- 1Key Laboratory for Coastal Marine Eco-Environment Process and Carbon Sink of Hainan province/Yazhou Bay Innovation Institute/Key Laboratory of Utilization and Conservation for Tropical Marine Bioresources of Ministry of Education/Modern Marine Ranching Engineering Research Center of Hainan, Hainan Tropical Ocean University, Sanya, China
- 2Marine College, Shandong University, Weihai, Shandong, China
This study quantified the absorption ability of the seagrass Zostera japonica and the macroalgae Ulva pertusa for dissolved inorganic nitrogen (DIN) (ammonium and nitrate) and dissolved organic nitrogen (DON) (urea and glycine) under different light conditions. The plants were cultured in filtered seawater (31‰, 25°C) for 2 weeks under three light levels. Macroalgae and the above- and belowground parts of seagrasses were separately placed into four different manmade seawater solutions with DIN (ammonium and nitrate) and DON (urea and glycine) stable isotopes for 1 h. The results showed that macroalgae had higher absorption rates for ammonium and nitrate after higher light (14.67 ± 2.50 and 1.29 ± 0.16 mg−1 dry weight (DW) h−1) than after lower light (4.52 ± 0.95 and 0.18 ± 0.12 mg−1 DW h−1) treatments. Compared to the belowground seagrass portions that had previously been grown in high and low light conditions, the seagrass leaves assimilated ammonium more quickly. Z. japonica preferred glycine to nitrate and urea after the high- and low-light treatments; that is, the absorption rates of the belowground seagrass parts for glycine were 14.71 ± 1.85 and 6.38 ± 0.52 mg−1 DW h−1 after the high- and low-light treatments, respectively, which were higher than those of ammonium, nitrate, and urea. The absorption rates of algae were lower than those for ammonium previously grown under medium- and low-light treatments. These results indicate that light reduction can impact the assimilation of DIN by Z. japonica and U. pertusa, and both have the ability to directly assimilate DON. This study provides information that could help reduce the negative effects of eutrophication on macroalgae and seagrasses in order to protect seagrass meadows.
1. Introduction
Seagrasses provide important ecological services, including altering nutrient cycling; producing organic carbon; enhancing biodiversity and food sources; supporting critical habitats for economic animals such as nereids, sipunculids, shellfish, shrimps, crabs, and fish; and stabilizing sediments in coastal areas (Costanza et al., 1997; Hemminga and Duarte, 2000; Beck et al., 2001; Orth et al., 2006; Huang et al., 2017). In recent years, land-derived nitrogen loading, largely from anthropogenic origin, has increased the eutrophication in coastal areas, resulting in a decline in seagrass meadows (Valiela et al., 1992; Duarte, 1995; Orth et al., 2006). More than half of the seagrass areas are reduced when land-derived nitrogen loads exceed 100 kg N ha−1 year−1 (Valiela and Cole, 2002). Seagrasses serve as one of the sensitive indicators of nutrient pollution (Bricker et al., 2003). The nutrient concentrations in the environment may alter the competitive advantage of seagrasses and macroalgae. Seagrasses can absorb nutrients from seawater and sediments in oligotrophic environments, although they have lower nutrient absorption rates than macroalgae (Harvens et al., 2001; Han and Liu, 2014). Eutrophication may result in fast-growing macroalgal blooms (Duarte, 1995; Harvens et al., 2001), which often aggravate the decline of seagrasses in temperate estuaries (Valiela et al., 1997; Hauxwell et al., 2001; McGlathery, 2001; Burkholder et al., 2007) by promoting light reduction and increasing nutrient turnover in ecosystems, thereby altering the absorption ability of seagrasses for nitrogen (Han and Liu, 2014 and references therein; Han et al., 2016).
Nutrients are some of the major environmental factors that control the primary production of seagrasses (Harvens et al., 2001; Leoni et al., 2008). Sea nitrogen is composed of dissolved inorganic nitrogen (DIN), dissolved organic nitrogen (DON), and particulate organic nitrogen (PON) (Gruber, 2004; Zhang et al., 2021). Wastewater from aquaculture releases some inorganic and organic nutrients from uneaten feed and from feces of farmed animals (Holmer et al., 2008; Herbeck et al., 2013). DIN, such as ammonium and nitrate, is considered an important nitrogen source for seagrass and macroalgal assimilation (Valiela et al., 1997; Lee and Dunton, 1999; van Alstyne, 2008; Fan et al., 2014; Alexandre et al., 2015). Some seagrass species, such as Zostera noltii, can absorb more nitrate when ammonium is absent (Alexandre et al., 2011). Ammonium can be toxic to seagrasses because its accumulation can increase protein breakdown (van Katwijk et al., 1997). DON constitutes a large part of the total dissolved nitrogen pool in coastal areas (Tyler et al., 2005; Wang, 2015 and references therein). Urea and dissolved free amino acids are important nitrogen sources for autotrophic organisms (Bronk, 2002). The hydrolysis of seagrass leaf litter drives the rapid release of DON during the early phase of decomposition (Wang et al., 2014; Delgado et al., 2017; Prasad et al., 2019). To date, there have been few studies on the DON absorption of seagrasses (Vonk et al., 2008; van England et al., 2011; Alexandre et al., 2015). Some seagrasses (e.g., Cymodocea nodosa and Z. noltii) can absorb nitrogen from small organic substrates (van England et al., 2011). Alexandre et al. (2015) found that DON was a complementary nitrogen source to DIN, although Zostera marina preferred DON to nitrate. The macroalgal uptake of DIN in coastal waters is also well known (Valiela et al., 1997; van Alstyne, 2008; Fan et al., 2014). The macroalgal absorption of DON compounds is still not as well understood as that of DIN (Tyler et al., 2005; Xu, 2020; Zhang et al., 2021b). Phytoplankton and detritus supported by DIN are defined as PON (Zhang et al., 2021a), which could be difficult for seagrasses and macroalgae to directly absorb.
Light reduction from eutrophication and extreme climatic events such as hurricanes and tsunamis, resulting in pulsed turbidity, has been considered as the primary factor that leads to seagrass decline (Cambridge et al., 1986; Walker and McComb, 1992; Preen and Marsh, 1995). Light reduction may increase the mortality of seagrasses and decrease their growth and coverage (Preen and Marsh, 1995; Collier et al., 2007; Collier et al., 2011) by contracting respiratory and growth requirements with a combination of photosynthetic carbon fixation and reallocation of reserves (Ralph et al., 2007). Thin macroalgae (e.g., Ulva sp.) have lower light requirements than seagrasses; therefore, macroalgae can use more incidental light compared to seagrasses (Duarte, 1995). The growth superiority of the two communities may depend on irradiance (Moore and Wetezl, 2000), nutrient quantity (Lapointe et al., 1994) and type.
Limited light may have negative effects on the nitrogen absorption of seagrasses (Touchette et al., 2003). Because plants may have a relatively fixed cell quota for proteins, lipids, and carbohydrates (Hedges et al., 2002), they tend to absorb different elements with relatively fixed ratios (Gruber, 2004). Energy is consumed when plants assimilate nitrogen (Touchette et al., 2003). The metabolism of nitrogen in plants is strongly linked to the photosynthetic fixation of carbon (Turpin, 1991 and references therein) because nitrogen and carbon are needed to build living organic tissues (Griffiths et al., 2020). Nitrogen assimilation requires carbon in the respiratory pathway (Leoni et al., 2008). The synthesis of amino acids by seagrasses requires more carbon in comparison with their carbon fixation capacity under conditions of nitrogen enrichment (Turpin, 1991 and references therein; Brun et al., 2002), which will result in a lower carbohydrate content, leading to an internal carbon limit (Touchette et al., 2003; Inverse et al., 2004). Macroalgal blooms resulting from eutrophication may lead to light reduction and may impact the nitrogen cycle in seagrass ecosystems (Han and Liu, 2014; Moreira-Saporiti et al., 2021), thus altering the ability of seagrasses to absorb inorganic and organic forms of nitrogen.
Zostera japonica, one of the dominant seagrass species in the Shandong coast of China, such as Swan Lake, has shown a decreasing trend, although it invaded and expanded along the Pacific Northwest coast (Ruesink et al., 2010; Han et al., 2017). The high nutrient and organic matter loads originating from benthos aquaculture and wastewater discharge could have contributed to the decline of Z. japonica in Shandong coast (Zhang et al., 2014; Han et al., 2017). In some areas along the Shandong coast, macroalgal mats (e. g., Ulva pertusa) have replaced the seagrass meadows (Zhang et al., 2014). Moreover, macroalgal mats cover the seagrass meadows during extended algal blooms every summer from June to July in Swan Lake, Shandong (Han et al., 2016). Until now, there have only been a few studies on how macroalgal blooms induce light attenuation and how they impact the capability of seagrasses and macroalgae to absorb DIN and DON.
The overall aim of this study was to compare the ability of seagrasses and green algae to absorb DIN and DON after light stress. Our hypotheses were as follows: firstly, plants require different amounts of energy for the two nitrogen forms (DIN and DON), and the nitrogen absorption ability of plants is lower because of the small amount of energy left for assimilation at lower light levels. Secondly, the light availability and the amount of energy required for nutrient uptake differ between seagrass and macroalgal species. Our results could provide information for environment managers regarding the control of nutrient inputs by intensive human activities and the reduction of the negative effects of macroalgae when macroalgal blooms result in more DIN and DON released into coastal eutrophication ecosystems in early low-light stress.
2. Materials and methods
2.1. Experimental design
Z. japonica and U. pertusa were collected from Swan Lake, whichis located in Rongcheng City, Shandong Province, China (37.3382° N–37.3588° N, 122.5551° E–122.5793° E), and were exposed for 2 weeks to three light levels: high light (HL 160 mol photons m−2 s-1), medium light (ML, 40% HL), and low light (LL, 10% HL). The seagrasses and macroalgae were collected in June when the daylight is longer than the dark, so light was set up with a photoperiod of 18-h light and 6-h dark. Light intensity was measured using an underwater fluorometer (DIVING-PAM, WALZ company, Germany). Round macroalgae (wet weight, 2 g) were placed in PVC cylinders with muddy sand (diameter, 11 cm; height, 10 cm). On top of the cylinders were nets with large holes to maintain sufficient light and to keep the plants inside. Z. japonica propagules (one apical shoot plus the first lateral shoot, and respective internodes; wet biomass, 11.35 ± 0.52) were buried in the same PVC cylinders as mentioned above. The cylinders were filled with muddy sand. The depth of the sediment cover of the propagules was 1.5 cm. A total of 75 macroalgae and seagrasses were cultivated. There were 25 macroalgal replicates and 25 seagrass replicates for each light treatment in three different tanks. The PVC cylinders were submerged in aerated and filtered seawater (31‰). The seawater flowed slowly and was changed once every 2 days. The temperature was maintained at 25° using constant temperature system in the climate laboratory. The temperature and salinity of the seawater were measured using a YSI 30 portable meter (YSI, Yellow Springs, OH, USA).
2.2. Stable nitrogen isotope treatments and plant measurements
After 2 weeks, the seagrasses and algae were separately divided into five groups for each light treatment, with five replicates for each group. In one group, the dry biomass of the macroalgae was weighed. The leaf length, width, rhizome node diameter, node length, and root length of seagrasses were measured, and the above- and belowground dry biomass was weighed. The total dry biomass was also calculated. Finally, the N content and δ15N‰ of the macroalgae and the aboveground (leaves) and belowground (roots with rhizomes) parts of seagrasses were measured using IRMS (MAT253; Thermo Fisher, Waltham, MA, USA) (n = 5).
Four different artificial seawater solutions with DIN and DON stable isotopes (Sigma-Aldrich, St. Louis, MO, USA) were prepared. The DIN solution contained ammonium (ammonium chloride, 10 μmol/L) and nitrate (sodium nitrate, 10 μmol/L), while the DON solution contained urea (10 μmol/L) and glycine (1 μmol/L). The glycine concentration is lower than that of DIN and urea in natural environments; therefore, the concentration of the glycine solution was lower than that of ammonium, nitrate, and urea. In the other four plant groups, the macroalgae and the above- and belowground parts of seagrasses were separately placed in the above-mentioned solutions. Each plant tissue was cultured in a container with 1 L stable nitrogen isotope solution, for a total of 180 treatments [three light treatments × four nitrogen solutions × three plant tissues (macroalgae and aboveground and belowground parts of seagrasses) × five replicates]. The seagrasses and macroalgae were incubated for 2–3 h in stable nitrogen isotope solutions in order to study their absorption ability (van England et al., 2011). Our preliminary experiments indicated that 1 h was sufficient for the absorption of DIN and DON stable isotopes by Z. japonica and U. pertusa. Therefore, in this study, 1 h was taken as the absorption time for plant tissues (macroalgae, aboveground parts and belowground parts of seagrasses) to absorb stable nitrogen isotopes. The temperature was maintained at 25°C. Lastly, the δ15N‰ values of macroalgae and the above- and belowground parts of seagrasses were measured using IRMS (MAT253; Thermo Fisher) (n = 5).
2.3. Data calculation
δ15N‰, which can be used to compare the amount of nitrogen absorbed by plants or plant tissues, was calculated as follows:
In Equation 1, Rstandard and Rsample denote the 15N/14N of the standard and the sample, respectively. Nitrogen in air was used as the analysis standard for stable nitrogen isotopes.
Isotope excess (Esample) was calculated as the difference between the isotope fraction in the sample (Fsample) and the natural abundance (i.e., initial isotope fraction, Fnat) (van England et al., 2011), as follows:
The specific uptake rate of 15N, Vsample [in micromoles 15N per milligram dry weight (DW) per hour], was calculated using the following equation:
where POM is the value of the stable nitrogen isotope in the plant tissue (in micromoles 15N) (van England et al., 2011). Correction of varying substrate concentrations was accomplished by dividing Vsample by the substrate concentration (nitrogen added, Nadded) and multiplying by 100 to convert to % (mg DW)−1 h−1.
%Vsample can be used to compare the nitrogen uptake rates of the different plants or plant tissues.
2.4. Statistical analysis
For the effects of light treatments on macroalgal dry biomass, seagrass leaf length, leaf width, rhizome node diameter, node and root lengths, total dry biomass, and above- and belowground dry biomass, the δ15N values of plants were analyzed using one-way ANOVA and post-hoc tests. The light and nitrogen effects on the %Vsample of algae and the above- and belowground components of seagrasses were separately analyzed using two-way ANOVA and post-hoc tests. Differences in the %Vsample values among groups (light × nitrogen × plant tissues) were analyzed using three-way ANOVA and post-hoc tests. When ANOVA was significant (p < 0.05), Tukey’s test was applied to determine which treatments were significantly different.
3. Results
3.1. Physiological and morphological parameters of algae and seagrasses
The N% and δ15N‰ of algae after light treatment were not significantly different (Table 1). The dry biomass of U. pertusa after HL treatment was significantly higher than that after ML and LL treatments (p < 0.01) (Table 1). The highest (0.32 ± 0.05 g) and the lowest (0.25 ± 0.05 g) values were recorded for the HL and ML treatments, respectively (Table 1).
The total dry biomass of Z. japonica in the HL treatment was significantly higher than that in the lower light treatments (p < 0.05) (Table 2). The highest and the lowest total dry biomass values in the HL and LL treatments were 2.14 ± 0.39 and 1.19 ± 0.23 mg, respectively (Table 2). The belowground dry biomass of Z. japonica was significantly different after the three light treatments (p < 0.05) (Table 2). The highest (1.04 ± 0.17 mg) and the lowest (0.55 ± 0.13 mg) belowground dry biomass values were found in the HL and LL treatments, respectively (Table 2).
The leaf length, width, and rhizome diameter of the seagrasses showed significant differences after the three light treatments (p < 0.05) (Table 2). The highest (6.17 ± 0.81 mg) and the lowest (3.36 ± 0.69 mg) values for seagrass leaf length were recorded in the HL and LL treatments, respectively (Table 2), while the highest (0.96 ± 0.14 mm) and the lowest (0.77 ± 0.09 mm) values for seagrass leaf width were found in the HL and ML treatments, respectively (Table 2). On the other hand, the highest (1.07 ± 0.12 mm) and the lowest (0.96 ± 0.07 mm) values for seagrass rhizome diameter were recorded in the HL and LL treatments, respectively (Table 2). The rhizome node and root lengths of seagrasses did not differ significantly among the three light treatments (Table 2).
The δ15N values of algae after the absorption of ammonium by plant tissues were significantly higher than those of the other three nutrients and natural groups after each light treatment (p < 0.01) (Figure 1). The highest (97.26 ± 30.84‰) and the lowest (35.65 ± 9.15‰) δ15N values of algae after ammonium absorption were found after HL and LL treatments, respectively (Figure 1).
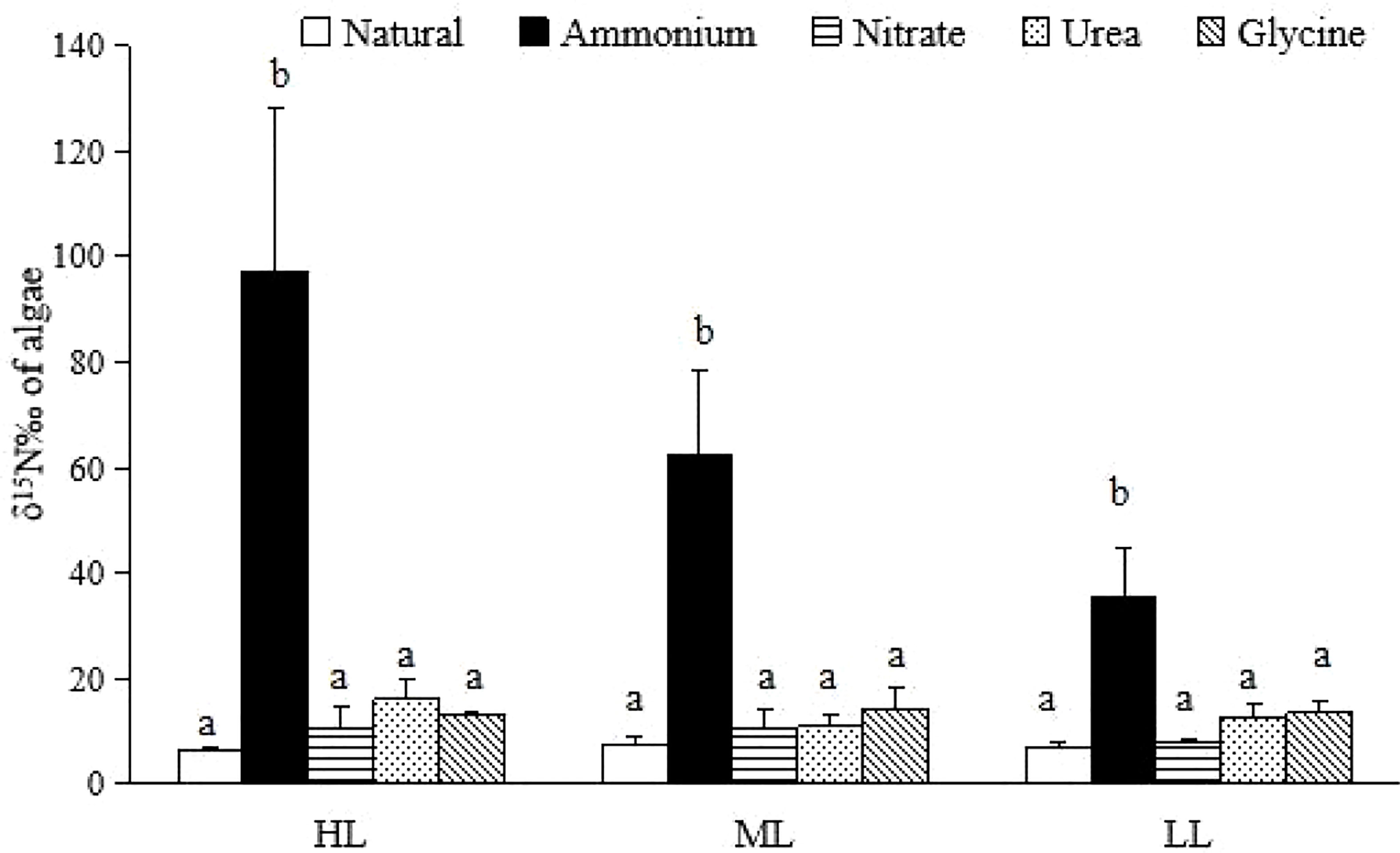
Figure 1 The δ15N value of U. pertusa. The different letters indicated significant differences in the same light treatment..
The δ15N values of the aboveground seagrass tissues after the absorption of ammonium were significantly higher than those of the natural groups and other three nutrients previously grown under each light treatment (p < 0.01) (Figure 2A). The δ15N values of the aboveground seagrass tissues after ammonium absorption were 62.99 ± 15.94‰, 91.95 ± 34.38‰, and 74.14 ± 22.49‰ after the HL, ML, and LL treatments, respectively (Figure 2A). The δ15N value of seagrass belowground tissues after nutrient absorption was significantly different after each light treatment (Figure 2B, p<0.05). The highest δ15N values of seagrass belowground tissues after ammonium absorption appeared after HL (67.90±10.49‰), ML (128.37±38.74‰) and LL (64.66±22.06‰) treatments (Figure 2B).
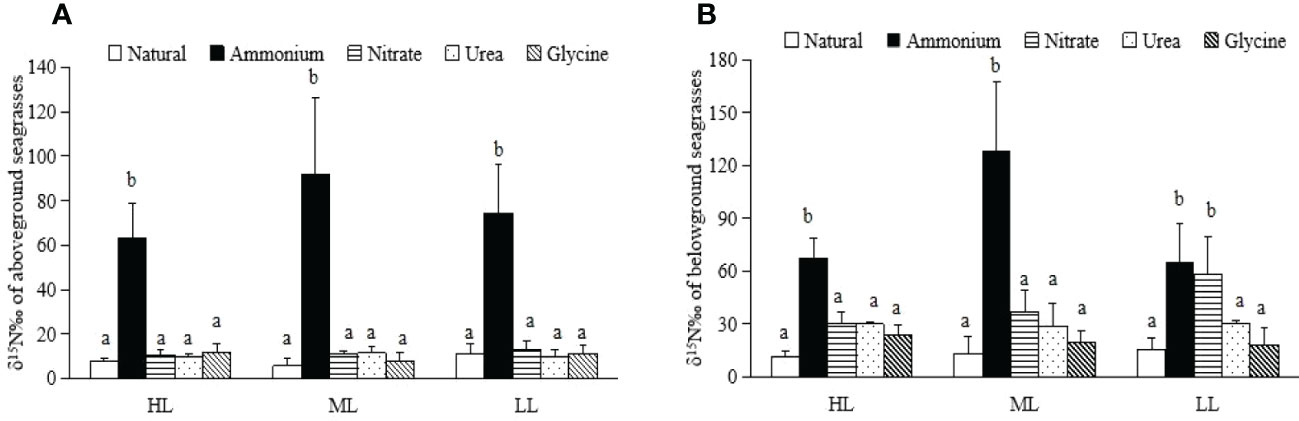
Figure 2 δ15N values in the aboveground and belowground tissues of Zostera japonica. (A) Aboveground seagrasses. (B) Belowground seagrasses.
3.2. %Vsample value of alagae and seagrasses
Both nitrogen and light significantly impacted the %Vsample values of algae (p < 0.001) (Figure 3A and Table 3). The highest absorption rates of algae for ammonium (14.67 ± 2.50 mg−1 DW h−1), nitrate (1.29 ± 0.16 mg−1 DW h−1), and urea (1.67 ± 0.65 mg−1 DW h−1) were found after HL treatment (Figure 3A). The lowest %Vsample values of algae for ammonium (4.52 ± 0.95 mg−1 DW h−1) and nitrate (0.18 ± 0.12 mg−1 DW h−1) occurred after LL treatment (Figure 3A). The lowest %Vsample value of algae for urea was 0.58 ± 0.41 mg−1 DW h−1, which was found after ML treatment (Figure 3A).
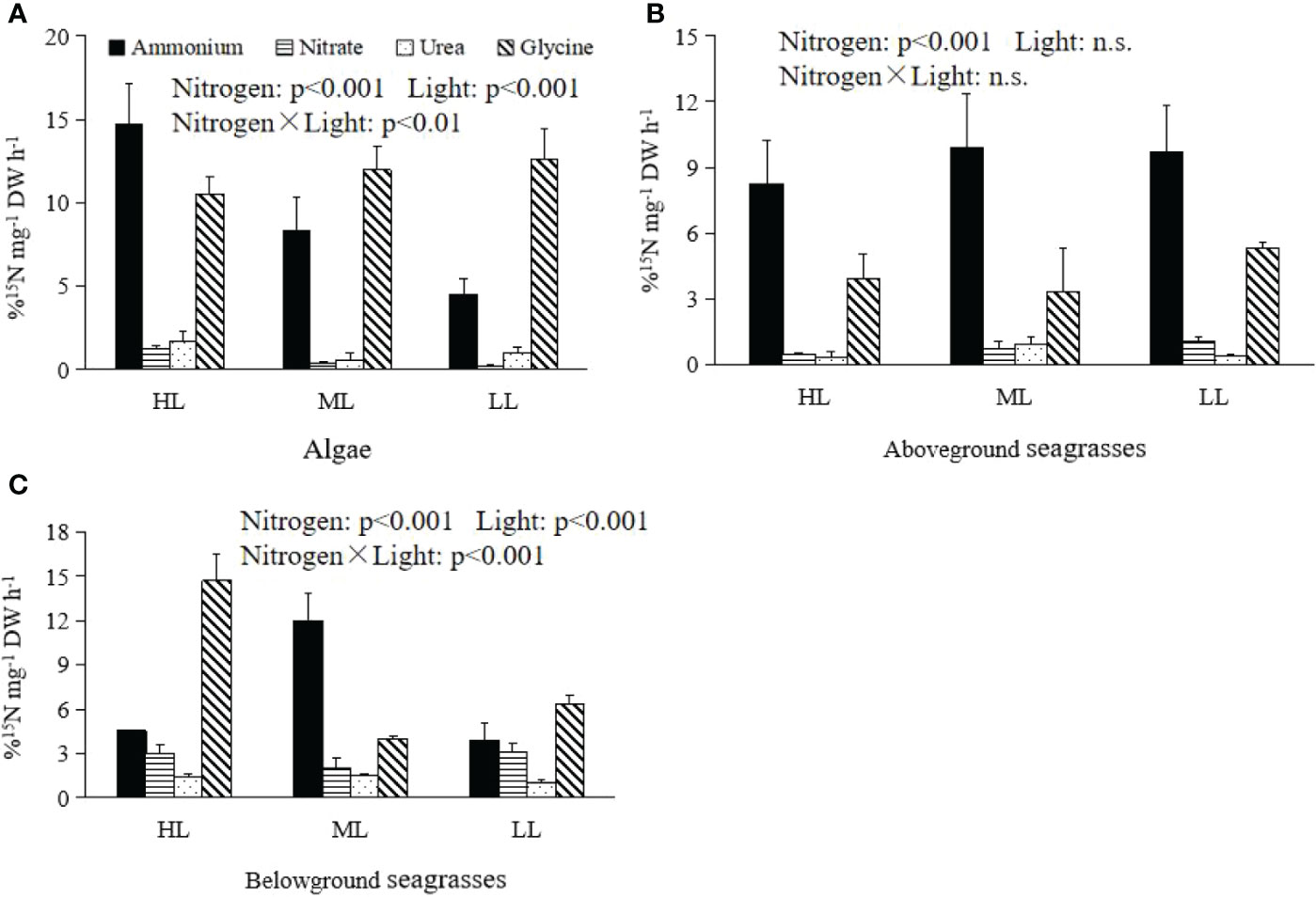
Figure 3 The N absorption rates between algae and the aboveground and belowground parts of seagrasses for the different N sources afterlight treatments. (A) Algae. (B) Aboveground seagrasses. (C) Belowground seagrasses.

Table 3 Analysis of variance of the effects of light and nitrogen on the %Vsample values of plant tissues.
Nitrogen had significant effects on the %Vsample values of the aboveground seagrass parts (p < 0.001) (Figure 3B and Table 3). The %Vsample values of the aboveground seagrass parts for ammonium were higher than those for glycine after all light treatments (Figure 3B). The highest ammonium absorption rates of the aboveground seagrasses were 8.27 ± 1.97, 9.93 ± 2.42, and 9.70 ± 2.12 mg−1 DW h−1 after HL, ML, and LL treatments, respectively (Figure 3B). For glycine, the highest absorption rates of the aboveground seagrasses were 3.92 ± 1.11, 3.35 ± 1.99, and 5.32 ± 0.27 mg−1 DW h−1 after HL, ML, and LL treatments, respectively (Figure 3B).
Light and nitrogen significantly impacted the %Vsample values of the belowground seagrass parts (p < 0.001) (Figure 3C and Table 3). After HL, ML, and LL treatments, the %Vsample values of the belowground seagrass parts for ammonium were 4.56 ± 0.04, 11.97 ± 1.89, and 3.87 ± 1.16 mg−1 DW h−1, respectively, while those for glycine were 14.71 ± 1.85, 3.98 ± 0.20, and 6.38 ± 0.52 mg−1 DW h−1, respectively (Figure 3C).
Nitrogen also altered the %Vsample values of algae and the above- and belowground seagrass parts under different light pressures (p < 0.001) (Table 4). The %Vsample values of algae for ammonium were higher than those of seagrass leaves after HL treatment, but were lower than those of seagrass leaves after LL treatments (Figure 3). For ammonium, the %Vsample values of the aboveground seagrass parts were higher than those of the belowground parts after HL and LL treatments (Figure 3). For nitrite, the %Vsample values of the belowground seagrass parts were higher than those of the aboveground seagrass parts and algae after all light treatments (Figure 3). The %Vsample values of algae for nitrate after LL treatments were lower than those of the aboveground seagrass parts, while these values in the belowground seagrass parts for urea after all treatments were higher than those in the aboveground seagrass parts (Figure 3). For glycine, the %Vsample values of algae were higher than those of seagrass leaves after all light treatments, while these values in the belowground seagrass parts were higher than those of the aboveground seagrass parts after HL treatment (Figure 3).
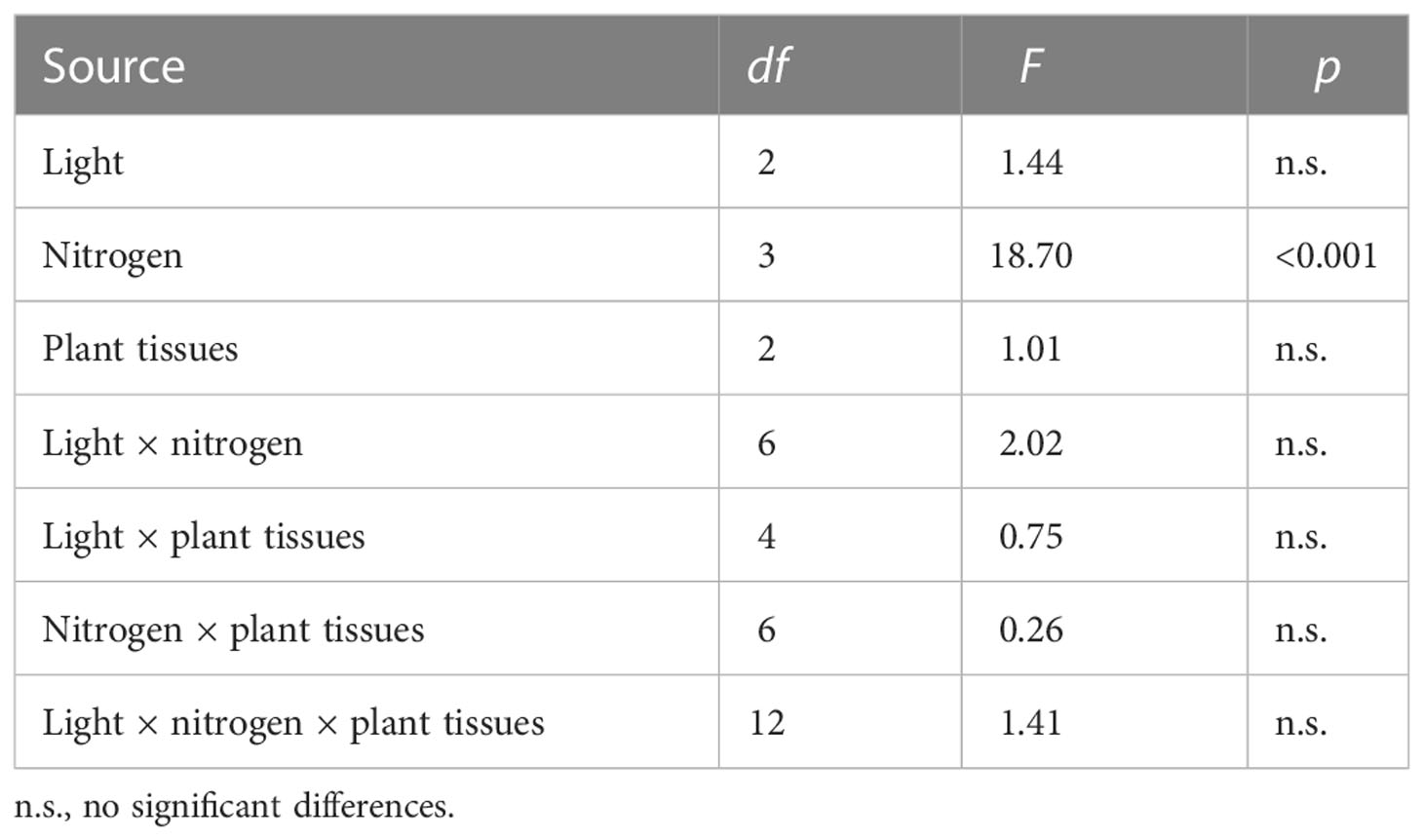
Table 4 Analysis of variance of the three-factor model with the %Vsample of plants as the response variable and light, nitrogen, and plant tissues (algae and the aboveground and belowground seagrasses) and all possible interactions.
The three-way ANOVA (light × nitrogen × plant tissues) illustrated the significant effects of nitrogen type on the ability of plant tissues (algae and the above- and belowground parts of seagrasses) to absorb nitrogen and showed that light and the nitrogen type altered the absorption ability of algae and seagrasses for nitrogen (Table 4).
4. Discussion
This study showed that the dry weight of U. pertusa after HL treatment was significantly higher than that after lower light treatments (Table 1), which is similar to the results of van Alstyne (2008) and Schmid et al. (2021), who found that macroalgae showed a higher growth rate under higher light than under lower light conditions. Macroalgae have evolved physiological responses to light reduction, driving photosynthesis (Takahashi and Murata, 2008; Esteban et al., 2015), thereby impacting algal biomass. The physiological responses of algae to stress can increase amino acids or reduce sugars at the cellular level (Sharma et al., 2016). High light intensity increases the concentrations of β-carotene and zeaxanthin in marine macroalgae (Xie et al., 2020), resulting in an increase in storage lipids in some algae (Khotimchenko and Yakovleva, 2005). However, some macroalgae showed lower chlorophyll a (Chl-a) and pigment composition under higher light conditions (see, for example, Esteban et al., 2015; Schmid et al., 2021). Ulva fenestra exhibited higher concentrations of lipids and higher proportions of polyunsaturated fatty acids in low-light habitats than in moderate-light conditions (Hotimchenko, 2002). Ulva lactuca grown under high-light conditions had lower nitrogen, carbon, pigment, and dimethylsulfoniopropionate concentrations relative to algae in low light (van Alstyne, 2008). This could be due to the physiological responses and resource allocation of macroalgae to light reduction being species-specific.
Light availability is the main factor limiting the growth and distribution of seagrasses compared with all other factors (Dennison et al., 1993; Lee et al., 2007). Seagrasses show physiological and morphological responses to light reduction depending on localized environmental conditions and timescales (Bertelli and Unsworth, 2018; Kim et al., 2019; Zhang et al., 2020). The responses of seagrasses to light reduction also include biomass decrease (Holmer and Laursen, 2002) and growth reduction (Rruiz and Romero, 2001). The total dry biomass and belowground biomass of Z. japonica in the HL treatment were significantly higher than those in the lower light treatments in this study (Table 2), which is in accordance with other reports on seagrass habitats (Z. marina and C. nodosa: Silva et al., 2013; Collier et al., 2016; Z. muelleri: Griffiths et al., 2020).
Exposure to low light may lead to carbonhydrate reduction in seagrasses (Hasler-Sheetal et al., 2016; Kumar et al., 2017). Smaller species are less resistant to light reduction (Collier et al., 2016), and the responses of seagrasses to light reduction are species-specific (Collier et al., 2007; Manassa et al., 2017; Statton et al., 2018). For example, the low light requirements of Z. noltii, Posidonia oceanica, Thalassia testudinum, Halodule pinifolia, and Syringodium filiforme for survival were 2%, 10%, 14%, 14%, and 24% of surface irradiance (SI), respectively (Leoni et al., 2008 and references therein). Our results showed that the rhizome diameters of the seagrasses in the HL treatment were significantly higher than those in the lower treatments (Table 2). The lower rhizome diameters of seagrasses demonstrate that carbon can be transported from belowground to the aboveground parts of seagrasses under low-light conditions due to disruption of the carbon fixation and energy metabolism (Griffiths et al., 2020). In this study, the leaf length and width were longer and thicker, respectively, in the HL treatment than in the ML and LL treatments (Table 2). A smaller area of seagrass leaves further limits photosynthesis. Our experiments prevented seagrasses from receiving nutrients and carbon via adjacent plants, as a result of isolating plant cultivation. This may be different from natural conditions, which should be considered in the future.
Light reduction and nitrogen availability can affect the physiological or biochemical properties of seaweeds by altering their carbon and nitrogen contents (Cruz-Rivera and Hay, 2003; Buapet et al., 2008). Although van Alstyne (2008) found that light and nitrate always independently affect the algal response, the absorption of nutrients by some algae is related to light and carbon metabolism (Dortch, 1990). The nitrogen concentrations in void tissues are often correlated with the DIN concentrations of seawater (Fujita et al., 1989; Björnsäter and Wheeler, 1990; Teichberg et al., 2010; Fan et al., 2014). In this study, it was found that the δ15N values of the algal tissues after ammonium absorption were significantly higher than those of the other three nutrients and natural groups after each light treatment (Figure 1). The absorption rate of ammonium by algae is higher than that of nitrate because the uptake of ammonium into cells requires less energy than nitrate (Flynn, 1991).
During the macroalgal bloom and decay phases, Ulva sp. are an important source of DON in the aquatic environment (Tyler et l., 2001; Zhang et al., 2021). During the early period of macroalgal blooms, green macroalgae can take up DIN, thus lowering the DIN concentration in ambient seawater and effectively relieving eutrophication (Zhang and Wang, 2017). Subsequently, algae assimilate DIN into DON, continuously releasing DON into the ambient environment, or depositing it into sediments (Li et al., 2016). This explains the increase of the DON concentration in the culture environment, mainly because the DON produced by Ulva sp. was released into the ambient media (Sharp, 1977). Higher DON concentrations may increase the soluble sugar and protein contents of macroalgae (Xu, 2020). Tyler et al. (2005) found that Gracilaria vermiculophylla can assimilate urea and amino acids and that U. lactuca has higher uptake rates than G. vermiculophylla. The DON and ammonium from decomposed algae can be important nitrogen sources and take part in the nitrogen cycle in seagrass ecosystems, thereby further changing the biochemical cycle processes in the long term (Zhang et al., 2021b).
It is conceivable that the nitrogen uptake of seagrasses may change during or after macroalgal blooms. Macroalgal blooms lead to light limitations, which affect the functions of seagrass ecosystems, such as nutrient uptake. In this study, light reduction and the nitrogen type altered the absorption ability of seagrasses and algae for nitrogen (Figure 3). After light reduction, seagrass growth is limited, which may result in a reduction in the nutrient requirements for growth. The physiological responses of seagrasses to low irradiance include the increase in the nitrogen and amino acid contents in tissues (van Lent et al., 1995; Longstaff and Dennison, 1999; Leoni et al., 2008; McMahon et al., 2013). Nitrogen enrichment can promote the demand for energy and carbon skeletons from photosynthates to drive the assimilation of DIN (Inverse et al., 2004), affecting the productivity and survival of seagrasses, which may aggravate the deleterious effects of low light (Villazán et al., 2013). Our results showed that the aboveground parts of seagrasses had higher absorption ability for ammonium than for nitrate, urea, and glycine after all light treatments (Figure 3). Some seagrass species have increased affinity for ammonium over nitrate (Terrados and Williams, 1997; Lee and Dunton, 1999). The preferential uptake of ammonium by seagrasses may be due to the physiological demands associated with nitrate uptake (Nayar et al., 2018 and references therein). Furthermore, seagrass tissues require far less energy than nitrate to transform ammonium into organic nitrogen (Nayar et al., 2018). Sandoval-Gil et al. (2015) found that seagrass roots showed reduced capacity to absorb ammonium compared to leaves because of the very high availability of this nutrient in sediments. This is in agreement with our results, in which the absorption rates of ammonium by the aboveground parts of seagrasses were higher than those of the belowground parts after HL and LL treatments (Figure 3). Lee and Dunton (1999) found that ammonia is mostly absorbed by the rhizomes and roots of seagrasses. The belowground parts of seagrasses grow in sediments, where there is less oxygen concentration than in coastal waters; therefore, seagrass roots and rhizomes may adapt to environments with less oxygen through evolved physiological responses. The substratum type can influence the leaf versus root nutrient uptake, and fine-grained sediments generally support a higher absorption rate of seagrasses for ammonium (Bulthuis et al., 1992). We recommend further research involving the combined effects of sediment types on the absorption ability of seagrasses for organic and inorganic nitrogen nutrients to lower the negative effects of eutrophication on seagrass ecosystems through a long-term investigation.
Sandoval-Gil et al. (2019) found that nitrate is mainly absorbed by seagrass leaves. This is contrary to our results, in which the absorption rates of nitrate by the belowground seagrass parts were significantly higher than those of seagrass leaves and macroalgae after all light treatments (Figure 3). Nitrate enrichment in sediments can stimulate the growth of seagrasses (Peralta et al., 2003), while water column nitrate enrichment may lead to the die-off of seagrasses (Leoni et al., 2008). Zimmerman et al. (1987) reported that most nitrogen assimilation occurs in the roots; however, limited light conditions interfere with the nitrogen assimilation in the roots because the root–rhizome system is photosynthesis-dependent (Pregnall et al., 1987). The nitrogen uptake rates of seagrass roots depend on the belowground biomass of seagrasses (Kraemer et al., 1997; Leoni et al., 2008). Some seagrass species (e.g., Z. noltii) can absorb more nitrate in the absence of ammonium (Alexandre et al., 2011). The assimilation of seagrasses into nitrate requires more energy because it involves an active transport system (Lepoint et al., 2002), which may aggravate the potentially harmful effects of nitrate enrichment, such as toxicity and metabolic costs (Burholder et al., 1992; Sandoval-Gil et al., 2015). Our results demonstrate that limited light use did not alter the absorption ability of the above- and belowground parts of seagrasses for nitrogen, especially nitrate.
When the nitrogen requirement is lower than the uptake, the absorbed nutrients may be reserved in the plant tissues as amino acids or proteins (Udy et al., 1999; Invers et al., 2004). The carbon requirements for synthesizing amino acids can exceed the carbon fixation capacity under nitrogen enrichment conditions. The results of this study showed that the absorption ability of seagrasses for glycine was significantly higher than that for nitrate and urea after HL and LL treatments (Figure 3), which is in agreement with Alexandre et al. (2015), who found that Z. marina showed preferential uptake of DON over nitrate and that DON was a complementary nitrogen source compared to DIN. Seagrass tissues can limit the use of nitrogen once they reach substrate saturation (Touchette and Burkholder, 2000). The lower concentration of glycine than nitrate and urea may have enhanced the uptake ratio of seagrasses to glycine in this study. The belowground parts of seagrasses had higher absorption rates for urea than the aboveground parts after all light treatments (Figure 3). The urea in coastal waters resulting from agricultural fertilizers, coastal aquaculture, domestic sewage, and industrial waste can be deposited in sediments (Li et al., 2015), thereby providing an organic nitrogen source for the roots and rhizomes of seagrasses. Organic nitrogen (e. g., amino acid nitrogen) can be mineralized and directly assimilated by plants and microbes (Dong et al., 2021). For example, some dinoflagellates can absorb urea and nitrate (Wang, 2015). Vonk et al. (2008) found that seagrasses may prefer organic nitrogen at low ambient nitrogen concentrations; however, several studies have shown that seagrasses more preferred absorbing DIN (Burkholder et al., 1992; Lee and Dunton, 1999; Sandoval-Gil et al., 2015). The DON uptake of seagrasses may be more widespread than is traditionally recognized (van England et al., 2011). Microorganisms can facilitate the assimilation of DON by mineralizing amino acids in the seagrass Posidonia sinuosa, which may promote the growth and productivity of seagrasses (Tarquinio et al., 2018). However, the microbial catabolism of amino acids can lead to exuded ammonium, which is harmful to plant tissues at extremely high concentrations (van Katwijk et al., 1997). The mechanisms by which microorganisms affect the uptake of DON by seagrasses require further research.
Under lower light conditions, seagrasses may need more energy for the absorption of organic nitrogen in order to reduce the negative effects of light limitation (Collier et al., 2010). A reduced photosynthetic carbon fixation under low light conditions leads to less carbon being transferred from the leaves to seagrass roots and rhizomes. This may explain our results where the assimilation of glycine by Z. japonica roots and rhizomes after HL treatment was higher than that of the lower treatment (Figure 3D). Processes that minimize the utilization of reserved carbon by reducing the organic nitrogen assimilation within seagrass roots and rhizomes may contribute to shading tolerance. Our results indicate that Z. japonica quickly responded to short-term light reduction due to changes in organic nitrogen metabolism processes. The internal assimilation to organic nitrogen and the transfer mechanism to carbon of seagrasses should be considered in the future.
5. Conclusion
The eutrophication problem is complex because it may change the primary producers and environmental variables through ecosystem self-organization. Overall, this study provides evidence that shading alters the absorption ability of macroalgae and seagrasses for inorganic and organic nitrogen, although it is also necessary to deeply understand the difference between the limited, highly artificial study and the non-linear, highly interactive responses in the seagrass meadows. Light reduction may alter the competition status between seagrasses and macroalgae through a change in their inorganic and organic absorption strategies. This difference should be well considered in future coastal management and seagrass protection. Regular seagrass monitoring, coupled with long-term studies to assess the effects of macroalgal bloom progression and duration on seagrass ecosystems, is required. Additional tests of the thresholds of the different nitrogen sources and their effects under different environmental, social, and economic contexts are also required.
Data availability statement
The original contributions presented in the study are included in the article/supplementary material. Further inquiries can be directed to the corresponding author.
Author contributions
QH and CQ: Conceptualization. QH and YC: Methodology. QH, WZ, and YC: Formal analysis. QH, CQ, and FZ: Writing—original draft preparation. QH, MZ, YS, and CQ: Writing—review and editing. All authors contributed to the article and approved the submitted version.
Funding
This research was supported by the projects ofthe National Natural Science Foundation of China (41730529) andthe High-Level Talented Person Project of the Natural ScienceFoundation of Hainan Province (420RC657).
Acknowledgments
We thank Laura M. Soissons for her support during the experiment design.
Conflict of interest
The authors declare that the research was conducted in the absence of any commercial or financial relationships that could be construed as a potential conflict of interest.
Publisher’s note
All claims expressed in this article are solely those of the authors and do not necessarily represent those of their affiliated organizations, or those of the publisher, the editors and the reviewers. Any product that may be evaluated in this article, or claim that may be made by its manufacturer, is not guaranteed or endorsed by the publisher.
References
Alexandre A., Hill P. W., Jones D. L., Santos R. (2015). Dissolved organic nitrogen: A relevant, complementary source of nitrogen for the seagrass Zostera marina. Limnology Oceanography 60, 1477–1483. doi: 10.1002/lno.10084
Alexandre A., Silva J., Bouma T. J., Santos R. (2011). Inorganic nitrogen uptake kinetics and whole-plant nitrogen budget in the seagrass Zostera noltii. J. Exp. Mar. Biol. Ecology 401, 7–12. doi: 10.1016/j.jembe.2011.03.008
Beck M. W., Heck K. L. Jr., Able K. W., Childers D. L., Eggleston D. B., Gillanders B. M., et al. (2001). The identification, conservation, and management of estuarine and marine nurseries for fish and invertebrates. BioScience 51, 633–641. doi: 10.1641/0006-3568(2001)051[0633:TICAMO]2.0.CO;2
Bertelli C. M., Unsworth R. K. F. (2018). Light stress responses by the eelgrass, Zostera marina (L). Front. Environ. Science 6, 39. doi: 10.3389/fenvs.2018.00039
Björnsäter B. R., Wheeler P. A. (1990). Effect of nitrogen and phosphorus supply on growth and tissue composition of Ulva fenestrata and Enteromorpha intestinalis (Ulvales, chlorophyta). J. Phycology 26, 603–611. doi: 10.1111/j.0022-3646.1990.00603.x
Bricker S. B., Ferreira J. G., Simas T. (2003). An integrated methodology for assessment of estuarine trophic status. Ecol. Modelling 169, 39–60. doi: 10.1016/S0304-3800(03)00199-6
Bronk D. A. (2002). “Dynamics of organic nitrogen,” in Biogeochemistry of marine dissolved organic matter. Eds. Hansell D. A., Carlson C. A. (San Diego: Academic Press), 153–247.
Brun F. G., Hernández I., Vergara J. J., Peralta G., Pérez-Lloérnz J. L. (2002). Assessing the toxicity of ammonium pulses to the survival and growth of Zostera noltii. Mar. Ecol. Progess Series 225, 177–187. doi: 10.3354/meps225177
Buapet P., Hiranpan R., Ritchie R. J., Prathep A. (2008). Effect of nutrient inputs on growth, chalorophyll, and tissue nutrient concentration of Ulva reticulate from a tropical habitat. ScienceAsia 34, 245–252. doi: 10.2306/scienceasia1513-1874.2008.34.245
Bulthuis D. A., Axelrad D. M., Mickelson M. J. (1992). Growth of the seagrass Heterozostera tasmanica limited by nitrogen in port phillip bay, Australia. Mar. Ecol. Prog. Series 89, 269–275. doi: 10.3354/meps089269
Burkholder J. M., Noga E. J., Hobbs C. H., Glasgow Jr. H. B. (1992). New ‘phantom’ dinoflagellate is the causative agent of major estuarine fish kills. Nature 358, 407–410. doi: 10.1038/358407a0
Burkholder J. M., Tomasko D. A., Touchette B. W. (2007). Seagrasses and eutrophication. J. Exp. Mar. Biol. Ecology 350, 46–72. doi: 10.1016/j.jembe.2007.06.024
Cambridge M. I., Chiffings A. W., Brittain C., Moore L., McComb A. J. (1986). The loss of seagrass in Cockburn sound, Western australia. II. possible causes of seagrass decline. Aquat. Botany 24, 269–285. doi: 10.1016/0304-3770(86)90062-8
Collier C. J., Adams M. P., Langlois L., Waycott M., O’Brien K. R., Maxwell P. S., et al. (2016). Thresholds for morphological response to light reduction for four tropical seagrass species. Ecol. Indicators 67, 358–366. doi: 10.1016/j.ecolind.2016.02.050
Collier C. J., Lavery P. S., Masini R. J., Ralph P. J. (2007). Morphological, growth and meadow characteristics of the seagrass Posidonia sinuosa along a depth-reltated gradient of light availability. Mar. Ecol. Prog. Series 337, 103–115. doi: 10.3354/meps337103
Collier C. J., Prado P., Lavery P. S. (2010). Carbon and nitrogen translocation in response to shading of the seagrass Posidonia sinuosa. Aquat. Botany 93, 47–54. doi: 10.1016/j.aquabot.2010.03.003
Collier C. J., Uthicke S., Waycott M. (2011). Thermal tolerance of two seagrass species at contrasting light levels: implications for future distribution in the great barrier reef. Limnology Oceanography 56, 2200–2210. doi: 10.4319/lo.2011.56.6.2200
Costanza R., d’Arge R., de Groot R., Farber S., Grasso M., Hannon B., et al. (1997). The value of the world’s ecosystem services and natural capital. Nature 387, 253–260. doi: 10.1038/387253a0
Cruz-Rivera E., Hay M. (2003). Prey nutritional quality interacts with chemical defenses to affect consumer feeding and fitness. Ecol. Monographs 73, 483–506. doi: 10.1890/0012-9615(2003)073[0483:PNQIWC]2.0.CO;2
Delgado M., Carlos M., Cintra-Buenrostro E., Fierro-Cabo A. (2017). Decomposition and nitrogen dynamics of turtle grass (Thalassia testudinum) in a subtropical estuarine system. Wetlands Ecol. Management 25, 667–681. doi: 10.1007/s11273-017-9543-1
Dennison W. C., Orth R. J., Moore K. A., Stevenson J. C., Carter V., Kollar S., et al. (1993). Assessing water quality with submersed aquatic vegetation: habitat requirements as barometers of Chesapeake bay health. Bioscience 43, 86–94. doi: 10.2307/1311969
Dong S. H., Lv H. J., Zhou F., Zhang X. C., He H. B., Zhang X. D., et al. (2021). Variation of soil organic nitrogen fractions in maize field during growing season and its response to current year and long-term straw returning. Chin. J. Ecology 41, 73–80. doi: 10.13292/j.1000-4890.20220.1036
Dortch Q. (1990). The interaction between ammonium and nitrate uptake in phytoplankton. Mar. Ecol. Prog. Series 61, 183–201. doi: 10.3354/meps061183
Duarte C. M. (1995). Submerged aquatic vegetation in relation to different nutrient regimes. Ophelia 41, 87–112. doi: 10.1080/00785236.1995.10422039
Esteban R., Barrutia O., Artetxe U., Fernandez-Marin B., Hernandez A., Garcia-Plazaola J. I. (2015). Internal and external factors affecting photosynthetic pigment composition in plants: a meta-analytical approach. New Phytologist 206, 268–280. doi: 10.1111/nph.13186
Fan X., Xu D., Wang Y., Zhang X., Cao S., Mou S., et al. (2014). The effect of nutrient concentrations, nutrient ratios and temperature on photosynthesis and nutrient uptake by Ulva prolifera: implications for the explosion in green tides. J. Appl. Phycology 26, 537–544. doi: 10.1007/s10811-013-0054-z
Flynn K. J. (1991). Algal carbon-nitrogen metabolism: A biochemical basis for modeling the interaction between nitrate and ammonium uptake. J. Plankton Res. 13 (2), 373–387. doi: 10.1093/plankt/13.2.373
Fujita R., Wheeler P., Edwards R. L. (1989). Assessment of macroalgal nitrogen limitation in a seasonal upwelling region. Mar. Ecol. Prog. Series 53, 293–303. doi: 10.3354/meps053293
Griffiths L. L., Melvin S. D., Connolly R. M., Pearson R. M., Brown C. J. (2020). Metabolomic indicators for low-light stress in seagrass. Ecol. Indicators 114, 106316. doi: 10.1016/j.ecolind.2020.106316
Gruber G. (2004). “The dynamics of the marine nitrogen cycle and its influence on atmospheric CO2 variations,” in The ocean carbon cycle and climate. Eds. Follows M., Oguz T. (Amstertam: LOS Press), 97–148.
Han Q. Y., Liu D. Y. (2014). Macroalgae blooms and their effects on seagrass ecosystems. J. Ocean Univ. China 13, 791–798. doi: 10.1007/s11802-014-2471-2
Han Q. Y., Soissons L. M., Bouma T. J., van Katwijk M. M., Liu D. Y. (2016). Combined nutrient and macroalgae loads lead to response in seagrass indicator properties. Mar. pollut. Bulletin 106, 174–182. doi: 10.1016/j.marpolbul.2016.03.004
Han Q. Y., Soissons L. M., Liu D. Y., van Katwijk M. M., Bouma T. J. (2017). Individual and population indicators of Zostera japonica resond quickly to experimental addition of sediment-nutrient and organic matter. Mar. pollut. Bulletin 114, 201–209. doi: 10.1016/j.marpolbul.2016.08.084
Harvens K. E., Hauxwell J., Tyler A. C., Thomas S., McGlathery K. J., Cebrián J., et al. (2001). Complex interactions between autotrophs in shallow marine and freshwater ecosystems: implications for community responses to nutrient stress. Environ. Pollution 113, 95–107. doi: 10.1016/S0269-7491(00)00154-8
Hasler-SheEtal H., Castorani M. C. N., Glud R. N., Canfield D. E., Holmer M. (2016). Metabolomics reveals cryptic interactive effects of species interactions and environmental stress on nitrogen and sulfur metabolism in seagrass. Environ. Sci. Technology 50, 11602–11609. doi: 10.1021/acs.est.6b04647
Hauxwell J., Cebrián J., Furlong C., Valiela I. (2001). Macroalgal canopies contribute to eelgrass (Zostera marina) decline in temperate estuarine ecosystems. Ecology 82, 1007–1022. doi: 10.1890/0012-9658(2001)082[1007:MCCTEZ]2.0.CO;2
Hedges J. I., Baldock J., Gelinas Y., Lee C., Person M., Wakeham S. (2002). The biochemical and elemental compositions of marine plankton: A NMR perspective. Mar. Chem. 78, 47–63. doi: 10.1016/S0304-4203(02)00009-9
Hemminga M., Duarte C. M. (2000). Seagrass ecology (Cambridge, United Kingdom: Cambridge University Press).
Herbeck L. S., Unger D., Wu Y., Jennerjahn T. C. (2013). Effluent, nutrient and organic matter export from shrimp and fish ponds causing eutrophication in coastal and back-reef waters of NE hainan. Continental Shelf Res. 57, 92–104. doi: 10.1016/j.csr.2012.05.006
Holmer M., Argyrou M., Dalsgaard T., Danovaro R., Diaz-Almela E., Duatre C. M., et al. (2008). Effects of fish farm waste on Posidonia oceanica meadows: synthesis and provision of monitoring and management tools. Mar. pollut. Bulletin 56, 1618–1629. doi: 10.1016/j.marpolbul.2008.05.020
Holmer M., Laursen L. (2002). Effect of shading of Zostera marina (eelgrass) on sulfur cycling in sediments with contrasting organic matter and sulfide pools. J. Exp. Mar. Biol. Ecology 270, 25–37. doi: 10.1016/S0022-0981(02)00015-1
Hotimchenko S. (2002). Fatty acid composition of algae from habitats with varying amounts of illumination. Russian J. Mar. Biol. 28, 218–220. doi: 10.1023/A:1016861807103
Huang G. S., Li X. B., Tang Y. J., Liu J. L., Liu N., Ke X. R., et al. (2017). An experiment of the ecological breeding for aquatic economic animals in Sonneratia apetala artificial plantation. J. Guangdong Univ. Education 37 (3), 85–89. doi: 10.3969/j.issn.2095-3798.2017.03.013
Inverse O., Kraemer G. P., Pérez M., Romero J. (2004). Effect of nitrogen addition on nitrogen metabolism and carbon reserves in the temperate seagrass Posidonia oceanica. J. Exp. Mar. Biol. Ecology 303, 97–114. doi: 10.1016/j.jembe.2003.11.005
Khotimchenko S. V., Yakovleva I. M. (2005). Lipid composition of the red alga Tichocarpus crinitus exposed to different levels of photon irradiance. Phytochemistry 66, 73–79. doi: 10.1016/j.phytochem.2004.10.024
Kim M., Pernice M., Watson-Lazowski A., Guagliardo P., Kilburn M. R., Larkum A. W. D., et al. (2019). Effect of reduced irradiance on 13C uptake, gene expression and protein activity of the seagrass Zostera mulleri. Mar. Environ. Res. 149, 80–89. doi: 10.1016/j.marenvres.2019.06.004
Kraemer G. P., Mazzella L., Alberte R. S. (1997). Nitrogen assimilation and partitioning in the Mediterranean seagrass Posidonia oceanica. Mar. Ecology 18, 287–300. doi: 10.1111/j.1439-0485.1997.tb00435.x
Kumar M., Padula M. P., Davey P., Pernice M., Jiang Z., Sablok G., et al. (2017). Proteome analysis reveals extensive light stress-response reprogramming in the seagrass Zostera muelleri (Alismatales, zosteraceae) metabolism. Front. Plant Science 7, 2023. doi: 10.3389/fpls.2016.02023
Lapointe B. E., Tomasko D. A., Matzie W. R. (1994). Eutrophication and trophic state classification of seagrass communities in the Florida keys. Bull. Mar. Sci. 54, 696–717. doi: 10.1515/botm.1994.37.3.277
Lee K. S., Dunton K. H. (1999). Inorganic nitrogen acquisition in the seagrass Thalassia testudinum: development of a whole plant nitrogen budget. Limnology Oceanography 44, 1204–1215. doi: 10.4319/lo.1999.44.5.1204
Lee K., Park S. R., Kim Y. K. (2007). Effects of irradiance, temperature, and nutrients on growth dynamics of seagrasses: a review. J. Exp. Mar. Biol. Ecology 350, 144–175. doi: 10.1016/j.jembe.2007.06.016
Leoni V., Vela A., Pasqualini V., Pergent-Martini C., Pergent G. (2008). Effects of experimental modifications of light levels and nutrient concentrations on seagrass: a review. Aquat. Conservation: Mar. Freshw. Ecosystems 18, 202–220. doi: 10.1002/aqc.842
Lepoint G., Millet S., Dauby P., Gobert S., Bouquegneau J. M. (2002). Annual nitrogen budget of the seagrass Posidonia oceanica as determined by in situ uptake experiments. Mar. Ecol. Prog. Series 237, 87–96. doi: 10.3354/meps237087
Li Z. L., Shi X. Y., Zhang C. S. (2015). Distribution characteristics of urea and constitution of dissolved nitrogen in the bohai sea and huanghai sea in spring. Environ. Science 36, 3999–4004. doi: 10.13227/j.hjkx.2015.11.008
Li H., Zhang Y., Han X., Shi X., Rivkin R. B., Legendre L. (2016). Growth responses of Ulva prolifera to inorganic and organic nutrients: implications for macroalgal blooms in the southern yellow Sea. Sci. Rep. 6, 26498. doi: 10.1038/srep26498
Longstaff B. J., Dennison W. C. (1999). Seagrass survival during pulsed turbidity events: the effects of light deprivation on the seagrasses Halodule pinfolia and Halophila ovalis. Aquat. Botany 65, 105–121. doi: 10.1016/S0304-3770(99)00035-2
Manassa R. P., Smith T. M., Beardall J., Keough M. J., Cook P. L. M. (2017). Capacity of a temperate intertidal seagrass species to tolerate changing environmental conditions: significance of light and tidal exposure. Ecol. Indicators 81, 578–586. doi: 10.1016/j.ecolind.2017.04.056
McGlathery K. (2001). Macroalgal blooms contribute to the decline of seagrass in nutrient-enriched coastal water. J. Phycology 37, 453–456. doi: 10.1046/j.1529-8817.2001.037004453.x
McMahon K., Collier C., Lavery P. S. (2013). Identifying robust bioindicators of light stress in seagrasses: a meta-analysis. Ecol. Indicator 30, 7–15. doi: 10.1016/j.ecolind.2013.01.030
Moore K. A., Wetzel R. L. (2000). Seasonal variations in eelgrass (Zostera marina l.) response to nutrient enrichment and reduced light availability in experimental ecosystems. J. Exp. Mar. Biol. Ecology 244, 1–28. doi: 10.1016/S0022-0981(99)00135-5
Moreira-Saporiti A., Hoeijmakers D., Msuya F. E., Reuter H., Teichberg M. (2021). Seaweed farming pressure affects seagrass and benthic macroalgae dynamics in chwaka bay (Zanzibar, Tanzania). Regional Environ. Change 21, 1–12. doi: 10.1007/s10113-020-01742-2
Nayar S., Loo M. G. K., Tanner J. E., Longmore A. R., Jenkins G. P. (2018). Nitrogen acquisition and resource allocation strategies in temperate seagrass Zostera nigricaulis: uptake, assimilation and translocation processes. Sci. Rep. 8, 17151. doi: 10.1038/s41598-018-35549-3
Orth R. J., Carruthers T. J. B., Dennison W. C., Duarte C. M., Fourqurean J. W., Heck K. L., et al. (2006). A global crisis for seagrass ecosystems. BioScience 56, 987–996. doi: 10.1641/0006-3568(2006)56[987:AGCFSE]2.0.CO;2
Peralta G., Bouma T. J., van Soelen J., Pérez-Lloréns J. L., Hernández I. (2003). On the use of sediment fertilization for seagrass restoration: a mesocosm study on Zostera marina l. Aquat. Botany 75, 95–110. doi: 10.1016/S0304-3770(02)00168-7
Prasad M. H. K., Ganguly D., Paneerselvam A., Ramesh R., Purvaja R. (2019). Seagrass litter decomposition: an additional nutrient source to shallow coastal waters. Environ. Monit. Assessment 191, 5. doi: 10.1007/s10661-018-7127-z
Preen A. R., Marsh H. (1995). Responses of dugongs to large-scale loss of seagrass from hervey bay, Queensland, Australia. Wildlife Res. 22, 507–519. doi: 10.1071/WR9950507
Pregnall A. M., Smith R. D., Alberte R. S. (1987). Glutamine synthetase activity and free amino acid pools of eelgrass (Zostera marina l.) roots. J. Exp. Mar. Biol. Ecology 106, 211–228. doi: 10.1016/0022-0981(87)90094-3
Ralph P. J., Durako M. J., Enriquez S., Collier C. J., Doblin M. A. (2007). Impact of light limitation on seagrasses. J. Exp. Mar. Biol. Ecology 350, 176–193. doi: 10.1016/j.jembe.2007.06.017
Rruiz J. M., Romero J. (2001). Effects of in situ experimental shading on the mediterranean seagrass Posidonia oceanica. Mar. Ecol. Prog. Series 215, 107–120. doi: 10.3354/meps215107
Ruesink J. L., Hong J. S., Wisehart L., Hacker S. D., Dumbauld B. R., Hessing-Hessing-Lewis M., et al. (2010). Congener comparison of native (Zostera marina) and introduced (Z. japonica) eelgrass at multiple scales within a pacific Northwest estuary. Biol. Invasions 12, 1773–1789. doi: 10.1007/s10530-009-9588-z
Sandoval-Gil J. M., Ávila-López M. C., Camacho-Ibar V. F., Hernández-López J., Zertuche-González J. A., Cabello-Pasini A. (2019). Regulation of nitrate uptake by the seagrass Zostera marina during upwelling. Estuaries Coasts 42, 731–742. doi: 10.1007/s12237-019-00523-3
Sandoval-Gil J. M., Camacho-Ibar V. F., Ávila-López M. C., Hernández-López J., Zertuche-González J. A., Cabello-Pasini A. (2015). Dissolved inorganic nitrogen uptake kinetics and δ15N of Zostera marina l. (eelgrass) in a coastal lagoon with oyster aquaculture and upwelling influence. J. Exp. Mar. Biol. Ecology 472, 1–13. doi: 10.1016/j.jembe.2015.06.018
Schmid M., Guihéneuf F., Nitschke U., Stengel D. B. (2021). Acclimation potential and biochemical response of four temperate macroalgae to light and future seasonal temperature scenarios. Algal Res. 54, 102190. doi: 10.1016/j.algal.2021.102190
Sharma S. K., Singh G., Kumar H., Kataria R. (2016). Effect of temperature on viscometric properties of aliphatic amino acids glycine/L-alanine/L-valine in aqueous solutions of tetraethylammonium iodide. J. Mol. Liquids 216, 516–525. doi: 10.1016/j.molliq.2016.01.053
Sharp J. H. (1977). Excretion of organic matter by marine phytoplankton: do healthy cells do it? Limnology Oceanography 22, 381–399. doi: 10.4319/lo.1977.22.3.0381
Silva J., Barrote I., Costa M. M., Albano S., Santos R. (2013). Physiological responses of zostera marina and cymodocea nodosa to light-limitation stress. PloS One 8, e81058. doi: 10.1371/journal.pone.0081058
Statton J., McMahon K., Lavery P., Kendrick G. A. (2018). Determining light stress response for a tropical multi-species seagrass assemblage. Mar. pollut. Bulletin 128, 508–518. doi: 10.1016/j.marpolbul.2018.01.060
Takahashi S., Murata N. (2008). How do environmental stresses accelerate photoinhibition? Trends Plant Science 13, 178–182. doi: 10.1016/j.tplants.2008.01.005
Tarquinio F., Bourgoure J., Koenders A., Laverock B., Säwström C., Hyndes G. A. (2018). Microorganisms facilitate uptake of dissolved organic nitrogen by seagrass leaves. Multidiscip. J. Microbial Ecology 12, 2796–2800. doi: 10.1038/s41396-018-0218-6
Teichberg M., Fox S. E., Olsen Y. S., Valjma I., Martinetto P., Iribarne O., et al. (2010). Eutrophication and macroalgal blooms in temperate and tropical coastal waters: nutrient enrichment experiments with ulva spp. Global Change Biol. 16, 2624–2637. doi: 10.1111/j.1365-2486.2009.02108.x
Terrados J., Williams S. L. (1997). Leaf versus root nitrogen uptake by the surfgrass Phyllospadix torreyi. Mar. Ecol. Prog. Series 149, 267–277. doi: 10.3354/meps149267
Touchette B. W., Burkholder J. M. (2000). Review of nitrogen and phosphorus metabolism in seagrasses. J. Exp. Biol. Ecology 250, 133–167. doi: 10.1016/S0022-0981(00)00195-7
Touchette B. W., Burkholder J. M., Glasgow H. B. (2003). Variations in eelgrass (Zotera marina l.) morphology and internal nutrient composition as influenced by increased temperature and water column nitrate. Estuaries 26, 142–155. doi: 10.1007/BF02691701
Turpin D. H. (1991). Effects of inorganic n availability on algal photosynthesis and carbon metabolism. J. Phycology 27, 14–20. doi: 10.1111/j.0022-3646.1991.00014.x
Tyler A. C., McGlathery K. J., Anderson I. C. (2001). Macroalgae mediation of dissolved organic nitrogen fluxes in a temperate coastal lagoon. Estuar. Coast. Shelf Science 53, 155–168. doi: 10.1006/ecss.2001.0801
Tyler A. C., McGlathery K. J., Macko S. A. (2005). Uptake of urea and amino acids by the macroalgae Ulva lactuca (Chlorophyta) and Gracilaria vermiculophylla (Rhodophyta). Mar. Ecol. Prog. Series 294, 161–172. doi: 10.3354/meps294161
Udy J. W., Dennison W. C., Long W. J. L., McKenzie L. J. (1999). Responses of seagrasses to nutrients in the great barrier reef, Australia. Mar. Ecol. Prog. Series 185, 257–271. doi: 10.3354/meps185257
Valiela I., Cole M. L. (2002). Comparative evidence that salt marshes and mangroves may protect seagrass meadows from land-derived nitrogen loads. Ecosystems 5, 92–102. doi: 10.1007/s10021-001-0058-4
Valiela I., Foreman F., LaMontagne M., Hersh D., Costa J., Peckol P., et al. (1992). Couplings of watersheds and coastal waters: sources and consequences of nutrient enrichment in waquoit bay, Massachusetts. Estuaries 15, 443–457. doi: 10.2307/1352389
Valiela I., McGlelland J., Hauxwell J., Behr P. J., Hersh D., Foreman K. (1997). Macroalgal blooms in shallow estuaries: controls and ecophysiological and ecosystem consequences. Limnology Oceanography 42, 1105–1118. doi: 10.4319/lo.1997.42.5_part_2.1105
van Alstyne K. L. (2008). “Ecological and physiological roles of dimethylsulfoniopropionate (DMSP) and its products in marine macroalgae,” in Algal chemical ecology. Ed. Amsler C. D. (Heidelberg: Springer), 173–194.
van England T., Bouma T. J., Morris E. P., Brun F. G., Peralta G., Lara M., et al. (2011). Potential uptake of dissolved organic matter by seagrasses and macroalgae. Mar. Ecol. Prog. Series 427, 71–81. doi: 10.3354/meps09054
van Katwijk M. M., Vergeer L. H. T., Schmitz G. H. W., Roelofd J. G. M. (1997). Ammonium toxicity in eelgrass Zostera marina. Mar. Ecol. Prog. Series 157, 159–173. doi: 10.3354/meps157159
van Lent F., Verschuure J. M., van Veghel M. L. J. (1995). Comparative study on population of Zostera marina l. (eelgrass): in situ nitrogen enrichment and light manipulation. J. Exp. Mar. Biol. Ecology 185, 55–76. doi: 10.1016/0022-0981(94)00131-V
Villazán B., Pedersen M. F., Brun F. G., Vergara J. J. (2013). Elevated ammonium concentrations and low light from a dangerous synergy for eelgrass Zostera marina. Mar. Ecol. Prog. Series 493, 141–154. doi: 10.3354/meps10517
Vonk J. A., Middelburg J. J., Stapel J., Bouma T. J. (2008). Dissolved organic nitrogen uptake by seagrasses. Limnology Oceanography 53, 542–548. doi: 10.4319/lo.2008.53.2.0542
Walker D. I., McComb A. J. (1992). Seagrass degradation in Australian coastal waters. Mar. pollut. Bulletin 25, 191–195. doi: 10.1016/0025-326X(92)90224-T
Wang X. J. (2015). Effects of urea on the growth of typical algae in East China Sea (Qingdao, China: Master Thesis in University of Ocean University of China).
Wang X., Chen R. F., Cable J. E., Cherrier J. (2014). Leaching and microbial degradation of dissolved organic matter from salt marsh plants and seagrasses. Aquat. Sci. 76, 595–609. doi: 10.1007/s00027-014-0357-4
Xie X. J., Lu X. P., Wang L. P., He L. W., Wang G. C. (2020). High light intensity increases the concentrations of β-carotene and zeaxanthin in marine red macroalgae. Algae Res. 47, 101852. doi: 10.1016/j.algal.2020.101852
Xu R. S. (2020). Effects of different of nitrogen sources on the growth and biochemical components of monostroma nitidium gametophytes (Zhanjiang, China: Master Thesis in University of Guangdong Ocean University).
Zhang T., Wang X. (2017). Release and microbial degradation of dissolved organic matter (DOM) from the macroalgae. Ulva prolifera Mar. pollut. Bulletin 125, 192–198. doi: 10.1016/j.marpolbul.2017.08.029
Zhang P. Y., Xin Y., Zhong X. S., Yan Z. W., Jin Y. M., Yan M. J., et al. (2021). Integrated effects of Ulva prolifera bloom and decay on nutrients inventory and cycling in marginal sea of China. Chemosphere 264, 128389. doi: 10.1016/j.chemosphere.2020.128389
Zhang X., Zhou Y., Adams M. P., Wang F., Xu S. C., Wang P. M., et al. (2020). Plant morphology and seed germination responses of seagrass (Zostera japonica) to water depth and light availability in ailian bay, northern China. Mar. Environ. Res. 162, 105082. doi: 10.1016/j.marenvres.2020.105082
Zhang X. M., Zhou Y., Liu P., Wang F., Lui B. J., Liu X. J., et al. (2014). Temporal pattern in the bloom-forming macroalgae Chaetomorpha linum and Ulva pertusa in seagrass beds, swan lake lagoon, north China. Mar. pollut. Bulletin 89, 229–238. doi: 10.1016/j.marpolbul.2014.09.054
Keywords: species competition, light reduction, organic nitrogen, inorganic nitrogen, macroalgal blooms, seagrasses
Citation: Han Q, Qiu C, Zeng W, Chen Y, Zhao M, Shi Y and Zheng F (2023) Effect of DIN and DON sources on the nitrogen uptake of the seagrass Zostera japonica and the macroalgae Ulva pertusa previously grown in different light levels. Front. Mar. Sci. 10:1015323. doi: 10.3389/fmars.2023.1015323
Received: 09 August 2022; Accepted: 17 January 2023;
Published: 03 February 2023.
Edited by:
Zhan Hu, Sun Yat-sen University, Zhuhai Campus, ChinaReviewed by:
Peidong Zhang, Ocean University of China, ChinaDilip Kumar Jha, National Institute of Ocean Technology, India
Copyright © 2023 Han, Qiu, Zeng, Chen, Zhao, Shi and Zheng. This is an open-access article distributed under the terms of the Creative Commons Attribution License (CC BY). The use, distribution or reproduction in other forums is permitted, provided the original author(s) and the copyright owner(s) are credited and that the original publication in this journal is cited, in accordance with accepted academic practice. No use, distribution or reproduction is permitted which does not comply with these terms.
*Correspondence: Qiuying Han, aGFucWl1eWluZzAzMTJAc2luYS5jb20=