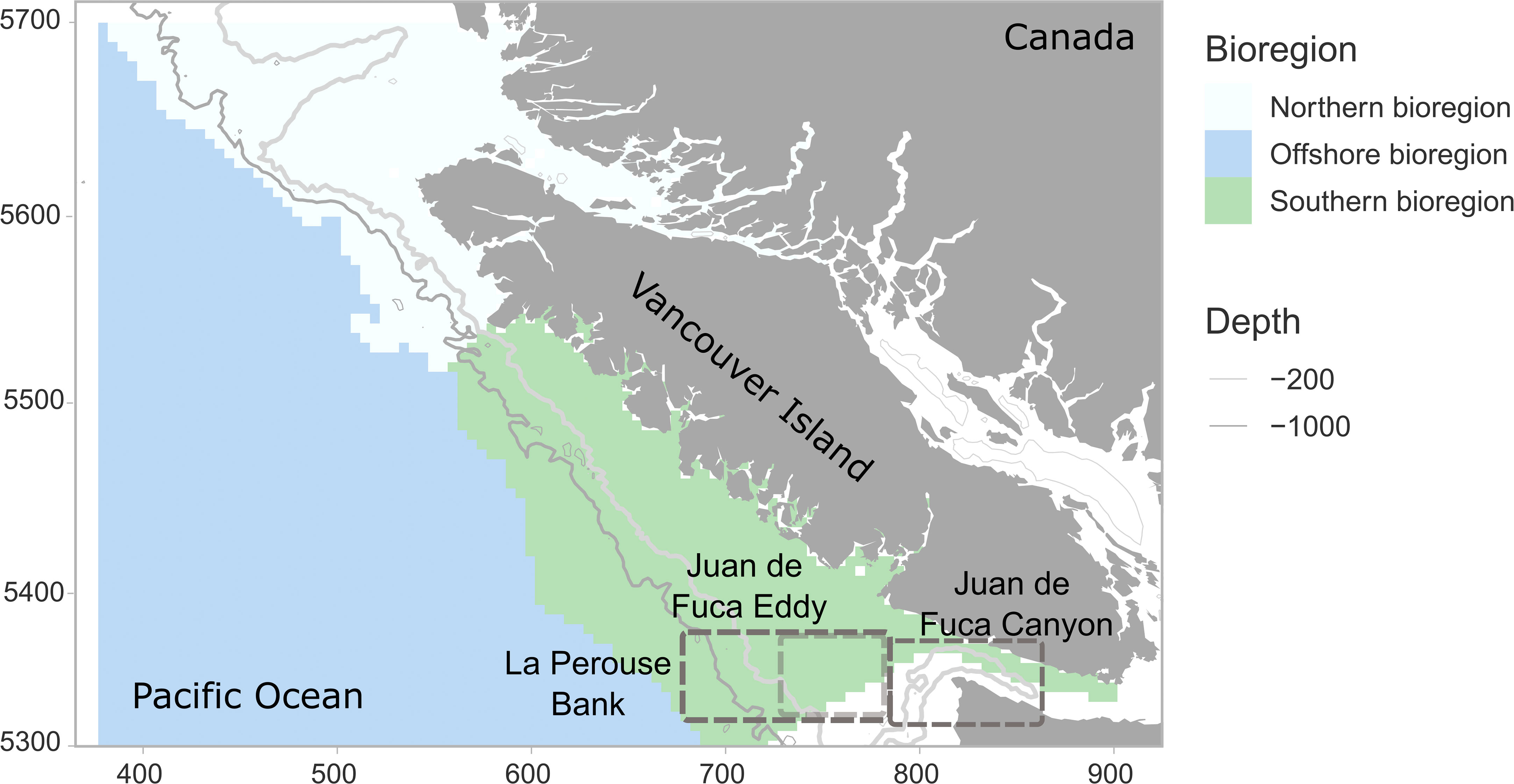
Figure 1 The study area showing boundaries for the southern, northern, and offshore bioregions. Depth contours are shown for 200m and 1000m, indicating the position of the continental shelf and slope and the Juan de Fuca canyon. The location of the La Perouse Bank, the Juan de Fuca Canyon, and the Juan de Fuca Eddy is indicated by grey boxes (the eddy position is shown by a lighter grey box). X and Y axis units are Universal Transverse Mercator in km (UTM - zone 9).
The main aim of this work was to evaluate the effects of fluctuations in oceanography and climate on the distribution of two key euphausiid species on the west coast of Vancouver Island, E. pacifica and T. spinifera. This is a region of complex oceanography, exhibiting both high spatial and temporal variability among years. The first step in this study was to analyse the links between fine-scale oceanographic conditions such as temperature and salinity with broader patterns in annual climate. For example, 1). Were there spatial differences between bioregions in temperature and current patterns that reflected the transition from the California Current with conditions in the Alaska Current, and 2). Were there temporal differences in physical conditions that reflected large-scale northeast Pacific Ocean events such as the 2014-2016 MHW? The second step was to determine how oceanographic variability associated with larger-scale Pacific fluctuations affected the biomass of euphausiids on the shelf and slope in spring, using spatiotemporal species distribution models. We hypothesized that the occurrence of the MHW event in 2014-2016 would negatively affect surveyed biomass of euphausiids based on observed responses from macrozooplankton communities in other areas of the northeast Pacific (e.g. Lavaniegos et al., 2019; Robertson and Bjorkstedt, 2020) and that patterns would be different between species, with T. spinifera more negatively impacted by warm events than E. pacifica. Although both species exhibit a wide distribution, T. spinifera is typically more northerly associated while E. pacifica is distributed further south (Brinton, 1976; Brinton, 1981). Understanding the links between fine-scale environmental patterns, larger-scale climate events and the flow-on effects for secondary producers such as euphausiids supporting diverse marine predators, is essential for predicting the resilience of marine systems to future climatic variability.
2. Methods
Euphausiid biomass and oceanographic conditions have been surveyed off the west coast of Vancouver Island, Canada with regularity since the 1970s (see Figure 1 for the boundaries of the study region). We used data between 1996 and 2019 as this period exhibited the most consistent spatial coverage. Seasonal variability was eliminated by analysing only euphausiid and in situ physical data collected in spring (April, May, June). This period also highlighted differences in the timing of the spring transition between years, which usually occurs between April and June on this part of the coast and initiates primary and secondary production cycles. It is also an important period for the arrival of southern migratory fish species such as Pacific hake, Pacific sardine, and Pacific mackerel that spend the summer foraging along the continental shelf (Demer et al., 2012; Malick et al., 2020).
This analysis is split into two parts to address the two-step aim of this study outlined above. We first analysed variability in local physical variables and larger scale climate indices in each bioregion using Principal Component Analysis, to characterise spatial differences between bioregions and interannual variability in oceanography between years, and to explore how this fine-scale in situ variability related to larger-scale Pacific Ocean patterns such as the Pacific Decadal Oscillation (bioregions boundaries are shown in Figure 1). This part of the analysis highlighted specific variables that were important in driving the oceanographic signature on the shelf during spring and were used in the next analytical step. The second analysis used a series of species-specific spatiotemporal species distribution models to assess the relative importance of these ‘dominant’ environmental variables for structuring the biomass of E. pacifica, T. spinifera and total euphausiid biomass (all species combined). We used a geostatistical distribution modelling approach (Shelton et al., 2014; Thorson et al., 2015) using a long time-series (23 years) of euphausiid biomass data.
2.1. Euphausiid data collection and processing
Data on the biomass of E. pacifica and T. spinifera were obtained through a variety of surveys carrying out zooplankton net-tows along the west coast of Vancouver Island (Figure 1). These data have been collated systematically and are archived in the Institute of Ocean Science (IOS) Zooplankton database (https://open.canada.ca/data/en/dataset/9447ecf8-a7f7-4904-8ab0-3c597c534c4b). Euphausiid samples were collected mostly by vertical hauls, with a small number by oblique hauls (92%, 8% respectively) using paired double bongo nets from near-bottom or a maximum of 250m depth to the surface (net diameter 56-58mm and 236-251μm mesh size). The maximum depth was set to 250m for several reasons; this depth allowed us to include the most data as most tows in the dataset were to ~250m, and this depth sampled the largest proportion of euphausiids as they tend to be distributed in the water column between 50 and 250m during the day. In addition, previous studies on euphausiids in this area used this depth as the maximum sampling depth at offshore locations, allowing this study to be comparable to previous work. Samples were truncated from the analysis when this protocol was not observed and there was large (+30m) disparity between the bottom depth and the lowest sampling depth. Vertical and oblique hauls were considered comparable for biomass estimates when corrected via the volume of water filtered by the net which was determined by a flowmeter. We analyzed data from daylight hauls only, as these were more numerous than night hauls. Paired bongo nets are biased towards the collection of smaller zooplankton, and there is a substantial bias for adult euphausiids which can avoid the net when sampled during the day (Shaw and Robinson, 1998). We applied a correction factor to the data to help account for this bias developed based on night-time euphausiid tows using black nets and strobes, which is considered best practice for catching adult euphausiids (see Mackas et al., 2001). Biases were assumed to be consistent across high and low densities of euphausiids, with biomass estimates likely underestimated consistently both spatially and across the time-series. Biomass values of adults only (euphausiids ≥10mm in length) were standardised via the volume filtered and sampling depth to vertically integrated dry weight biomass mg m2, which is considered more robust to variability in sampling depth than volumetric biomass estimates (Ohman and Lavaniegos, 2002). More detailed euphausiid sampling, processing, and laboratory protocols can be found in the Supplementary Material. All described patterns in this manuscript are reflective of adult euphausiids only.
2.2. Physical variables, upwelling indicators and climate indicators
Surface to near-bottom depth profiles of various water-column properties were obtained using a Rosette sampler fitted with a Sea-Bird 911+ CTD (Conductivity, Temperature and Depth sensor), often in tandem with zooplankton tows. CTDs were matched in time and space with zooplankton tows: CTD casts which occurred on the same day and within 5km were selected. We discarded zooplankton stations that lacked a corresponding CTD sample from the analysis resulting in a dataset of n=673 individual sampling points across 23 years (see Supplementary Material Figure S1). Temperature and salinity were used to calculate in situ water column properties using the R package ‘oce’ (Kelley and Richards, 2021) for each cast selected (see Figure S1 for spatial distribution of stations). We calculated mixed layer depth (m), mean temperature (°C), salinity and density (kg/m3) within the mixed layer and below the mixed layer, stratification (density difference between the mixed layer density and the sub-mixed layer density, kg/m3), and depth of the 26.0 density isopycnal (m). This metric gives an in situ indication of upwelling, as shallower depths indicated higher isopycnal tilts at the coast (e.g. Mackas et al., 1997). See Supplementary Methods for all water-column calculations.
Upwelling indicators (NOAA Fisheries – Southwest Fisheries Science Centre (see https://oceanview.pfeg.noaa.gov/products/upwelling/bakun), were based on monthly mean data from the Bakun upwelling index at 48°N 125°W (Bakun, 1973), which was interpolated to a 1-degree coastal grid. We obtained monthly mean data for the North-South component of geostrophic wind (m s-1), overall magnitude (speed) of surface wind (m s-1), geostrophic wind-stress-curl (MPa m-1), and the North-South and East-West components of Ekman transport (kg m-1 s-1). In the south bioregion (the start of the California Current system), the spring transition from winter downwelling to summer upwelling is triggered by a switch in wind direction from northward to southward, driving the westward (offshore) movement of surface water through Ekman transport. Upwelling can also be driven through positive wind stress curl, through a mechanism called Ekman pumping (Wang et al., 2011). We extracted data between 1996 and 2019 for the months of April, May and June, to match the timing of euphausiid data and other environmental covariates.
The Ocean Niño Index (ONI) was used as an indicator of El Niño and La Niña events for the eastern tropical Pacific (https://origin.cpc.ncep.noaa.gov/products/analysis_monitoring/ensostuff/ONI_v5.php). Data were aggregated into 3-month timesteps so we used values for April-May-June to match euphausiid sampling data. To capture longer-term oscillation patterns, we used the Pacific Decadal Oscillation (PDO) and the North Pacific Gyre Oscillation (NPGO). Both PDO and NGPO data had monthly values and were directly matched to euphausiid sampling months. The PDO index (based on SST) was downloaded from https://psl.noaa.gov/data/correlation/pdo.data and represents ocean-atmosphere variability over the mid-latitude north Pacific basin (Mantua and Hare, 2002). The NPGO was chosen to reflect variability in salinity, nutrient upwelling, and surface chlorophyll‐a (Chl‐a), therefore potentially providing a closer link with biological conditions in the northeast Pacific (Di Lorenzo et al., 2008, data downloaded from: www.o3d.org/npgo/). There is evidence that winter ENSO, PDO and NPGO are strongly correlated with spring SST and upwelling, and therefore could influence biological productivity at a lag of one season (Black et al., 2011; Chenillat et al., 2012). We included both a 3-month lag (winter mean - December, January, February) and no lag (spring mean - April, May, June) in further analyses.
2.3. Statistical analyses
2.3.1. Multivariate analyses to characterise oceanographic conditions
We first characterised interannual variability in the oceanography of each spatial bioregion in spring using Principal Component Analyses (PCAs). Physical data were averaged across stations within each bioregion for each year, creating a spring climatology which filtered out local station to station variability and allowed us to focus on interannual variability. A PCA for each bioregion was constructed using the R package ‘vegan’ (Oksanen et al., 2020). All covariates were scaled prior to PCA by subtracting their mean and dividing by 1 standard deviation. In PCA, the first two principal components (PC1 and PC2) explain the highest percentage of variability in the original dataset and provide a lower dimensional representation of the underlying patterns, with some variables loading positively and some negatively across each axis. Positive or negative loadings across these axis group or separate years with similar or different conditions.
2.3.2. Spatiotemporal modelling of euphausiid biomass
We modelled the spatiotemporal distribution of E. pacifica and T. spinifera using a specialised framework which includes the Integrated Nested Laplace Approximation (INLA, Rue et al., 2009) combined with Stochastic Partial Differential Equations (SPDE, Lindgren et al., 2011). This approach involves constructing a spatial mesh as an approximation of the Gaussian Markov random field, which compensates for irregularity of sampling between time steps (here, years) by interpolating predictions to other locations across the mesh (Lindgren and Rue, 2015). This framework also makes these models excellent at handling both spatial and temporal autocorrelation, which is a common problem with this type of survey data. Unmeasured spatial and spatiotemporal environmental variability was accounted for through the spatial and spatiotemporal random effect structure, in this case independent and identically distributed (IID) random fields estimated for each year. Our spatial mesh used vertices selected using a k-means algorithm (Shelton et al., 2014) at a specified number of locations or ‘knots’. These models have been shown to be highly effective for species distribution modelling of biomass data collected through surveys (Shelton et al., 2014; Ward et al., 2015). Our model was based on a generalised additive modelling (GAM) framework as exploratory analysis indicated non-linearity of relationships between predictors and response variables. We included fixed effects which represent variability in the response attributed to key environmental variables which were identified through the PCA described above in the first analytical step. An additional random effect for year was included to give the model flexibility to allow some variation in overall mean biomass outside of the variability explained via the fixed effects and spatiotemporal random fields.
Total euphausiid biomass (E. pacifca + T. spinifera + other euphausiid species; n=13) was also modelled as a comparison to inform and support future work in this region that might use acoustically derived biomass of euphausiids (which is not species-specific). All euphausiid biomass data were continuous and zero-inflated, therefore we used the Tweedie distribution with a log-link for all models (Tweedie, 1984; Dunn and Smyth, 2005; Wood et al., 2016). Models were fit using the R package sdmTMB (Anderson et al., 2020), We confirmed that models had converged by checking that the Hessian matrix was positive and the maximum absolute gradient across fixed effects was < 0.005.
We used PCA to inform covariate selection; results indicated some envrionmental variables were more important in driving local conditions than others. For example, we assumed that variables which loaded strongly across the first two PC axes, and were common between bioregions, were having a large effect on the local environment and should be included in euphausiid modelling. Therefore, we chose mixed-layer temperature over sub-mixed layer temperature, sub-mixed layer salinity over mixed-layer salinity, and the PDO over ONI or NPGO (see Table S2 for loadings; strong loadings are highlighted in bold), although we still tested the inclusion of alternative climate variables in models. Out of the upwelling indicator variables, both E-W Ekman and N-S surface wind loaded strongly, therefore we ran separate models for each variable with the other omitted as they were highly correlated. The same was true for wind-stress-curl vs magnitude of surface wind, and spring PDO vs previous winter PDO (see Table S3). This iterative process helped in highlighting variables with the strongest statistical significance, even if they were equally suspected of having biological relevance.
Mixed-layer temperature and sub-mixed-layer salinity were fixed variables included in every testing iteration of models, as indicated by PCA. We then ran eight full models for each euphausiid species testing the addition of every combination of other strongly loading variables outlined above. The most parsimonious covariate combination was selected based on AIC and inspection of the model residuals. We also tested the inclusion of an interaction term for mixed layer depth and mixed-layer temperature (which are inter-related due to upwelling in the spring), and all models performed better with the inclusion of this term. For all species, the same covariate combination exhibited the lowest AIC, therefore we used the same variables in final models for E. pacifica, T. spinifera and total euphausiids. Conditional effects plots were used to explore the relationship between the response and each of the predictors in the models (E. pacifica, T. spinifera and total euphausiids) with all other variables held at their median values.
From these models, we predicted an index of relative biomass trends through time and across space. To do this, we required a prediction grid containing estimates of all the covariates at the same points in space in each year. We used data from the same CTD casts as in the euphausiid models and all other CTD casts from surveys in the study region which occurred in spring (April, May, June) but that we had previously discarded as they did not have a corresponding euphausiid station, to create models of these covariates with independent and identically distributed spatiotemporal random fields. No fixed effects were included in these models, therefore predictions were akin to smoothed spatiotemporal averages while making no assumptions about mechanisms. A quadratic effect was included for month which followed a random walk by year so that each month was related to the one before it, both within and between years. Predictions of covariates were made over the 5km2 grid (UTM zone 9 projection) for the month of May for each year of sampling (between 1996 and 2019), to eliminate month to month variability. All cells with predicted conditions outside the range of the observed data were excluded. Observations vs. predictions for each covariate model are shown in Figure S3. This covariate grid was used to calculate average biomass distributions and interannual relative biomass indices for each species.
3. Results
E. pacifica and T. spinifera were the dominant species of euphausiid in the study region between 1996 and 2019 (Table 1). T. inspinata was the next most numerous species, sometimes accounting for ~30% of a sample. However, the biomass of this species was too inconsistent across years to allow modelling. All other euphausiid species contributed less than 5% of the total community (see Table 1).
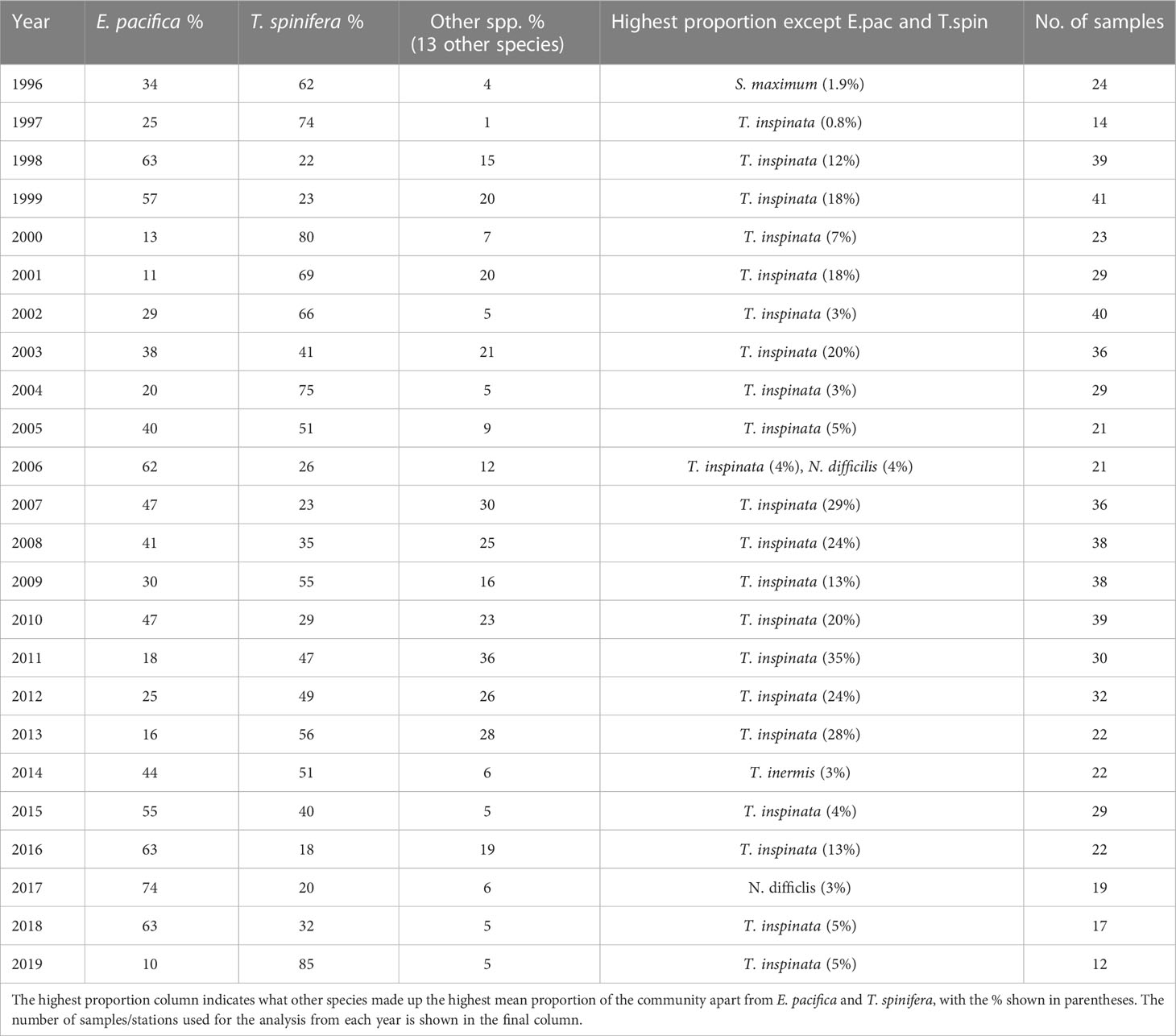
Table 1 Mean % contribution of E. pacifica, T. spinifera, and other spp. to the overall euphausiid community at sampling stations off the west coast of Vancouver Island between 1996 and 2019.
3.1. Interannual and regional variability in environmental conditions
Several trends were apparent through the time-series; southward wind and offshore Ekman current became increasingly negative, indicating increased upwelling, or an earlier transition from winter downwelling to summer upwelling (Figure 2). In addition, wind-stress-curl became increasingly positive which also leads to increased upwelling through Ekman pumping (Ekman suction). These patterns were present in all three bioregions. In the offshore bioregion there was an increase in stratification across the water column that resulted in a decrease in temperature and an increase in salinity and density of the sub-mixed layer.
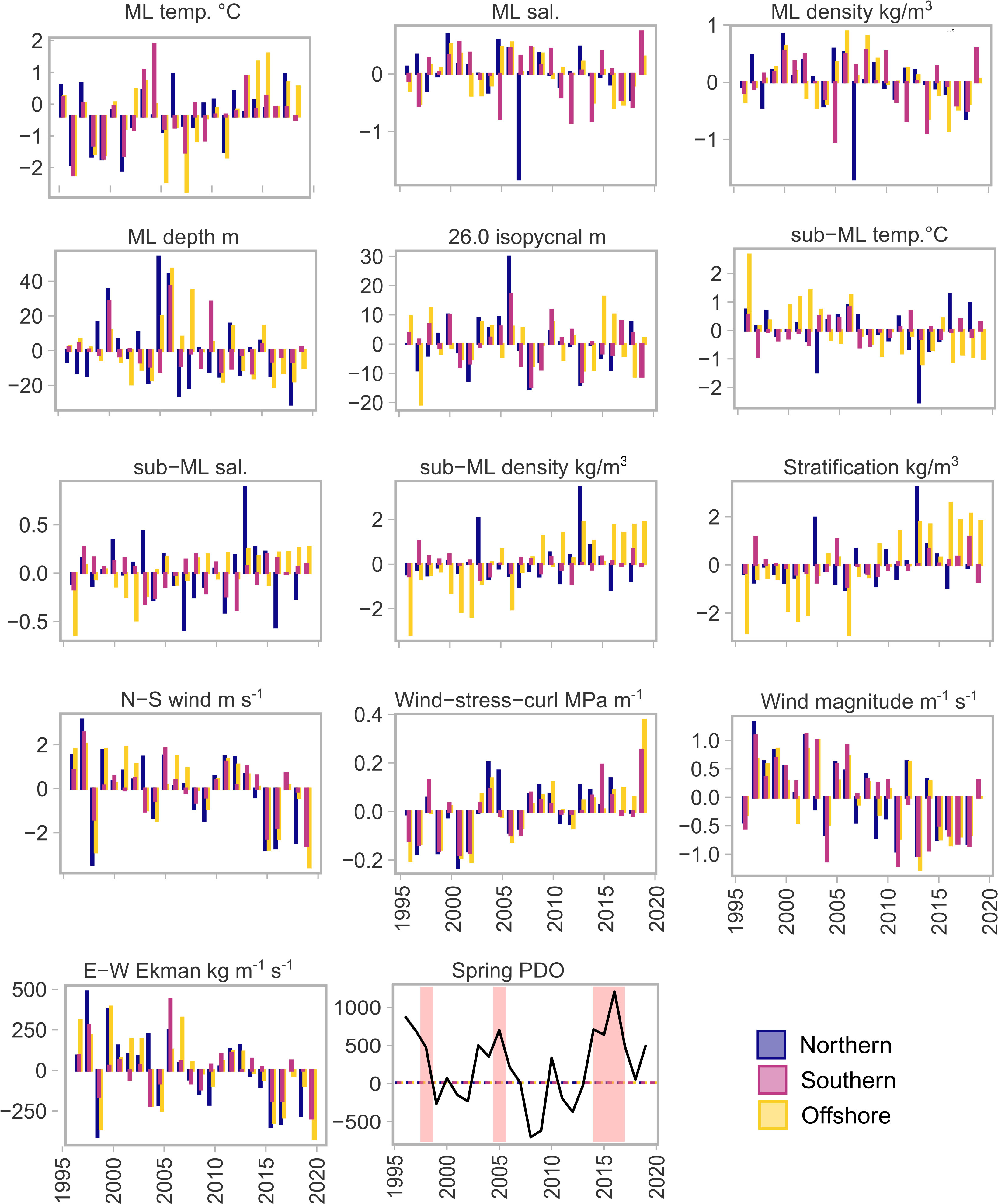
Figure 2 Annual mean anomaly (across stations) for each bioregion, of all variables considered in multivariate and spatiotemporal analysis of ocean-climate conditions. Spring PDO shows the PDO phase during April, May and June of each year - red highlighted regions represent ‘warm’ periods that are mentioned in the text; the 1997 El Niño, the 2005 ‘warm’ event, and the 2014-2016 MHW event. Anomalies were calculated based on the time-series analysed: 1996-2019. ML, mixed layer; temp., temperature °C; sal., salinity; N-S, north-south; E-W, east-west. See Figure 1 for bioregion boundaries.
The years with El Niño-like warm events displayed slightly different characteristics to years where El Niño’s also coincided with marine heatwaves (spring 2015 and 2016). The El Niño of 1997 and the El Niño-like conditions in 2005 were characterised by strong eastward (onshore, positive) Ekman current indicating downwelling (Figure 2), and perhaps a later transition to spring upwelling. The spring of 2015 and 2016 however, were characterised by strong negative (offshore) Ekman transport in all three bioregions, driven by southward meridional winds (Figure 2).
The temperature of the water column during the years categorised as ‘warm’ (spring 1997/8, 2005, 2014-2016 and 2019), was also quite variable between years and not consistent across bioregions. In the MHW years of 2015-2016 the event was observable in the offshore bioregion, with spring mixed layer temperatures 1-2°C higher than the mean, but on the continental shelf in the south and north bioregion temperatures were only ~0.5°C above the long-term mean (Figure 2). Both 1998 and 2005 exhibited above average temperatures during spring in the mixed layer, but again only in a single bioregion; 1998 was warmer in the north bioregion, while 2005 was warm in the south bioregion. Sub-mixed layer spring temperatures were consistently below average between 2013 and 2019 in the offshore region, and only slightly above the mean in the other two regions (Figure 2).
Across all bioregions, PCA consistently grouped years with warm events together which also tended to be years during positive phases of the PDO and ONI, and negative phases of the NPGO. Winter and spring PDO and ONI were often grouped with mixed layer temperature and sub-mixed layer temperature, wind-stress-curl and stratification. These variables were clustered with the warmer, positive PDO years: the 2014-2016 and 2019 marine heatwaves, the 1998 El Niño and the 2005 warm event all clustered negatively in PC1 for all regions (Figure 3). Negative values in PC1 therefore generally described years in positive PDO and ONI phases and with warmer conditions, fresher salinity, lower density, shallower mixed layer depths, and higher stratification. On the opposite (positive) end of PC1, winter and spring NPGO were grouped with mixed layer depth, mixed layer salinity, mixed layer density and wind magnitude. Positive NPGO phases, and negative PDO and ONI phases, loaded positively across PC1, with a deeper, more saline, and denser mixed layer, and higher wind magnitude. Positive values in PC1 tended to be La Niña years; this relationship was particularly clear in the south and offshore bioregions (Figures 3A, C, Table 2).
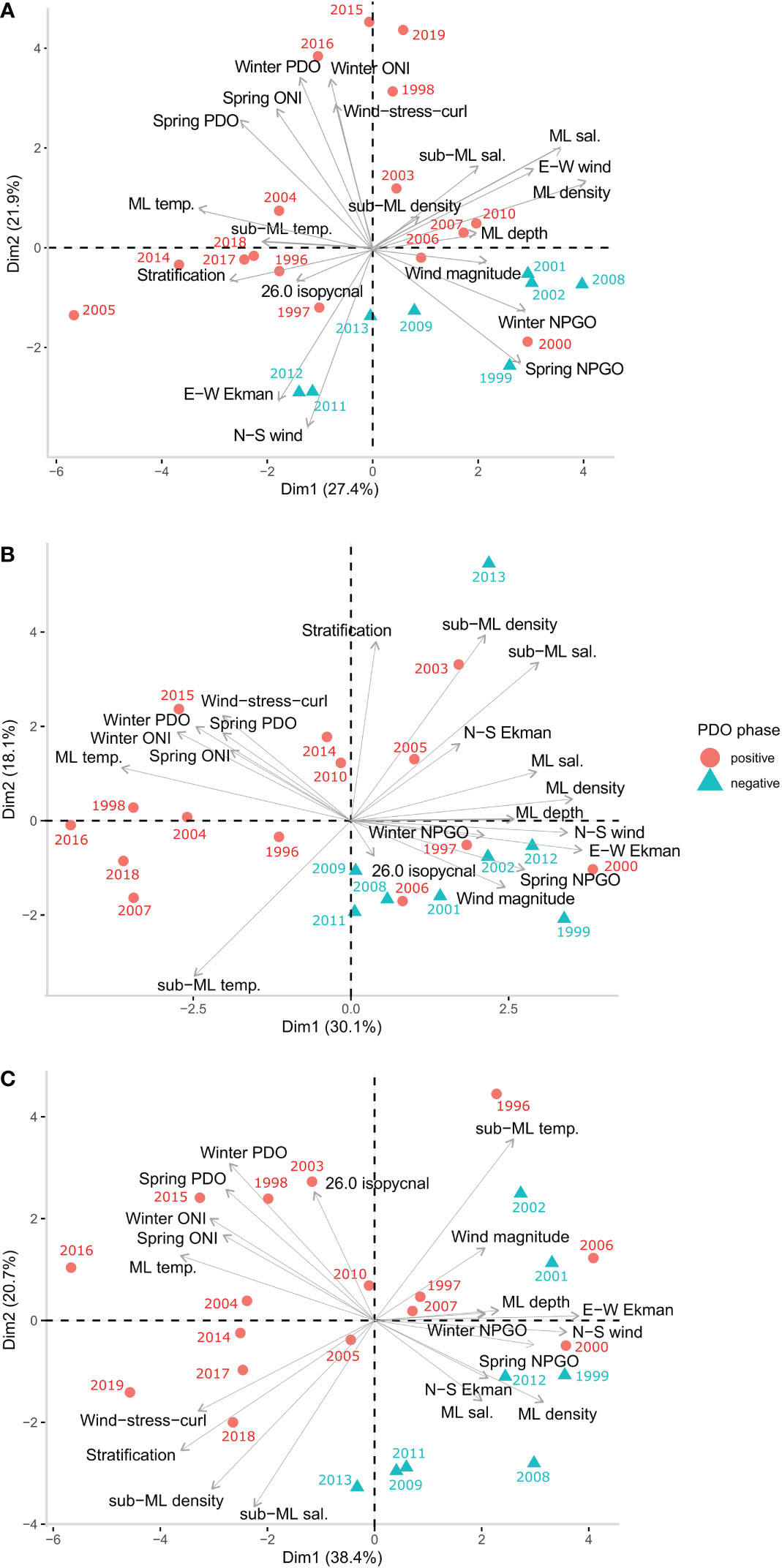
Figure 3 Ordination biplots of PC1 and PC2 for each bioregion, (A) South bioregion, (B) North bioregion and (C) Offshore bioregion. Oceanographic and physical covariates are overlaid as (grey) arrows on top of years (annual means) which are coloured according to PDO phase during April, May and June. ML, mixed layer; temp., temperature °C; sal., salinity; N-S, north-south; E-W, east-west; PDO, pacific decadal oscillation; ONI, Ocean Niño index; NPGO, north pacific gyre oscillation.
In the south bioregion E-W Ekman and N-S wind loaded negatively in PC1 (Figure 3A). Warm years in positive PDO and ONI which loaded negatively in PC1 were therefore characterised mainly by stronger onshore Ekman current and winds from the south. This suggests that generally stronger offshore Ekman transport occurred in negative phases of the PDO and ONI, and positive phases of the NPGO (positive end of PC1). However, in the north and offshore regions this pattern was reversed, with E-W Ekman and N-S winds loading positively in PC1, and therefore stronger onshore flow occurring during negative phases on the PDO and ONI.
3.2. Interannual and spatial variability in euphausiid biomass
Peaks for both species and total euphausiids tended to coincide with warm periods, or just preceding them in the south bioregion (see Figure 4). The highest annual mean anomalies for all regions and species were associated with the MHW but occurred first for T. spinifera in the south bioregion in 2013, for E. pacifica in the south in 2014, for all species in the north bioregion in 2015, and for E. pacifica in the offshore region in 2016. The peak in 2005 of T. spinifera biomass may be misleading as only one station exhibited a high biomass value (~3700 compared with 50-700 m2 nmi-2 at all other stations sampled), therefore both this peak and the peak in total euphausiids in this year should be treated with caution. The biomass of all euphausiid groups decreased into 2018 and 2019 across all bioregions (Figure 4).
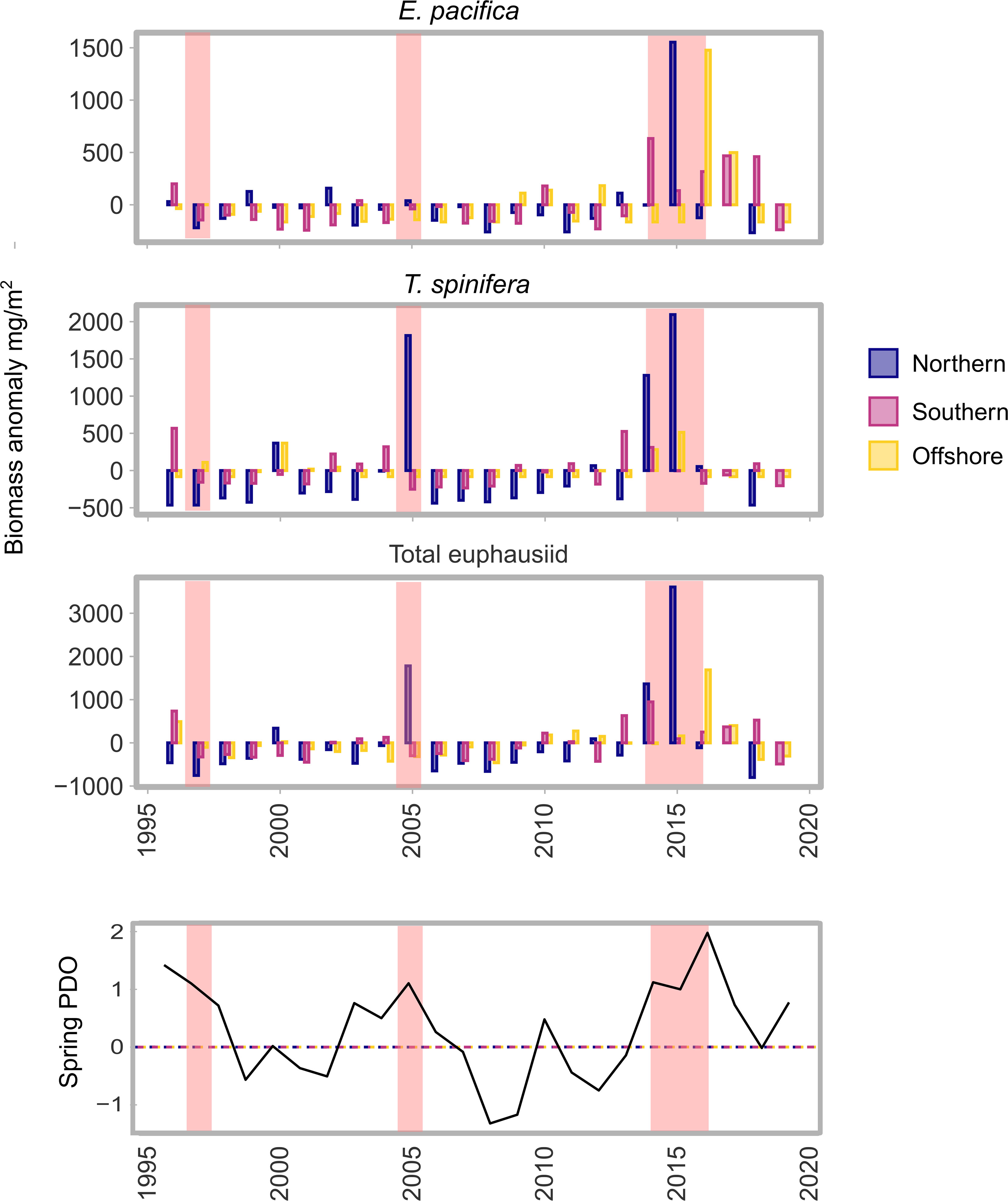
Figure 4 Annual mean anomaly (across stations) of sampled euphausiid biomass for each bioregion. Anomalies were calculated based on the time-series analysed: 1996-2019. Spring PDO shows the PDO phase during April, May and June of each year. Red highlighted regions represent ‘warm’ periods that are mentioned in the text; the 1997 El Niño, the 2005 ‘warm’ event, and the 2014-2016 MHW event.
3.3. Spatiotemporal relationships between environmental conditions and euphausiid biomass
The final model structure for E. pacifica, T. spinifera and total euphausiid biomass was: s(mag. surface wind, k = 3) + s(sub-mixed layer salinity, k = 3) + s(EW ekman, k = 3) + s(mixed layer temperature, mixed layer depth, k = 3) + s(spring PDO, k = 3). There were both similarities and differences in the estimated relationships between each euphausiid group and these environmental variables. For all three, sub-mixed layer salinity followed by spring PDO showed the strongest independent effects (Figure 5A). Both E. pacifica and total euphausiid biomass exhibited a strong positive relationship with increasing salinity. For T. spinifera this relationship was quadratic, and salinities greater than 33.5 had a negative effect on biomass, indicating a different response to high salinity by E. pacifica and T. spinifera. Relationships for spring PDO (with no time lag) were strongly positive for all species, indicating that euphausiid biomass was generally higher in the study region in PDO positive periods. Interestingly, all euphausiid groups showed a weak relationship with both magnitude of surface winds and Ekman current (Figure 5A).
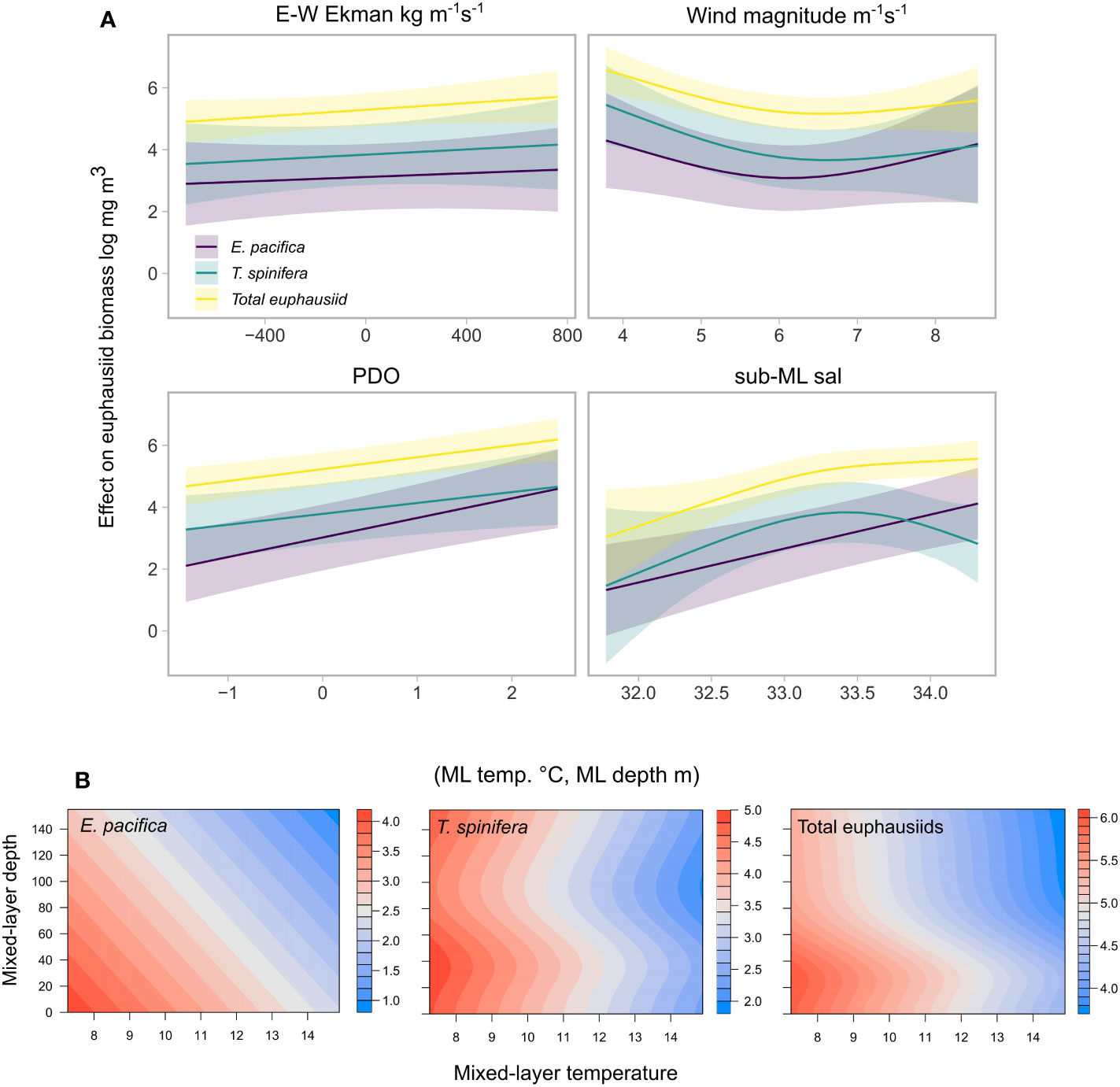
Figure 5 Conditional effects plots for each variable used in spatiotemporal modelling of E. pacifica, T. spinifera, and total euphausiids, with all other variables held at their median. (A). X-axis represented the range of each predictor represented in the model; Y-axis represents the effect on the biomass of each euphausiid group on the link scale (log-link), shaded sections represent +/- 95% confidence interval above and below the estimate of the smooth. (B). The conditional effects of the smooth interaction of mixed-layer temperature and mixed layer depth for euphausiid biomass for each euphausiid model.
The effect of warmer temperatures on euphausiid biomass varied with mixed layer depth to a differing degree for each species (Figure 5B). The highest E. pacifica biomass was present in areas with cooler temperatures and with shallower mixed layer depths, and the magnitude of the effect was similar across the values of the variables. For T. spinifera, higher temperatures still resulted in lower biomass, but this effect was strongest at intermediate and the very shallowest of mixed layer depths: highest biomass occurred at the coolest temperatures and when the mixed layer depth was between 25 and 50m. For total euphausiids, biomass dropped off steeply when the mixed layer depth dropped below 40m, which might reflect a combination of the two relationships for the other species.
Observations vs. predictions for each covariate model are shown in Figure S2. Estimated relative biomass peaked for both total euphausiids and E. pacifica during the MHW years and PDO positive period between 2012 and 2019 in the south bioregion, and to a certain extent in the offshore bioregion (Figure 6A). Estimated biomass showed a peak in 2016 for the whole area for both total euphausiids and E. pacifica, however the largest peak in biomass was predicted to occur during the 1997 El Niño event. Estimated biomass of all groups fell during the 1998 La Nina event following a change of PDO sign from positive to negative (Figure 2) and remained low until the warm event of 2005. In the north region, T. spinifera biomass was also high between 2012 and 2015, although it was comparatively lower than E. pacifica across the rest of the timeseries. The predicted spatial distribution of biomass showed that E. pacifica was more abundant further from the coast than T. spinifera, which was distributed consistently across the shelf (Figure 6B). Both species exhibited peak biomass within the Juan de Fuca canyon system off the southwest corner of the U.S.A, and off the northwest tip of Vancouver Island (see Figure 1 for the location of the canyon).
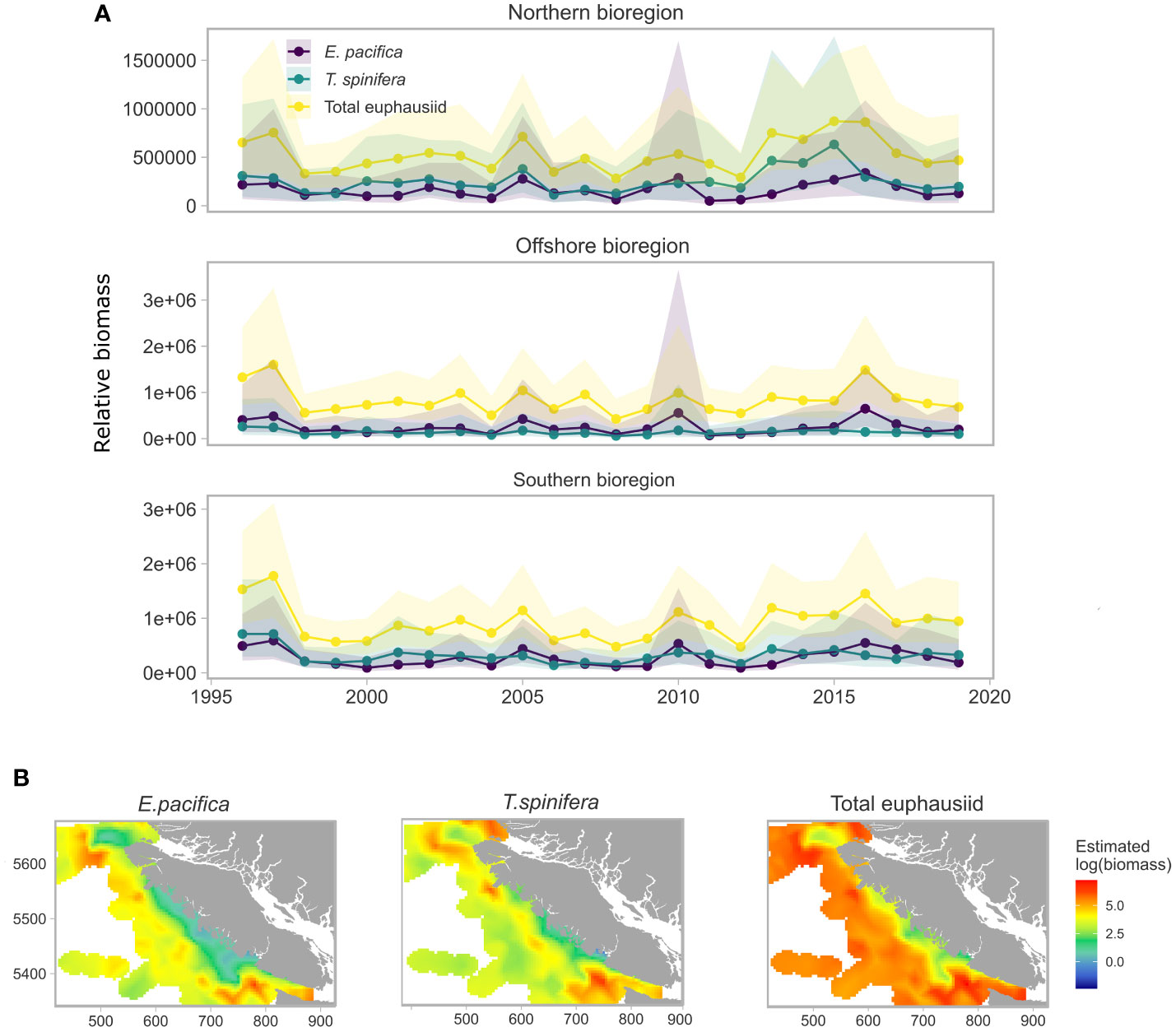
Figure 6 Spatial and temporal means estimated from euphausiid models and predicted on 5km2 covariate grid. (A) Relative mean predicted biomass of E. pacifica, T. spinifera and total euphausiid for each bioregion for each year, (B). Spatial distribution of mean predicted biomass for each of the three euphausiid groups.
4. Discussion
The oceanographic environment off the west coast of Vancouver Island exhibited some different spatiotemporal patterns to the southern portion of the California Current. We found that ‘warm’ events had variable local effects that differed across even small spatial scales. For example, the MHW years (2015-16) exhibited different effects in the offshore compared with both inshore bioregions, where observable temperature increases in the mixed layer were minimal. There were also similarities across bioregions, and we found clear signals of PDO, ONI, and NPGO cycling both in the local oceanographic environment and in the effects on euphausiid biomass and distribution, with PDO showing a clear and positive relationship with biomass for all euphausiid groups modelled. It was expected that the MHW would negatively affect euphausiid biomass on the coast. However, observed E. pacifica biomass, and to a lesser extent the two other euphausiid groups analysed, exhibited peak biomass levels during 2015. Overall higher biomass of euphausiids during perturbation events such as MHWs and through PDO positive periods could have a positive effect on ecosystem functioning during these events as euphausiids would provide a reliable food source for predators when the biomass of other forage species might be reduced.
4.1. Species-specific euphausiid biomass links with environmental and climate variability
Euphausiids in the California Current have been found to respond in conflicting ways to increased upwelling (Mackas et al., 2004; Francis et al., 2012; Kalyn, 2014; Benoit‐Bird et al., 2019; Rockwood et al., 2020). Proximity to upwelling centres can have the negative effect of transporting euphausiids offshore and away from productivity at the coast (Santora et al., 2011; []Santora et al., 2012). For high biomass of euphausiids to develop, a balance is required between being close enough to upwelling that production is enhanced, but being within an adjacent area of retention created by complex bathymetry or currents so that individuals are retained in production centers (Santora et al., 2018). We did not find a strong relationship with the upwelling metrics we used in our modelling, however the biomass of euphausiids did decrease with increased surface wind. The magnitude of surface wind will in part reflect upwelling as stronger meridional wind at the surface increases Coriolis force, therefore stimulating upwelling. Higher wind at the surface would likely affect the distribution of surface productivity, therefore this decrease in euphausiid biomass could be a result of a local reduction in food, or a direct redistribution of euphausiids at night when they are clustered at the surface. Cooler temperatures at the surface also indicates upwelling and may therefore also be a proxy for increased primary productivity. The relationship between mixed layer depth, temperature and euphausiid biomass is likely to reflect this process. We found the combined effect of mixed layer depth and temperature had a significant effect on all euphausiids, although the relationship differed between species. For E. pacifica and overall euphausiid biomass, biomass decreased in areas with a deeper mixed layer depth. During the 1997 El Niño event in the California Current, a decrease in euphausiid abundance coincided with a rapid increase in both temperature and mixed-layer depth (Marinovic et al., 2002), hypothesised to be due to very low primary productivity as a result of the El Niño event.
The biomass of E. pacifica, T. spinifera and total euphausiids was highly correlated with positive phases of the PDO, and therefore overall warmer temperatures in the mixed layer. There is some evidence that euphausiid biomass at higher latitudes may respond positively to warm events, while further south in the California Current the effects of warm events seem to be negative. For example, Tanasichuk (1998) described an increase in the biomass of E. pacifica during the 1992-93 El Niño off the west coast of Vancouver Island. Biomass anomalies of euphausiids off southern Vancouver Island were also positive during the warm PDO regime of the 1990s, only reducing after the El Niño/La Nina events of 1998-1999 (Mackas et al., 2001). Off California, Cimino et al. (2020) found that warm El Niño conditions resulted in lower than average euphausiid abundance, of both species; results which are mirrored across multiple other studies (Marinovic et al., 2002; Peterson et al., 2002; Brinton and Townsend, 2003). Average temperatures off the west coast of Vancouver Island are cool year-round (10.5°C for April, May, June across 23 years of data used for this analysis). The optimum recorded temperature for growth efficiency of E. pacifica is 11.4°C (Iguchi and Ikeda, 1995). Warmer temperatures at higher latitudes likely positively influences the metabolic rates of these euphausiids, compared with the southern California Current where they are living closer to their thermal maximum.
Despite euphausiid populations exhibiting some positive responses to past warm events at higher latitudes, responses to the 2014-2016 MHW in the northeast Pacific were generally negative across all larger zooplankton (Cavole et al., 2016; McKinstry et al., 2022). Therefore, the observed peak in E. pacifica biomass during the MHW years (2014-2016) off the west coast of Vancouver Island (48-51°N) was distinctive from responses of the same species of euphausiid further south. In fact, the abundance of euphausiids decreased everywhere south of 48°N in 2014 and 2015 (Leising et al., 2015), including off Oregon (Peterson et al., 2017), and the southern California Current (Lavaniegos et al., 2019; Cimino et al., 2020). Despite only representing a small spatial region, our dataset spans 23 years, and therefore ‘normal’ decadal fluctuations in biomass should be well represented; the peak in E. pacifica biomass was significantly larger than other variability in the dataset. Although to a lesser extent than E. pacifica, the peak T. spinifera biomass of the time-series also occurred during the MHW event. In the California Current, T. spinifera often becomes less abundant during El Niño warm events, and reproduction is often associated with periods of cold ocean temperatures as far north as Oregon (Brinton, 1981; Feinberg et al., 2010). The increase in biomass during the MHW was therefore particularly surprising for this species.
We propose three hypotheses that may explain the peak in euphausiid biomass observed during the MHW. First, in terms of the local environment, the shelf and slope seemed to be less affected by the event compared with the southern California Current. For example, Ekman transport was negative (offshore) in the spring of 2015 indicating upwelling activity. This was recorded elsewhere in the northern California Current, while off California negative (offshore) Ekman transport was weaker than average (Leising et al., 2015). Temperatures in the mixed-layer off Vancouver Island in 2015 were just above average in the north and south bioregion, only rising 1-2°C above the dataset average in the offshore region (see Figure 2). The negative Ekman transport leading to upwelling of cooler water onto the shelf likely helped maintain temperatures in the mixed layer just above average (~12°C) off the west coast of Vancouver Island. Marinovic et al. (2002), recorded negative effects of the 1998 El Niño on E. pacifica biomass in Monterey Bay, however, SSTs reached ~17°C there, 5°C warmer than the peak temperature recorded in our study during the MHW.
Second, unprecedented northward shifts in southern biota, including euphausiids, occurred between 2014 and 2015 as a result of a series of anomalous poleward flows of the California Undercurrent during the second half of 2014, that could account for ~300-500km of displacement (Friedman et al., 2018; Sanford et al., 2019). Decreased biomass of E. pacifica and T. spinifera reported in more southern areas of the California Current might partly be due to northward (poleward) displacement of these species. This hypothesis is somewhat supported by the large peaks in euphausiid biomass found in the north bioregion at the transition zone with the Alaska Current, which might be an area of retention during periods of strong poleward-flow of the California Undercurrent.
Third, the MHW correlated with reduced biomass of large, sub-arctic species of copepods along the west coast of Vancouver Island, leading to a shift in zooplankton community composition towards a small, subtropical copepod community (Perry et al., 2019). Euphausiids and sub-arctic copepod species such as Neocalanus spp. typically exhibit a mirror-image fluctuation in biomass in this region (Mackas et al., 2001), possibly due to competition for the same resources, as both are omnivorous and proportionally high diatom-grazers (Ohman, 1984; Bargu et al., 2003; El-Sabaawi et al., 2009). The negative effect of the MHW on large copepods in this region may have reduced competition during a period when diatom abundance was fairly low in the nearshore (Peña et al., 2019).
It is interesting to note that the entire zooplankton community off the west coast of Vancouver Island exhibited an anomalous peak in biomass during the MHW years (Perry et al., 2019). This response is seemly distinct from surrounding systems; in the California Current south of Vancouver Island and in the Gulf of Alaska to the north, planktivorous birds such as common murres (Uria aalge) (Piatt et al., 2020) and Cassin’s auklet (Jones et al., 2018) exhibited massive mortality events in response to the MHW. This was linked to a decrease in the lipid content, and therefore nutritional value, of available mesozooplankton (Cass et al., 2020), which was also cited as the reason for declines in fish such as Walleye Pollock in Alaska (Rogers et al., 2021). These results seem to suggest negative effects of the MHW on plankton communities across trophic levels. However, looking in more detail at the results of Arimitsu et al. (2021), the declines in euphausiid biomass reported over multiple areas of Alaska were not for E. pacifica and T. spinifera, but rather for cold water species such as T. inermis and T. longipes. In fact, although absolute values were not reported in this study, it appears as though the biomass of E. pacifica may have increased in many areas in the MHW years, compared with the few years before the event. Off California reductions in the size distribution of E. pacifica were also described during this event (Robertson and Bjorkstedt, 2020), which is an important metric of lower-trophic level quality for predators that is currently lacking for the Canadian northeast Pacific. The effects of this event on euphausiids are complicated and seem to vary across latitude and across species, which display very localised responses.
4.2. Implications for local predator populations
Variability in the biomass and distribution of euphausiids has flow-on effects to local predators, particularly central-place foragers such as seabirds. For example, in 2005 reduced euphausiid biomass off California was linked to reproductive failure of a euphausiid predator, Cassin’s Auklet (Sydeman et al., 2006). 2005 was a very unusual year for the California Current climate, with warming occurring only north of 35 N, but with a similar extent and ecosystem response to that of a major El Niño event (Peterson et al., 2006).
The 2015-16 MHW event was correlated with changes in the distribution of numerous pelagic fishes which meant that predation pressure for euphausiids was very different from other years studied. Age-0 hake were found off Washington and Oregon, meaning that spawning centers shifted northwards during the event (Morgan et al., 2019), and age-1 hake were observed in Canadian waters as far as the southwest coast of Vancouver Island (48.8°N), which is unusual (Gauthier et al., 2016). In addition, in the California Current the Northern Anchovy (Engraulis mordax) and Pacific Sardine (Sardinops sagax) biomass cycles are usually highly predictable, with sardine abundant during warm periods such as positive PDO regimes, and anchovy abundant during cool periods. However, the biomass of sardines was unusually low in the California Current in the years prior to 2014 (Lindegren and Checkley, 2013; Thompson et al., 2019; Ren and Rudnick, 2021). During the MHW the predictability of this stock broke down completely, with low biomass and a change in migration patterns with less of the north-wards migrating stock making it to Canadian waters during 2015-2018 (Muhling et al., 2020). In addition, although biomass estimates for herring during this period are not available, the biomass of herring usually decreases in warm years (Robinson and Ware, 1994; McFarlane et al., 1997), which may be due to increased predation by a larger proportion of the Hake stock in Canadian waters during warm years. Numbers of herring in the Gulf of Alaska were very low during the MHW (Arimitsu et al., 2021b). Euphausiids are the main prey of Pacific herring, sardines, anchovies and hake (McFarlane et al., 2010), therefore changes in their distribution during the event would have vastly changed the predator landscape for euphausiids off the west coast of Vancouver Island compared to other years, and might help explain the high biomass of euphausiids observed during spring 2015.
The waters around Vancouver Island have seen increases in the abundance of baleen whales such as Humpback whales (Megaptera novaeangliae) between spring and fall in recent years (Nichol et al., 2017; McMillan et al., 2019), which also consume euphausiids (Dalla Rosa et al., 2012). Sightings of Humpback whales were particularly high in the Salish sea during 2014 and 2015 (Calambokidis et al., 2018). The effect of this new foraging pressure on euphausiid populations, and implications for other euphausiid predators, has not yet been quantified. Large marine predators have been documented to shift their migratory patterns during warm events such as marine heatwaves, therefore changing their impact on forage species during these events (Carroll et al., 2021; Hammerschlag et al., 2022). These food web interactions are complex, and our findings support the need for greater understanding of how fluctuations in the biomass of key food web species such as euphausiids propagate upwards to higher trophic levels from a whole ecosystem perspective. Understanding these interactions will help the predictability of stocks for commercially important species, particularly during anomalous climate conditions.
5. Conclusion
The effects of acute climate events such as MHWs on local oceanography and the response of coastal ecosystems appears to be highly spatially variable. While euphausiids in the California Current (Lavaniegos et al., 2019) and Alaska (Rogers et al., 2021) were negatively impacted in some way, our models indicate that for the area of the shelf sampled during the MHW, spring euphausiid biomass was high off the west coast of Vancouver Island. There are also indications that adjacent nearshore coastal ecosystems and local fish assemblages are minimally affected by MHW events (Robinson et al., 2022), while other coastal areas are extremely affected (Arias-Ortiz et al., 2018; Rogers-Bennett and Catton, 2019), and the response of biological communities can vary widely across trophic levels and between populations. Characterising long-term lower-trophic level variability in response to climate, and the implications for the food-webs they support, will help to better predict the effects of these MHW events.
Data availability statement
The datasets presented in this study can be found in online repositories. The names of the repository/repositories and accession number(s) can be found below: https://open.canada.ca/data/en/dataset/9447ecf8-a7f7-4904-8ab0-3c597c534c4b.
Author contributions
RE conceived of the idea, carried out the analysis and wrote the manuscript. PE contributed to the analysis and manuscript drafts. CR and SG conceived of the idea and contributed to manuscript drafts. All authors contributed to the article and approved the submitted version.
Acknowledgments
We would like to acknowledge personnel from Fisheries and Oceans Canada and the Canadian Coast Guard who collected both the euphausiid and CTD data from the west coast of Vancouver Island through multiple sampling programs, aboard many ships including the CCGS John P. Tully and the CCGS W.E. Ricker. We especially thank Moira Galbraith and David L. Mackas who have collected much of this data, processed and identified the samples, maintained the database, and published much of the previous work in this region. Finally, we thank Ron Tanasichuk for his comments on an earlier version of this work. Two external reviewers have greatly helped improve this study. This work was funded by Fisheries and Oceans Canada (DFO) Aquatic Climate Change Adaptation Services Program (ACCASP).
Conflict of interest
The authors declare that the research was conducted in the absence of any commercial or financial relationships that could be construed as a potential conflict of interest.
Publisher’s note
All claims expressed in this article are solely those of the authors and do not necessarily represent those of their affiliated organizations, or those of the publisher, the editors and the reviewers. Any product that may be evaluated in this article, or claim that may be made by its manufacturer, is not guaranteed or endorsed by the publisher.
Supplementary material
The Supplementary Material for this article can be found online at: https://www.frontiersin.org/articles/10.3389/fmars.2023.1031485/full#supplementary-material
References
Abraham C. L., Sydeman W. J. (2004). Ocean climate, euphausiids and auklet nesting: inter-annual trends and variation in phenology, diet and growth of a planktivorous seabird, ptychoramphus aleuticus. Mar. Ecol. Prog. Ser. 274, 235–250. doi: 10.3354/meps274235
Abraham C. L., Sydeman W. J. (2006). Prey-switching by cassin’s auklet ptychoramphus aleuticus reveals seasonal climate-related cycles of euphausia pacifica and thysanoessa spinifera. Mar. Ecol. Prog. Ser. 313, 271–283. doi: 10.3354/meps313271
Allen S. E., Vindeirinho C., Thomson R. E., Foreman M. G., Mackas D. L. (2001). Physical and biological processes over a submarine canyon during an upwelling event. Can. J. Fish. Aquat. Sci. 58, 671–684. doi: 10.1139/f01-008
Amaya D. J., Miller A. J., Xie S.-P., Kosaka Y. (2020). Physical drivers of the summer 2019 north pacific marine heatwave. Nat. Commun. 11, 1–9. doi: 10.1038/s41467-020-15820-w
Anderson S. C., Ward E. J., Barnett L. A. K., English P. A. (2020) sdmTMB: Spatiotemporal species distribution GLMMs with “TMB. Available at: https://pbs-assess.github.io/sdmTMB/index.html.
Arias-Ortiz A., Serrano O., Masqué P., Lavery P. S., Mueller U., Kendrick G. A., et al. (2018). A marine heatwave drives massive losses from the world’s largest seagrass carbon stocks. Nat. Clim Change 8, 338–344. doi: 10.1038/s41558-018-0096-y
Arimitsu M. L., Piatt J. F., Hatch S., Suryan R. M., Batten S., Bishop M. A., et al. (2021). Heatwave-induced synchrony within forage fish portfolio disrupts energy flow to top pelagic predators. Global Change Biol. 27(9), 1859–1878. doi: 10.1111/gcb.15556
Bargu S., Marinovic B., Mansergh S., Silver M. W. (2003). Feeding responses of krill to the toxin-producing diatom pseudo-nitzschia. J. Exp. Mar. Biol. Ecol. 284, 87–104. doi: 10.1016/S0022-0981(02)00494-X
Beamish R. J., Sweeting R. M., Neville C. M. (2004). Improvement of juvenile pacific salmon production in a regional ecosystem after the 1998 climatic regime shift. Trans. Am. Fisheries Soc. 133, 1163–1175. doi: 10.1577/T03-170.1
Benoit-Bird K. J., Waluk C. M., Ryan J. P. (2019). Forage species swarm in response to coastal upwelling. Geophysical Res. Lett. 46, 1537–1546. doi: 10.1029/2018GL081603
Black B. A., Schroeder I. D., Sydeman W. J., Bograd S. J., Wells B. K., Schwing F. B. (2011). Winter and summer upwelling modes and their biological importance in the California current ecosystem: WINTER AND SUMMER UPWELLING MODES. Global Change Biol. 17, 2536–2545. doi: 10.1111/j.1365-2486.2011.02422.x
Brinton E. (1960). Changes in the distribution of euphausiid crustaceans in the region of the California current. Calif. Coop. Oceanic Fish. Invest. Rep. 7, 137–146.
Brinton E. (1976). Population biology of euphausia pacifica off southern California. Fishery Bull. 74, 733–762.
Brinton E. (1981). Euphausiid distributions in the California current during the warm winter-spring of 1977–78, in the context of a 1949–1966 time series. California Cooperative Oceanic Fisheries Investigations Rep. 22, 135–154.
Brinton E., Townsend A. (2003). Decadal variability in abundances of the dominant euphausiid species in southern sectors of the California current. Deep Sea Res. Part II: Topical Stud. Oceanography 50, 2449–2472. doi: 10.1016/S0967-0645(03)00126-7
Brodeur R. D. (1986). Northward displacement of the euphausiid nyctiphanes simplex Hansen to Oregon and Washington waters following the El NiÑo event of 1982―83. J. Crustacean Biol. 6, 686–692. doi: 10.2307/1548382
Brodeur R. D., Auth T. D., Phillips A. J. (2019). Major shifts in pelagic micronekton and macrozooplankton community structure in an upwelling ecosystem related to an unprecedented marine heatwave. Front. Mar. Sci. 6, 212. doi: 10.3389/fmars.2019.00212
Calambokidis J., Flynn K., Dobson E., Huggins J. L., Perez A. (2018). Return of the giants of the salish Sea: Increased occurrence of humpback and gray whales in inland waters, Vol. 7.
Carroll G., Brodie S., Whitlock R., Ganong J., Bograd S. J., Hazen E., et al. (2021). Flexible use of a dynamic energy landscape buffers a marine predator against extreme climate variability. Proc. R. Soc. B 288, 20210671. doi: 10.1098/rspb.2021.0671
Cass C., Bjorkstedt E., Robertson R. (2020) Impacts of marine heatwaves on euphausia pacifica in the northern California current. Available at: https://agu.confex.com/agu/osm20/meetingapp.cgi/Paper/648154 (Accessed March 22, 2021).
Cavole L. M., Demko A. M., Diner R. E., Giddings A., Koester I., Pagniello C. M., et al. (2016). Biological impacts of the 2013–2015 warm-water anomaly in the northeast pacific: Winners, losers, and the future. Oceanography 29, 273–285. doi: 10.5670/oceanog.2016.32
Chenillat F., Rivière P., Capet X., Di Lorenzo E., Blanke B. (2012). North pacific gyre oscillation modulates seasonal timing and ecosystem functioning in the California current upwelling system. Geophysical Res. Lett. 39(L01606), 1–6. doi: 10.1029/2012GL053111
Cheung W. W., Frölicher T. L. (2020). Marine heatwaves exacerbate climate change impacts for fisheries in the northeast pacific. Sci. Rep. 10, 1–10. doi: 10.1038/s41598-020-63650-z
Cimino M. A., Santora J. A., Schroeder I., Sydeman W., Jacox M. G., Hazen E. L., et al. (2020). Essential krill species habitat resolved by seasonal upwelling and ocean circulation models within the large marine ecosystem of the California current system. Ecography. 43(10), 1536–49. doi: 10.1111/ecog.05204
Dalla Rosa L., Ford J. K., Trites A. W. (2012). Distribution and relative abundance of humpback whales in relation to environmental variables in coastal British Columbia and adjacent waters. Continental Shelf Res. 36, 89–104. doi: 10.1016/j.csr.2012.01.017
Demer D. A., Zwolinski J. P., Byers K. A., Cutter G. R., Renfree J. S., Sessions T. S., et al. (2012). Prediction and confirmation of seasonal migration of pacific sardine (Sardinops sagax) in the California current ecosystem.
Di Lorenzo E., Mantua N. (2016). Multi-year persistence of the 2014/15 north pacific marine heatwave. Nat. Climate Change 6, 1042–1047. doi: 10.1038/nclimate3082
Di Lorenzo E., Schneider N., Cobb K. M., Franks P. J. S., Chhak K., Miller A. J., et al. (2008). North pacific gyre oscillation links ocean climate and ecosystem change. Geophysical Res. Lett. 35, L08607. doi: 10.1029/2007GL032838
Dunn P. K., Smyth G. K. (2005). Series evaluation of tweedie exponential dispersion model densities. Stat Computing 15, 267–280. doi: 10.1007/s11222-005-4070-y
El-Sabaawi R., Dower J., Kainz M., Mazumder A. (2009). Interannual variability in fatty acid composition of the copepod neocalanus plumchrus in the strait of Georgia, British Columbia. Mar. Ecol. Prog. Ser. 382, 151–161. doi: 10.3354/meps07915
Feinberg L. R., Peterson W. T., Shaw C. T. (2010). The timing and location of spawning for the euphausiid thysanoessa spinifera off the Oregon coast, USA. Deep Sea Res. Part II: Topical Stud. Oceanography 57, 572–583. doi: 10.1016/j.dsr2.2009.10.007
Fiechter J., Santora J. A., Chavez F., Northcott D., Messié M. (2020). Krill hotspot formation and phenology in the California current ecosystem. Geophysical Res. Lett. 47, e2020GL088039. doi: 10.1029/2020GL088039
Francis T. B., Scheuerell M. D., Brodeur R. D., Levin P. S., Ruzicka J. J., Tolimieri N., et al. (2012). Climate shifts the interaction web of a marine plankton community. Global Change Biol. 18, 2498–2508. doi: 10.1111/j.1365-2486.2012.02702.x
Friedman W. R., Santora J. A., Schroeder I. D., Huff D. D., Brodeur R. D., Field J. C., et al. (2018). Environmental and geographic relationships among salmon forage assemblages along the continental shelf of the California current. Mar. Ecol. Prog. Ser. 596, 181–198. doi: 10.3354/meps12598
Gauthier S., Stanley C., Nephin J. (2016). State of the physical, biological and selected fishery resources of pacific Canadian marine ecosystems in 2015. Can. Tech. Rep. Fish. Aquat. Sci. 3179, 230. Distribution and abundance of Pacific Hake (Merluccius productus). In Chandler, P.C., King, S.A., and Perry, R.I. (Eds.). 2016.
Gladics A., Suryan R., Brodeur R., Segui L., Filliger L. (2014). Constancy and change in marine predator diets across a shift in oceanographic conditions in the northern California current. Mar. Biol. 1, 837–851. doi: 10.1007/s00227-013-2384-4
Hammerschlag N., McDonnell L. H., Rider M. J., Street G. M., Hazen E. L., Natanson L. J., et al. (2022). Ocean warming alters the distributional range, migratory timing, and spatial protections of an apex predator, the tiger shark (Galeocerdo cuvier). Global Change Biol. 28, 1990–2005. doi: 10.1111/gcb.16045
Hipfner J. M. (2008). Matches and mismatches: Ocean climate, prey phenology and breeding success in a zooplanktivorous seabird. Mar. Ecol. Prog. Ser. 368, 295–304. doi: 10.3354/meps07603
Hipfner J. (2009). Euphausiids in the diet of a north pacific seabird: Annual and seasonal variation and the role of ocean climate. Mar. Ecol. Prog. Ser. 390, 277–289. doi: 10.3354/meps08209
Hipfner J. M., Galbraith M., Bertram D. F., Green D. J. (2020). Basin-scale oceanographic processes, zooplankton community structure, and diet and reproduction of a sentinel north pacific seabird over a 22-year period. Prog. Oceanography 182, 102290. doi: 10.1016/j.pocean.2020.102290
Iguchi N., Ikeda T. (1995). Growth, metabolism and growth efficiency of a euphausiid crustacean euphausia pacifica in the southern Japan Sea, as influenced by temperature. J. Plankton Res. 17, 1757–1769. doi: 10.1093/plankt/17.9.1757
Jones T., Parrish J. K., Peterson W. T., Bjorkstedt E. P., Bond N. A., Ballance L. T., et al. (2018). Massive mortality of a planktivorous seabird in response to a marine heatwave. Geophysical Res. Lett. 45, 3193–3202. doi: 10.1002/2017GL076164
Kalyn S. L. (2014). The impact of ocean climate variability on prey availability and the reproductive performance of a planktivorous seabird, ancient murrelet (Synthliboramphus antiquus) (Canada: Royal Roads University).
Kelley D., Richards C. (2021) Oce: Analysis of oceanographic data. Available at: https://CRAN.R-project.org/package=oce.
Lavaniegos B. E., Jiménez-Herrera M., Ambriz-Arreola I. (2019). Unusually low euphausiid biomass during the warm years of 2014–2016 in the transition zone of the California current. Deep Sea Res. Part II: Topical Stud. Oceanography 169–170, 104638. doi: 10.1016/j.dsr2.2019.104638
Leising A. W., Schroeder I. D., Bograd S. J., Abell J., Durazo R., Gaxiola-Castro G., et al. (2015). State of the California current 2014-15: Impacts of the warm-water" blob". California Cooperative Oceanic Fisheries Investigations Rep. 56, 31–68.
Lindegren M., Checkley D. M. Jr (2013). Temperature dependence of pacific sardine (Sardinops sagax) recruitment in the California current ecosystem revisited and revised. Can. J. Fisheries Aquat. Sci. 70, 245–252. doi: 10.1139/cjfas-2012-0211
Lindgren F., Rue H. (2015). Bayesian Spatial modelling with r-INLA. J. Stat. Software 63, 1–25. doi: 10.18637/jss.v063.i19
Lindgren F., Rue H., Lindström J. (2011). An explicit link between Gaussian fields and Gaussian Markov random fields: The stochastic partial differential equation approach. J. R. Stat. Society: Ser. B (Statistical Methodology) 73, 423–498. doi: 10.1111/j.1467-9868.2011.00777.x
Lu B., Mackas D. L., Moore D. F. (2003). Cross-shore separation of adult and juvenile euphausiids in a shelf-break alongshore current. Prog. Oceanography 57, 381–404. doi: 10.1016/S0079-6611(03)00107-1
Mackas D. L., Galbraith M. (2002). Zooplankton community composition along the inner portion of line p during the 1997–1998 El niño event. Prog. Oceanography 54, 423–437. doi: 10.1016/S0079-6611(02)00062-9
Mackas D., Galbraith M., Faust D., Masson D., Young K., Shaw W., et al. (2013). Zooplankton time series from the strait of Georgia: Results from year-round sampling at deep water locations 1990–2010. Prog. Oceanography 115, 129–159. doi: 10.1016/j.pocean.2013.05.019
Mackas D. L., Kieser R., Saunders M., Yelland D. R., Brown R. M., Moore D. F. (1997). Aggregation of euphausiids and pacific hake (Merluccius productus) along the outer continental shelf off Vancouver island. Can. J. Fish. Aquat. Sci. 54, 2080–2096. doi: 10.1139/f97-113
Mackas D. L., Peterson W. T., Zamon J. E. (2004). Comparisons of interannual biomass anomalies of zooplankton communities along the continental margins of British Columbia and Oregon. Deep Sea Res. Part II: Topical Stud. Oceanography 51, 875–896. doi: 10.1016/j.dsr2.2004.05.011
Mackas D. L., Thomson R. E., Galbraith M. (2001). Changes in the zooplankton community of the British Columbia continental margin 1985-1999, and their covariation with oceanographic conditions. Can. J. Fisheries Aquat. Sci. 58, 685–702. doi: 10.1139/f01-009
Malick M. J., Hunsicker M. E., Haltuch M. A., Parker-Stetter S. L., Berger A. M., Marshall K. N. (2020). Relationships between temperature and pacific hake distribution vary across latitude and life-history stage. Mar. Ecol. Prog. Ser. 639, 185–197. doi: 10.3354/meps13286
Mantua N. J., Hare S. R. (2002). The pacific decadal oscillation. J. oceanography 58, 35–44. doi: 10.1023/A:1015820616384
Marinovic B. B., Croll D. A., Gong N., Benson S. R., Chavez F. P. (2002). Effects of the 1997–1999 El niño and la niña events on zooplankton abundance and euphausiid community composition within the Monterey bay coastal upwelling system. Prog. Oceanography 54, 265–277. doi: 10.1016/S0079-6611(02)00053-8
McFarlane G., Schweigert J., Detering J., Hodes V. (2010). Diet analysis of pacific Sardine(Sardinops sagax) off the West coast of Vancouver island, British Columbia from 1997 to 2008. Rep. California Cooperative Oceanic Fisheries Investigations 51, 169–181.
McFarlane G. A., Ware D. M., Thomson R. E., Mackas D. L., Robinson C. L. K. (1997). Physical, biological and fisheries oceanography of a large ecosystem (west coast of Vancouver island) and implications for management. Oceanologica Acta 20, 191–200.
McGowan J. A. (1998). Climate-ocean variability and ecosystem response in the northeast pacific. Science 281, 210–217. doi: 10.1126/science.281.5374.210
McKinstry C. A., Campbell R. W., Holderied K. (2022). Influence of the 2014–2016 marine heatwave on seasonal zooplankton community structure and abundance in the lower cook inlet, Alaska. Deep Sea Res. Part II: Topical Stud. Oceanography 195, 105012. doi: 10.1016/j.dsr2.2021.105012
McMillan C. J., Towers J. R., Hildering J. (2019). The innovation and diffusion of “trap-feeding,” a novel humpback whale foraging strategy. Mar. Mammal Sci. 35, 779–796. doi: 10.1111/mms.12557
Morgan C. A., Beckman B. R., Weitkamp L. A., Fresh K. L. (2019). Recent ecosystem disturbance in the northern California current. Fisheries 44, 465–474. doi: 10.1002/fsh.10273
Muhling B. A., Brodie S., Smith J. A., Tommasi D., Gaitan C. F., Hazen E. L., et al. (2020). Predictability of species distributions deteriorates under novel environmental conditions in the California current system. Front. Mar. Sci. 7. doi: 10.3389/fmars.2020.00589
Nichol L. M., Wright B. M., Hara P. O., Ford J. K. (2017). Risk of lethal vessel strikes to humpback and fin whales off the west coast of Vancouver island, Canada. Endangered Species Res. 32, 373–390. doi: 10.3354/esr00813
Nickels C. F., Sala L. M., Ohman M. D. (2018). The morphology of euphausiid mandibles used to assess selective predation by blue whales in the southern sector of the California current system. J. Crustacean Biol. 38, 563–573. doi: 10.1093/jcbiol/ruy062
Nickels C. F., Sala L. M., Ohman M. D. (2019). The euphausiid prey field for blue whales around a steep bathymetric feature in the southern California current system. Limnology Oceanography 64, 390–405. doi: 10.1002/lno.11047
Ohman M. (1984). Omnivory by euphausia pacifica: The role of copepod prey. Mar. Ecol. Prog. Ser. 19, 125–131. doi: 10.3354/meps019125
Ohman M. D., Lavaniegos B. E. (2002). Comparative zooplankton sampling efficiency of a ring net and bongo net with comments on pooling of subsamples. California Cooperative Oceanic Fisheries Investigations Rep. 162–173.
Oksanen J., Blanchet F. G., Friendly M., Kindt R., Legendre P., McGlinn D., et al. (2020) Vegan: Community ecology package. Available at: https://CRAN.R-project.org/package=vegan.
Peña M. A., Nemcek N., Robert M. (2019). Phytoplankton responses to the 2014–2016 warming anomaly in the northeast subarctic pacific ocean. Limnology Oceanography 64, 515–525. doi: 10.1002/lno.11056
Perry R. I., Galbraith M., Young K., Sastri A. (2019). 50. zooplankton responses along the west coast of Vancouver island to the NE pacific marine heatwave. State Physical Biol. Selected Fishery Resour. Pacific Can. Mar. Ecosyst. 2019 218.
Peterson W. T., Emmett R., Goericke R., Venrick E., Mantyla A., Bograd S. J., et al. (2006). The state of the California current 2005-2006: warm in the north, cool in the south. California Cooperative Oceanic Fisheries Investigations Rep. 47, 30.
Peterson W. T., Fisher J. L., Strub P. T., Du X., Risien C., Peterson J., et al. (2017). The pelagic ecosystem in the northern California current off Oregon during the 2014–2016 warm anomalies within the context of the past 20 years. J. Geophysical Research: Oceans 122, 7267–7290. doi: 10.1002/2017JC012952
Peterson W. T., Keister J. E., Feinberg L. R. (2002). The effects of the 1997–99 El Niño/La niña events on hydrography and zooplankton off the central Oregon coast. Prog. Oceanography 54, 381–398. doi: 10.1016/S0079-6611(02)00059-9
Peterson W. T., Schwing F. B. (2003). A new climate regime in northeast pacific ecosystems. Geophysical Res. Lett. 30, 1896. doi: 10.1029/2003GL017528
Piatt J. F., Parrish J. K., Renner H. M., Schoen S. K., Jones T. T., Arimitsu M. L., et al. (2020). Extreme mortality and reproductive failure of common murres resulting from the northeast pacific marine heatwave of 2014-2016. PloS One 15, e0226087. doi: 10.1371/journal.pone.0226087
Qualls K. M. (2019). Drivers of euphausiid abundance and biomass in the kitimat fjord system (BC Canada: Oregon State University).
Ren A. S., Rudnick D. L. (2021). Temperature and salinity extremes from 2014-2019 in the California current system and its source waters. Commun. Earth Environ. 2, 1–9. doi: 10.1038/s43247-021-00131-9
Ressler P. H., Brodeur R. D., Peterson W. T., Pierce S. D., Vance P. M., Røstad A., et al. (2005). The spatial distribution of euphausiid aggregations in the northern California current during august 2000. Deep Sea Res. Part II: Topical Stud. Oceanography 52, 89–108. doi: 10.1016/j.dsr2.2004.09.032
Robertson R. R., Bjorkstedt E. P. (2020). Climate-driven variability in euphausia pacifica size distributions off northern California. Prog. Oceanography 188, 102412. doi: 10.1016/j.pocean.2020.102412
Robinson C. L. K. (2000). The consumption of euphausiids by the pelagic fish community off southwestern Vancouver island, British Columbia. J. Plankton Res. 22, 1649–1662. doi: 10.1093/plankt/22.9.1649
Robinson C. L., Ware D. M. (1994). Modelling pelagic fish and plankton trophodynamics off southwestern Vancouver island, British Columbia. Can. J. Fisheries Aquat. Sci. 51, 1737–1751. doi: 10.1139/f94-175
Robinson C. L. K., Yakimishyn J., Evans R. (2022)Minimal effects of the 2014-16 marine heatwave on fish assemblages found in eelgrass meadows on the southwestern coast of Vancouver island, British Columbia, Canada (Accessed August 23, 2022).
Rockwood R. C., Elliott M. L., Saenz B., Nur N., Jahncke J. (2020). Modeling predator and prey hotspots: Management implications of baleen whale co-occurrence with krill in central California. PloS One 15, e0235603. doi: 10.1371/journal.pone.0235603
Rogers-Bennett L., Catton C. A. (2019). Marine heat wave and multiple stressors tip bull kelp forest to sea urchin barrens. Sci. Rep. 9, 1–9. doi: 10.1038/s41598-019-51114-y
Rogers L. A., Wilson M. T., Duffy-Anderson J. T., Kimmel D. G., Lamb J. F. (2021). Pollock And “the blob”: Impacts of a marine heatwave on walleye pollock early life stages. Fish Oceanogr 30, 142–158. doi: 10.1111/fog.12508
Rue H., Martino S., Lindgren F., Simpson D., Riebler A., Krainski E. T. (2009). INLA: functions which allow to perform a full Bayesian analysis of structured additive models using integrated nested Laplace approximation. R Package version 0.0.
Sanford E., Sones J. L., García-Reyes M., Goddard J. H., Largier J. L. (2019). Widespread shifts in the coastal biota of northern California during the 2014–2016 marine heatwaves. Sci. Rep. 9, 1–14. doi: 10.1038/s41598-019-40784-3
Santora J., Hazen E., Schroeder I., Bograd S., Sakuma K., Field J. (2017). Impacts of ocean climate variability on biodiversity of pelagic forage species in an upwelling ecosystem. Mar. Ecol. Prog. Ser. 580, 205–220. doi: 10.3354/meps12278
Santora J. A., Sydeman W. J., Schroeder I. D., Reiss C. S., Wells B. K., Field J. C., et al. (2012). Krill space: A comparative assessment of mesoscale structuring in polar and temperate marine ecosystems. ICES J. Mar. Sci. 69, 1317–1327. doi: 10.1093/icesjms/fss048
Santora J. A., Sydeman W. J., Schroeder I. D., Wells B. K., Field J. C. (2011). Mesoscale structure and oceanographic determinants of krill hotspots in the California current: Implications for trophic transfer and conservation. Prog. Oceanography 91, 397–409. doi: 10.1016/j.pocean.2011.04.002
Santora J. A., Zeno R., Dorman J. G., Sydeman W. J. (2018). Submarine canyons represent an essential habitat network for krill hotspots in a Large marine ecosystem. Sci. Rep. 8, 7579. doi: 10.1038/s41598-018-25742-9
Scannell H. A., Johnson G. C., Thompson L., Lyman J. M., Riser S. C. (2020). Subsurface evolution and persistence of marine heatwaves in the northeast pacific. Geophysical Res. Lett. 47, e2020GL090548. doi: 10.1029/2020GL090548
Sen Gupta A., Thomsen M., Benthuysen J. A., Hobday A. J., Oliver E., Alexander L. V., et al. (2020). Drivers and impacts of the most extreme marine heatwave events. Sci. Rep. 10, 19359. doi: 10.1038/s41598-020-75445-3
Shaw W., Robinson C. L. K. (1998). Night versus day abundance estimates of zooplankton at two coastal stations in British Columbia, Canada. Mar. Ecol. Prog. Ser. 175, 143–153. doi: 10.3354/meps175143
Shelton A. O., Thorson J. T., Ward E. J., Feist B. E. (2014). Spatial semiparametric models improve estimates of species abundance and distribution. Can. J. Fish. Aquat. Sci. 71, 1655–1666. doi: 10.1139/cjfas-2013-0508
Sprogis K. R., Christiansen F., Wandres M., Bejder L. (2018). El Niño southern oscillation influences the abundance and movements of a marine top predator in coastal waters. Global Change Biol. 24, 1085–1096. doi: 10.1111/gcb.13892
Swartzman G., Hickey B., Kosro P. M., Wilson C. (2005). Poleward and equatorward currents in the pacific Eastern boundary current in summer 1995 and 1998 and their relationship to the distribution of euphausiids. Deep Sea Res. Part II: Topical Stud. Oceanography 52, 73–88. doi: 10.1016/j.dsr2.2004.09.028
Sydeman W. J., Bradley R. W., Warzybok P., Abraham C. L., Jahncke J., Hyrenbach K. D., et al. (2006). Planktivorous auklet ptychoramphus aleuticus responses to ocean climate 2005: Unusual atmospheric blocking? Geophysical Res. Lett. 33, L22S09.
Sydeman W. J., Santora J. A., Thompson S. A., Marinovic B., Lorenzo E. D. (2013). Increasing variance in north pacific climate relates to unprecedented ecosystem variability off California. Global Change Biol. 19, 1662–1675. doi: 10.1111/gcb.12165
Tanasichuk R. (1998). Interannual variations in the population biology and productivity of euphausia pacifica in barkley sound, Canada, with special reference to the 1992 and 1993 warm ocean years. Mar. Ecol. Prog. Ser. 173, 163–180. doi: 10.3354/meps173163
Tanasichuk R. W., Ware D. M., Shaw W., McFarlane G. A. (1991). Variations in diet, daily ration, and feeding periodicity of pacific hake (Merluccius productus) and spiny dogfish (Squalus acanthias) off the lower west coast of Vancouver island. Can. J. Fisheries Aquat. Sci. 48, 2118–2128. doi: 10.1139/f91-251
Thompson A. R., Harvey C. J., Sydeman W. J., Barceló C., Bograd S. J., Brodeur R. D., et al. (2019). Indicators of pelagic forage community shifts in the California current large marine ecosystem 1998–2016. Ecol. Indic. 105, 215–228. doi: 10.1016/j.ecolind.2019.05.057
Thorson J. T., Shelton A. O., Ward E. J., Skaug H. J. (2015). Geostatistical delta-generalized linear mixed models improve precision for estimated abundance indices for West coast groundfishes. ICES J. Mar. Sci. 72, 1297–1310. doi: 10.1093/icesjms/fsu243
Tweedie M. C. (1984). “An index which distinguishes between some important exponential families,” in Indian statistical institute golden Jubilee International conference. 579–604.
Wang Q., Kalogiros J. A., Ramp S. R., Paduan J. D., Buzorius G., Jonsson H. (2011). Wind stress curl and coastal upwelling in the area of Monterey bay observed during AOSN-II. J. Phys. Oceanography 41, 857–877. doi: 10.1175/2010JPO4305.1
Ward E. J., Jannot J. E., Lee Y.-W., Ono K., Shelton A. O., Thorson J. T. (2015). Using spatiotemporal species distribution models to identify temporally evolving hotspots of species co-occurrence. Ecol. Appl. 25, 2198–2209. doi: 10.1890/15-0051.1
Ware D. M., Thomson R. E. (2005). Bottom-up ecosystem trophic dynamics determine fish production in the northeast pacific. science 308, 1280–1284. doi: 10.1126/science.1109049
Wells B. K., Santora J. A., Field J. C., MacFarlane R. B., Marinovic B. B., Sydeman W. J. (2012). Population dynamics of Chinook salmon oncorhynchus tshawytscha relative to prey availability in the central California coastal region. Mar. Ecol. Prog. Ser. 457, 125–137. doi: 10.3354/meps09727
Wolf S. G., Sydeman W. J., Hipfner J. M., Abraham C. L., Tershy B. R., Croll D. A. (2009). Range-wide reproductive consequences of ocean climate variability for the seabird cassin’s auklet. Ecology 90, 742–753. doi: 10.1890/07-1267.1
Keywords: krill, time-series, zooplankton, climate change, oceanography, trophic, generalised additive model (GAM)
Citation: Evans R, English PA, Gauthier S and Robinson CLK (2023) Quantifying the effects of extreme events and oceanographic variability on the spatiotemporal biomass and distribution of two key euphausiid prey species. Front. Mar. Sci. 10:1031485. doi: 10.3389/fmars.2023.1031485
Received: 30 August 2022; Accepted: 09 January 2023;
Published: 25 January 2023.
Edited by:
Angel Borja, Technology Center Expert in Marine and Food Innovation (AZTI), SpainReviewed by:
Richard Reed Veit, College of Staten Island, United StatesAlicia Sutton, Murdoch University, Australia
Copyright © 2023 Evans, English, Gauthier and Robinson. This is an open-access article distributed under the terms of the Creative Commons Attribution License (CC BY). The use, distribution or reproduction in other forums is permitted, provided the original author(s) and the copyright owner(s) are credited and that the original publication in this journal is cited, in accordance with accepted academic practice. No use, distribution or reproduction is permitted which does not comply with these terms.
*Correspondence: Rhian Evans, cmhpYW5lQGhrdS5oaw==