- 1Laoshan Laboratory, Qingdao, China
- 2CAS Key Laboratory of Marine Ecology and Environmental Sciences, Institute of Oceanology, Chinese Academy of Sciences, Qingdao, China
- 3University of Chinese Academy of Sciences, Beijing, China
- 4Center for Ocean Mega-Science, Chinese Academy of Sciences, Qingdao, China
Eutrophication in aquaculture areas concurrently leads to a high incidence of dissolved oxygen deficiency and toxic algal blooms. The combined effects of hypoxia and typical toxic algae on cultured organisms should be given sufficient consideration. Abalone breeding in China has greatly suffered from hypoxia and toxic Karenia mikimotoi blooms for many years. In this study, the individual and combined effects of the toxic dinoflagellate, K. mikimotoi, and hypoxia on juvenile abalone were determined based on abalone survival and oxidative stress indicators in their gills, hepatopancreas and hemolymph. The results showed that at a density of 106 to 3×107 cells/L, K. mikimotoi alone had a negligible influence on the survival of juvenile abalone under sufficient dissolved oxygen (DO) conditions. The 24 h-half lethal concentration (LC50) of DO alone for juvenile abalone was 0.75 mg/L in seawater. When K. mikimotoi was added at a density of 3×106 cells/L, the 24 h-LC50 of DO for juvenile abalone significantly increased to 2.59 mg/L, indicating obvious synergistic effects. The individual effects of hypoxia or K. mikimotoi on the oxidative stress indicators were limited, and only the superoxide dismutase (SOD) activity in the abalone gills significantly decreased under K. mikimotoi stress. However, the combined stress of hypoxia and K. mikimotoi led to significant changes in the antioxidant indicators in all tested tissues. The SOD activity in gills and hepatopancreas decreased, while the SOD and catalase (CAT) activity and malondialdehyde (MDA) content in the hemolymph increased due to the combined stress of hypoxia and K. mikimotoi. These results illustrated that the synergistic effects of hypoxia and K. mikimotoi caused serious oxidative damage in abalone and that the hemolymph exhibited greater sensitivity than did the gills and hepatopancreas. Further investigation found that K. mikimotoi increased the oxygen consumption rate in abalone and that hypoxia enhanced the hemolytic toxicity of K. mikimotoi. These results revealed that hypoxia and typical toxic algae cause synergistic harm to cultured organisms, which is expected to provide a new understanding of the destructive mechanisms of typical toxic algal blooms in aquacultural areas.
Introduction
Due to climate change and eutrophication, organisms are often threatened by the combined effects of dissolved oxygen (DO) deficiencies and harmful algal blooms (HABs), especially those in aquacultural waters. Global oxygen levels in the ocean and in coastal waters have decreased by 2% annually over the past 50 years, leading to widespread occurrence of hypoxia (Schmidtko et al., 2017). Aquacultural areas are prone to hypoxia due to their high-density farming structure and high organic matter content (Matos et al., 2016; Yang et al., 2021). As aquacultural areas are usually nearshore or in inner bays and involve a weak exchange of water, DO deficiency could occur for a long time. Generally, water with a DO content of less than 2 mg/L is regarded as hypoxic, while in cultured water, biological hypoxia can occur when the DO content is less than 5 mg/L (Gobler and Baumann, 2016; Wu et al., 2016). Hypoxia affects energy metabolism and the immune system, hindering normal physiological activities and even causing the death of organisms. On the other hand, the generally high level of eutrophication in aquacultural waters provides the basis for the formation and maintenance of HABs, making the aquacultural waters a high-frequency area of HABs (Glibert et al., 2005; Heisler et al., 2008). Cultured organisms are threatened by HABs, especially those of toxic dinoflagellates, which can directly poison them. The high density of toxic algae could further aggravate hypoxia through serious oxygen consumption at night and during the decomposition period, making it harder for cultured organisms to survive. Therefore, with this underlying trend of more frequent HAB occurrences and severe hypoxia (Gilbert et al., 2009; Lee et al., 2016; Breitburg et al., 2018), many coastal aquacultural areas have both a high incidence of HABs and hypoxia. The combined effects of hypoxia and toxic algae on cultured organisms should be given sufficient attention.
Karenia mikimotoi is a typical toxic dinoflagellate, and hemolytic toxins are regarded as its main type of poison (Li et al., 2019a; Niu et al., 2021). It was first discovered in Kyoto Bay of Japan in 1935, and then it appeared in many coastal waters around the world, including waters near Japan, South Korea, Britain, France, India, and the US (Li et al., 2019a; Hu et al., 2022). China is the area that was most impacted by K. mikimotoi blooms. Since they first appeared in China in 1998, K. mikimotoi blooms have occurred more than 120 times and have become an annual disaster; the largest distribution of K. mikimotoi was nearly 1.4×104 km2, and its duration ranges from 2 days to 35 days. K. mikimotoi blooms usually cause massive amounts of various fish, shellfish and mammals to die, and they have been regarded as the second largest HAB disaster in China (Lv et al., 2019; Chen et al., 2021). Studies have shown that during the outbreak of K. mikimotoi blooms, algal cells with large biomasses make their biological oxygen demand much higher than the DO level in seawater, which can lead to the occurrence of hypoxia (O’Boyle et al., 2016). In addition, K. mikimotoi blooms occur mostly in spring and summer when seawater stratification is severe, which will further aggravate the formation and deterioration of hypoxia (Brand et al., 2012). Therefore, cultured organisms almost inevitably face the combined effects of K. mikimotoi toxicity and hypoxia when K. mikimotoi blooms occur in aquacultural areas. However, the combined effect of K. mikimotoi and hypoxia on typical cultured organisms still lacks research.
Abalone is a typical gastropod with great economic importance. In 2020, the production of abalone in China reached more than 2 billion tons, accounting for nearly 90% of the world’s total abalone production (Fisheries Bureau of the Ministry of Agriculture, 2021). However, the areas of abalone breeding in China are frequently threatened by toxic K. mikimotoi blooms, especially at night, when the high density of K. mikimotoi cells causes massive consumption of respiratory oxygen, resulting in both toxic and hypoxic effects on abalone. As a kind of nocturnal organism, abalone has a higher oxygen demand at night, which makes abalone more susceptible to the combined effects of toxicity and hypoxia. Indeed, K. mikimotoi blooms have caused heavy losses in abalone production many times. For example, K. mikimotoi blooms have resulted in countless abalone deaths, with an estimated economic loss of 2.01 billion yuan (approximately $330 million) (Li et al., 2019a). Therefore, using cultured abalone as the research object to investigate the combined effects of K. mikimotoi and hypoxia is typical and necessary. By comparing hypoxia stress, K. mikimotoi stress, and their combination, the survival rate and oxidative stress indicators in different tissues and organs of abalone were tested to illustrate the combined effects of hypoxia and typical toxic algae. This study is expected to be helpful for understanding the disaster-causing mechanism of typical toxic HAB species.
Materials and methods
Organism culture and preparation
K. mikimotoi was isolated from waters near Fujian Province and preserved at the Institute of Oceanology, Chinese Academy of Sciences, Qingdao, China. The seawater was filtered through a 0.47 μm fiber membrane and heated at 121°C for 30 min, and L1 media without silicate (Guillard and Hargraves, 1993) was then added to the K. mikimotoi culture. The salinity of the culture medium was 31 psu. K. mikimotoi was cultured at 20 ± 1°C under a light intensity of approximately 50-60 μmol photons/(m2·s), which was supplied by cool-white fluorescent bulbs on a 12:12 light-dark cycle. The algae used in the experiments were cultured to the mid-to-late exponential growth phase at cell densities of 3.0×107 cells/L.
Juvenile abalone Haliotis discus hannai was purchased from a breeding facility in Qingdao, China. During the rearing period of the juvenile abalone, the seawater was fully aerated and changed daily, and the juvenile abalone were fed dried kelp. Abalone individuals with similar sizes (shell length 25.72 ± 1.20 mm, weight 1.75 ± 0.30 g) were selected for the experiment after 24 h of starvation.
Survival impact
The experimental container was a plastic rectangular box with a volume of 16 L (0.08 m2 × 0.2 m). The DO content was set to 0.2-2.0 mg/L, with 0.2 mg/L as a gradient. A DO probe was placed into the box to monitor the DO concentration in real time. To maintain the different DO concentrations, air and pure nitrogen were injected into the box, and an electronic DO controller (Jenco 6308DT, accuracy ± 0.05 mg/L) was used to set the DO concentration, receive the monitoring signal of the DO probe, and adjust the aeration of air and pure nitrogen to maintain the DO concentration at the set DO value. In addition, 20 juvenile abalone were placed in each box to investigate the survival of juvenile abalone under different DO contents for 24 h. The treatments were performed in triplicate, and the 24 h half lethal concentration (LC50) of abalone under hypoxia stress was calculated based on the fitting equation.
To investigate the effects of different densities of K. mikimotoi on the survival of abalone, 20 abalone in triplicate were placed in seawater with algal densities of 106, 3×106, 5×106, 107, and 3×107 cells/L, and the DO content in the seawater was maintained above 8 mg/L through slow aeration. The survival of abalone was observed within 24 h.
To choose a proper K. mikimotoi density for exploring the combined effects of density with hypoxia, the density of K. mikimotoi blooms in Fujian coastal waters was analyzed; these blooms were chosen because Fujian Province is not only the main area for abalone farming but is also the area most impacted by K. mikimotoi blooms. The density of K. mikimotoi was chosen to be 3×106 cells/L according to the medium density of the K. mikimotoi bloom in Fujian Province from 2012 to 2022 (Supplementary Figure 1). The DO content was set as 2-5 mg/L, and the survival of abalone was observed after 24 h. All treatments were performed in triplicate. The abalone was judged to be dead when its gastropod adhesion disappeared and there was no response to multiple stimulations. The temperature of the experimental water was 20 ± 1°C, and a shading curtain was used for shading during the experiment to simulate the dark environment for abalone survival. The 24 h-LC50 of abalone under the combined stress of hypoxia and K. mikimotoi was calculated through a fitting equation.
Measurements of oxidative stress indicators
The control group (group C), hypoxia treatment group (DO 2 mg/L, group H), K. mikimotoi treatment group (K. mikimotoi 3×106 cells/L, group K), and synthesis treatment group (DO 2 mg/L, K. mikimotoi 3×106 cells/L, group S) were set in triplicate with the same container described above. Twenty abalone were placed in each box of the group, and one surviving individual (3 abalone per treatment) was randomly selected from each box to sample the oxidative stress indicator at 0 h, 3 h, 6 h, 12 h, 18 h, and 24 h. The hemolymph was collected by cutting longitudinally in the pedal sinus of the foot muscle in the abalone with a sterilized scalpel. Approximately 200 µL of hemolymph was collected from each individual by sterilized syringe. Then, the gills, hepatopancreas and hemolymph of juvenile abalone were collected and stored frozen at -80°C for analysis. Before the assay, the gills and hepatopancreas were homogenized into 10% tissue with phosphate-buffered saline (PBS, pH 7.2-7.4; Solarbio, China) and centrifuged at 4000×g for 10 min, and then the supernatant was used for the experiment. Superoxide dismutase (SOD) was detected by xanthine oxidase method, and one unit of SOD activity was defined as the amount of enzyme required for 1 mg tissue proteins in 1 ml of a reaction mixture SOD inhibition rates to 50%. The catalase (CAT) was detected by ammonium molybdate method, and one unit of CAT activity was defined as 1 mg of tissue protein that consumed 1 µmol H2O2 for 1 second. The malondialdehyde (MDA) content was measured by the 2-thiobarbituric acid (TBA) method. The tissue protein content was measured by the Bradford method for the calculation of SOD and CAT activity and MDA content. Both the SOD and CAT activity and the MDA and protein contents were measured by reagent kits (Nanjing Jiancheng Bioengineering Institute, Nanjing, China), determined using an EnSight microplate reader (PerkinElmer, USA) and read at 550 nm, 405 nm, 532 nm and 595 nm, respectively.
Oxygen consumption rate calculation
Two abalone were labeled and placed in a 1 L cell culture bottle without illumination at 20 ± 1°C. The calibrated DO probe was inserted into the water body with DO content above 7 mg/L. After the bottle was sealed, the DO content was recorded every 30 min for 24 h to track the abalone oxygen consumption rate in the seawater. The oxygen consumption rate of the same abalone individuals in seawater with a K. mikimotoi density of 3×106 cells/L was also tracked as described above. This experiment was conducted in duplicate. The oxygen consumption rate of abalone in the seawater (R) and in the K. mikimotoi culture (R′) was calculated using the following formula (Ahmed et al., 2008):
where I is the DO content at the beginning of the experiment; F is the final DO content; V is the volume of the container; v is the volume of abalone; t is the time interval and OCK is the oxygen consumption of K. mikimotoi.
Determination of the hemolytic activity of K. mikimotoi
K. mikimotoi was added to the square box at a density of 5×106 cells/L. The control group was slowly aerated, the DO content was maintained at 8.5 mg/L, and the DO content of the experimental groups was maintained with the electronic DO controller described above at 3, 2, 1.5, and 1.0 mg/L. After 2 h of treatment, 200 mL of algae fluid was filtered onto a GF/F membrane (0.7 µm), and the extraction and determination of hemolytic toxins were carried out in parallel for each treatment.
The extraction of hemolytic toxin was performed as follows: Membranes were extracted using a mixed solution of chloroform, methanol and water with a volume ratio of 13:7:5 (v/v). After adding the grinding particles, the mixture was fully ground with a sample preparation system (MP, FastPrep-24) and then centrifuged at 3000 r/min for 10 min. The supernatant was removed for nitrogen blowing at 40°C until it evaporated and was dry. The solution was reconstituted with 1 mL methanol to obtain a crude extract, and then the crude extract was used to dissolve rabbit blood cells (Yuanye, China) for experiments.
The hemolytic toxin was determined as follows: A standard series of digitonin solutions were configured to draw a working curve. A mixture of 0.1 mL of the toxin extract, 0.3 mL of citric acid buffer solution and 1.60 mL of 0.5% (v/v) fresh rabbit red blood was heated in a water bath at 37°C for 30 min and then centrifuged at 800 r/min for 10 min, and the absorbance of the supernatant was measured at 540 nm. The calculation of hemolytic activity was performed according to the industry standard (HY/Y 151-2013), and the hemolytic activity was defined as hemolytic units (HU) which represents 1 ml reaction solution that can dissolve 50% of rabbit blood cells within 30 min at 37°C.
Statistical analysis
The data analysis method of this study was two-way analysis of variance (ANOVA), and Duncan’s method was used for post hoc multiple comparisons. The data involved in correlation analysis and two-way ANOVA were analyzed and processed with SPSS 22.0, and the graphs were drawn in Origin 9.0.
Results
Survival of abalone
In this study, the effects of different densities of K. mikimotoi under sufficient DO content on the survival of abalone were investigated. The results showed that under aeration conditions, the 24-h survival rate of abalone was nearly 100%. When investigating the effect of DO content on abalone, it was found that abalone had a certain tolerance to hypoxia. The 24 h LC50 values was 0.75 mg/L according to the logistic models (Figure 1A). When K. mikimotoi was present at a density of 3×106 cells/L, the 24 h LC50 that was calculated by the exponential models increased to 2.59 mg/L (Figure 1B).
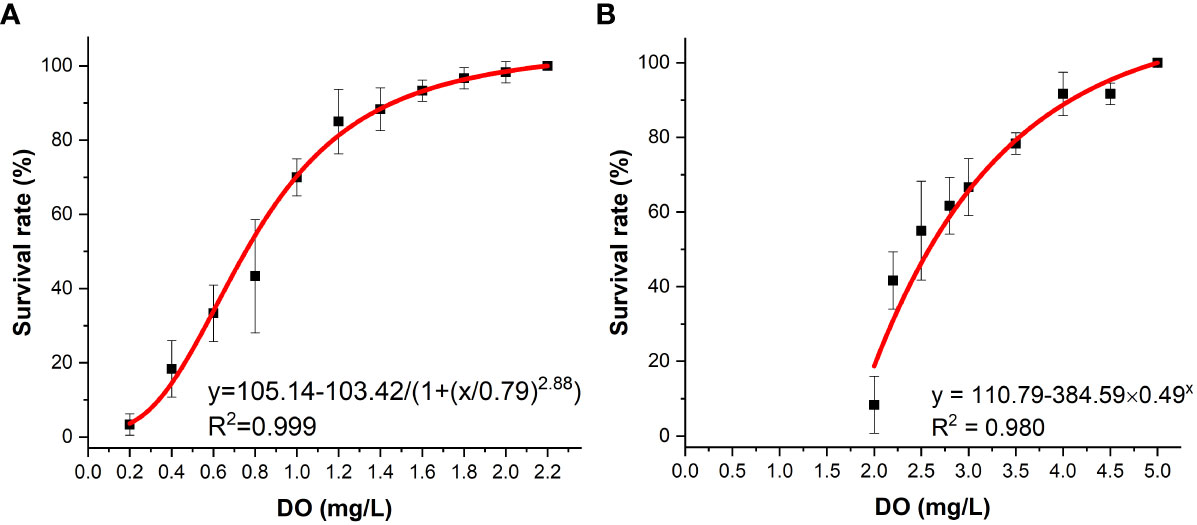
Figure 1 Survival of H. discus hannai in the seawater system (A) and in the K. mikimotoi system (B) under different DO contents.
Oxidative stress indicators
The changes in CAT activity, SOD activity and MDA content in the gills of abalone under different treatments were tracked. The CAT activity decreased slightly with time, SOD activity exhibited a decreasing tendency first and then increased, with 12 h as the inflection point, while MDA content fluctuated with time (Figure 2). Through statistical analysis, it was found that there was no significant difference in the CAT activity and MDA content among the different treatment groups (p > 0.05), and only the SOD enzyme activity was significantly different among the treatment groups (p< 0.05) (Table 1). Duncan multiple comparisons showed that hypoxic stress alone did not cause significant changes in SOD enzyme activity in abalone gills, while K. mikimotoi stress alone and the combined stress of hypoxia and K. mikimotoi caused a significant decrease in SOD activity in abalone gills.
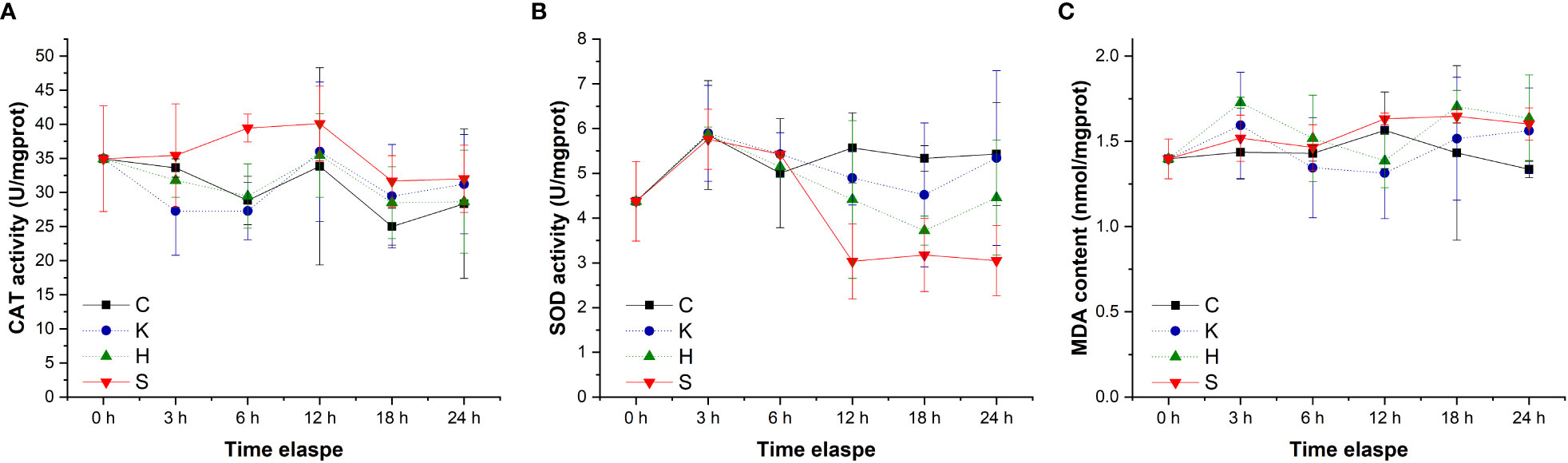
Figure 2 Changes in the CAT activity (A), SOD activity (B) and MDA content (C) in the gills of H. discus hannai in different treatment groups. The solid line with black squares represents the control group; the dotted line with blue circles represents the group with the K. mikimotoi treatment; the dotted line with green triangles represents the group with the hypoxia treatment; the solid line with inverted triangle represents the group with both the K. mikimotoi and the hypoxia treatments.

Table 1 Summary of two-way ANOVA testing the effects of the treatment and time on CAT and SOD activity and MDA content in the gills of H. discus hannai (* represents a significant difference compared with the control, p< 0.05).
The determination of oxidative stress indicators in the hepatopancreas of abalone in each treatment group showed that the changes in CAT activity and MDA content with time were negligible, and the difference between groups was not significant (Figure 3). Compared with the control group, hypoxia stress, K. mikimotoi stress alone and their combination led to a decrease in SOD activity in the hepatopancreas of abalone after 6 h. Through further statistical analysis, it was found that the CAT activity and MDA content in the hepatopancreas of abalone were not significantly affected by time, treatment or their interaction (p > 0.05) (Table 2). The SOD activity in the hepatopancreas of abalone was significantly affected by time, and it was found by performing Duncan multiple comparisons that the SOD activity was significantly inhibited by the combined stress of hypoxia and K. mikimotoi (p< 0.05).
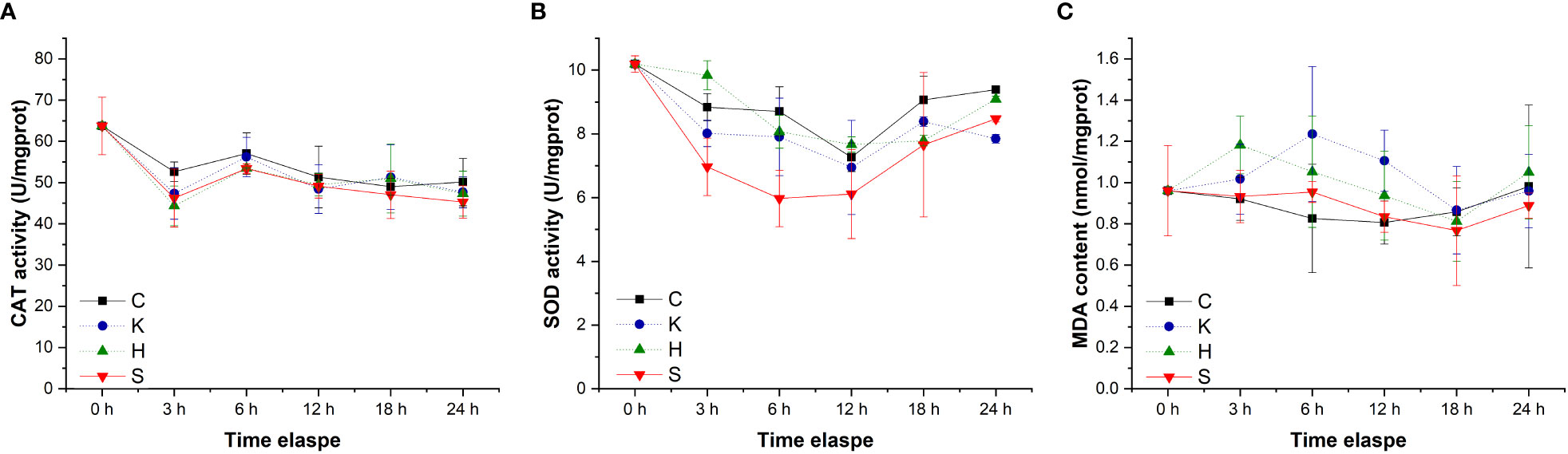
Figure 3 Changes in the CAT activity (A), SOD activity (B) and MDA content (C) in the hepatopancreas of H. discus hannai in different treatment groups. The solid line with black squares represents the control group; the dotted line with blue circles represents the group with the K. mikimotoi treatment; the dotted line with green triangles represents the group with the hypoxia treatment; the solid line with inverted triangles represents the group with both the K. mikimotoi and the hypoxia treatments.

Table 2 Summary of two-way ANOVA testing the effects of the treatment and time on CAT and SOD activity and MDA content in the hepatopancreas of H. discus hannai (* represents a significant difference compared with the control, p< 0.05).
By tracking the oxidative stress indicators of abalone hemolymph, it was found that with the extension of time, the CAT activity in the group C, group K, and group H were relatively stable. When the stresses of hypoxia and K. mikimotoi combined, the CAT activity in the abalone hemolymph increased with time, from 34.33 U/mgprot at the beginning to 70.46 U/mgprot at 24 h, which was significantly different from that in the other three groups (p< 0.05) (Figure 4). The SOD activity in the abalone hemolymph was slightly lower within 12 h and then slightly higher than that in the control with the hypoxia or K. mikimotoi treatment alone, while it was higher than that in the control at all time points due to the combined stress of hypoxia and K. mikimotoi. Compared with the control group, in the three experimental groups, the MDA content increased at 3 h, but the MDA content in the abalone hemolymph in the group K and group H decreased to the same level as the control at 6 h, while the MDA content in the abalone hemolymph in group S remained at a high level. Through Duncan multiple comparisons, it was found that hypoxia or K. mikimotoi stress alone did not cause significant changes in CAT activity, SOD activity or MDA content in the abalone hemolymph, while their combination resulted in insignificant increases in CAT and SOD activity and MDA content in the abalone hemolymph (p< 0.05) (Table 3).
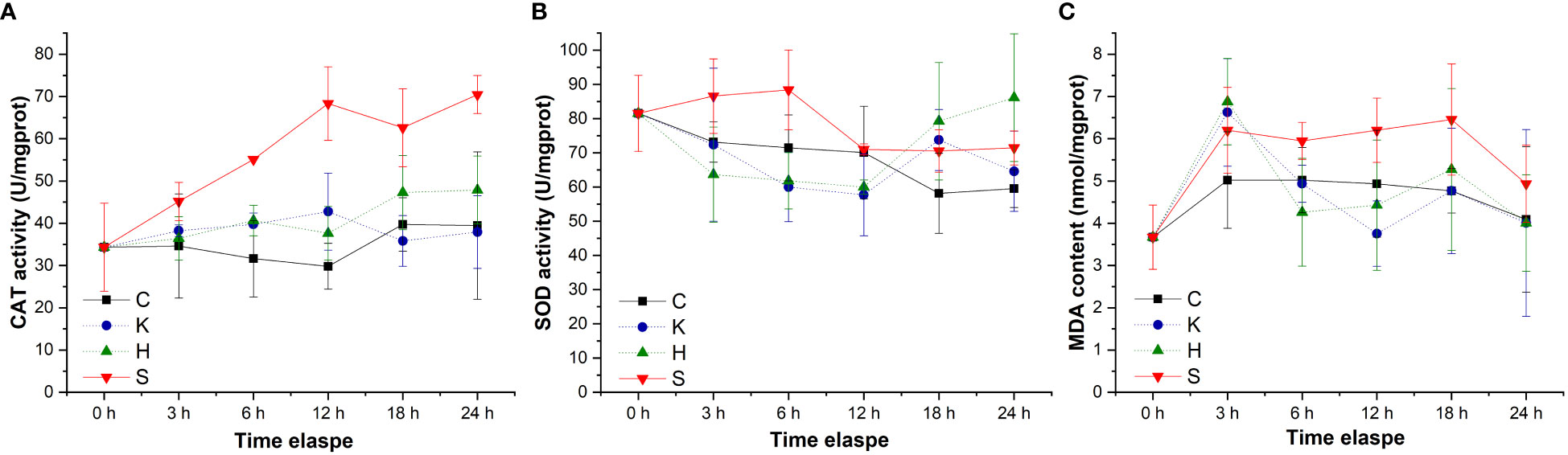
Figure 4 Changes in the CAT activity (A), SOD activity (B) and MDA contents (C) in the hemolymph of H. discus hannai in different treatment groups. The solid line with black squares represents the control group; the dotted line with blue circles represents the group with the K. mikimotoi treatment; the dotted line with green triangles represents the group with the hypoxia treatment; the solid line with inverted triangles represents the group with both the K. mikimotoi and the hypoxia treatments.

Table 3 Summary of two-way ANOVA testing the effects of the treatment and time on CAT and SOD activity and MDA content in the hemolymph of H. discus hannai (* represents a significant difference compared with the control, p< 0.05).
The effects of K. mikimotoi on the oxygen consumption rate of abalone
The oxygen consumption rates of the same individuals in the seawater and the K. mikimotoi system indicated that K. mikimotoi aggravated the consumption of DO in abalone (Figure 5). The oxygen consumption of seawater itself was negligible, and due to low illumination, 1 L of K. mikimotoi consumed 0.16 mg of DO within 24 h (Table 4). Through calculation, it was found that the average oxygen consumption rate of abalone in the seawater system was 0.086 ± 0.016 mg/ind/h, and the average oxygen consumption rate in the K. mikimotoi system was 0.103 ± 0.008 mg/ind/h, with an increase of more than 20% compared with that in the seawater.
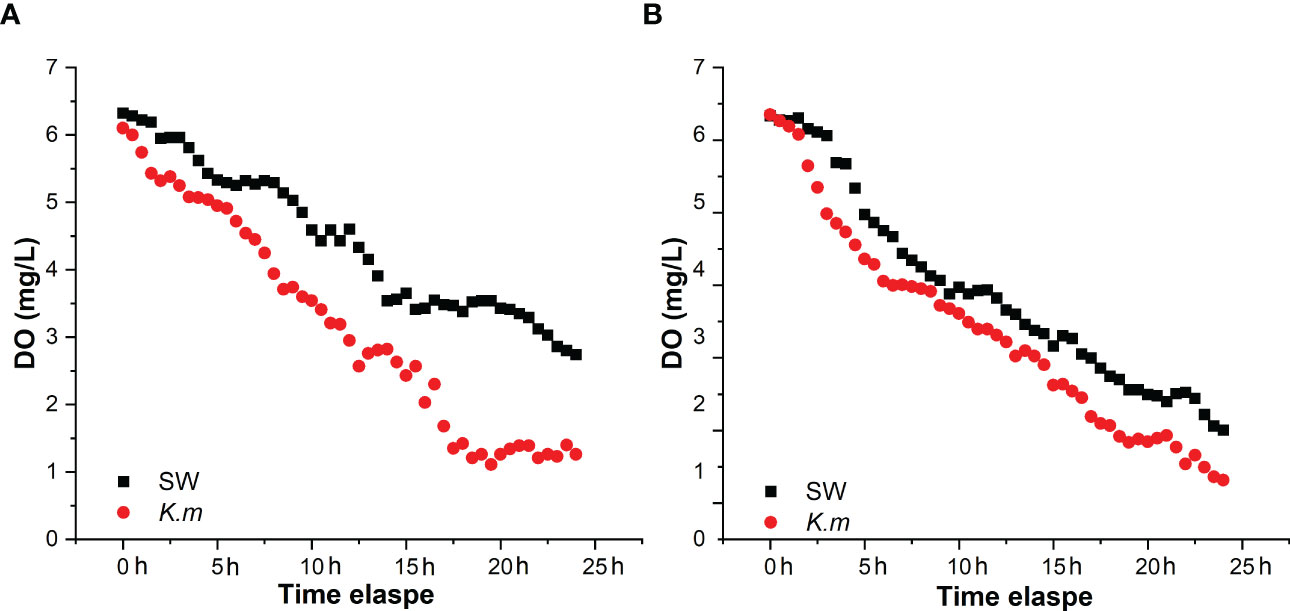
Figure 5 DO consumption of H. discus hannai in the seawater and K. mikimotoi treatment groups in group (A) and group (B) The black square and the red dot represent the DO value in the seawater and the K. mikimotoi culture, respectively.
The effects of DO on hemolytic toxins
The results showed that in the control group with sufficient DO, the hemolytic activity of K. mikimotoi was 6.64×10-6 HU/cell (Figure 6). After hypoxia stress with DO contents of 3 mg/L, 2 mg/L, 1.5 mg/L and 1 mg/L for 2 h, the hemolytic activity of K. mikimotoi increased significantly, reaching 7.12×10-6 HU/cell, 16.62×10-6 HU/cell, 25.65×10-6 HU/cell, and 31.35×10-6 HU/cell, respectively. A DO content of 2 mg/L and below significantly increased the hemolytic activity of K. mikimotoi (p< 0.05).
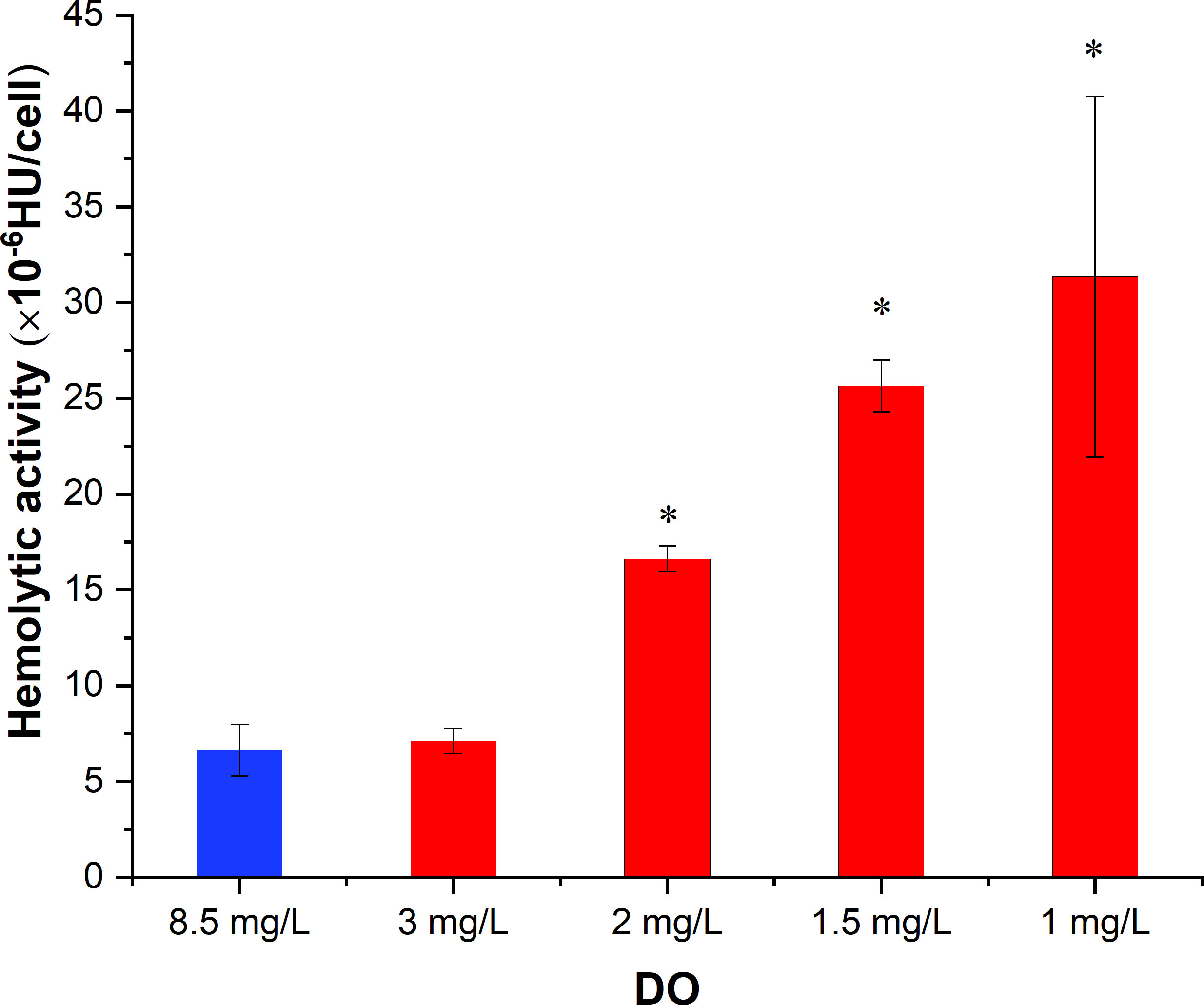
Figure 6 Hemolytic activity of K. mikimotoi under different DO conditions (* represents a significant difference compared with the control, p< 0.05).
Discussion
Hypoxia tolerance is quite different among species. Compared to fish, shellfish are generally more tolerant to hypoxia, but because of their limited ability to swim, their survival is threatened if the DO content continues to decline. Most bivalves have a strong tolerance to hypoxia. For example, studies have shown that the 20-day LC50 values of DO to Ruditapes philippinarum and Chlamys farreri are 0.57 mg/L and 1.8 mg/L, respectively (Li et al., 2019b; Li et al., 2020). In this study, abalone is a marine gastropod that is widely distributed. Studies have shown that gastropods also have a certain tolerance to hypoxia because they are distributed in the bottom environment, in which the DO levels are relatively low, or live in the intertidal zone, in which exposure to air periodically occurs (Vaquer-Sunyer and Duarte, 2008; Levin et al., 2009). Samuel et al. (2019) investigated the effect of DO content on the survival of H. fulgens, and the 24-h LC50 of DO content at 26°C was 0.23 ± 0.73 mg/L. Shen (2018) found that the 24-h LC50 of DO content to H. discus hannai at 20°C was 0.80 mg/L. In this study, the 24-h LC50 of DO content in the seawater for abalone was 0.75 mg/L, which was similar to the results of previous studies, indicating that abalone itself has a certain tolerance to hypoxia. Algal blooms are considered to be one of the main causes of marine hypoxia. A hypoxic condition with a DO concentration at 2.2 mg/L was observed in Donegal Bay due to a K. mikimotoi bloom (O’Boyle et al., 2016), which was still within a tolerable range for abalone, suggesting that hypoxia alone is not the main cause of massive abalone mortality in the field.
As a typical toxic alga, K. mikimotoi can produce hemolytic toxins, fish toxins, cytotoxins, etc. A harmful algal bloom occurs when the density of K. mikimotoi exceeds 106 cells/L. In recent years, studies have found that the toxic effects of K. mikimotoi are the strongest through the direct contact of intact K. mikimotoi cells with organisms, and their toxicity depends on the strain (Li et al., 2017; Yan et al., 2022). The algal strain used in this study was isolated from the coast of Fujian. Relevant studies show that this strain poses a certain threat to the survival of a variety of zooplankton and fish, such as turbot Scophthalmus maximus and marine medaka Oryzias melastigma (Li et al., 2017; Zhang et al., 2022). Lin et al. (2016a) investigated the effect of the K. mikimotoi Fujian strain on the survival of H. discus hannai. The results showed that at a density of 107 cells/L, K. mikimotoi caused the 48h survival rate of H. discus hannai to be more than 80%. When the K. mikimotoi density increased to 3×107 cells/L, the survival of H. discus hannai decreased to 66.7%. In this study, under an environment with sufficient DO, 3×107 cells/L K. mikimotoi did not cause the death of juvenile H. discus hannai within 24 h. The results of this study and previous studies showed that K. mikimotoi had limited effects on the survival of H. discus hannai in the short term.
Recent studies have found that when the DO content is sufficient, the toxic effects of K. mikimotoi are relatively limited, while under environments without aeration, K. mikimotoi could cause the death of fish and shellfish even at their tolerant DO content (Lin et al., 2016a; Li et al., 2019a). This indicates that the hypoxic environment exacerbates the toxic effects of K. mikimotoi on marine organisms. On the basis of previous studies, this study further investigated the effects of K. mikimotoi under different degrees of hypoxia on the survival of juvenile abalone by accurately regulating the DO content in water. During the 24 h experimental period, the stress of K. mikimotoi at a density of 3×106 cells/L alone did not cause the death of juvenile abalone, and the same survival influence was found under hypoxia stress at a DO content of 2 mg/L. When hypoxia and K. mikimotoi stress were combined, however, the survival rate of juvenile abalone was significantly reduced to less than 10%. This clearly shows that hypoxia and K. mikimotoi have a synergistic effect on the survival of juvenile abalone. Previous studies on the synergistic effects of environmental factors on aquatic organisms have mostly focused on substances such as heavy metals, organic pollutants, pesticides, etc. (Goswami et al., 2014; Zou et al., 2017; Mandich, 2018; Pittura et al., 2018; Tang et al., 2020; Zhang et al., 2021). In recent years, the synergistic effect of typical toxic harmful algae and hypoxia has attracted attention. For example, studies have shown that in freshwater, the stress of hypoxia combined with Microcystis aeruginosa caused more serious harm to the gills, stomach, intestine and crystal rod of Hyriopsis cumingii than that of a single stress (Hu et al., 2016; Wu et al., 2017). Karenia brevis reduced the survival rate of stone crabs by 7.2% in an environment with sufficient DO, while K. brevis reduced the 24-h survival rate of stone crabs by 43% in a hypoxic environment, and the stone crabs exhibited obvious hysteresis behavior at the same time (Gravinese et al., 2020). This shows that the synergistic effects of a hypoxic environment and toxic algae may be an important disaster-causing mechanism of typical toxic HABs and is worthy of more research.
All aerobic organisms have antioxidant defense systems to prevent the production of excess reactive oxygen species (ROS), and changes in oxidative stress indicators can reflect the response of organisms to environmental stress. This system consists of enzymes that can be induced by oxidative stress, including SOD and CAT. SOD can remove excess free radicals so that the generation and elimination of free radicals are in a dynamic balance, thereby preventing the damage of free radicals to the organism. CAT can quickly remove the toxic H2O2 produced by cell metabolism. MDA is a product of lipid peroxidation. Therefore, changes in SOD and CAT activity and MDA content in organisms are regarded as antioxidative responses to environmental changes. The individual and synergistic effects of hypoxia and K. mikimotoi on the gills, hepatopancreas, and hemolymph of juvenile abalone, which are the main organs and tissues involved in respiration, detoxification and immunity, were also investigated in this study. Although the gills, hepatopancreas, and hemolymph are regarded as organs and tissues in abalone that are sensitive to environmental stress, their antioxidant responses to hypoxia stress, K. mikimotoi stress, and both of these stresses combined were quite different. Little response was observed with the hepatopancreas and gill tissues to the experimental treatment, and only the SOD activity of the gills was significantly changed due to K. mikimotoi stress. Previous studies have found that K. mikimotoi can cause damage to the gills of marine medaka, salmon and other fish (Mitchell and Rodger, 2007; Zhang et al., 2022). In other studies, it was also observed that K. mikimotoi at a density of 107 cells/L had adverse effects on the microstructure and SOD and CAT activities of abalone gills (Lin et al., 2016b), which indicates that the hemolytic toxins of K. mikimotoi cause certain oxidative harm to the respiratory system of abalone. In this study, compared with the gills and hepatopancreas, the hemolymph of abalone was more sensitive to the treatment, especially the synergistic stress of hypoxia and K. mikimotoi. Abalone relies on innate immunity, and hemolymph is the main tissue of its immunity, which may be responsible for its sensitivity to environmental stress (Shen et al., 2019). In general, except for SOD activity in the gills of juvenile abalone, no other oxidative stress indicators were found to be significantly different from those of the control after treatment with hypoxia stress or K. mikimotoi stress alone. When the stresses of hypoxia and K. mikimotoi were combined, their synergistic stress caused significant decrease of the SOD activity in the gills and hepatopancreas of juvenile abalone. Moreover, the stress of hypoxia combined with K. mikimotoi affected all the oxidative stress indicators in the hemolymph. The activities of SOD and CAT were significantly enhanced, and the MDA content was significantly increased, showing a top-down oxidative damage process. That is, the large generation of ROS caused the elevation of SOD activity and the accumulation of H2O2, and excess H2O2 further resulted in an increase in CAT activity and MDA content (Figure 7). The related results of oxidative stress indicators further demonstrated that the synergistic effect of hypoxia and K. mikimotoi causes serious harm to the immune system of abalone, resulting in the antioxidant system disorder, indicating that the abalone suffered obvious oxidative damage.
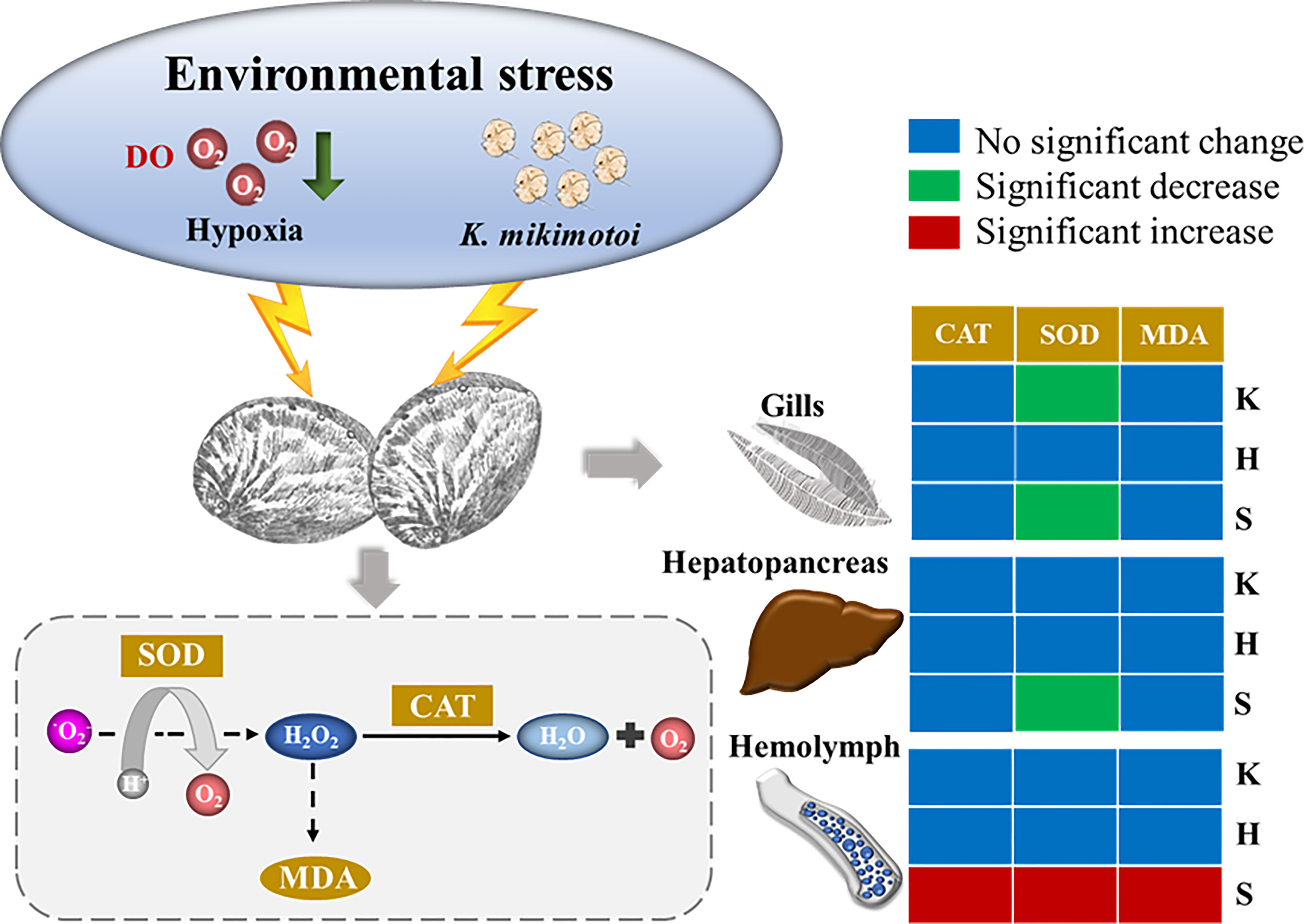
Figure 7 Overall schematic figure of the effects of hypoxia (H), K. mikimotoi (K) and their synergy (S) on SOD activity, CAT activity and MDA content after24 h of exposure in juvenile abalone. Blue, green and red colors in the box represent no significant change, a significant decrease and a significant increase, respectively.
To analyze the enhanced pathway of hypoxia and K. mikimotoi synergistic toxicity, this study further focused on the interaction between K. mikimotoi toxicity and DO content. First, it was found that K. mikimotoi could affect the oxygen consumption rate of abalone. Ahmed et al. (2008) estimated that the oxygen consumption rates of H. discus discus, H. gigantea, H. madaka and their hybrids (more than 2 g) were 0.030-0.040 mL/g/h, which equals 0.086-0.114 mg/ind/h. The abalone used in this study was H. discus hannai weighed less than 2 g. Considering that the oxygen consumption rate increases with the increase in abalone body weight and varies by species, it is reasonable that the oxygen consumption rate in this study was at 0.086 mg/ind/h on average. Generally, the oxygen consumption rate value of H. discus hannai in seawater in this study is consistent with the record in the literature (Ahmed et al., 2008). When K. mikimotoi exists, its toxicity could directly damage the gill tissues of fish and shellfish, which may reduce the rate of oxygen utilization by the organism. To maintain normal metabolism, this reduction in rate can be compensated by more consumption. In addition, studies have shown that when organisms are affected by toxic substances such as heavy metals and organic compounds, their respiratory metabolism increases for detoxification (Engel and Fowler, 1979; Unkiewicz-Winiarczyk and Gromysz-Kałkowska, 2012). It is necessary for abalone to participate in more physiological metabolic activities that are related to detoxification, and these activities result in higher consumption of DO.
In addition, in the present study, hypoxia promoted the hemolytic toxicity of K. mikimotoi, which made the situation for the abalone even worse. Due to the mysterious composition of the hemolytic toxin in K. mikimotoi, the toxicity level of K. mikimotoi is usually represented in terms of hemolytic activity. Hemolytic activity is an indicator that can reflect individual cell toxicity and therefore unrelated to algal density. Some studies suggest that toxins are an environmental deterrent that are produced by algal cells; when environmental conditions are unfavorable, algal toxins increase for defense purposes. A large number of studies have shown that a lack of nutrients, such as nitrate and phosphate, as well as the discomfort of light, temperature, pH, etc., can significantly increase toxic algal toxins (Indrasena and Gill, 2000; Wang and Hsieh, 2002; Murata et al., 2006). However, there is still a lack of research on the effects of DO on algal toxins. Although algal cells can generate oxygen through photosynthesis, components for their respiration and metabolism still need to be provided by the external environment, especially under limited light conditions. This means that DO content may be a limiting environmental factor. Based on the results, it is speculated that when the DO content is insufficient, algal cells may be directly stimulated by hypoxia to increase toxin production.
Conclusions
This study confirmed that hypoxia and K. mikimotoi caused strong synergistic effects on typical organisms. The synergistic effects of hypoxia and K. mikimotoi could significantly decrease SOD activity in gill tissue and hepatopancreas of abalone. Compared with the gill and hepatopancreas, the hemolymph of the abalone was more sensitive to the synergistic stress of hypoxia and K. mikimotoi. The SOD and CAT activity and the MDA content in the hemolymph of the abalone were significantly enhanced, indicating that abalone suffered strong oxidative damage. Further investigation regarding the reason for their synergistic activity showed that K. mikimotoi promoted the oxygen consumption rate of juvenile abalone, and hypoxia led to a significant increase in the hemolytic activity of K. mikimotoi; these activities may be the essential causes for the synergistic enhancement effects of K. mikimotoi combined with hypoxia.
Data availability statement
The original contributions presented in the study are included in the article/Supplementary Material. Further inquiries can be directed to the corresponding author.
Author contributions
YZ contributed to methodology, investigation, formal analysis, writing - original draft. XS contributed to funding acquisition, resources, supervision, writing - review & editing. PZ contributed to methodology and investigation. All authors contributed to the article and approved the submitted version.
Funding
This research was funded by the Intergovernmental Innovation Cooperation Project of the Ministry of Science and Technology, (No. 2022YFE0136400), the National Natural Science Foundation of China (No. 41976148), the Laoshan Laboratory (No. LSKJ202203700), and the Research Start-up Fund Project of the Cross Research Center in the Pilot National Laboratory for Marine Science and Technology (No. JCZX202030).
Acknowledgments
The authors would like to acknowledge Xiaojun Meng and Yuxia Zhong for their assistance.
Conflict of interest
The authors declare that the research was conducted in the absence of any commercial or financial relationships that could be construed as a potential conflict of interest.
Publisher’s note
All claims expressed in this article are solely those of the authors and do not necessarily represent those of their affiliated organizations, or those of the publisher, the editors and the reviewers. Any product that may be evaluated in this article, or claim that may be made by its manufacturer, is not guaranteed or endorsed by the publisher.
Supplementary material
The Supplementary Material for this article can be found online at: https://www.frontiersin.org/articles/10.3389/fmars.2023.1029512/full#supplementary-material
Supplementary Figure 1 | The occurrence of K. mikimotoi blooms in Fujian Province (China) from 2012 to 2022. (A) The columns represents the density of K. mikimotoi in each bloom; (B) Frequency histogram of K. mikimotoi blooms.
References
Ahmed F., Segawa S., Yokota M., Watanabe S. (2008). Effect of light on oxygen consumption and ammonia excretion in Haliotis discus discus, H. gigantea, H. madaka and their hybrids. Aquaculture 279 (1-4), 160–165. doi: 10.1016/j.aquaculture.2008.03.040
Brand L. E., Campbell L., Bresnan E. (2012). Karenia: the biology and ecology of a toxic genus. Harmful Algae 14, 156–178. doi: 10.1016/j.hal.2011.10.020
Breitburg D. L., Levin L. A., Oschlies A., Grégoire M., Chavez F. P., Conley D. J., et al. (2018). Declining oxygen in the global ocean and coastal waters. Science 359 (6371), eaam7240. doi: 10.1126/SCIENCE.AAM7240
Chen B., Wang K., Guo H., Lin H. (2021). Karenia mikimotoi blooms in coastal waters of China from 1998 to 2017. Estuarine Coast. Shelf Sci. 249, 107034. doi: 10.1016/j.ecss.2020.107034
Engel D. W., Fowler B. A. (1979). Factors influencing cadmium accumulation and its toxicity to marine organisms. Environ. Health Perspect. 28, 81–88. doi: 10.2307/3428908
Fisheries Bureau of the Ministry of Agriculture (2021). China Fishery Statistical Yearbook. (Beijing: China Agriculture Press).
Gilbert D., Rabalais N. N., Diaz R. J., Zhang J. (2009). Evidence for greater oxygen decline rates in the coastal ocean than in the open ocean. Biogeosci. Discussions 7 (5), 2283–2296. doi: 10.5194/bgd-6-9127-2009
Glibert P., Seitzinger S., Heil C., Burkholder J. A., Kelly V. (2005). The role of eutrophication in the global proliferation of harmful algal blooms. Oceanography 18 (2), 198–209. doi: 10.5670/oceanog.2005.54
Gobler C. J., Baumann H. (2016). Hypoxia and acidification in ocean ecosystems: coupled dynamics and effects on marine life. Biol. Lett. 12 (5), 20150976. doi: 10.1098/rsbl.2015.0976
Goswami P., Hariharan G., Godhantaraman N., Munuswamy N. (2014). An integrated use of multiple biomarkers to investigate the individual and combined effect of copper and cadmium on the marine green mussel (Perna viridis). J. Environ. Sci. Health Part A 49 (13), 1564–1577. doi: 10.1080/10934529.2014.938534
Gravinese P. M., Munley M. K., Kahmann G., Cole C., Lovko V., Blum P., et al. (2020). The effects of prolonged exposure to hypoxia and Florida red tide (Karenia brevis) on the survival and activity of stone crabs. Harmful Algae 98, 101897. doi: 10.1016/j.hal.2020.101897
Guillard R. R. L., Hargraves P. E. (1993). Stichochrysis immobilis is a diatom, not a chrysophyte. Phycologia 32 (3), 234–236. doi: 10.2216/i0031-8884-32-3-234.1
Heisler J., Glibert P. M., Burkholder J. M., Anderson D. M., Cochlan W., Dennison W. C., et al. (2008). Eutrophication and harmful algal blooms: a scientific consensus. Harmful Algae 8 (1), 3–13. doi: 10.1016/j.hal.2008.08.006
Hu L., Wang R., Wang M., Cannizzaro J., Garrett M., Harper M., et al. (2022). The inactivation effects and mechanisms of Karenia mikimotoi by non-metallic elements modified TiO2 (SNP-TiO2) under visible light. Sci. Total Environ. 820, 153346. doi: 10.1016/j.hal.2022.102289
Hu M., Wu F., Yuan M., Liu Q., Wang Y. (2016). Combined effects of toxic cyanobacteria Microcystis aeruginosa and hypoxia on the physiological responses of triangle sail mussel Hyriopsis cumingii. J. Hazard. Mater. 306, 24–33. doi: 10.1016/j.aquatox.2019.05.013
Indrasena W. M., Gill T. A. (2000). Thermal degradation of partially purified paralytic shellfish poison toxins at different times, temperatures, and pH. J. Food Sci. 65 (6), 948–953. doi: 10.1111/j.1365-2621.2000.tb09398.x
Lee Y. G., Jeong D. U., Lee J. S., Choi Y. H., Lee M. O. (2016). Effects of hypoxia caused by mussel farming on benthic foraminifera in semi-closed gamak bay, south Korea. Mar. pollut. Bull. 109 (1), 566–581. doi: 10.1016/j.marpolbul.2016.01.024
Levin L. A., Ekau W., Gooday A. J., Jorissen F., Middelburg J. J., Naqvi S. W. A., et al. (2009). Effects of natural and human-induced hypoxia on coastal benthos. Biogeosciences 6 (10), 2063–2098. doi: 10.5194/bg-6-2063-2009
Li Q., Sun S., Zhang F., Wang M., Li M. (2019b). Effects of hypoxia on survival, behavior, metabolism and cellular damage of Manila clam (Ruditapes philippinarum). PloS One 14 (4), e0215158. doi: 10.1371/journal.pone.0215158
Li X., Yan T., Lin J., Yu R., Zhou M. (2017). Detrimental impacts of the dinoflagellate Karenia mikimotoi in fujian coastal waters on typical marine organisms. Harmful Algae 61, 1–12. doi: 10.1016/j.hal.2016.11.011
Li X., Yan T., Yu R., Zhou M. (2019a). A review of Karenia mikimotoi: bloom events, physiology, toxicity and toxic mechanism. Harmful Algae 90, 101702. doi: 10.1016/j.hal.2019.101702
Li Q., Zhang F., Wang M., Li M., Sun S. (2020). Effects of hypoxia on survival, behavior, and metabolism of zhikong scallop Chlamys farreri Jones et Preston 1904. J. Oceanol. Limnol. 38 (2), 351–363.
Lin J. N., Yan T., Zhang Q. C., Wang Y. F., Liu Q., Zhou M. J. (2016a). The detrimental impacts of Karenia mikimotoi blooms on the abalone haliotis discus hannai in fujian province. Mar. Environ. Sci. 35 (1), 27–34.
Lin J. N., Yan T., Zhang Q. C., Wang Y. F., Liu Q., Zhou M. J. (2016b). Effects of Karenia mikimotoi blooms on antioxidant enzymes in gastropod abalone, Haliotis discus hannai. Mar. Sci. 40, 17–22. doi: 10.11759/hykx20150330001
Lv S., Cen J., Wang J., Ou L. (2019). The research Status quo, hazard, and ecological mechanisms of Karenia mikimotoi red tide in coastal waters of China. Oceanol. Limnol. Sinica. 50, 487–484. doi: 10.11693/hyhz20181000255
Mandich M. (2018). Ranked effects of heavy metals on marine bivalves in laboratory mesocosms: a meta-analysis. Mar. Pollut. Bull. 131, 773–781. doi: 10.1016/j.marpolbul.2018.04.068
Matos C. R., Mendoza U., Diaz R., Moreira M., Belem A. L., Metzger E., et al. (2016). Nutrient regeneration susceptibility under contrasting sedimentary conditions from the Rio de Janeiro coast, Brazil. Mar. Pollut. Bull. 108 (1-2), 297–302. doi: 10.1016/j.marpolbul.2016.04.046
Mitchell S., Rodger H. (2007). Pathology of wild and cultured fish affected by a Karenia mikimotoi bloom in Ireland 2005. Bulletin-European Assoc. Fish Pathol. 27 (1), 39–42.
Murata A., Leong S. C. Y., Nagashima Y., Taguchi S. (2006). Nitrogen: phosphorus supply ratio may control the protein and total toxin of dinoflagellate Alexandrium tamarense. Toxicon 48 (6), 683–689. doi: 10.1016/j.toxicon.2006.08.004
Niu X., Xu S., Yang Q., Xu X., Zheng M., Li X., et al. (2021). Toxic effects of the dinoflagellate Karenia mikimotoi on zebrafish (Danio rerio) larval behavior. Harmful Algae 103, 101996. doi: 10.1016/j.hal.2021.101996
O’Boyle S., McDermott G., Silke J., Cusack C. (2016). Potential impact of an exceptional bloom of Karenia mikimotoi on dissolved oxygen levels in waters off Western Ireland. Harmful Algae 53, 77–85. doi: 10.1016/j.hal.2015.11.014
Pittura L., Avio C. G., Giuliani M. E., d'Errico G., Keiter S. H., Cormier B., et al. (2018). Microplastics as vehicles of environmental PAHs to marine organisms: combined chemical and physical hazards to the Mediterranean mussels, Mytilus galloprovincialis. Front. Mar. Sci. 5. doi: 10.3389/fmars.2018.00103
Samuel C. L., Norma Yolanda H. S., Salvador Emilio L. C., Pedro C. H., Felipe De Jesus A. V., Maria Teresa S. (2019). Survival and respiration of green abalone (Haliotis fulgens) facing very short-term marine environmental extremes. Mar. Freshw. Behav. Physiol. 52 (1), 1–15. doi: 10.1080/10236244.2019.1607734
Schmidtko S., Stramma L., Visbeck M. (2017). Decline in global oceanic oxygen content during the past five decades. Nature 542 (7641), 335–339. doi: 10.1038/nature21399
Shen Y. W. (2018). Effects of hypoxia stress on survival, immune physiology of abalone (Haliotis discus hannai and its hybrid with h. fulgens) and primary study on molecular basis (Dissertation, Xiamen:Xiamen University).
Shen Y., Huang Z., Liu G., Ke C., You W. (2019). Hemolymph and transcriptome analysis to understand innate immune responses to hypoxia in pacific abalone. Comp. Biochem. Physiol. Part D: Genomics Proteomics 30, 102–112. doi: 10.1016/j.cbd.2019.02.001
Tang Y., Rong J., Guan X., Zha S., Shi W., Han Y., et al. (2020). Immunotoxicity of microplastics and two persistent organic pollutants alone or in combination to a bivalve species. Environ. Pollut. 258, 113845. doi: 10.1016/j.envpol.2019.113845
Unkiewicz-Winiarczyk A., Gromysz-Kałkowska K. (2012). Effect of temperature on toxicity of deltamethrin and oxygen consumption by Porcellio scaber latr (Isopoda). Bull. Environ. Contam. Toxicol. 89 (5), 960–965. doi: 10.1007/s00128-012-0814-5
Vaquer-Sunyer R., Duarte C. M. (2008). Thresholds of hypoxia for marine biodiversity. Proc. Natl. Acad. Sci. 105 (40), 15452–15457. doi: 10.1073/pnas.0803833105
Wang D. Z., Hsieh D. P. (2002). Effects of nitrate and phosphate on growth and C2 toxin productivity of Alexandrium tamarense CI01 in culture. Mar. pollut. Bull. 45 (1-12), 286–289. doi: 10.1016/S0025-326X(02)00183-2
Wu F., Kong H., Shang Y., Zhou Z., Gul Y., Liu Q., et al. (2017). Histopathological alterations in triangle sail mussel (Hyriopsis cumingii) exposed to toxic cyanobacteria (Microcystis aeruginosa) under hypoxia. Aquaculture 467, 182–189. doi: 10.1016/j.aquaculture.2016.05.026
Wu Z., You F., Wen A., Ma D., Zhang P. (2016). Physiological and morphological effects of severe hypoxia, hypoxia and hyperoxia in juvenile turbot (Scophthalmus maximus l.). Aquacult. Res. 47 (1), 219–227. doi: 10.1111/are.12483
Yan T., Li X. D., Tan Z. J., Yu R. C., Zou J. Z. (2022). Toxic effects, mechanisms, and ecological impacts of harmful algal blooms in China. Harmful Algae 111, 102148. doi: 10.1016/j.hal.2021.102148
Yang B., Gao X., Zhao J., Liu Y., Xie L., Lv X., et al. (2021). Potential linkage between sedimentary oxygen consumption and benthic flux of biogenic elements in a coastal scallop farming area, north yellow Sea. Chemosphere 273, 129641. doi: 10.1016/j.chemosphere.2021.129641
Zhang P., Song X., Zhang Y., Zhu J., Shen H., Yu Z. (2022). Assessing the effect of modified clay on the toxicity of Karenia mikimotoi using marine medaka (Oryzias melastigma) as a model organism. Toxics 10 (3), 1–19. doi: 10.3390/jmse9080822
Zhang X., Wang X., Yan B. (2021). Single and combined effects of phenanthrene and polystyrene microplastics on oxidative stress of the clam (Mactra veneriformis). Sci. Total Environ. 771, 144728. doi: 10.1016/j.scitotenv.2020.144728
Keywords: hypoxia, Karenia mikimotoi, Haliotis discus hannai, oxidative stress, synergistic effects
Citation: Zhang Y, Song X and Zhang P (2023) Combined effects of toxic Karenia mikimotoi and hypoxia on the juvenile abalone Haliotis discus hannai. Front. Mar. Sci. 10:1029512. doi: 10.3389/fmars.2023.1029512
Received: 27 August 2022; Accepted: 22 May 2023;
Published: 12 June 2023.
Edited by:
Gang Li, Chinese Academy of Sciences (CAS), ChinaCopyright © 2023 Zhang, Song and Zhang. This is an open-access article distributed under the terms of the Creative Commons Attribution License (CC BY). The use, distribution or reproduction in other forums is permitted, provided the original author(s) and the copyright owner(s) are credited and that the original publication in this journal is cited, in accordance with accepted academic practice. No use, distribution or reproduction is permitted which does not comply with these terms.
*Correspondence: Xiuxian Song, c29uZ3h4QHFkaW8uYWMuY24=