- 1Departamento de Ciencias Geológicas, Facultad de Ciencias Exactas y Naturales, Instituto de Geociencias Básicas, Universidad de Buenos Aires, Aplicadas y Ambientales de Buenos Aires (IGEBA-UBA-CONICET), Buenos Aires, Argentina
- 2Norwegian Research Center AS (NORCE) Norwegian Research Center AS, Climate & Environment Department, Bergen, Norway
- 3Marine Sciences, South Atlantic Environmental Research Institute, Stanley, Falkland Islands
- 4Gothenburg Global Biodiversity Centre and Department of Marine Sciences, University of Gothenburg, Gothenburg, Sweden
- 5Departamento de Biologia & CESAM (Center for Environmental and Marine Studies), Universidade de Aveiro, Aveiro, Portugal
- 6Ocean Systems, Royal Netherlands Institute for Sea Research (NIOZ), Texel, Netherlands
- 7National Oceanography Centre, European Way, Southampton, United Kingdom
- 8Center for Marine Biodiversity and Conservation, Scripps Institution of Oceanography, University of California San Diego, La Jolla, CA, United States
- 9Louisiana Universities Marine Consortium, Chauvin, LA, United States
- 10Scottish Association for Marine Science, Oban, United Kingdom
- 11Guy Harvey Oceanographic Research Center, Nova Southeastern University, Dania Beach, FL, United States
- 12Norwegian Institute for Water Research, Marine Biology, Bergen, Norway
- 13Biology Department, Temple University, Philadelphia, PA, United States
The deep ocean comprises complex ecosystems made up of numerous community and habitat types that provide multiple services that benefit humans. As the industrialization of the deep sea proceeds, a standardized and robust set of methods and metrics need to be developed to monitor the baseline conditions and any anthropogenic and climate change-related impacts on biodiversity, ecosystem function, and ecosystem services. Here, we review what we have learned from studies involving offshore-energy industries, including state-of-the-art technologies and strategies for obtaining reliable metrics of deep-sea biodiversity and ecosystem function. An approach that includes the detection and monitoring of ecosystem services, with open access to baseline data from multiple sectors, can help to improve our global capacity for the management of the deep ocean.
Introduction
In the past century that humankind has been studying the deep ocean (defined here as the water column and seafloor below 200 m water depth), we have come to understand that it plays a key role in sustaining the well-being of multiple and diverse life forms through a myriad of different ecosystem functions (Thurber et al., 2014; Danovaro et al., 2020; Levin et al., 2020). The deep ocean also provides important benefits to human well-being, collectively known as ecosystem services (MA, 2005), but also referred to as nature’s contribution to people. We adopt the term ecosystem services, as the alternate expression emphasizes the role of culture and indigenous and local knowledge in defining nature’s links with people (Díaz et al., 2018, Box 1). Intact and high- functioning ecosystems provide benefits both directly and indirectly, including biodiversity, natural resources (such as food, genetic resources, energy, minerals), regulation of climate and biogeochemical pathways, innovation in science and technology, spiritual connections, and inspiration for design, literature, and art (MA, 2005, Thurber et al., 2014; Le et al., 2017; Mejjad and Rovere, 2021).
Box 1. Definition of key concepts.
Ecosystem Services: The benefits to humans obtained from ecosystem processes, whether or not there exists a market for the benefits in question (MA, 2005, Thurber et al., 2014; Le et al., 2017; Yow Mulalap et al., 2020; DOSI 2021a; Tilot et al., 2021).
● Supporting Services: The functions of the target ecosystem that support processes and services generated by other processes in the same and other ecosystems (e.g., Element cycling, Nutrient cycling, Metabolic activity, Habitat provision, Primary and secondary production, Connectivity, Dispersal, Respiration, Bioturbation).
● Provisioning Services: The benefits of the “products” obtained from ecosystems (e.g., Fisheries, Novel genetic resources (e.g., for biopharmaceutical, biomimetics, bioengineering), Energy (e.g., oil and gas, wind, waves, sun, ocean current, ocean thermal gradients, green hydrogen, biomass for biofuels), Minerals (e.g., manganese nodules, phosphorite deposits, etc.), Industrial agents, Biomaterials).
● Regulating Services: The regulation benefits generated by the processes and cycles of ecosystems. (e.g., Climate regulation, Carbon sequestration, Population regulation, Biogeochemical regulation, Biological control, Detoxification, and repositories for pollutants).
● Cultural Services: The benefits for cultural development. (e.g., Education, Science, Inspiration for art, Inspiration for technology, Stewardship, Spiritual value, Traditional knowledge)
Environmental Impact Assessment (EIAs): Formal process conducted prior to the initiation of a project, aimed at determining the potential impact on the environment of the industrial activities based on the type of ecosystem (usually containing environmental and socio-economic components of the ecosystem). The main components of EIAs include: 1) description of the project; 2) environmental baseline conditions; 3) assessment of the potential impact on the environment; 4) proposed mitigation of impacts and 5) identification of knowledge gaps (Glasson & Therivel, 2019; Jones et al., 2019a).
Clean Energy: compared with fossil fuel energy sources, clean energies should meet the following requirements to be considered as “clean”: lower or null GHG emission; low environmental impacts besides the emissions, low impacts on climate-regulating ecosystem services (Carley and Konisky, 2020; Jager et al., 2021).
Ecosystem-Based Management: Ecosystem-based management (EBM) is an approach with the objective of protecting and enhancing sustainability, diversity, and productivity of natural resources that integrates biological, social, and economic components of ecosystems (Frazão Santos et al., 2020).
Humans often exploit these ecosystem services without a good understanding of the impacts on the processes that sustain them (Levin et al., 2020; Mejjad and Rovere, 2021). For example, fisheries bottom trawls remove coral colonies, which can be hundreds to thousands of years old (Watling and Norse, 1998; Roberts et al., 2005) and provide nursery and breeding grounds for the same species that are targeted by the fisheries (Freiwald et al., 2004; Clark et al., 2016). In another example, the Deepwater Horizon (DWH) oil spill caused significant damage to coral colonies that were hundreds of years old (Prouty et al., 2016), and complete recovery of the ecosystem and its services may require time scales on the same order-of-magnitude (Girard & Fisher, 2018). In order to be more effective in weighing the cost-benefit ratio of industrial exploitation and in designing mitigation measures for associated impacts, we need to better integrate ecosystem services as a whole into our environmental impact assessment (EIA) (Box 1) and management frameworks.
The deep ocean houses the least known set of ecosystems on Earth, and it remains a logistical and economic challenge to study, especially for Low/Middle Income Countries (Glover et al., 2009; Thaler & Amon, 2019; Howell et al., 2020; Howell et al., 2021). The advancement of ocean-based economic initiatives (such as the “Blue Growth Initiative” launched by FAO in 2013 and the EU’s “blue-growth strategy”, Eikeset et al., 2018) pose a challenge for environmental management and monitoring that transcends political boundaries, as there is only one connected ocean, and it is rapidly changing (FAO, 2017; Laffoley et al., 2020). In many settings, our lack of fundamental knowledge of the baseline states of biodiversity and ecosystem structure have hampered our ability to effectively manage natural resources (Glover et al., 2018). For example, the DWH oil spill occurred in one of the best-known areas of the deep ocean, with decades of research effort, and yet the assessment of the impacts required fundamental exploration in the immediate vicinity of the disaster (Fisher et al., 2014). Furthermore, the available baseline data lacked information about the ecosystem functions and services in the immediate surrounding impacted areas (Cordes et al., 2016; Schwing et al., 2017; Halanych et al., 2021; Sutton et al., 2022).
Although the COVID-19 pandemic resulted in an ~5% decrease in global Oil & Gas (O&G) consumption in 2020 (Cornwall, 2020), demand and production are projected to grow by ~50% until 2040, with the highest expansion (69%) in production concentrated in the deep sea (Watts, 2012; Brent et al., 2020). For example, in 2020, 178 O&G platforms at water depths > 1,000 m accounted for 86% of Gulf of Mexico oil and 54% of gas production, with these percentages and total oil production increasing dramatically over the past decade (Murawski et al., 2020). It is currently not clear how much O&G exploitation can be sustained while still achieving the Paris Agreement targets, but the industry position is that its expansion is necessary even in the most sustainable rebalance scenarios (Equinor, 2022).
The deep ocean is potentially an important part of the solution to achieve net-zero carbon emissions. Recent technological advances, high demand for clean energy, and the great potential for the offshore areas of the open ocean (any part of the ocean, from the surface to the seafloor, where waters depths exceed 200 m) to provide energy resources make a future increase in offshore renewable energy exploitation likely (Bailey et al., 2014; Junqueira et al., 2020; Global Wind Energy Council, 2021). Most of these energy projects are centered around the development of wind farms (Global Wind Energy Council, 2021) but others include marine hydrokinetic energy (tidal, wave, and ocean current), solar energy, thermal gradient energy (geo-thermal or ocean-thermal energy conversion), as well as the production of green hydrogen or biofuels (Hammar et al., 2017, Haugan et al., 2019). These are all considered to be “clean” energy (Box 1) sources mainly owing to their low or null emission of greenhouse gasses (GHG, Carley and Konisky, 2020), but they can also lead to other environmental effects that require consideration (Luderer et al., 2019). Facing the climate crisis requires preserving the climate-regulation services provided by the deep sea (Queirós et al., 2016; Sala et al., 2021; Hilmi et al., 2021) while also increasing the use of its energy resources. Clean energy production in the open ocean must be measured, mitigated, monitored, and considered both in relation and in addition to other anthropogenic pressures, such as climate change, and managed in an integrated framework (Hammar et al., 2017, Figure 1).
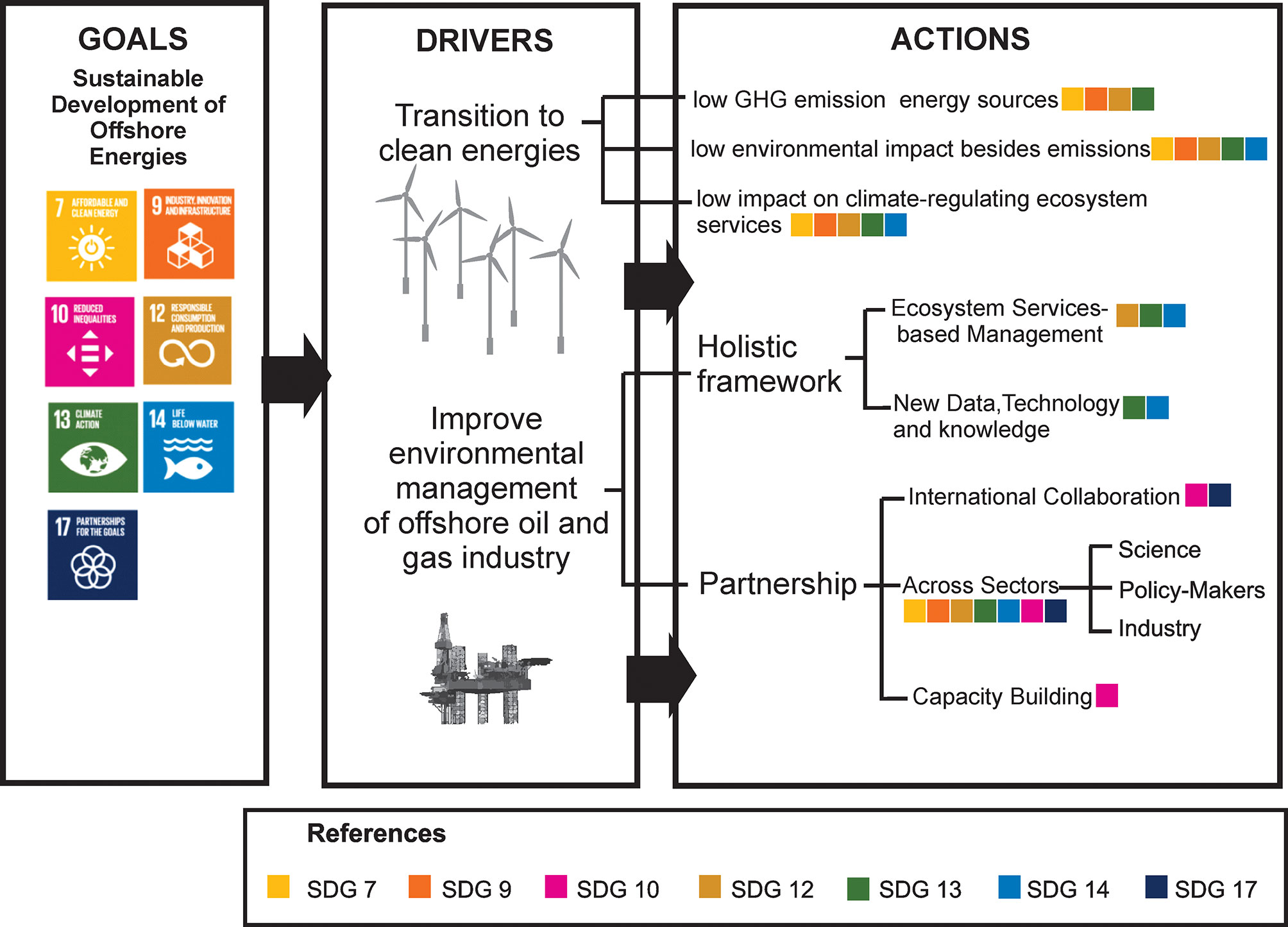
Figure 1 The sustainable development of offshore energy industries framed onto the United Nations Sustainable Development Goals (SDGs). We propose two main drivers and examples of specific actions that can play key roles meeting some SDGs. The SDGs that can be met by each action (illustrated by their respective colors in the squares) are SDG 7, “Clean and affordable energy”; SDG 9, “Industry innovation and infrastructure”; SDG 10, “Reduced inequalities”; SDG 12, “Responsible consumption”; SDG 13, “Climate action”; SDG 14, “Life below water” and SDG 17, “Partnership for the goals”.
The global growth in offshore energy industrial activities will increase the environmental impact that threatens ocean ecosystems and the maintenance of the benefits and services derived by humans. Ecosystem-based management (EBM) (Box 1) is an approach with the objective of protecting and enhancing sustainability, diversity, and productivity of natural resources by integrating the biotic, social, and economic aspects of ecosystems (Frazão Santos et al., 2020). EBM is promoted by many regulatory bodies and international agreements such as the United Nations Convention on the Law of the Sea (UNCLOS) and the Convention on Biological Diversity (CBD) (Douvere & Ehler, 2009). This approach is preferred for setting strategic goals and objectives in the deep sea (Tunnicliffe et al., 2020; Sala et al., 2021), but has rarely been implemented there.
United Nations bodies such as the Food and Agriculture Organization (FAO) and the International Seabed Authority (ISA) provide recommendations and regulations for environmental impact assessments (EIA) in international waters that could be adapted and used by countries within their different Exclusive Economic Zones (EEZs). The FAO and ISA are primarily focused on fisheries and deep-sea mining, respectively, but to the best of our knowledge, there are no equivalents in the offshore energy industry. Furthermore, there is limited feedback between FAO and ISA, which manage areas beyond national jurisdiction (ABNJ), and the offshore O&G industry, which operates largely within EEZs. Notably, parties that signed UNCLOS (UNCLOS Part XII, Article 208) are committed to implementing rules and regulations for seabed mining within their EEZs that, as a minimum, meet those developed for ABNJ. To understand, mitigate, and manage the impact of offshore industries in the global ocean there is a need for improving and harmonizing the approaches used for EIAs across the different offshore industries, and efforts toward equal accessibility to environmental data and technology across EEZs.
The generation of electricity is the single most important source of CO2 emissions (40% of total CO2 emissions) (Luderer et al., 2019). At the same time, electricity offers the highest potential for low-cost global strategies to reduce GHG emissions to near zero by 2050, in line with the Paris Agreement (Luderer et al., 2019, Haugan et al., 2019). These strategies seek to minimize both the production and the use of fossil fuels through expansion of clean energy-based generation of electricity together with increasing electrification of the global energy system across multiple sectors such as transportation, construction, and industry (Luderer et al., 2019, Haugan et al., 2019). Beyond GHG emissions, the sustainable development of the global energy system in the deep ocean requires considerations on the social and environmental aspects associated with its development, such as those established in the UN Sustainable Development Goals (SDGs) adopted by the United Nations in 2015 (Luderer et al., 2019, Haugan et al., 2019). In the case of offshore energy, the future of this sector is relevant for SDG 7 “Clean and affordable energy”, SDG 9: “Industry innovation and infrastructure”, SDG 12: “Responsible consumption”, SDG 13: “Climate action”, and SDG 14: “Life below water”. Furthermore, there is a need for equity and justice both in the accessibility of deep-ocean ecosystems and their resources, together with collaborative networks (e.g., across sectors and geographic boundaries) for effective management, which are important for SDG 10: “Reduced inequalities” and SDG 17: “Partnerships for the goals” (Figure 1).
The potential impacts on ecosystem services remain unclear for ocean-based renewables (Haugan et al. 2020, Mejjad & Rovere, 2021). The current approaches of EIA for O&G do not typically provide data on the ecosystem functions and services affected by industrial activities (Sommer et al., 2019; Mejjad & Rovere, 2021). There is a need for better integration of science into industry and policy to both build understanding of the complex environmental responses of the deep sea to disturbance and to guide its effective management (Sommer et al., 2019; Kujawinski et al., 2020). This requires revising the current approaches to EIAs and the design of a new framework for the holistic integration of environmental and social aspects related to ecosystem functions and services that are affected by industrial activities (Figure 1). The design of this new framework needs to be flexible, so that new knowledge and technologies developed to study deep-sea ecosystems can be integrated, as this is a rapidly changing field (Sommer et al., 2019).
Here, we propose a preliminary design for this new framework, which seeks to augment ecosystem-based management to include ecosystem services-based management. The current approaches to environmental management of offshore O&G (in waters deeper than 200 m and inside the EEZs) are reviewed, focusing on opportunities for improvements through a unified effort across social sectors and geographic regions. We believe that a number of cross-sectoral and international partnerships can act as key enablers for the integration of factors necessary for effective management, such as environmental data acquisition and interpretation, research and technological innovation, capacity building, industrial risk reduction, and financial and political aspects. These partnerships can shape the direction of offshore-energy industrial development in congruence with priority areas of the UN SDGs including climate change, education, and poverty (Novaglio et al., 2022). Finally, we propose a new framework that includes ecosystem services within ecosystem-based management and discuss how it can guide the management of both upcoming renewable-energy development and other offshore industries.
Environmental impact assessments in offshore oil and gas industry
In order to fully incorporate ecosystem services into the management of offshore industries, we first need to examine the current process for the oil and gas industry, derive the relevant successes, and identify the existing gaps. The EIA is an important tool for decision-makers to understand and mitigate the impact of a project. The EIA process should develop a plan that includes: 1) monitoring programs; 2) management of activities; and 3) mitigation actions, which include temporal and spatial management actions (Glasson and Therivel, 2019, Jones et al., 2019a). Within each jurisdiction there are different requirements for EIAs but there are some shared structures and requirements used for O&G. The main components of EIAs include: 1) description of the project; 2) environmental baseline conditions; 3) assessment of the potential impact on the environment; 4) proposed mitigation of impacts and 5) identification of knowledge gaps (Jones et al., 2019a). Each of these elements could incorporate a description of ecosystem services that are provided, enhanced, impaired or need investigation.
There are many countries for which EIAs are not required and the companies are “self-regulated” under the premise of following established best practices and internal industrial policies. In many areas, EIAs are only required prior to exploratory drilling (Box 2), or EIAs are required but the methods are not stipulated. For example, in Portugal the companies are required to take “all measures to prevent pollution”, or in Tanzania “environmental protections should follow best practices requirements” (Cordes et al., 2016). The recommended approach to an EIA would be following those currently implemented in some countries (e.g., Australia, Canada, Barbados, and Norway), which have a robust screening process to ensure that EIAs are required for projects that may have significant effects. This would certainly trigger an EIA for any major project, such as before drilling for exploration or production of O&G. An EIA should stipulate that environmental monitoring is carried out to assess impact. In some jurisdictions, monitoring is required to be completed at regular intervals, for example every three years (Norway) or five years (Barbados) (Cordes et al., 2016).
Box 2. Activities involved at each phase of development of the offshore oil and gas industry.
Exploratory phase:
Seismic survey: The exploratory phase has a first phase, the seismic survey, which is aimed at detecting and mapping hydrocarbon reservoirs up to kilometers below the seafloor. The acoustic signal is emitted by airguns and the signal returning from the bottom and kilometers below the seabed is “heard” by the hydrophone arrays that are arranged in the streamer. The arrangement of airguns and streamers can cover kilometers. Compared with other seismic equipment, the acoustic signal has high potency and low frequency to prioritize high penetration, but also has relatively low resolution. Surveys are usually conducted in the form of a grid that crosses the track of the vessel perpendicularly in a limited area, during slow navigation. This allows 3D and 4D models to be reconstructed with the seismic records obtained.
Exploratory drilling: This is the second phase of the exploratory phase and is aimed at obtaining rock samples and making in situ measurements (up to 4 km below the seafloor) in the area of interest (selected from seismic records and a range of remote sensing techniques used to characterize the formations) to establish the existence and nature of hydrocarbon reservoirs. It is conducted using temporary rigs or drillships. Rigs or drillships use a series of anchors or dynamic positioning (DP) to maintain position. The drilled well typically has a surface diameter of up to 1 m and is cased with steel pipes held in place with cement. It is drilled with drilling muds (seawater with natural and synthetic additives). Blow-out preventers with valves are used to secure and control the well. Rock cuttings are usually treated and returned to sea. Once finished (usually after 1-3 months), the well is cemented, secured, and abandoned.
Production phase: In production phase, an operational field includes various wells with installation of surface and/or submersed infrastructure. The surface infrastructure is composed of large floating installations for production and storage as well as for offloading vessels. Submerged infrastructure includes manifolds, control cables, and export lines for producing and exporting the hydrocarbons, and connecting the several wells that together typically comprise the production field (Cordes et al., 2016).
Decommissioning: After the production ends, the platforms are dismantled and may be recovered to shore. However, as this is expensive and has high environmental risks, alternative uses for abandoned oil platforms are being developed (e.g., hotels, Zawawi et al., 2012). Decommissioning and the alternative options are associated with different types of environmental impacts, which vary with time and geographic space and require site-specific integral assessment (Sommer et al., 2019).
The types of variables on which to focus in an EIA are determined by the types and importance of impacts that are expected from the activity. These are typically identified in the scoping stage of an EIA (Durden et al., 2018). In some cases, scoping is documented in a report, reviewed, and approved by the regulator to guide the development of the full EIA. Based on the types of impacts expected from O&G activity, the typical design of an EIA is to establish baseline conditions of the physical environment (e.g. topography, sediment grain size), environmental chemistry (including concentrations of any materials that are likely to be discharged), as well as benthic and pelagic community structure (distribution and abundance of fauna in different size classes) often focusing on marine mammal distribution (to avoid impacts from seismic surveys, vessel strikes, and direct oiling). Good opportunities to identify and fill information gaps arise from the growing availability of environmental data, new technologies, analytical techniques and computer power (Sommer et al., 2019; Kujawinski et al., 2020). Scientists and citizens should have access to the information from all stages of the EIA process, and this transparency may help industries and governments build trust and obtain feedback to improve their practices.
The impacts of an activity are determined by detecting significant change in the baseline conditions that were present prior to the onset of activity. Being aware that baseline conditions are, by nature, spatially and temporally dynamic, the assessment of these conditions should allow decision-makers to differentiate environmental stochasticity from those conditions attributable to direct human disturbance activities (Nowacek et al., 2013). This approach integrates physical and biological studies of the seafloor and water column and should be included in EIAs for all phases of a project, including exploration (alluding to potential impacts of exploratory wells), production, and decommissioning (Box 2).
In general, any EIA submitted by the companies should propose environmental impact mitigation measures that avoid areas particularly sensitive to environmental impacts. This implies that the stakeholders and the companies need to identify these areas prior to the onset of O&G activities. Environmental monitoring should be required in the operation area, the area of influence, and the exclusion or control areas. Best practices for BACI (Before-After/Control-Impact) studies during monitoring include a strategic selection of both exclusion zones for conservation (i.e., vulnerable marine ecosystems such as cold seeps and cold-water coral reefs) and for control sites, as well as benthic species that serve as ecological indicators of anthropogenic disturbance (Cordes et al., 2016).
As the production life-time of an offshore platform is reaching an end, the decommissioning process is begun (Box 2). There are many approaches to decommissioning including full removal, partial removal, and leaving in place e.g., to preserve artificial reefs (Fowler et al., 2019). Hem et al. (2014) estimated that in 2015 annual costs of decommissioning were $2.4 billion and predicted an increase of the investment by 2040 to $13 billion. While removal of structures will theoretically return the habitat to its original state, impacts to the seafloor can be caused by removal through direct damage and reintroduction of contaminants (Cordes et al., 2016).
The policies to manage decommissioning of abandoned oil platforms range from complete removal in the North Sea (Fowler et al., 2019; Sommer et al., 2019) and Australia (Chandler et al., 2017), to alternative use options such as in the United States, where abandoned oil platforms are used as artificial reefs for preservation or enhancement of fish stock via programs known as “Rigs to Reefs” (Macreadie et al., 2011). Submerged foundations, pipes, cables, and scour protection add hard substrate to the environment and inevitably attract a range of marine fauna including commercially significant fishes (Bergström et al., 2013; Hammar et al., 2017). Abandoned structures, if left at the seafloor, may act as hindrance to bottom trawling, an activity with well-known negative impact on marine ecosystems and, thus, mirror the positive effects from no-take MPA’s (Fowler et al., 2019). Some oil platforms that are left in place are reused for other purposes. For example, in the North Sea there are some initiatives trying to use the oil platforms to exploit geothermal energy (Fowler et al., 2019). In Norway and in Saudi Arabia some more exotic initiatives include the transformation of abandoned O&G platforms into tourist attractions (Zawawi et al., 2012). In addition, decommissioned offshore O&G energy infrastructure has been identified as a potential site for future carbon capture and storage (CCS) activities (Neele et al., 2011). Furthermore, the abandoned O&G infrastructure could be used for environmental monitoring, taking advantage of existing power distribution and communication infrastructure to establish cabled fixed-point observatories (FPOs) or networks of marine autonomous systems (MAS, Jones et al., 2019b).
The ecosystem services provided by artificial reefs can be different from those generated by the original communities. For example, the Rigs-to-Reefs program may facilitate the restoration of deep-water benthos (such as cold-water corals) by restricting access to deep-water trawlers, but can negatively impact existing benthic communities leading to undesirable changes in their functions and services (biogeochemical cycling, detoxification, marine food webs) (Macreadie et al., 2011; Sommer et al., 2019). The addition of other functions to existing infrastructure such as the conversion of O&G wells to Carbon Capture and Storage (CCS) systems (Sommer et al., 2019) carries its own set of environmental risks and potential impacts (Levin et al., 2022). Thus, the full range of environmental risks and benefits of each option for reducing, reusing, and building new infrastructure, including the ecosystem service outcomes of each option, must be considered at the site-specific and regional scales.
Offshore energy in the 21st century
The development of marine renewable energies would positively impact all 17 SDGs (Hoegh-Guldberg et al., 2019). Marine renewable energies (Figure 2), compared to coal-fired power, have low CO2 and negligible emissions of Hg, SO2, and NO2; improve human health (through decrease in contamination); and can provide higher employment and better gender balance in the work environment (Haugan et al., 2019). There is consensus that marine renewables play an important role for the global energy transition in line with both decreasing human contributions to global warming and supply for increased energy demand (Jager et al., 2021; Mejjad and Rovere, 2021). However, the potentially negative impacts of marine renewable energies on SDG 14 (“Life below water: Conserve and sustainably use the oceans, seas and marine resources for sustainable development”) in the deep ocean may pose a challenge to their expansion (Haugan et al., 2019).
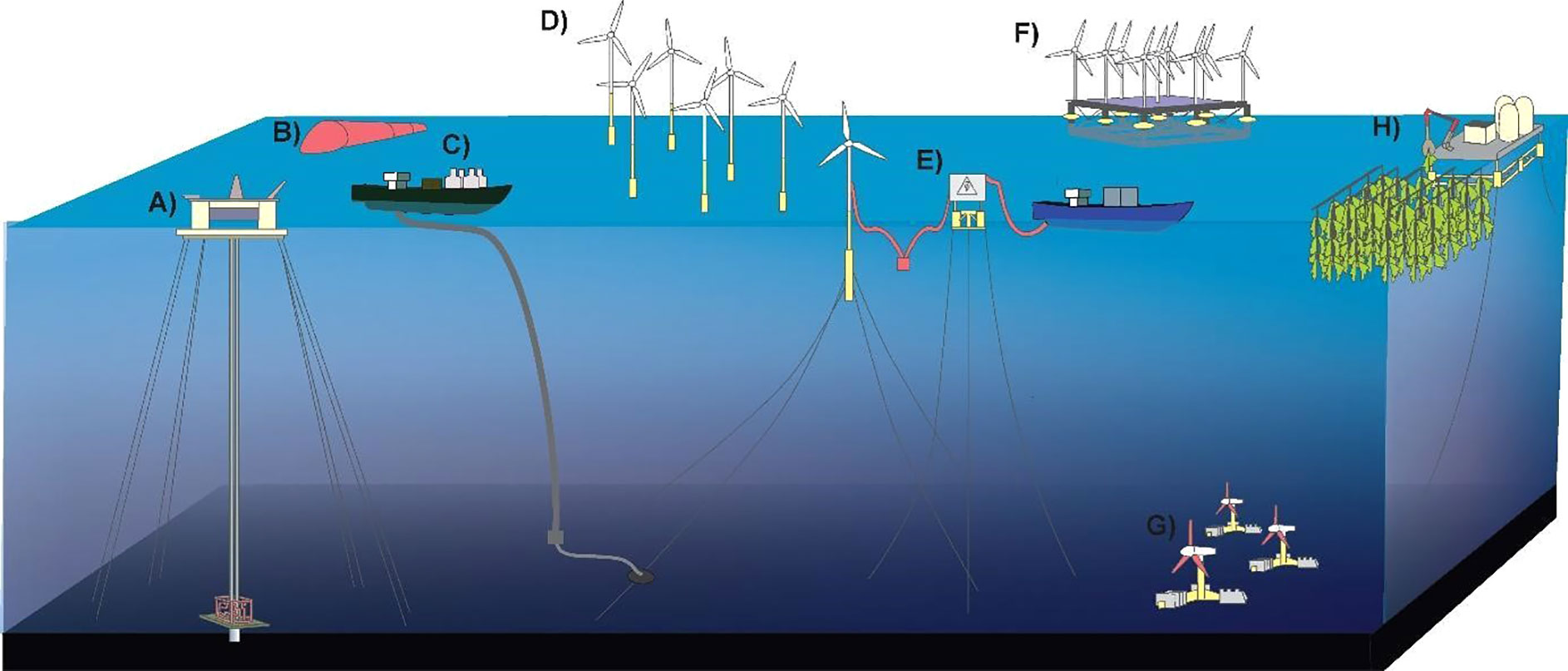
Figure 2 Schematic representation of different types of energy industries in current or potential development in the deep ocean. (A) Oil and gas, (B) wave energy, (C) ocean thermal energy conversion (OTEC), (D) wind energy, (E) green hydrogen, (F) device combining wind, sun and wave energy, (G) ocean current energy, (H) biofuels.
In the global deep ocean, most marine renewable energies are still at an experimental state, resulting in high scientific uncertainty about their potential impacts on ecosystem services (Jager et al., 2021; Mejjad and Rovere, 2021). Enhancing research on less mineral-intensive renewable energy (instead of the current ones that require rare minerals and contribute to the demand for accelerating deep-sea mining), as well as on risks to ecosystem services is important for their expansion in line with SDG 14 (Haugen et al., 2019). At the same time, both understanding and mitigating the impacts of new offshore installations requires baseline study designs tailored to site-specific characteristics and ideally specific to the impacts on ecosystem services (Haugan et al., 2019, Jager et al., 2021; Mejjad and Rovere, 2021). The global offshore waters has a high renewable energy potential and the expansion of its sustainable exploitation to meet the Paris Agreement target is overdue (Haugan et al., 2019). Joint efforts among science, industry, and regulatory bodies will accelerate the expansion of sustainable exploitation of renewable energy in the offshore.
Offshore wind
Globally, offshore installations (over deep water) present a technical wind energy potential (without considering cost constraints and some technological elements) of 76,757 TW per year near shore (sites within 60 km) and 257,081 TW per year further offshore (60-300 km offshore) (IEA, 2019a, Haugan et al., 2019). Of the near-shore sites, 29.82% of the global potential is within the North America EEZ, 19.3% in Europe and 18.8% in Asia. The same regions have the most potential in offshore waters, with 22.9% in North America, 20.23% in Europe, 18.96% in Eurasia, and 16.09% in the Asia-Pacific region (IEA, 2019a). Europe currently dominates the installation of infrastructure for offshore-wind energy exploitation (Haugan et al., 2019, Mejjad & Rovere, 2021). The European Commission designed a strategic roadmap that would allow for an accelerated and significant expansion of offshore wind farms in its region (Haugan et al., 2019). It is estimated that China will overtake Europe in installed capacity by early 2030 and that the USA will be third in this respect (Haugan et al., 2019, IEA, 2019b).
The cost per MW of installed power decreased in the last decade and the cost of operation and management per megawatt-hour (MWh) produced is also expected to decrease thanks to more robust turbine designs suitable for deep-ocean installations (Haugan et al., 2019). Deep waters require floating support structures (as opposed to shallow waters that require bottom-fixed structures) that are less mature and more expensive (Haugan et al., 2019). However, floating support structures are suitable for standardized mass production without site-specific requirements, which could lower their cost and make them comparable to bottom-fixed structures (Haugan et al., 2019).
The environmental risks of offshore energy production have been assessed mainly for shallow water but remain uncertain in the deep sea. Increased turbulence around the submerged structures and shifts in Ekman transport causing local upwelling/downwelling may increase upper-ocean mixing (Broström, 2008; Floeter et al., 2022). Variability in vertical mixing at the mesoscale has been shown to be a significant, but generally overlooked factor controlling the distribution of heat, nutrients, and carbon in the ocean (Busecke and Abernathey, 2019). Fouling organisms on submerged structures are also considered to have a regional scale impact on the function of pelagic ecosystems when offshore wind projects are realized according to ambitious plans for future electricity demand (Slavik et al., 2019). One study showed that fouling organisms can increase carbon assimilation significantly, with the potential to increase blue carbon sequestration (Mavraki et al., 2020). Support structures have also been shown to act as artificial reefs, providing shelter, food, and spawning substrate for fish (Bergström et al., 2013). The reef effect in combination with the removal of bottom trawling increases diversity and abundance of fish inside offshore wind farms (Methratta and Dardick, 2019), potentially modifying the ecosystem services provided by the original habitat. These studies are limited to shelf depth areas and there is a need to augment research to understand the impact on ecosystem services in the deep sea, particularly considering the scale of the expansion (covering large offshore areas in a short time) required in the energy transition (Haugan et al., 2019, Mejjad and Rovere, 2021).
With large-scale wind farm development progressing, other concerns include changes in atmospheric mixing and its climatic implications (Wang and Prinn, 2011), collision of fauna with overhead and submerged infrastructure (Haugan et al., 2019), noise pollution, and wildlife interactions with electromagnetic fields from cables (Hammar et al., 2017; Copping et al., 2020). However, it should be noted that approximately 10,000 times more birds are killed per year by domestic cats (2.4 billion) than by all of the wind turbines in the U.S. and Canada (234,000) (Allison et al., 2019). Underwater noise from turbines is heard by fishes within a range of a few tens of kilometers (which is variable between different fish species depending on their hearing abilities as well as between different turbine types) and would affect the maximum acoustic signaling distance of fishes (Wahlberg and Westerberg, 2005, Haugen et al., 2019). Similarly, electro- and magneto-sensitive species have shown clear awareness of artificial electromagnetic fields, possibly modifying behavior, development, and physiology (Copping et al., 2020). Although lab and field based studies have not found deleterious effects of noise and electromagnetic fields on a range of organisms, effects of long-term and large-scale exposure have not been investigated. In terms of cultural services, the increased commercial interest for offshore wind farms over deep water drove greater interest in this environment and attracted general attention to deep-ocean ecosystem services (Mejjad & Rovere, 2021). There is potential for offshore wind farms over deep water farms not to negatively impact SDG14, but this requires proper planning and taking appropriate mitigation measures (WWF, 2014, Haugan et al., 2019).
Other marine renewable energies
Hydrokinetic energy converters (Figure 2) differ mainly in the depth at which they operate (Hammar et al., 2017), with wave and tidal systems consisting of small units operating in shallow waters (0-200 m) and ocean current power systems being larger and deployed in deeper waters (~100+ m) (Hammar et al., 2017). These systems would need to be anchored to the seafloor, resulting in impacts to the benthos. They also involve the movement of large volumes of water across a turbine, which can lead to elevated mortality in the plankton (assuming larger organisms are excluded from the intake), and increased surface mixing that has numerous effects on ocean circulation. The outcome of these impacts on ecosystem services is less well understood, particularly changes in the composition of plankton communities and the net effect of increased mixing on the transport of heat and carbon in the different parts of the ocean.
Ocean Thermal Energy Conversion (OTEC) technologies (Figure 2) are based on the use of thermal gradients in the ocean to create electricity but are mostly in the experimental stage of offshore development. The most readily available thermal gradient that can be used to generate electricity is the temperature difference between surface water (warm) and deep-sea water (cold) (Thurber et al., 2014). This technology has a hypothetical capacity to provide 4000 times the global energy need (Thurber et al., 2014). Thermoelectric generators could also be used to generate power from hydrothermal vents, either by directly tapping the hydrothermal plume or by installing high-pressure thermosyphons in wells on the hydrothermal mound (Thurber et al., 2014). OTEC technology relocates large volumes of water at high flow rates between different depths, potentially leading to changes in water temperature, changes in flow patterns, relocation of pollutants, and displacement of organisms. Although cold seawater pumped up from the deep sea by OTEC devices could be used for mariculture, refrigeration, air conditioning and production of potable water, the massive flow rates needed for OTEC on a large scale can affect global thermohaline circulation and threaten other functions and services, particularly regulatory services, provided by the deep ocean (Thurber et al., 2014).
Green hydrogen is a clean energy carrier and precursor of hydrogen-based fuels (electrofuels) that is produced from a renewable source, in contrast to the hydrogen produced by fossil fuel sources (Ibrahim et al., 2022). Offshore hydrogen production relies on electrolysis of seawater powered by the electricity generated by wind farms and transported using submarine hydrogen pipelines (Ibrahim et al., 2022, Figure 2). While still in the experimental phase, offshore electrolysers would require 1) back-up battery systems due to discontinuous electricity supply by wind turbines; 2) on-site desalination plants for pre-treatment of seawater before electrolysis (although electrolysers capable of directly using seawater are in development); and 3) on-site compressors to pressurize pipelines (Ibrahim et al., 2022). The impacts of desalinization include: 1) feed water intake, 2) high salinity brine discharge 3) discharge of other concentrated chemical species, and 4) potential use of fossil fuels to supplement energy supply for pumps and compressors (Panagopoulos & Haralambous, 2020). The use of surface intake systems can trap marine animals on the suction racks causing their injury or death (Panagopoulos and Haralambous, 2020). This impact, and others (e.g., chemical contamination, energy consumption), can be reduced by using subsurface intake systems (Panagopoulos and Haralambous, 2020). Brine discharge poses a series of effects known to affect marine species (and associated ecosystem services) such as its high salinity (1.6 to 2.1 times higher than seawater) that can cause ‘lethal osmotic shock’ to some species; heavy metals and residues of chemicals (Panagopoulos & Haralambous, 2020).
Offshore cultivation of marine biomass (e.g., macroalgae) can be used to produce biofuels (biodiesel, biobutanol), food, and chemicals (Fernand et al., 2017, Figure 2). Marine macroalgae have advantages such as being efficient photosynthetic organisms, exhibiting fast growth, bearing valuable chemical compounds, fixing inorganic carbon (allowing its use as carbon trap and then as fuel), and sequestering dissolved inorganic nutrients (Fernand et al., 2017). Some offshore biorefineries currently exist in Israel, The Netherlands, France, Germany, and Norway (Fernand et al., 2017). For offshore marine biomass cultivation, there is currently no universal definition, and concepts include using tidal flat farms, floating cultivation, ring cultivation, wind-farm, or oil-rig integrated systems (Fernand et al., 2017). The use of marine macroalgae to produce biofuels may avoid competition for space between agriculture and terrestrial biofuel production (Fernand et al., 2017) but will potentially affect local primary productivity and derived ecosystem services, such as fish stocks and carbon sequestration, among others.
Global management of offshore industries
Global efforts to guide unified and up-to-date management of offshore industries are showcased through two UN bodies, the ISA that manages deep seabed mining in ABNJ and the FAO governance of commercial fishing (and many other activities outside of the focus here). Pelagic fisheries beyond EEZs are managed by Regional Fisheries Management Organizations/Agreements (RFMOs) and the Antarctic Treaty organization. There are 11 demersal fisheries RFMOs and five pelagic (mostly tuna) fisheries RFMOs. The Southwest Atlantic Ocean, Eastern Tropical Pacific, and Eastern Indian Ocean are among the few areas in the ocean that do not have a demersal RFMO. The RFMOs follow the FAO Guidelines for conducting impact assessments of deep-sea fisheries, identifying Vulnerable Marine Ecosystems (VMEs), and preventing significant adverse impacts (SAIs) on VMEs.
In the case of deep-sea fisheries, the FAO provides criteria to identify the potential risks/impact associated with the fishing activities in different types of ecosystems. There are specific criteria for identifying VMEs, eg. uniqueness, rarity, fragility, and structural complexity and typical VMEs include cold-water coral reefs, sponge grounds, or methane seeps. VMEs support high biodiversity, fish nurseries, and provide ecosystem functions that yield provisioning, supporting, and regulating services (Le et al., 2017; Montserrat et al., 2019; Ottaviani, 2020; Maier et al., 2021; Le et al., 2022). Significant Adverse Impacts (SAIs) are defined as impacts that alter the structure or function of the ecosystem in a permanent or long-lasting manner (e.g. Figure 3). The FAO Guidelines are ecosystem-based and identify six factors to consider in determining the scale and significance of an impact (e.g., intensity or severity, spatial extent relative to the availability of the affected habitat, sensitivity/vulnerability of the ecosystem, ability of an ecosystem to recover, alteration of ecosystem functions, timing and duration in relation to the life-history of the species). Most importantly, the FAO guidelines provide a temporal scale for assessing impacts. SAIs occur whenever the impact causes the ecosystem’s recovery to exceed 5-20 years, as the impact is no longer considered temporary. Other mitigation strategies for SAIs include applying an encounter protocol where there is a temporary closure for part of the fishery based on a bycatch threshold. The United Nations General Assembly resolutions and FAO guidelines have been adopted on paper, but the implementation and criteria of the impact assessments conducted by the RFMOs are often deemed deficient (Rogers and Gianni, 2010; Weaver et al., 2011; DOSI, 2022). In Box 3 we propose how the criteria for carrying out impact assessments from FAO, 2009 could be adapted to the O&G industry, with type of impacts on benthic habitats for each industry given in Figure 3).
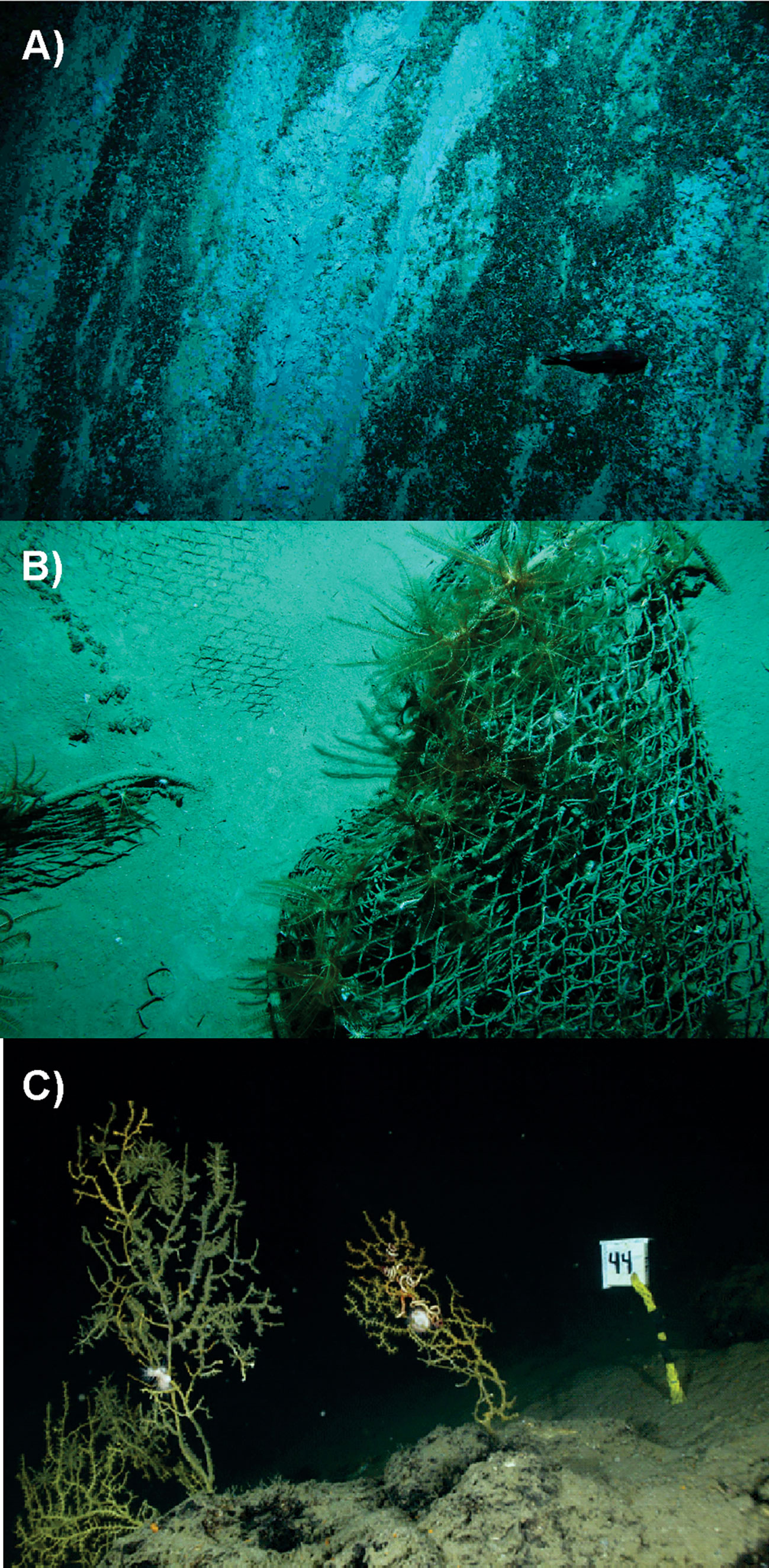
Figure 3 Destruction of benthic habitats derived from the activities of deep-sea fisheries and oil and gas. (A) trawled zone of the seabed. Photo courtesy of Malcom Clark. (B) lost net. Photo courtesy of Malcom Clark. (C) Paramuricea biscaya colonies showing varying degrees of impact from oil and the applied dispersants 18 months after the Deepwater Horizon blowout. Photo from the R/V Holiday Chouest expedition, Mississippi Canyon area, October 2011, courtesy of Erik Cordes.
Box 3. Criteria for carrying out impact assessments at different phases of development of oil and gas industrial activities. Adapted to the specific features of the offshore oil and gas industry from the criteria recommended by FAO (2009) for impact assessments in deep-sea fisheries, and supplemented with the focus on ecosystem services as presented here.
To assess if deep-sea oil and gas activities are likely to produce significant adverse impacts in a given area, impact assessments should include:
1. Assessment of the activities remaining in the development cycle:
a. Exploration phase:
i. Seismic surveys: type(s) of activities conducted or planned, including vessels and the features of airguns and hydrophone arrangements, acoustic signal features, area expected to be covered by the seismic pulse (including the vertical and lateral area in which it will be detectable above the ambient noise), efforts of seismic prospection and duration, seismic plan;
ii. Exploratory drilling: type(s) of activities conducted or planned, including vessels and features of the equipment used for drilling (indicating if it will be a vessel or a temporary platform), features of both the pipeline and the area expected to be covered by the sediment plume produced during drilling, high-resolution images of the seafloor from the target area, duration of the project and project plan;
b. Production phase: type(s) of activities conducted or planned, including vessels and the features of the platform, description of the amount, type and treatment of discharges, area covered by anchors and all the submersed infrastructure, efforts of production duration, production plan; spill risk and mitigation
c. Decommissioning phase: type(s) of activities conducted or planned, including vessels, area expected to be covered by sediment plumes and contaminants, description of infrastructure expected to be removed and the one that will be abandoned, duration and decommissioning plan;
2. Best available scientific and technical information on the current state of ecosystem services in the operation area, including baseline information of the distribution and abundance of ecosystems, habitats, communities, and species of economic interest along with the ecosystem services that they provide, against which future changes are to be compared;
3. Identification, description, and locations of key indicator species and VMEs known or likely to occur in the operation area;
4. Data and methods used to identify, describe, and assess the impacts of the activity, the identification of gaps in knowledge, and an evaluation of uncertainties in the information presented in the assessment;
5. Identification, description, and evaluation of the occurrence, scale, and duration of likely impacts, including cumulative impacts of activities covered on ecosystem services, VMEs, and indicator species in the operation area and the area of influence;
6. Risk assessment of likely impacts by the installations and/or operations to determine which impacts are likely to be significant adverse impacts on ecosystem services derived from the habitats, particularly impacts on VMEs; and
7. The proposed mitigation and management measures to be used to prevent significant adverse impacts on ecosystem services and ensure long-term conservation of VMEs and other resources, and the measures to be used to monitor effects of the installations and/or operations.
Commercial deep seabed mining (DSM) has not yet started on an industrial scale, but large areas are under exploration for DSM within national jurisdiction and in ABNJ or “the Area” (the seafloor in ABNJ). The ISA is the independent organization through which States that are Parties to UNCLOS organize, authorize, and control all mineral-resources-related activities in the Area for the benefit of humankind as a whole (www.isa.org). In so doing, the ISA has the mandate to ensure the effective protection of the marine environment from harmful effects that may arise from deep-seabed related activities (UNCLOS, Article 145).
Before any deep seabed mining exploration can start in the Area, exploitation regulations must be developed, and several requirements must be met by contractors, including the preparation of Environmental Impact Assessments (EIAs) (ISBA/25/LTC/6/Rev.1). At present, guidance for exploration EIAs has been developed and is now used for collector testing, but the EIA format for exploitation has not been finalized. The current draft of Environmental Impact Assessment guidance in the draft exploitation regulations (ISBA/25/C/WP.1) includes instructions to “address diversity, abundance, biomass, community-level analyses, connectivity, trophic relationships, resilience, ecosystem function and temporal variability”, plus work on ecosystem models and appropriate ecosystem indicators, but it does not directly mention ecosystem services. The specific draft standards and guidelines for the environmental impact assessment process (ISBA/27/C/4), however, prescribe consideration of the ecosystem services when assessing the significance of the impacts of the activities. The standards and guidelines for the resulting Environmental Impact Statement indicate that it must include a description of the services provided by the existing physicochemical and socioeconomic environments, however, there is no mention of the ecosystem services provided by the biological environment (ISBA/27/C/5).
Recommendations in the literature advice protecting rare and vulnerable hydrothermal vent habitats from mining impact (e.g., Van Dover et al., 2018; Sigwart et al., 2019; Gollner et al., 2021). In the latest draft, regional environmental management plan (REMP) for the northern Mid Atlantic Ridge, active vent fields are listed as “Sites in Need of Protection” (ISBA/27/C/38). To facilitate management, typical vent species such as Rimicaris shrimps or Riftia tube worms may be considered as VME indicators (Tunnicliffe et al., 2020). At abyssal plain sites targeted for mining of polymetallic nodules, a recently discovered sponge endemic to nodules has been suggested as an indicator (Lim et al., 2017). A REMP for the polymetallic nodules fields in the Clarion Clipperton Zone has been developed and has been recently updated (Seascape Consultants LTD, 2014).
Ecosystem services-based management of offshore energy
Protecting the value of biodiversity, ecosystems, and ecosystem services in the open ocean and deep sea requires a holistic approach that enables environmental management planning (Tunnicliffe et al., 2020). For example, Sala et al. (2021) proposed preserving biodiversity, food, and climate security as strategic goals for protecting the global ocean. They estimated that the most effective way to achieve these goals would be to combine the three objectives into a coordinated cross-sectoral global effort. Future management of offshore energy should include measures and indicators of ecosystem function and services to improve the conservation of the many critical organisms and processes that often go overlooked in environmental impact assessments.
Here we consider ecosystem services as the benefits to humans obtained from ecosystem processes, whether or not there exists a market for the benefits in question (Box 1, MA, 2005). There are different frameworks for environmental management that include ecosystem services, among which the Millennium Ecosystem Assessment (MA) has been a fundamental pillar. Other frameworks include Common International Classification of Ecosystem Services (CICES), The Economics of Ecosystems and Biodiversity (TEEB), and the Intergovernmental Platform on Biodiversity and Ecosystem Services (IPBES). MA has been widely applied to the management of terrestrial and freshwater environments but there are no equivalent efforts in the open ocean (Mejjad and Rovere, 2021). This management framework provides mechanisms for decision-makers to achieve sustainable development objectives, understand trade-offs and develop cost-effective policies in a better way (Thurber et al., 2014).
Growing research is focused at identifying and classifying deep-sea ecosystem services (e.g., Thurber et al., 2014; Orcutt et al., 2020), quantifying ecosystem services based on functional traits (Le et al., 2022), assessing them in relation to industrial activities (e.g., Mejjad and Rovere, 2021), valuation of services (Ottaviani, 2020), and the communication of this new scientific understanding to decision makers (e.g., Jobstvogt et al., 2014; Teneva et al., 2022). Assessing ecosystem services improves, and in some ways simplifies, the understanding of the mechanisms behind any change and allows their economic valuation (Thurber et al., 2014; Le et al., 2017; Mejjad and Rovere, 2021), providing a more realistic understanding of the environmental and social costs of exploiting deep-ocean resources. Moreover, impacts on key components of an ecosystem that may have low resilience (i.e., sensitivity to direct impact, long recovery times, etc.) jeopardizes the derivation of the direct and indirect benefits of the services they support. Thus, assessing the industrial impacts on ecosystem services will also help to fill basic knowledge gaps (Thurber et al., 2014; Le et al., 2017; Mejjad and Rovere, 2021; Amon et al., 2022). This assessment requires adopting the premise that we cannot measure everything, nor do we need to, but rather that we should set data priorities to add feasibility, and recognize that any approach will be improved as further research on deep-sea ecosystem services is available.
Diversity, abundance, distribution, and interaction (within and between) species and habitats are factors that determine the (dynamic) structure of ecosystems (Thurber et al., 2014; Sommer et al., 2019, Figure 4). The integrated analysis of these components through statistical modeling promotes an understanding of their ecological functions (Sommer et al., 2019). Once these links are established, the assessment and monitoring of species abundance can be explicitly tied to an outcome in terms of a quantifiable ecosystem service. The identification and measurement of these indicators can improve the information quality of environmental baseline studies.
Ecosystem service indicators can vary by region and habitat type, but many of these are consistent and can be constrained and generalized. In non-chemosynthetic systems, more diverse communities generally have higher rates of ecosystem function and therefore provide more robust ecosystem services. However, at methane seeps, highest function is not always associated with higher diversity (Ashford et al., 2021a), which emphasizes the need to assess ecosystem services on a site-specific basis. A more detailed approach is suggested for studies of species of particular interest given their conservation value, such as mammals, or species of commercial value, such as certain fishes (e.g., billfishes, tunas). Ecological function of individual species can often be determined using biological traits analysis (BTA), which can provide information on ecological function and predict ecosystem services related to the species present in the area (Bremner et al., 2006, Levin et al., 2017; Bravo et al., 2021; Le et al., 2022).
For the deep seabed, the importance of microbial taxa (i.e., prokaryotes and protists) and meiofauna cannot be overstated (Orcutt et al., 2020). Microbial deep-sea biomass represents 10-30% of all biomass on Earth (Corinaldesi, 2015), meiofauna dominate metazoan biomass and diversity below 3,000 m (Rex et al., 2006; Wei et al., 2010; Schratzberger and Ingels, 2018), and protists are known to be important members of deep-sea food webs and carbon sequestration (Gooday et al., 1992; Gooday, 1993). Deep-sea benthic metabolism is largely driven by microbial taxa (Turley, 2000; Glud, 2008), and small organisms therefore play key roles in deep-sea ecosystem functions (Nascimento et al., 2012; Bonaglia et al., 2014). While large, charismatic fauna may form the basis of habitat mapping and protection (Danovaro et al., 2020; Bribiesca-Contreras et al., 2022), efficient monitoring and conservation action necessitates detecting early changes through indicator species at the base of the food web and ecosystem functions (Ingels et al., 2021). Indeed, those species are well suited for monitoring change in ecosystems worldwide (Schratzberger and Ingels, 2018; Ingels et al., 2021). Baseline characterizations must therefore also consider microbial and meiofaunal taxa to ensure holistic and comprehensive management of ecosystem services.
Approaches should consider connectivity and interactions with other populations, species, and habitats (including and throughout the water column) as key indicators of ecosystem health and services. Connectivity plays key roles in structuring and supporting the ecosystem services from the deep sea. For example, the connectivity between chemosynthetic ecosystems and background areas through transition zones and/or chemotones often concentrate a high quantity of ecosystem services (Figure 5, Levin et al., 2016; Ashford et al., 2021a, Ashford et al., 2021b, Gollner et al., 2021). In this instance, a lesson can be taken from the management of fisheries in the U.S., where Essential Fish Habitat (EFH) is managed in addition to the direct management of fish stocks (Moore et al., 2016) to ensure connectivity among habitats utilized by different life stages (Peterson et al., 2020).
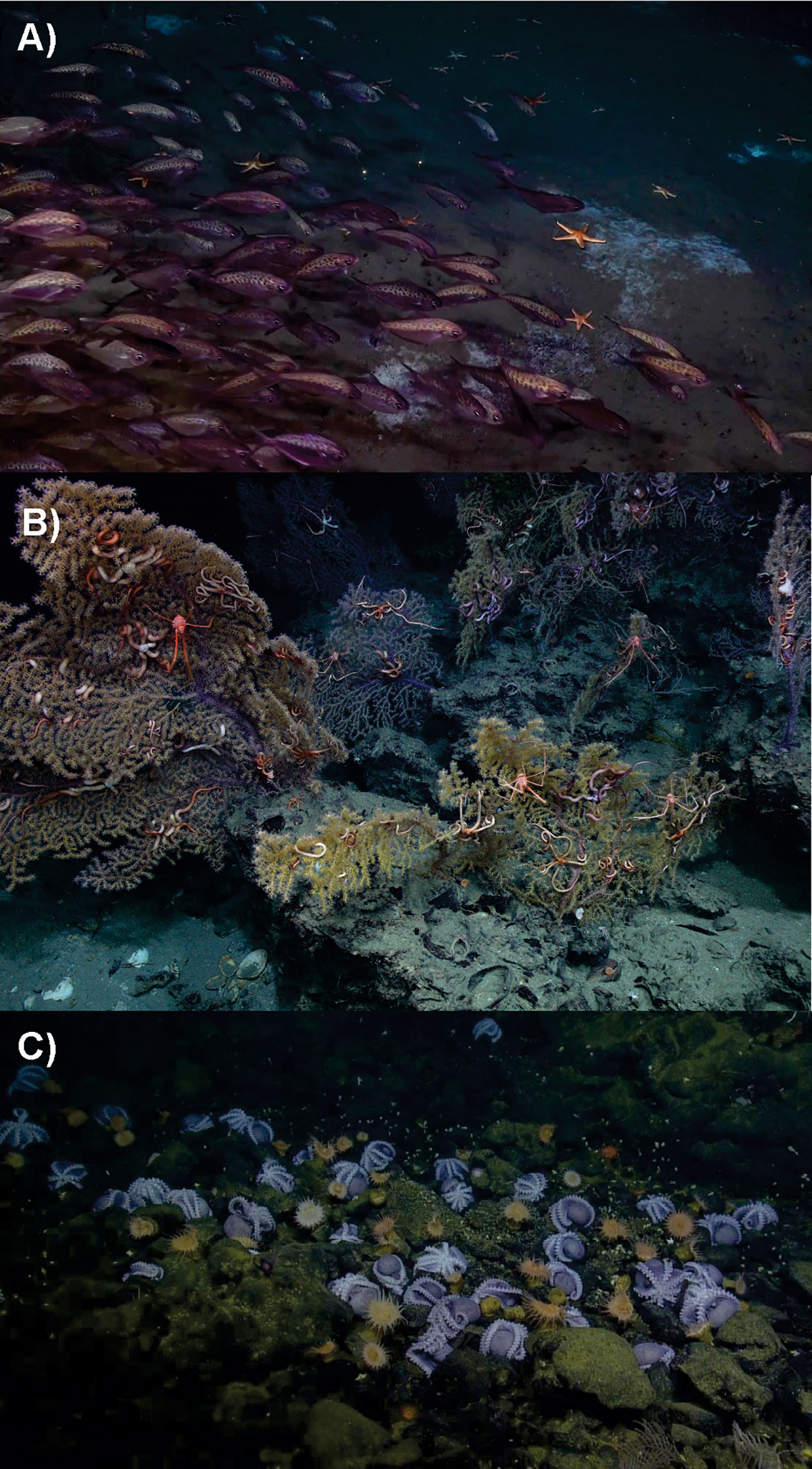
Figure 5 Connectivity between chemosynthetic ecosystems and background deep sea ecosystems. (A) A methane seep transition zone offshore North Carolina, USA. White bacterial mats indicate areas of active seepage, while the American butterfish (Peprilus triacanthus) and seastars are background deep-sea species visiting the seeps to take advantage of the chemosynthetic productivity. Image courtesy of Ivan Hürzeler, DEEP SEARCH project, and copyright Woods Hole Oceanographic Institution. (B) Seep transition zone with Paramuricea coral fans (like those impacted by the Deepwater Horizon oil spill) using substrate provided by seep-related carbonate rocks with embedded chemosynthetic clam shells. Image courtesy of Erik Cordes, ECOGIG consortium, and the Ocean Exploration Trust. (C) Extensive aggregations of brooding female octopuses (Muusoctopus robustus), “octopus garden”, associated with vents from Monterey Bay National Marine Sanctuary. Image courtesy of OCEAN EXPLORATION TRUST/NOAA.
Environmental management policies for offshore industries can optimize the design of environmental management plans (monitoring programs, activity management, temporal management, spatial management) and EIA requirements by incorporating the analysis of ecosystem service indicators. Integrating ecosystem services into existing management frameworks for well-studied ecosystems or processes would require limited efforts to fill specific knowledge gaps, while for less studied areas it would need programs to generate broad basic knowledge (Sommer et al., 2019). The data required are often currently available in well-studied areas but need to be adapted to the temporal and spatial scales of each management decision. This framework also addresses long-term climate change by assessing the climate-regulating services, but additional assessments of the effects on the distribution of heat and carbon in the ocean resulting from changes in ecosystem structure, including microbial and planktonic organisms, should be conducted.
Mapping deep-sea biota associated with benthic and water-column habitats is key to understanding the spatial distribution and connectivity of ecosystems and evolutionary processes (Danovaro et al., 2017; Glover et al., 2018; Danovaro et al., 2020; Swanborn et al., 2022). The extent, geographic range, and ecological functioning of deep-sea benthic habitats remain poorly understood (Brown et al., 2011). Even at the most basic level, only an estimated 20% of the seafloor has been mapped at resolutions similar to terrestrial surveys (Coley, 2022). In addition, most benthic ecology studies are conducted with “over-the-side” sampling gear without direct visualization of the seafloor, which limits the spatial coverage of their results (Bravo et al., 2020). Consequently, there is an urgent need to develop robust marine ecosystem mapping and visual survey methods for effective spatial seafloor management (Brown et al., 2011; Danovaro et al., 2020).
The deep-pelagic environments are not well studied, even less so than the benthic environments, with greatest attention on fishes and other conspicuous compartments (Webb et al., 2010). The deep pelagic is the largest ecosystem on Earth, harboring over 90% of the global biosphere with a unique biodiversity (Robison, 2009). It plays key roles in carbon export (Davison et al., 2013; Steinberg and Landry, 2017; Boyd et al., 2019), nutrient regeneration, and vertically connects epipelagic and deeper ecosystems through trophic interactions, which include providing resources for targeted fishes and protected species such as cetaceans and seabirds (Trueman et al., 2014; Drazen and Sutton, 2017; Torres and Bailey, 2022). However, the species/population perspective of environmental management often is focused primarily on fishes of commercial interest and/or mammalian megafauna (e.g., cetaceans, pinnipeds), neglecting the other compartments of the pelagic ecosystems that generate ecosystem services (e.g., plankton, deep-living nekton).
Environmental baseline studies for planktonic and micronektonic organisms from pelagic environments often do not exist in industry, although they may experience negative effects from their associated activities (e.g., Copping et al., 2020; Drazen et al., 2020; Daly et al., 2021; Sutton et al., 2022), and many gaps regarding the potential risks of impacts are not well characterized (Copping et al., 2020; Amon et al., 2022). Biogeographic ecoregions in the midwater tend to be larger than those of benthic ecoregions, because pelagic animals may move over 100s of kilometers and ocean currents may transport animals over equally long distances (Sutton et al., 2017). At the mesoscale, vertical migration is a dispersive mechanism, with transport in one direction during daytime, and the opposite direction during nighttime. These factors mean that impacts to midwater communities have the potential to spread far (Sutton et al., 2022). The awareness of large-scale changes in midwater pelagic environments driven by offshore industries is growing, with pioneering recommendations for its inclusion in the contractor’s seabed mining EIAs by the International Seabed Authority. For example, in the draft guidelines for the preparation of environmental impact statements (ISBA/27/C/5), it is recommended that the EIA includes a description of the midwater community (200 m depth to 50 m above the seafloor), describing the diversity, abundance, biomass, connectivity, trophic relationships, resilience, ecosystem functioning, and temporal variability for microbes, zooplankton, meso- and bathypelagic fishes, and deep-diving mammals across depth. Such an inclusion in the EIA should be adopted by other offshore industries, too.
The study of the water column should be carried out in an integrated manner using, as far as possible, a multi-modal approach (water column sensing and sampling with multiple gear types) that allows mapping the distribution and abundance of the different components of the ecosystems, and this should be carried out over time for long-term monitoring. The provision of habitat for shelter, breeding grounds, and nurseries is a key ecosystem service provided by a variety of habitats. To extend this concept, many species undergo ontogenetic migrations where they utilize numerous habitats over the course of their development from larvae to reproductive adults (Sutton, 2013; Longmore et al., 2014). This is a key ecosystem service of deep-sea habitats and these “connectivity corridors” should also be conserved to ensure the proper management of species providing provisioning services (Peterson et al., 2020). Finally, pelagic environments and their biological communities must be considered for holistic management across the entire biogeographic area, especially when impacts can extend from the seabed into the water column.
New technologies for improved impact assessment and monitoring
New technologies (Figure 6) have resulted in unexpected discoveries being made in the past few years, challenging well-accepted hypotheses of deep-sea geology and biology, and improving holistic views of deep-sea ecosystem services. For example, studies have found higher than expected geodiversity in Atlantic abyssal plains (Riehl et al., 2020) and unexpected ice-fish breeding grounds in the southern ocean (Purser et al., 2022) thanks to imagery systems like the Ocean Floor Observation and Bathymetry System (OFOBS), which are able to cover wide geographical ranges at depth (Piepenburg et al., 2017). Increasing interest in deep-sea resources calls for the development of novel monitoring technologies, to go beyond time-consuming and high-cost vessel-based surveys. To ensure effective management and protection actions, spatiotemporally extended monitoring strategies must be implemented, which allow environmental (physicochemical) and biodiversity data to be gathered in a high-throughput and parallel manner.
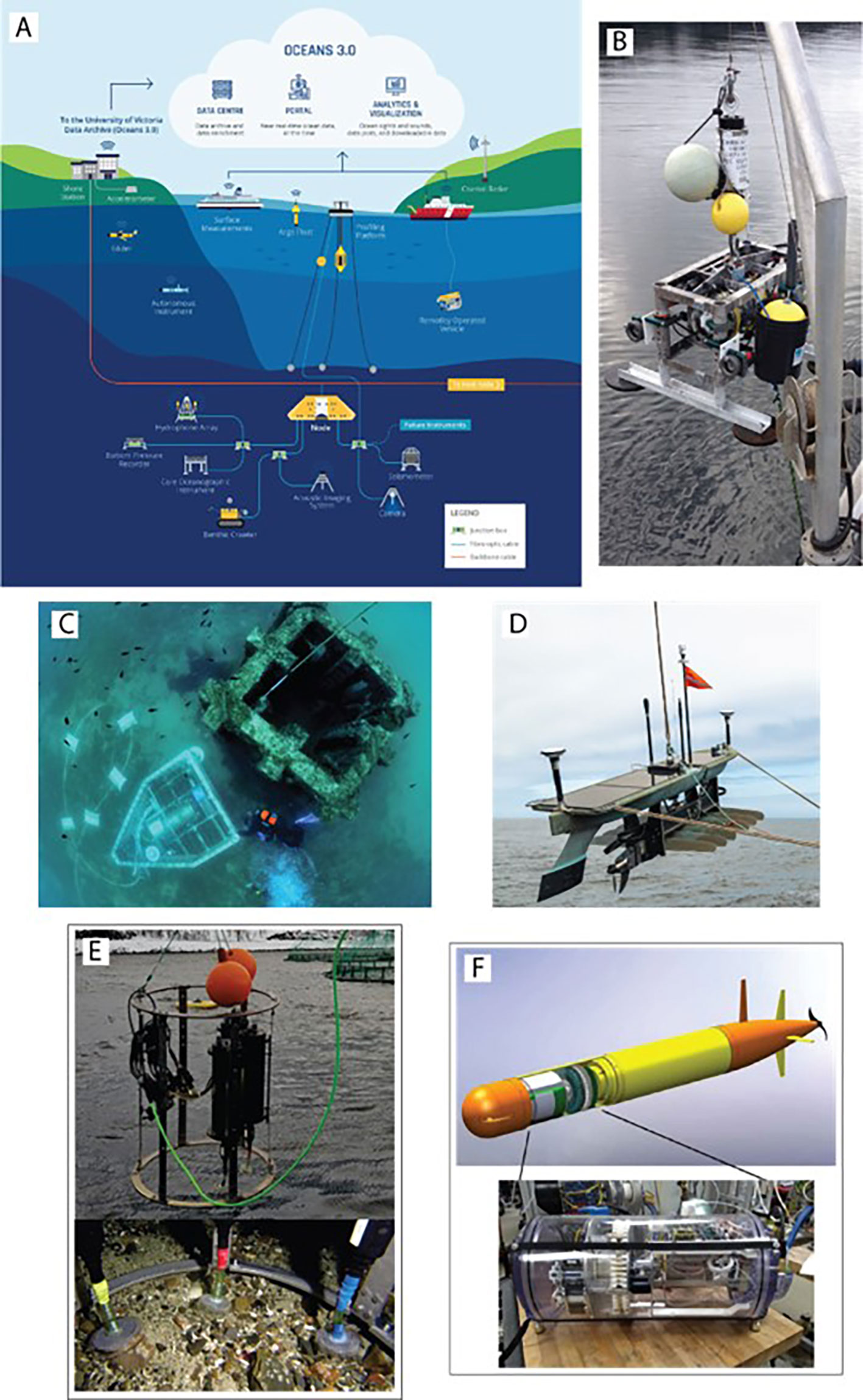
Figure 6 Ocean observation opportunities through new technologies. (A) An example of a high-tech platform combining cabled, docked, and autonomous devices as designed by Ocean Networks Canada (ONC); (B, C) Cabled observatories deployed on the seafloor in Canada (ONC) and Spain (adapted from Del Río et al., 2020; OBSEA) respectively; (D) A GPS-equipped glider for gathering oceanographic and pelagic data deployed by ONC; (E) The substrate-independent benthic sampler, a prototype of a semi-autonomous benthic eDNA sampling device (from Keeley et al., 2021), and (F) the Environmental Sample Processor for autonomous eDNA sampling of the water column (Images by Monterey Bay Aquarium Research Institute).
High-resolution, satellite-like images of the seafloor can be obtained using a combination of acoustic methods (deep and shallow seismic, side-scan sonar systems, single-and multibeam echosounders). Advances in synthetic aperture sonar (Thorsnes and Chand, 2022) and deployment of multibeam echosounders (MBES) on submergence assets (ROVs, AUVs, human-occupied vehicles or HOVs) have brought the ability to map the seafloor to even higher resolution. High-resolution bathymetry is the basis for benthic habitat mapping and seascape characterization, which can be used for ecosystem management (Brown et al., 2011; Bravo et al., 2020; Danovaro et al., 2020; Swanborn et al., 2022). These benthic habitat maps are a very important and widespread tool for predicting the spatial distribution of habitats suitable for settlement of vulnerable marine ecosystems (VMEs; FAO, 2009). They can also identify the suitability of habitat for structural elements that generate ecosystem services (Morato et al., 2020; Le et al., 2022).
However, there are examples of ecosystems whose boundaries cannot always be detected using seafloor-surface acoustic imaging. Chemosynthetic ecosystems associated with methane gas seeps are common in areas of O&G development, but the methane bubbles from seeps remain trapped in subsurface sediments and these can only be detected by high-resolution seismic (acoustic turbidity) data or by direct geochemical measurements on the ocean floor (Bravo et al., 2020; Bravo et al., 2021b). The seep biological community provides a significant ecosystem service of oxidizing the methane before it is released into the water column and potentially the atmosphere where it can be a powerful greenhouse gas (Marlow et al., 2014; Cavicchioli et al., 2019). Thus, the acoustic turbidity could be used to map the area covered by these ecosystems and target the area that needs direct sampling (Bravo et al., 2020; Bravo et al., 2021b) to assess their sphere of influence on surrounding ecosystems and ecosystem services, both in the overlying water and on the surrounding seafloor (Levin et al., 2016; DOSI 2021b). Similarly, the infaunal community surrounding cold-water coral reefs is significantly different than the community further away in terms of biodiversity and the functional traits that define ecosystem services (Demopoulos et al., 2018). Advances in extracting ecosystem-service information from seafloor imagery (e.g., Le et al., 2022) will facilitate incorporation of ecosystem-service mapping into management practices. The broad ecosystem-service boundaries of these habitats need to be incorporated in EIAs, baseline studies, and monitoring (Levin et al., 2016; DOSI 2021b).
Species distribution and predictive habitat modeling have advanced to the point where they can provide high-resolution and accurate predictions for management prioritization using primarily remote sensing data (e.g. Iacono et al., 2018; Georgian et al., 2020; Melo-Merino et al., 2020). This greatly decreases the cost of conducting an assessment, assuming there are existing species distribution data on which to base the models, and can greatly increase the area over which biological data can be generated. These methods are frequently used for single-taxon prediction, which can be applied to indicator taxa for designated VMEs or, in the scenario presented here, ecosystem services. Predictive habitat models have now progressed to allow for ensemble modeling of entire community or habitat types (Mata et al., 2020; Uhlenkott et al., 2021) and for projecting their response to changing conditions (Morato et al., 2020; Gasbarro et al., 2022).
Optoacoustic technologies (high-definition still and video image data coupled with active acoustic imaging, i.e., sonar) represent key techniques for the observation of deep-sea habitats and biological behaviors, allowing visualization and identification (to some degree) of the mega- and macrofauna at wider geographic scales. A current drawback is the computational capacities needed to process the huge amounts of imaging data, but the ability for machine learning algorithms to automatically detect and quantify key fauna is improving. While these algorithms require ecosystem-specific training and calibration, which can initially include direct sampling of fauna, they offer major opportunities for decreasing throughput time, reducing observer bias, and limiting the impacts of the surveys themselves (Aguzzi et al., 2019; Aguzzi et al., 2020; Stefanni et al., 2022). Although imaging has mostly been used to detect and identify fauna > 1 cm in size, new modeling approaches based on physically collected in situ samples are being developed to characterize temporal and spatial variability of smaller organisms (Stefanni et al., 2022).
The latest progress in robotics have allowed for the development of fixed (cabled), docked (tethered and untethered crawlers and rovers), and autonomous platforms as cross-disciplinary infrastructures, providing complementary and long-term monitoring data in the benthic and pelagic realms (Aguzzi et al., 2019; Aguzzi et al., 2020; Stefanni et al., 2022). Such platforms can be equipped with optical, biogeochemical, oceanographic, and acoustic biological sensors, enabling continuous and long-term (up to decades) monitoring of remote seafloor locations (Aguzzi et al., 2019). To complement benthic monitoring, the platforms can also be equipped with tethered Remotely Operated Vehicles (ROVs) and free-swimming Autonomous Underwater Vehicles (AUVs) to allow monitoring of the water column. The deployment of such observatory modules in clusters can allow expanding surveys from local- to ecosystem-level geographic scales, and successful implementations have already taken place in the North Pacific and Atlantic Oceans, and in the Mediterranean Sea (Stefanni et al., 2022). Similarly, high-tech sensors can be fitted on existing fiber optic telecommunications cables, which would increase ocean observing capacities to a global level (Howe et al., 2019).
Imaging does not always provide enough taxonomic resolution, and physical collection of voucher specimens is required to describe new taxa and validate species identification. Moreover, imaging monitoring strategies such as video transects or Baited Remote Underwater Video (BRUV) may result in biased data due to various avoidance or attraction behaviors (Stefanni et al., 2022). There will always be a need for integrative taxonomy, which couples morphological and genetic data, in deep-sea research and environmental assessments (Glover et al., 2015; Lins et al., 2021), and the capacity for this area of research needs to be continually developed globally.
Genetic identification can also be achieved from environmental samples (water, sediment, air) using environmental DNA (eDNA) metabarcoding, which is the high-throughput sequencing of genetic barcodes in DNA directly extracted from environmental samples (Taberlet et al., 2012). eDNA metabarcoding enables non-invasive and high throughput sampling, and allows the description of whole communities, from prokaryotes to metazoans, including the detection of “hidden” (rare, cryptic) diversity. A major limitation of this technique in the deep sea is the general lack of taxonomic knowledge resulting in incomplete reference databases for identifying the barcode sequences (Hestetun et al., 2020). Interpretation of the results of eDNA sequencing is also hampered by an incomplete knowledge of degradation rates under different environmental conditions in the deep sea (McCartin et al., 2022). Accurate voucher specimen provision/validation will likely remain a limitation in deep-sea metabarcoding strategies, although ecological status can be assessed from metabarcode data using taxonomy-free approaches to construct de novo biotic indices via the identification of indicator taxa (Lanzén et al., 2021a, Lanzén et al., 2021b, Mauffrey et al., 2021) or through supervised machine learning (Cordier et al., 2018, Lanzén et al., 2021b). Going beyond biotic indices, environmental genomics can also be applied to infer structural (e.g., ecological networks, phylogenetic structures) and functional (e.g., meta-genomics and transcriptomics) community metrics, making eDNA data applicable in ecosystem service-based monitoring frameworks (Cordier et al., 2018).
Combining imaging-based monitoring with eDNA has already been successfully showcased in the water column (Stat et al., 2019; Easson et al., 2020), and on cabled observatories (Mirimin et al., 2021).
These proof-of-concept studies mostly involved visually conspicuous taxa (fish and micronekton) and all sample collection steps were carried out manually. Practical high-throughput implementation will thus require the integration and development of automated eDNA sampling devices adapted to operate on ROVs or AUVs. Such “eco-genomic” sensors are already in development (Aguzzi et al., 2019), and examples include the environmental sample processor (ESP) for the water column (Yamahara et al., 2019), and the substrate-independent benthic sampler (SIBS) for the seafloor (Keeley et al., 2021). Increasing the level of automation and taxonomic resolution of bioimaging sensors will improve the quantitative aspect of offshore ecological monitoring, and greatly expand our taxonomic knowledge in the deep sea, which are both fundamental for implementing evidence-based conservation (Sutherland et al., 2004).
Improving the global capacity for offshore management
The notion of “energy justice” includes the equal opportunity for all individuals to have both access to decision-making processes around energy and to energy that is sustainable, safe, affordable, and sustains a decent lifestyle (SDG 7 “Affordable and Clean Energy”, Carley & Konisky, 2020). The sustainable aspect of this concept links it with the concept of “just transition”, which is aimed at pursuing equity and justice toward planning, implementation and assessment of every social-energy system change related with the energy transition (Carley & Konisky, 2020).
Renewables, and wind power in particular, continue to grow offshore (e.g., 30% annual growth between 2010 and 2018; International Energy Agency 2019) but disproportionately among different EEZs (Novaglio et al., 2022). Growth in offshore wind is mainly concentrated in the UK, EU and China while other regions have been slow to adopt renewables, resulting in uneven efforts and suboptimal benefits in terms of de-carbonization (Novaglio et al., 2022). Several countries do not adopt offshore wind because of the challenges posed by the grid capacity to sustain the growth of power generation or because the supply chain is not established yet (Poulsen and Lema, 2017; Novaglio et al., 2022). Furthermore, Low and Middle-Income countries cannot access the necessary means for the exploitation, or even the exploration, of their offshore renewable energy resources.
Science plays a key role in overcoming the gaps in knowledge offshore, in terms of both environmental data and technological innovation, that challenge the fulfillment of requirements for the energy transition and its effective management. Efforts aimed at the sustainable development of offshore energy need to integrate measures that facilitate equal and fair access to environmental data to inform and monitor the planning, implementation, and development of energy policies. Framed in justice and equity principles, this implies that access to the environmental data, the latest technologies, and the “know-how” is not relegated to the more economically powerful sectors of industry, politics, and science. However, a series of actions may help to overcome this problem: science-industry agreements, capacity building, and transparency.
Science-industry agreements
New areas of offshore O&G development are appearing in Low-/Middle-Income countries, which have limited capacity for expensive offshore research. In these areas, the O&G industry is supplying the vessels and vehicles required for the baseline characterization of the deep ocean, but often the data are not ending up in the hands of local researchers who would most benefit from them. The governing bodies of the EEZs where this activity is taking place must require the public deposition of the available data. Even if some data, such as the sub-surface profiles from the seismic data, remain proprietary, we suggest that the video/photographic surveys, biological and geological samples, and bathymetric data be made easily accessible and in appropriate formats for the scientific community to develop the robust baselines required to detect potential ecosystem-level impacts. This is especially important where there is limited capacity for offshore research, and where resource exploitation is occurring prior to scientific exploration (Cordes & Levin, 2018).
While ocean-based energy in wealthy, developed nations is moving on from O&G extraction to renewables such as offshore wind, many Low-/Middle-Income countries are just beginning to explore their deep-water fossil fuel resources with the intent to extract them. This is often done with a dearth of baseline information about seafloor or water column habitats and environments. Examples of this are present in South America (Argentina, Brazil, Guyana) and Africa (Kenya, Ghana, Namibia, South Africa). Early activities often involve exploratory cruises by the industries to provide baseline characterization and can act to build capacity, providing regional scientists with unique opportunities for bathymetric, hydrographic, biodiversity (taxonomic and genetic), and resource characterizations. Often, findings can stimulate research on new ecosystems (e.g. methane seep discovery) or taxa. For example, off-Argentina, an agreement between the local oil industry (YPF S.A.) and the National Scientific and Technical Research Council (CONICET) covers funding for geological instrumentation and partially covered the cost of six research cruises (undertaken from 2017 to 2022 on board the R/V Austral-CONICET) with permission for the scientists to publish the environmental data acquired.
Capacity building
A major new source of deep-sea access has come through philanthropic entities that have developed research vessels with capabilities for deep-sea exploration. Often these offer survey, sampling and telepresence opportunities, broadcasting real time deep-sea imagery from the ROV feed onto the internet for public consumption. Examples include assets of Ocean Exploration Trust (RV Nautilus and ROV Hercules), the Schmidt Ocean Institute (RV Falkor and ROV Subastian), Victor Vescovo’s Five Deeps operation and REV Ocean. Although deep-seabed mining has captured the attention of a number of these, with some cruises taking place in areas targeted for mining exploration, offshore energy development has not received as much attention. Focused research could inform decision-making by examining ecosystem attributes in areas targeted for future energy development, as well as by assessing impacts of offshore wind turbines, O&G infrastructure, or accidents (blowouts or spills) on the deep-sea environment, ecosystem services, or other human uses of the region.
Additional new knowledge may come through the UN Decade for Ocean Sciences for Sustainable Development. The UN Decade promotes science to achieve a healthy, resilient, productive, predicted, safe and accessible, inspiring and engaging ocean. Deep-ocean science emerging from the energy industry can potentially develop in multiple ways. A number of programs and projects focus on deep-sea exploration and coordinated observing (Deep Ocean Observing Strategy; Challenger 150, JETZON, One Ocean Network for Deep Observation, Crustal Ocean Biosphere Research Accelerator - COBRA) or new technologies (Ocean Biomolecular-Observing Network - OBON), but they do not have a clear focus on offshore energy.
Transparency
Much of the environmental data, innovation in research and technology is not shared and it is concentrated in a small number of countries and profitable companies and investors (Novaglio et al., 2022). A large amount of the deep sea environmental information and cutting-edge technologies available to humankind is owned by the O&G industry (obtained during exploration, exploitation, EIAs, etc.). However, much of this information is not accessible to the scientific and public community. Even if it is housed at government facilities; it is confidential information. Accessing the information that industries obtained since the 1970s would reduce costs for informing stakeholders of various management scenarios, including conservation initiatives as well as industrial development (Murray et al., 2018). At the same time, it would build trust in both the political and industrial sectors (Murray et al., 2018).
Key to the generation of scientifically robust baselines to inform the management of industrial activities is improving the accessibility of all deep-sea research efforts that occur globally. Many scientific data are published in open access repositories (for example on the Pangaea platform, or genbank), but datasets are often not comparable or difficult to find. The new global deep-sea biological research program ‘Challenger 150,’ provides a framework for international collaboration and cooperation among the scientific community to share knowledge, technology and establish standards for the application of knowledge to deep ocean management (Howell et al., 2020; Howell et al., 2021).
Conclusions
In this article, we propose a framework for ecosystem services-based management of the offshore energy industry. New technological solutions position the deep ocean as a promising frontier for the expansion and transition of energy industries (both O&G and marine renewables). For the deep-ocean energy industry to be aligned with SDGs 7, 9, 10, 12, 13, 14 and 17 there are still multiple challenges. We believe that a number of priority actions provide opportunities to overcome these challenges: 1) increase research for the development of both competitive and environmentally benign offshore renewable-energy technologies, considering, in addition to the requirement of low GHG emissions, their associated impacts on ecosystem services (SDG 9); 2) incorporate the measurement of ecosystem services into management strategies for industrial development in the deep ocean that draws on new interdisciplinary scientific approaches, data, and technologies available; and 3) strengthen international agreements and collaborations across sectors (science, decision makers, industry) and build capacity (SDG17) for fair and equitable access (SDG 10) to energy resources (SDG 7) as well as the other services and benefits derived from deep-ocean ecosystems (SDG 14). The transition of our energy systems and the effective management of ecosystem services are key to responding to the short timeframes involved in reaching the target of 1.5°C average global temperature increase by 2050 (SDG 13) (Box 4).
Box 4. Summary of best practices for environmental impact assessment (EIA) including data, technology and strategies.
The environmental data must address the impact on deep-sea essential ecological variables:
● What are the components of ecosystems that play key roles on ecosystem functions and services, how they relate with species, how they vary across space and time? Consider:
1. Type of habitats involved in provisioning services: sustaining high biodiversity and biomass of living resources, novel genetic resources, mineral and/or energy resources.
2. Habitats, species, communities involved in regulating services: regulation of climate, biogeochemical processes, detoxification, sequestration and cycling of pollutants.
3. Habitats, species, communities involved in supporting services (e.g., fish stock)
4. Habitats, species, communities involved in cultural services: traditional knowledge, art, technology, religion, science, etc.
● Biodiversity (species and genetic diversity, rarity, endemism, evolutionary processes, ecosystem redundancy)
● Connectivity (genetic, spatio-temporal, the ecotones and chemotones, between eco-regions, interspecific relations e.g., trophic links)
● Climate risk (how impacts may affect adaptive and evolution capacity to climate change -considering increasing temperature, decrease in oxygen, increasing acidification)?
● Synergetic pressure (what are the cumulative impacts for the specific area - fisheries, contamination, climate change, others? how do impacts affect the resilience ability of the habitat, species, community)?.
The required technology includes:
● multibeam echosounder bathymetry of the potential impact area (beyond the activity area)
● high-definition video and photo surveys of the seafloor using ROV/AUV/HOV
● physical samples of biota and sediments
● skilled taxonomists and specialists from other basic disciplines of marine sciences to calibrate, guide, and supervise the data acquired
Additional recommended technologies:
● high-definition optoacoustic imaging (sonar and video)
● eDNA metabarcoding– de novo biotic indices, structural community metrics
● metagenomics/metatranscriptomics- functional community metrics
● Robotics (cabled, docked and autonomous observatories)
● eco-genomic sensors (automated eDNA samplers)
● high resolution acoustic tools (3,5 kHz sub-bottom profilers, side scan sonars, vehicle mounted multibeam echosounders) for benthic and pelagic habitat mapping
Recommended strategies:
● Ecosystem-based recommendations by FAO and ISA addressing direct physical impact of the seabed could be applicable to those from offshore energies. Integration of management strategies for mining, deep-sea fisheries, and offshore energies.
● Harmonized and standardized management strategies and efforts for offshore industries across EEZs.
● Climate-based MPA design should be considered for exclusion of areas for control (monitoring) and/or conservation from the operation areas of offshore energy industries.
● Science-stakeholders (politics and industry) agreements to inform management policies (including but not limited to EIAs).
● Capacity building initiatives through industry-science-politics agreements, global scientific networks, and philanthropy for accessing cruises, environmental data, technologies, open access publications, etc.
● Transparency (improving accessibility to environmental data owned by the industry).
We recognize that there are many knowledge gaps about deep-sea ecosystem services (Mejjad and Rovere, 2021). But, now, after 100 years of deep-sea research, it is clearly understood that these systems are key for the well-being of both human society and Earth itself (Thurber et al., 2014; Mejjad and Rovere, 2021) and that preserving their services requires managing them directly and effectively. There is an urgent need to understand deep-sea ecosystem services and to integrate them into the scientific research, technology development, and management policies involved in the continued and expanded exploitation of energy resources in the deep ocean. We believe that overcoming this challenge is feasible by integrating new knowledge, data, and technologies in conjunction with collaborations across scientific networks, and from different sectors (scientific, policy makers, and industry). Firstly, this can help in understanding the relationship between the costs and the benefits of exploiting deep-sea resources (Thurber et al., 2014) and in this case, energy resources (whether renewable or O&G). Secondly, it can strengthen equitable and fair access to the sustainable use of deep-sea energy resources. The implementation of a framework based on ecosystem services will enable better informed decision-making on the exploitation of deep-sea energy resources.
Summary of suggested priority actions
We offer the following recommendations to integrate deep water O&G management with a more holistic view and stewardship of the ocean.
● Adopt an ecosystem services-based management framework for the offshore oil and gas industry built on an understanding of how ecosystem structure and function support the services that maintain health of marine life and human populations.
● Advance new technologies (such as eDNA and robotics) that enable and can expand baseline assessment and monitoring of deep-sea ecosystems, their functions and services.
● Build intellectual and infrastructure capacity among all nations to employ advanced technologies and tools to advance the incorporation of ecosystem services into ecosystem-based management strategies.
● Share knowledge and best practices of UN bodies governing other international industries with the offshore energy industry to improve the standardization of impact assessment, spatial protections, monitoring, transparency, and stakeholder engagement.
● Harmonize the activities of UN and regional management bodies across deep-sea realms (sea floor, water column) and resource extraction activities (energy, mining, fishing) and stressors (pollution, climate) to achieve sustainable development goals and ocean sustainability.
Author contributions
MEB, MIB, and EC wrote, edited and revised the text and figures. All authors contributed to the text and figures. All authors read, edited and approved the final manuscript.
Acknowledgments
We thank M. Clark for the pictures of deep-sea fisheries used in Figure 3. We also thank the two reviewers and the editor for the valuable comments that improved our manuscript. Publication of this article was funded in part by the Temple University Libraries Open Access Publishing Fund, and EEC would like to thank Lydia Spitzer for her generous support that went towards the remaining charges associated with this open-access publication. PE acknowledges financial support to CESAM by FCT/MCTES (UIDP/50017/2020+UIDB/50017/2020+ LA/P/0094/2020), through national funds. PE was funded through FCT in the scope of the framework contract foreseen in the numbers 4, 5 and 6 of the Article 23 of the Decree-Law 57/2016, of August 29, changed by Law 57/2017, of July 19. DOJ was supported by United Kingdom Natural Environment Research Council (NERC) grant NE/T010649/1 “Autonomous Techniques for anthropogenic Structure Ecological Assessment (AT-SEA)” and NE/T003537/1 “Seabed Mining And Resilience To Experimental impact”. LV was supported by the Norwegian Research Council project number 287934. This manuscript is a product of the Deep-Ocean Stewardship Initiative Offshore Energy Working Group.
Conflict of interest
The authors declare that the research was conducted in the absence of any commercial or financial relationships that could be construed as a potential conflict of interest.
Publisher’s note
All claims expressed in this article are solely those of the authors and do not necessarily represent those of their affiliated organizations, or those of the publisher, the editors and the reviewers. Any product that may be evaluated in this article, or claim that may be made by its manufacturer, is not guaranteed or endorsed by the publisher.
References
Aguzzi J., Albiez J., Flögel S., Godø O. R., Grimsbø E., Marini S., et al. (2020). A flexible autonomous robotic observatory infrastructure for bentho-pelagic monitoring. Sensors (Switzerland) 20 (6), 1614. doi: 10.3390/s20061614
Aguzzi J., Chatzievangelou D., Marini S., Fanelli E., Danovaro R., Flögel S., et al. (2019). “New high-tech flexible networks for the monitoring of deep-Sea ecosystems,” in Environ Sci Technol., 53 (12), 6616-6631. (American Chemical Society), 6616–6631. doi: 10.1021/acs.est.9b00409
Allison T. D., Diffendorfer J. E., Baerwald E. F., Beston J. A., Drake D., Hale A. M., et al. (2019). Impacts to wildlife of wind energy siting and operation in the united states. Issues Ecol. 21 (1), 2–18.
Amon D. J., Gollner S., Morato T., Smith C. R., Chen C., Christiansen C., et al(2022). Assessment of scientific gaps related to the effective environmental management of deep-seabed mining. Mar. Policy. doi: 10.1016/j.marpol.2022.105006
Ashford O., Guan S., Capone D., Rigney K., Rowley K., Cordes E., et al. (2021a). Relationships between biodiversity and ecosystem functioning proxies strengthen when approaching chemosynthetic deep-sea methane seeps. Proc. R. Soc 288, 20210950. doi: 10.1098/rspb.2021.0950
Ashford O., Guan S., Capone D., Rigney K., Rowley K., Orphan V., et al. (2021b). A chemosynthetic ecotone – ‘chemotone’ – in the sediments surrounding deep-sea methane seeps". Limnology Oceanogr. 66 (5), 1687–1702. doi: 10.1002/lno.11713
Bailey H., Brookes K. L., Thompson P. M. (2014). Assessing environmental impacts of offshore wind farms: lessons learned and recommendations for the future. Aquat. Biosyst. 10 (1), 1–13. doi: 10.1186/2046-9063-10-8
Bergström L., Sundqvist F., Bergström U. (2013). Effects of an offshore wind farm on temporal and spatial patterns in the demersal fish community. Mar. Ecol. Prog. Ser. 485, 199–210. doi: 10.3354/meps10344
Bonaglia S., Nascimento F. J. A., Bartoli M., Klawonn I., Brüchert V. (2014). Meiofauna increases bacterial denitrification in marine sediments. Nat. Commun. 5, 5133. doi: 10.1038/ncomms6133
Boyd P. W., Claustre H., Levy M., Siegel D. A., Weber T. (2019). Multi-faceted particle pumps drive carbon sequestration in the ocean. Nature 568 (7752), 327–335. doi: 10.1038/s41586-019-1098-2
Bravo M. E., Fiori S. M., Aliotta S., Amodeo M. R., Ginsberg S. (2020). Assessment of the impacts of shallow gas on taxonomic structure of benthic communities in bahía blanca estuary (Argentina). Estuarine Coast. Shelf Sci. 244, 106924. doi: 10.1016/j.ecss.2020.106924
Bravo M. E., Levin L. A., Fiori S. M., Aliotta S., Ginsberg S. (2021b). Can no-bubble methane seeps affect biological traits of benthic macroinvertebrates in coastal systems? Estuarine Coast. Shelf Sci. 261, 107525. doi: 10.1016/j.ecss.2021.107525
Brent Z. W., Barbesgaard M., Pedersen C. (2020). The blue fix: What's driving blue growth? Sustainability Sci. 15 (1), 31–43. doi: 10.1007/s11625-019-00777-7
Bremner J., Rogers S. I., Frid C. L. J. (2006). Methods for describing ecological functioning of marine benthic assemblages using biological traits analysis (BTA). Ecol. Indic. 6 (3), 609–622. doi: 10.1016/j.ecolind.2005.08.026
Bribiesca-Contreras G., Dahlgren T. G., Amon D. J., Cairns S., Drennan R., Durden J. M., et al. (2022). Benthic megafauna of the western clarion-clipperton zone, pacific ocean. ZooKeys 1113, 1–110. doi: 10.3897/zookeys.1113.82172. ZK.
Broström G. (2008). On the influence of large wind farms on the upper ocean circulation. J. Mar. Syst. 74, 585–591. doi: 10.1016/j.jmarsys.2008.05.001
Brown C. J., Smith S. J., Lawton P., Anderson J. T. (2011). Benthic habitat mapping: A review of progress towards improved understanding of the spatial ecology of the seafloor using acoustic techniques. Estuarine Coast. Shelf Sci. 92 (3), 502–520. doi: 10.1016/j.ecss.2011.02.007
Busecke J. J., Abernathey R. P. (2019). Ocean mesoscale mixing linked to climate variability. Sci. Adv. 5 (1), eaav5014. doi: 10.1126/sciadv.aav5014
Carley S., Konisky D. M. (2020). The justice and equity implications of the clean energy transition. Nat. Energy 5 (8), 569–577. doi: 10.1038/s41560-020-0641-6
Cavicchioli R., Ripple W. J., Timmis K. N., Azam F., Bakken L. R., Baylis M., et al. (2019). Scientists’ warning to humanity: microorganisms and climate change. Nat. Rev. Microbiol. 17 (9), 569–586. doi: 10.1038/s41579-019-0222-5
Chandler J., White D., Techera E. J., Gourvenec S., Draper S. (2017). Engineering and legal considerations for decommissioning of offshore oil and gas infrastructure in Australia. Ocean Eng. 131, 338–347. doi: 10.1016/j.oceaneng.2016.12.030
Clark M. R., Althaus F., Schlacher T. A., Williams A., Bowden D. A., Rowden A. A. (2016). The impacts of deep-sea fisheries on benthic communities: a review. ICES J. Mar. Sci. 73 (suppl_1), i51–i69. doi: 10.1093/icesjms/fsv123
Coley K. (2022). A global ocean map is not an ambition, but a necessity to support the ocean decade. Mar. Technol. Soc. J. 56 (3), 9–12. doi: 10.4031/MTSJ.56.3.3
Copping A. E., Freeman M. C., Gorton A. M., Hemery L. G. (2020). Risk retirement-decreasing uncertainty and informing consenting processes for marine renewable energy development. J. Mar. Sci. Eng. 8 (1), 1–22. doi: 10.3390/JMSE8030172
Cordes E. E., Jones D. O., Schlacher T. A., Amon D. J., Bernardino A. F., Brooke S., et al. (2016). Environmental impacts of the deep-water oil and gas industry: a review to guide management strategies. Front. Environ. Sci. 4, 58. doi: 10.3389/fenvs.2016.00058
Cordes E. E., Levin L. A. (2018). Exploration before exploitation. Science 359 (6377), 719–719. doi: 10.1126/science.aat2637
Cordier T., Forster D., Dufresne Y., Martins C. I. M., Stoeck T., Pawlowski J. W. (2018). Supervised machine learning outperforms taxonomy-based environmental DNA metabarcoding applied to biomonitoring. Mol. Ecol. Resour. 18 (6), 1381–1391. doi: 10.1111/1755-0998.12926
Corinaldesi C. (2015). New perspectives in benthic deep-sea microbial ecology. Front. Mar. Sci 2, 15. doi: 10.3389/fmars.2015.00017
Cornwall W. (2020). Renewable power surges as pandemic scrambles global energy outlook, new report finds. Sci. AAAS. doi: 10.1126/science.abc5463
Daly K. L., Remsen A., Outram D. M., Broadbent H., Kramer K., Dubickas K. (2021). Resilience of the zooplankton community in the northeast gulf of Mexico during and after the deepwater horizon oil spill. Mar. Poll. Bull. 163, 111882. doi: 10.1016/j.marpolbul.2020.111882
Danovaro R., Aguzzi J., Fanelli E., Billett D., Gjerde K., Jamieson A., et al. (2017). An ecosystem-based deep-ocean strategy. Science 355 (6324), 452–454. doi: 10.1126/science.aah7178
Danovaro R., Fanelli E., Aguzzi J., Billett D., Carugati L., Corinaldesi C., et al. (2020). Ecological variables for developing a global deep-ocean monitoring and conservation strategy. Nat. Ecol. Evol. 4 (2), 181–192. doi: 10.1038/s41559-019-1091-z
Davison P. C., Checkley D. M. Jr., Koslow J. A., Barlow J. (2013). Carbon export mediated by mesopelagic fishes in the northeast pacific ocean. Prog. Oceanogr. 116, 14–30. doi: 10.1016/j.pocean.2013.05.013
Del Río J., Nogueras M., Aguzzi J., Toma D., Masmitja I., Carandell M., et al. (2020). A decadal balance for a cabled observatory deployment. IEEE Access 8, 33163–33177. doi: 10.1109/ACCESS.2020.2973771
Demopoulos A. W., Bourque J. R., Durkin A., Cordes E. E. (2018). The influence of seep habitats on sediment macrofaunal biodiversity and functional traits. Deep Sea Res. Part I: Oceanogr. Res. Papers 142, 77–93. doi: 10.1016/j.dsr.2018.10.004
Díaz S., Pascual U., Stenseke M., Martín-López B., Watson R., Molnár Z., et al. (2018). Assessing nature’s contributions to people. Science 359, 270–272. doi: 10.1126/science.aap8826
DOSI (2021a). “The necessity of traditional knowledge for management of deep-seabed mining,” in Deep ocean stewardship initiative report. Available at: https://www.dosi-project.org/wp-content/uploads/072-DOSI-Policy-brief-Traditional-Knowledge-for-Management-of-DSM-long-V11.pdf.
DOSI (2021b). “Redefining the influence of chemosynthetic ecosystems for effective management,” in Deep ocean stewardship initiative report. Available at: https://www.dosi-project.org/wp-content/uploads/Chemosynthetic-Ecosystems-Policy-Brief.pdf.
DOSI (2022). “Review of impact assessments for deep-Sea fisheries on the high seas,” in Deep ocean stewardship initiative report. Available at: https://www.dosi-project.org/wpcontent/uploads/fisheries-impact-assessment-report.pdf.
Douvere F., Ehler C. N. (2009). New perspectives on sea use management: initial findings from European experience with marine spatial planning. J. Environ. Manage. 90 (1), 77–88. doi: 10.1016/j.jenvman.2008.07.004
Drazen J. C., Smith C. R., Gjerde K. M., Haddock S. H. D., Carter G. S., Choy C. A., et al. (2020). Midwater ecosystems must be considered when evaluating environmental risks of deep-sea mining. Proceedings of the National Academy of Sciences 30, 17455–17460. 117. doi: 10.1073/pnas.2011914117
Drazen J. C., Sutton T. T. (2017). Dining in the deep: the feeding ecology of deep-sea fishes. Annu. Rev. Mar. Sci. 9 (1), 337–366. doi: 10.1146/annurev-marine-010816-060543
Durden J. M., Lallier L. E., Murphy K., Jaeckel A., Gjerde K., Jones D. O. B. (2018). Environmental impact assessment process for deep-sea mining in ‘the area’. Mar. Policy 87, 194–202. doi: 10.1016/j.marpol.2017.10.013
Easson C. G., Boswell K. M., Tucker N., Warren J. D., Lopez J. V. (2020). Combined eDNA and acoustic analysis reflects diel vertical migration of mixed consortia in the gulf of Mexico. Front. Mar. Sci. 7, 552. doi: 10.3389/fmars.2020.00552
Eikeset A. M., Mazzarella A. B., Davíðsdóttir B., Klinger D. H., Levin S. A., Rovenskaya E., et al. (2018). What is blue growth? the semantics of “Sustainable development” of marine environments. Mar. Policy 87, 177–179. doi: 10.1016/j.marpol.2017.10.019
Equinor (2022) Energy perspectives report. Available at: https://www.equinor.com/sustainability/energy-perspectives?3563f6a20ed7=0.
Fernand F., Israel A., Skjermo J., Wichard T., Timmermans K. R., Golberg A. (2017). Offshore macroalgae biomass for bioenergy production: Environmental aspects, technological achievements and challenges. Renewable Sustain. Energy Rev. 75, 35–45. doi: 10.1016/j.rser.2016.10.046
Fisher C. R., Hsing P. Y., Kaiser C. L., Yoerger D. R., Roberts H. H., Shedd W. W., et al. (2014). Footprint of deepwater horizon blowout impact to deep-water coral communities. Proc. Natl. Acad. Sci. 111 (32), 11744–11749. doi: 10.1073/pnas.1403492111
Floeter J., Pohlmann T., Harmer A., Möllmann C. (2022). Chasing the offshore wind farm wind-wake-induced upwelling/downwelling dipole. Front. Mar. Sci. 9. doi: 10.3389/fmars.2022.884943
Food and Agriculture Organization (2009). Management of deep-sea fisheries in the high seas (Rome, Italy: FAO).
Food and Agriculture Organization (2017). “Management of deep-sea fisheries in the high seas, FAO, blue growth initiative,” in Partnering with countries to achieve the sustainable development goals (Rome, Italy: Food and Agriculture Organization).
Fowler A. M., Jørgensen A. M., Coolen J. W. P., Jones D. O. B., Svendsen J. C., Brabant R., et al. (2019). The ecology of infrastructure decommissioning in the north Sea: what we need to know and how to achieve it. ICES J. Mar. Sci. 77, 3. doi: 10.1093/icesjms/fsz143
Frazão Santos C., Agardy T., Andrade F., Calado H., Crowder L. B., Ehler C. N., et al. (2020). Integrating climate change in ocean planning. Nat. Sustainability 3 (7), 505–516. doi: 10.1038/s41893-020-0513-x
Freiwald A., Fossâ J. H., Grehan A., Koslow T., Roberts J. M. (2004). Cold-water coral reefs: out of sight-no longer out of mind (United Kingdom: UNEP-WCMC).
Gasbarro R., Sowers D., Margolin A., Cordes E. E. (2022). Distribution and predicted climatic refugia for a reef-building cold-water coral on the southeast US margin. Global Change Biol 28, 7108–7125. doi: 10.1111/gcb.16415
Georgian S. E., Kramer K., Saunders M., Shedd W., Roberts H., Lewis C., et al. (2020). Habitat suitability modelling to predict the spatial distribution of cold-water coral communities affected by the deepwater horizon oil spill. J. Biogeogr. 47 (7), 1455–1466. doi: 10.1111/jbi.13844
Girard F., Fisher C. R. (2018). Long-term impact of the deepwater horizon oil spill on deep-sea corals detected after seven years of monitoring. Biol. Conserv. 225, 117–127. doi: 10.1016/j.biocon.2018.06.028
Glasson J., Therivel R. (2019). Introduction to environmental impact assessment. Fifth edition (London: Routledge), 394. doi: 10.4324/9780429470738
Global Wind Energy Council (2021) Global wind report. Available at: https://gwec.net/global-wind-report-2021/.
Glover A., Dahlgren T., Wiklund H., Mohrbeck I., Smith C. R. (2015). An end-to-End DNA taxonomy methodology for benthic biodiversity survey in the clarion-clipperton zone, central pacific abyss. J. Mar. Sci. Eng. 4 (1), 2. doi: 10.3390/jmse4010002
Glover A. G., Sundberg P., Dahlgren T. G. (2009). In linnaeus’ wake: 300 years of marine discovery. Zoologica Scripta 38, 1–6. doi: 10.1111/j.1463-6409.2009.00375.x
Glover A. G., Wiklund H., Chen C., Dahlgren T. G. (2018). Managing a sustainable deep-sea ‘blue economy’ requires knowledge of what actually lives there. eLife 7, e41319. doi: 10.7554/eLife.41319
Glud R. N. (2008). Oxygen dynamics of marine sediments. Mar. Biol. Res. 4, 243–289. doi: 10.1080/17451000801888726
Gollner S., Colaço A., Gebruk A., Halpin P. N., Higgs N., Menini E., et al. (2021). Application of scientific criteria for identifying hydrothermal ecosystems in need of protection. Mar. Policy 132, 104641. doi: 10.1016/j.marpol.2021.104641
Gooday A. J. (1993). Deep-sea benthic foraminiferal species which exploit phytodetritus: characteristic features and controls on distribution. Mar. Micropaleontol. 22 (3), 187–205. doi: 10.1016/0377-8398(93)90043-W
Gooday A. J., Levin L. A., Linke P., Heeger T. (1992). “The role of benthic foraminifera in deep-sea food webs and carbon cycling,” in Deep-Sea food chains and the global carbon cycle. Eds. Rowe G. T., Pariente V. (the Netherlands: Kluwer Academic Publishers), 63–91.
Halanych K. M., Ainsworth C. H., Cordes E. E., Dodge R. E., Huettel M., Mendelssohn I. A., et al. (2021). Effects of petroleum by-products and dispersants on ecosystems. Oceanography 34 (1), 152–163. doi: 10.5670/oceanog.2021.123
Hammar L., Gullström M., Dahlgren T. G., Asplund M. E., Goncalves I. B., Molander S. (2017). Introducing ocean energy industries to a busy marine environment. Renewable Sustain. Energy Rev. 74, 178–185. doi: 10.1016/j.rser.2017.01.092
Haugan P. M., Levin L. A., Amon D., Hemer M., Lily H., Nielsen F. G. (2019). What role for ocean-based renewable energy and deep seabed minerals in a sustainable future? (Washington, DC: World Resources Institute). Available at: www.oceanpanel.org/blue-papers/ocean-energy-and-mineral-sources.
Hestetun J. T., Bye-Ingebrigtsen E., Nilsson R. H., Glover A. G., Johansen P. O., Dahlgren T. G. (2020). Significant taxon sampling gaps in DNA databases limit the operational use of marine macrofauna metabarcoding. Mar. Biodivers. 50 (5), 1–9. doi: 10.1007/s12526-020-01093-5
Hilmi N., Chami R., Sutherland M. D., Hall-Spencer J. M., Lebleu L., Benitez M. B., et al. (2021). The role of blue carbon in climate change mitigation and carbon stock conservation. Front. Clim 3. doi: 10.3389/fclim.2021.710546
Hoegh-Guldberg O., Jacob D., Taylor M., Guillén Bolaños T., Bindi M., Brown S., et al. (2019). The human imperative of stabilizing global climate change at 1.5 c. Science 365 (6459), eaaw6974. doi: 10.1126/science.aaw6974
Howe B. M., Arbic B. K., Aucan J., Barnes C., Bayliff N., Becker N., et al. (2019). Smart cables for observing the global ocean: Science and implementation. Front. Mar. Sci. 6. doi: 10.3389/fmars.2019.00424. Issue.
Howell K. L., Hilário A., Allcock A. L., Bailey D. M., Baker M., Clark M. R., et al. (2020). A blueprint for an inclusive, global deep-sea ocean decade field program. Front. Mar. Sci., 999. doi: 10.3389/fmars.2020.584861
Howell K. L., Hilário A., Allcock A. L., Bailey D., Baker M., Clark M. R., et al. (2021). A decade to study deep-sea life. Nat. Ecol. Evol. 5 (3), 265–267. doi: 10.1038/s41559-020-01352-5
Iacono C. L., Robert K., Gonzalez-Villanueva R., Gori A., Gili J. M., Orejas C. (2018). Predicting cold-water coral distribution in the cap de creus canyon (NW mediterranean): Implications for marine conservation planning. Prog. Oceanogr. 169, 169–180. doi: 10.1016/j.pocean.2018.02.012
Ibrahim O. S., Singlitico A., Proskovics R., McDonagh S., Desmond C., Murphy J. D. (2022). Dedicated large-scale floating offshore wind to hydrogen: Assessing design variables in proposed typologies. Renewable Sustain. Energy Rev. 160, 112310. doi: 10.1016/j.rser.2022.112310
IEA (2019a). “Offshore wind outlook 2019,” in World energy outlook special report. Available at: https://www.iea.org/reports/offshore-wind-outlook-2019.
IEA (2019b)World energy outlook 2019. In: . Available at: www.iea.org/reports/world-energy-outlook-2019.
Ingels J., Vanreusel A., Pape E., Pasotti F., Macheriotou L., Martínez Arbizu P., et al. (2021). Ecological variables for deep-ocean monitoring must include microbiota and meiofauna for effective conservation. Nat. Ecol. Evol. 5, 27–29. doi: 10.1038/s41559-020-01335-6
Jager H. I., Efroymson R. A., McManamay R. A. (2021). Renewable energy and biological conservation in a changing world. Biol. Conserv. 263, 109354. doi: 10.1016/j.biocon.2021.109354
Jobstvogt N., Watson V., Kenter J. O. (2014). Looking below the surface: The cultural ecosystem service values of UK marine protected areas (MPAs). Ecosystem Serv. 10, 97–110. doi: 10.1016/j.ecoser.2014.09.006
Jones D. O., Durden J. M., Murphy K., Gjerde K. M., Gebicka A., Colaço A., et al. (2019a). Existing environmental management approaches relevant to deep-sea mining. Mar. Policy 103, 172–181. doi: 10.1016/j.marpol.2019.01.006
Jones D. O., Gates A. R., Huvenne V. A., Phillips A. B., Bett B. J. (2019b). Autonomous marine environmental monitoring: Application in decommissioned oil fields. Sci. total Environ. 668, 835–853. doi: 10.1016/j.scitotenv.2019.02.310
Junqueira H., Robaina M., Garrido S., Godina R., Matias J. C. (2020). Viability of creating an offshore wind energy cluster: A case study. Appl. Sci. 11 (1), 308. doi: 10.3390/app11010308
Keeley N., Laroche O., Birch M., Pochon X. (2021). A substrate-independent benthic sampler (SIBS) for hard and mixed-bottom marine habitats: A proof-of-Concept study. Front. Mar. Sci. 8. doi: 10.3389/fmars.2021.627687
Kujawinski E. B., Reddy C. M., Rodgers R. P., Thrash J. C., Valentine D. L., White H. K. (2020). The first decade of scientific insights from the deepwater horizon oil release. Nat. Rev. Earth Environ. 1 (5), 237–250. doi: 10.1038/s43017-020-0046-x
Laffoley D., Baxter J. M., Amon D. J., Claudet J., Hall-Spencer J. M., Grorud-Colvert K., et al. (2020). “Evolving the narrative for protecting a rapidly changing ocean post COVID-19. Marine and Freshwater Ecosystems Aquatic conservation 31 (6), 1512-1534. doi: 10.1002/aqc.3512
Lanzén A., Dahlgren T. G., Bagi A., Hestetun J. T. (2021a). Benthic eDNA metabarcoding provides accurate assessments of impact from oil extraction, and ecological insights. Ecol. Indic. 130, 108064. doi: 10.1016/j.ecolind.2021.108064
Lanzén A., Mendibil I., Borja A., Alonso-Sáez L. (2021b). A microbial mandala for environmental monitoring: Predicting multiple impacts on estuarine prokaryote communities of the bay of Biscay. Mol. Ecol. 30 (13), 2969–2987. doi: 10.1111/mec.15489
Le J. T., Girguis P., Levin L. A. (2022). Using deep-sea images to examine ecosystem services associated with methane seeps. Mar. Environ. Res 181 (2022), 105740. doi: 10.1016/j.marenvres.2022.105740
Le J. T., Levin L. A., Carson R. T. (2017). Incorporating ecosystem services into environmental management of deep-seabed mining. Deep Sea Res. Part II: Topical Stud. Oceanogr. 137, 486–503. doi: 10.1016/j.dsr2.2016.08.007
Levin L. A., Baco A. R., Bowden D., Colaco A., Cordes E., Cunha M. R., et al. (2016). Hydrothermal vents and methane seeps: rethinking the sphere of influence. Front. Mar. Sci. 3. doi: 10.3389/fmars.2016.00072
Levin L. A., Cimoli L., Gjerde K., Harden-Davies H., Heimbach P., LaScala-Gruenewald D., et al. (2022). Designing, generating, and translating deep-ocean observations for and with international policy makers. ICES J. Mar. Sci. 79 (7), 1992–1995. doi: 10.1093/icesjms/fsac143
Levin L. A., Mendoza G. F., Grupe B. M. (2017). Methane seepage effects on biodiversity and biological traits of macrofauna inhabiting authigenic carbonates. Deep Sea Res. Part II: Topical Stud. Oceanogr. 137, 26–41. doi: 10.1016/j.dsr2.2016.05.021
Levin L. A., Wei C. L., Dunn D. C., Amon D. J., Ashford O. S., Cheung W. W., et al. (2020). Climate change considerations are fundamental to management of deep-sea resource extraction. Global Change Biol. 26 (9), 4664–4678. doi: 10.1111/gcb.15223
Lim S. C., Wiklund H., Glover A. G., Dahlgren T. G., Tan K. S. (2017). A new genus and species of abyssal sponge commonly encrusting polymetallic nodules in the clarion-clipperton zone, East pacific ocean. Systematics biodiversity 15 (6), 507–519. doi: 10.1080/14772000.2017.1358218
Lins L., Zeppilli D., Menot L., Michel L. N., Bonifácio P., Brandt M., et al. (2021). Toward a reliable assessment of potential ecological impacts of deep-sea polymetallic nodule mining on abyssal infauna. Limnology Oceanogr.: Methods 19 (9), 626–650. doi: 10.1002/lom3.10448
Longmore C., Trueman C. N., Neat F., Jorde P. E., Knutsen H., Stefanni S., et al. (2014). Ocean-scale connectivity and life cycle reconstruction in a deep-sea fish. Can. J. Fisheries Aquat. Sci. 71 (9), 1312–1323. doi: 10.1139/cjfas-2013-0343
Luderer G., Pehl M., Arvesen A., Gibon T., Bodirsky B. L., de Boer H. S., et al. (2019). Environmental co-benefits and adverse side-effects of alternative power sector decarbonization strategies. Nat. Commun. 10 (1), 1–13. doi: 10.1038/s41467-019-13067-8
MA (2005). Millennium ecosystem assessment, ecosystems and human well-being: Synthesis (Washington, DC: Island Press). Available at: https://www.millenniumassessment.org/documents/document.356.aspx.pdf.
Macreadie P. I., Fowler A. M., Booth D. J. (2011). Rigs-to-reefs: will the deep sea benefit from artificial habitat? Front. Ecol. Environ. 9 (8), 455–461. doi: 10.1890/100112
Maier S. R., Mienis F., Froe E., Soetaert K., Lavaleye M., Duineveld G., et al. (2021). Reef communities associated with ‘dead’ cold-water coral framework drive resource retention and recycling in the deep sea. Deep–Sea Res. I 175, 103574. doi: 10.1016/j.dsr.2021.103574
McCartin L. J., Vohsen S. A., Ambrose S. W., Layden M., McFadden C. S., Cordes E. E., et al. (2022). Temperature controls eDNA persistence across physicochemical conditions in seawater. Environ. Sci. Technol. 56, 12, 8629–8639. doi: 10.1021/acs.est.2c01672
Marlow J. J., Steele J. A., Ziebis W., Thurber A. R., Levin L. A., Orphan V. J. (2014). Carbonate hosted methanotrophy: an unrecognized methane sink in the deep sea. Nat. Commun. 5, 5094. doi: 10.1038/ncomms6094
Mata D., Muñoz A., Viscasillas L., Varas D. (2020). Geomorphic features and associated habitats of the Patagonian continental margin, southwestern Atlantic. In Seafloor Geomorphol. as Benthic Habitat, 721–734. Elsevier. doi: 10.1016/B978-0-12-814960-7.00043-9
Mauffrey F., Cordier T., Apothéloz-Perret-Gentil L., Cermakova K., Merzi T., Delefosse M., et al. (2021). Benthic monitoring of oil and gas offshore platforms in the north Sea using environmental DNA metabarcoding. Mol. Ecol. 30 (13), 3007–3022. doi: 10.1111/mec.15698
Mavraki N., Degraer S., Vanaverbeke J., Braeckman U. (2020). Organic matter assimilation by hard substrate fauna in an offshore wind farm area: a pulse-chase study. ICES J. Mar. Sci. 77, 2681–2693. doi: 10.1093/icesjms/fsaa133
Mejjad N., Rovere M. (2021). Understanding the impacts of blue economy growth on deep-Sea ecosystem services. Sustainability 13 (22), 12478. doi: 10.3390/su132212478
Melo-Merino S. M., Reyes-Bonilla H., Lira-Noriega A. (2020). Ecological niche models and species distribution models in marine environments: A literature review and spatial analysis of evidence. Ecol. Model. 415, 108837. doi: 10.1016/j.ecolmodel.2019.108837
Methratta E. T., Dardick W. R. (2019). Meta-analysis of finfish abundance at offshore wind farms. Rev. Fisheries Sci. Aquac. 27 (2), 242–260. doi: 10.1080/23308249.2019.1584601
Mirimin L., Desmet S., López Romero D., Fernandez Fernandez S., Miller D., Mynott S., et al. (2021). Don’t catch me if you can – using cabled observatories as multidisciplinary platforms for marine fish community monitoring: a case study combining underwater video and environmental DNA (eDNA) data. Sci. Total 773(15) 145351. doi: 10.1016/j.scitotenv.2021.145351
Montserrat F., Guilhon M., Corrêa P. V. F., Bergo N. M., Signori C. N., Tura P. M., et al. (2019). Deep-sea mining on the Rio grande rise (Southwestern atlantic): A review on environmental baseline, ecosystem services and potential impacts. Deep Sea Res. Part I: Oceanogr. Res. Papers 145, 31–58. doi: 10.1016/j.dsr.2018.12.007
Moore C., Drazen J. C., Radford B. T., Kelley C., Newman S. J. (2016). Improving essential fish habitat designation to support sustainable ecosystem-based fisheries management. Mar. Policy 69, 32–41. doi: 10.1016/j.marpol.2016.03.021
Morato T., González-Irusta J. M., Dominguez-Carrió C., Wei C. L., Davies A., Sweetman A. K., et al. (2020). Climate-induced changes in the suitable habitat of cold-water corals and commercially important deep-sea fishes in the north Atlantic. Global Change Biol. 26 (4), 2181–2202. doi: 10.1111/gcb.14996
Murawski S. A., Hollander D. J., Gilbert S., Gracia A. (2020). “Deepwater oil and gas production in the gulf of Mexico and related global trends. chapter 2,” in Scenarios and responses to future deep oil spills - fighting the next war. Eds. Murawski S. A., Hollander D., Ainsworth C., Gilbert S., Paris C. B., Schlüter M., Wetzel D. (Cham: Springer).
Murray F., Needham K., Gormley K., Rouse S., Coolen J. W., Billett D., et al. (2018). Data challenges and opportunities for environmental management of north Sea oil and gas decommissioning in an era of blue growth. Mar. Policy 97, 130–138. doi: 10.1016/j.marpol.2018.05.021
Nascimento F. J. A., Naslund J., Elmgren R. (2012). Meiofauna enhances organic matter mineralization in soft sediment ecosystems. Limnology Oceanogr. 57, 338–346. doi: 10.4319/lo.2012.57.1.0338
Neele F., Wu H., Hendriks C., Brandsma R. (2011). Planning of CCS development in the Netherlands offshore. Energy Proc. 4, 2756–2763. doi: 10.1016/j.egypro.2011.02.178
Novaglio C., Bax N., Boschetti F., Emad G. R., Frusher S., Fullbrook L., et al. (2022). Deep aspirations: towards a sustainable offshore blue economy. Rev. fish Biol. fisheries 32 (1), 209–230. doi: 10.1007/s11160-020-09628-6
Nowacek D. P., Bröker K., Donovan G., Gailey G., Racca R., Reeves R. R., et al. (2013). Responsible practices for minimizing and monitoring environmental impacts of marine seismic surveys with an emphasis on marine mammals. Aquat. Mammals 39 (4), 356. doi: 10.1578/AM.39.4.2013.356
Orcutt B. N., Bradley J. A., Brazelton W. J., Estes E. R., Goordial J. M., Huber J. A., et al. (2020). Impacts of deep-sea mining on microbial ecosystem services. Limnology oceanogr. 65 (7), 1489–1510. doi: 10.1002/lno.11403
Ottaviani (2020). Economic value of ecosystem services from the deep seas and the areas beyond national jurisdiction (Rome: FAO). FAO Fisheries and Aquaculture Circular No. 1210. doi: 10.4060/ca8340en
Panagopoulos A., Haralambous K. J. (2020). Environmental impacts of desalination and brine treatment-challenges and mitigation measures. Mar. pollut. Bull. 161, 111773. doi: 10.1016/j.marpolbul.2020.111773
Peterson C. H., Franklin K. P., Cordes E. E. (2020). Habitat connectivity enhances restoration success in a changing gulf of Mexico ecosystem. Elementa 8, 16. doi: 10.1525/elementa.016
Piepenburg D., Buschmann A., Driemel A., Grobe H., Gutt J., Schumacher S., et al. (2017). Seabed images from southern ocean shelf regions off the northern Antarctic peninsula and in the southeastern weddell Sea. Earth System Sci. Data 9 (2), 461–469. doi: 10.5194/essd-9-461-2017
Poulsen T., Lema R. (2017). Is the supply chain ready for the green transformation? the case of offshore wind logistics. Renew sust energ Rev. 73, 758–771. doi: 10.1016/j.rser.2017.01.181
Prouty N. G., Fisher C. R., Demopoulos A. W., Druffel E. R. (2016). Growth rates and ages of deep-sea corals impacted by the deepwater horizon oil spill. Deep Sea Res. Part II: Topical Stud. Oceanogr. 129, 196–212. doi: 10.1016/j.dsr2.2014.10.021
Purser A., Hehemann L., Boehringer L., Tippenhauer S., Wege M., Bornemann H., et al. (2022). Hot off the press: A vast icefish breeding colony discovered in the Antarctic. Deep Sea Life 18), 51–52. doi: 10013/epic.04e2106f-3422-4e6b-aacc-958a0f85cd31
Queirós A. M., Huebert K. B., Keyl F., Fernandes J. A., Stolte W., Maar M., et al. (2016). Solutions for ecosystem-level protection of ocean systems under climate change. Global Change Biol. 22 (12), 3927–3936. doi: 10.1111/gcb.13423
Rex M. A., Etter R. J., Morris J. S., Crouse J., McClain C. R., Johnson N. A., et al. (2006). Global bathymetric patterns of standing stock and body size in the deep-sea benthos. Mar. Ecol. Prog. Ser. 317, 1–8. doi: 10.3354/meps317001
Riehl T., Wölfl A. C., Augustin N., Devey C. W., Brandt A. (2020). Discovery of widely available abyssal rock patches reveals overlooked habitat type and prompts rethinking deep-sea biodiversity. Proc. Natl. Acad. Sci. United States America 117 (27), 15450-15459. doi: 10.1073/pnas.1920706117
Roberts C. M., Hawkins J. P., Gell F. R. (2005). The role of marine reserves in achieving sustainable fisheries. Philos. Trans. R. Soc. B: Biol. Sci. 360 (1453), 123–132. doi: 10.1098/rstb.2004.1578
Robison B. H. (2009). Conservation of deep pelagic biodiversity. Conserv. Biol. 23 (4), 847–858. doi: 10.1111/j.1523-1739.2009.01219.x
Rogers A. D., Gianni M. (2010)The implementation of UNGA resolutions 61/105 and 64/72 in the management of deep-Sea fisheries on the high seas. Available at: http://www.savethehighseas.org/publicdocs/61105-Implemention-ExecSummary.pdf.
Sala E., Mayorga J., Bradley D., Cabral R. B., Atwood T. B., Auber A., et al. (2021). Protecting the global ocean for biodiversity, food and climate. Nature 38 (4), 1–6. doi: 10.1038/s41586-021-03371-z
Schratzberger M., Ingels J. (2018). Meiofauna matters: The roles of meiofauna in benthic ecosystems. J. Exp. Mar. Biol. Ecol. 502, 12–25. doi: 10.1016/j.jembe.2017.01.007
Schwing P. T., O’Malley B. J., Romero I. C., Martínez-Colón M., Hastings D. W., Glabach M. A., et al. (2017). Characterizing the variability of benthic foraminifera in the northeastern gulf of Mexico following the deepwater horizon event, (2010–2012). Environ. Sci. pollut. Res. 24 (3), 2754–2769. doi: 10.1007/s11356-016-7996-z
Seascape Consultants LTD (2014). Review of the implementation of the environmental management plan for the clarion-clipperton zone. Rep. Int. Seabed Authority. https://isa.org.jm/files/documents/EN/20Sess/LTC/CCZ-EMPRev.pdf
Sigwart J. D., Chen C., Thomas E. A., Allcock A. L., Böhm M., Seddon M. (2019). Red listing can protect deep-sea biodiversity. Nat. Ecol. Evol. 3 (8), 1134–1134. doi: 10.1038/s41559-019-0930-2
Slavik K., Lemmen C., Zhang W., Kerimoglu O., Klingbeil K., Wirtz K. W. (2019). The large-scale impact of offshore wind farm structures on pelagic primary productivity in the southern north Sea. Hydrobiologia 845, 35–53. doi: 10.1007/s10750-018-3653-5
Sommer B., Fowler A. M., Macreadie P. I., Palandro D. A., Aziz A. C., Booth D. J. (2019). Decommissioning of offshore oil and gas structures–environmental opportunities and challenges. Sci. total Environ. 658, 973–981. doi: 10.1016/j.scitotenv.2018.12.193
Stat M., John J., DiBattista J. D., Newman S. J., Bunce M., Harvey E. S. (2019). Combined use of eDNA metabarcoding and video surveillance for the assessment of fish biodiversity. Conserv. Biol. 33 (1), 196–205. doi: 10.1111/cobi.13183
Stefanni S., Mirimin L., Stanković D., Chatzievangelou D., Bongiorni L., Marini S., et al. (2022). Framing cutting-edge integrative deep-Sea biodiversity monitoring via environmental DNA and optoacoustic augmented infrastructures. Front. Mar. Sci. 8. doi: 10.3389/fmars.2021.797140
Steinberg D. K., Landry M. R. (2017). Zooplankton and the ocean carbon cycle. Annu. Rev. Mar. Sci. 9 (1), 413–444. doi: 10.1146/annurev-marine-010814-015924
Sutherland J., Peet A. H., Soulsby R. (2004). Evaluating the performance of morphological models. Coast. Eng. 51 (8-9), 917–939. doi: 10.1016/j.coastaleng.2004.07.015
Sutton T. T. (2013). Vertical ecology of the pelagic ocean: classical patterns and new perspectives. J. fish Biol. 83 (6), 1508–1527. doi: 10.1111/jfb.12263
Sutton T. T., Clark M. R., Dunn D. C., Halpin P. N., Rogers A. D., Guinotte J., et al. (2017). A global biogeographic classification of the mesopelagic zone. Deep Sea Res. Part I: Oceanogr. Res. Papers 126, 85–102. doi: 10.1016/j.dsr.2017.05.006
Sutton T. T., Milligan R., Boswell K., Cook A., Cornic M., Daly K., et al. (2022). The open-ocean gulf of Mexico and Deepwater horizon: A decadal synthesis of research. Front. Mar. Res. 9. doi: 10.3389/fmars.2022.753391
Swanborn D. J., Huvenne V. A., Pittman S. J., Woodall L. C. (2022). Bringing seascape ecology to the deep seabed: A review and framework for its application. Limnology Oceanogr. 67 (1), 66–88. doi: 10.1002/lno.11976
Taberlet P., Coissac E., Hajibabaei M., Rieseberg L. H. (2012). Environmental DNA. Mol. Ecol. 21 (8), 1789–1793. doi: 10.1111/j.1365-294X.2012.05542.x
Teneva L., Strong A. L., Agostini V., Bagstad K. J., Drakou E. G., Ancona Z., et al. (2022). Estimating the pelagic ocean’s benefits to humanity can enhance ocean governance. Mar. Policy 136, 104906.
Thaler A. D., Amon D. (2019). 262 voyages beneath the Sea: a global assessment of macro- and megafaunal biodiversity and research effort at deep-sea hydrothermal vents. PeerJ 7, e7397. doi: 10.7717/peerj.7397
Thorsnes T., Chand S. (2022). Seabed mapping using synthetic aperture sonar and AUV-important tools for studies of cold seep habitats (No. EGU22-13440). Copernicus Meetings. (2022) doi: 10.5194/egusphere-egu22-13440
Thurber A. R., Sweetman A. K., Narayanaswamy B. E., Jones D. O., Ingels J., Hansman R. L. (2014). Ecosystem function and services provided by the deep sea. Biogeosciences 11 (14), 3941–3963. doi: 10.5194/bg-11-3941-2014
Tilot V., Willaert K., Guilloux B., Chen W., Mulalap C. Y., Gaulme F., et al. (2021). Traditional dimensions of seabed resource management in the context of deep Sea mining in the pacific: Learning from the socio-ecological interconnectivity between island communities and the ocean realm. Front. Mar. Sci. 8, 637938. doi: 10.3389/fmars.2021.637938
Torres J. J., Bailey T. (2022). Life in the open ocean: the biology of pelagic species (Hoboken: Wiley Blackwell).
Trueman C. N., Johnston G., O'hea B., MacKenzie K. M. (2014). Trophic interactions of fish communities at midwater depths enhance long-term carbon storage and benthic production on continental slopes. Proc. R. Soc. B: Biol. Sci. 281 (1787), 20140669. doi: 10.1098/rspb.2014.0669
Tunnicliffe V., Metaxas A., Le J., Ramirez-Llodra E., Levin L. A. (2020). Strategic environmental goals and objectives: setting the basis for environmental regulation of deep seabed mining. Mar. Policy 114, 1-10. doi: 10.1016/j.marpol.2018.11.010
Turley C. (2000). Bacteria in the cold deep-sea benthic boundary layer and sediment–water interface of the NE Atlantic. FEMS Microbiol. Ecol. 33, 89–99. doi: 10.1111/j.1574-6941.2000.tb00731.x
Uhlenkott K., Vink A., Kuhn T., Gillard B., Martínez Arbizu P. (2021). Meiofauna in a potential deep-sea mining area—influence of temporal and spatial variability on small-scale abundance models. Diversity (Switzerland: PANGAEA) 13, 3. doi: 10.3390/d13010003
Van Dover C. L., Arnaud-Haond S., Gianni M., Helmreich S., Huber J. A., Jaeckel A. L., et al. (2018). Scientific rationale and international obligations for protection of active hydrothermal vent ecosystems from deep-sea mining. Mar. Policy 90, 20–28. doi: 10.1016/j.marpol.2018.01.020
Wahlberg M., Westerberg H. (2005). Hearing in fish and their reactions to sounds from offshore wind farms. Mar. Ecol. Prog. Ser. 288, 295–309. doi: 10.3354/meps288295
Wang C., Prinn R. G. (2011). Potential climatic impacts and reliability of large-scale offshore wind farms. Environ. Res. Lett. 6 (2), 025101. doi: 10.1088/1748-9326/6/2/025101
Watling L., Norse E. A. (1998). Disturbance of the seabed by mobile fishing gear: a comparison to forest clearcutting. Conserv. Biol. 12 (6), 1180–1197. doi: 10.1046/j.1523-1739.1998.0120061180.x
Watts M. (2012). A tale of two gulfs: life, death, and dispossession along two oil frontiers. Am. Q 64 (3), 437–467. doi: 10.1353/aq.2012.0039
Weaver P. P. E., Benn A., Arana P. M., Ardron J. A., Bailey D. M., Baker K., et al. (2011). Report of an international scientific workshop National Oceanography Centre, Southampton. Available at: https://epic.awi.de/id/eprint/24870/.
Webb T. J., Vanden Berghe E., O'Dor R. (2010). Biodiversity's big wet secret: the global distribution of marine biological records reveals chronic under-exploration of the deep pelagic ocean. PloS One 5 (8), e10223. doi: 10.1371/journal.pone.0010223
Wei C. L., Rowe G. T., Escobar-Briones E., Boetius A., Soltwedel T., Caley M. J., et al. (2010). Global patterns and predictions of seafloor biomass using random forests. PloS One 5 (12), e15323. doi: 10.1371/journal.pone.0015323
WWF (2014). Environmental impacts of offshore wind in the north Sea– a literature review (Oslo: WWF). Available at: https://www.wwf.no/assets/attachments/84-wwf_a4_report:_havvindrapport.pdf.
Yamahara K. M., Preston C. M., Birch J., Walz K., Marin R. III, Jensen S., et al. (2019). In situ autonomous acquisition and preservation of marine environmental DNA using an autonomous underwater vehicle. Front. Mar. Sci. 6, 373. doi: 10.3389/fmars.2019.00373
Yow Mulalap C., Frere T., Huffer E., Hviding E., Paul K., Smith A., et al. (2020). Traditional knowledge and the BBNJ instrument. Mar. Policy 122, 104103. doi: 10.1016/j.marpol.2020.104103
Keywords: offshore renewable energies, offshore oil and gas industry, deep ocean capacity building, capacity building, observing technologies, ecosystem-based management, environmental impact assessment, deep sea industries
Citation: Bravo ME, Brandt MI, van der Grient JMA, Dahlgren TG, Esquete P, Gollner S, Jones DOB, Levin LA, McClain CR, Narayanaswamy BE, Sutton T, Victorero L and Cordes EE (2023) Insights from the management of offshore energy resources: Toward an ecosystem-services based management approach for deep-ocean industries. Front. Mar. Sci. 9:994632. doi: 10.3389/fmars.2022.994632
Received: 15 July 2022; Accepted: 28 November 2022;
Published: 12 January 2023.
Edited by:
Jacopo Aguzzi, Institute of Marine Sciences (CSIC), SpainReviewed by:
Philip Weaver, Seascape Consultants Ltd, United KingdomMarzia Rovere, Institute of Marine Sciences, Italy
Copyright © 2023 Bravo, Brandt, van der Grient, Dahlgren, Esquete, Gollner, Jones, Levin, McClain, Narayanaswamy, Sutton, Victorero and Cordes. This is an open-access article distributed under the terms of the Creative Commons Attribution License (CC BY). The use, distribution or reproduction in other forums is permitted, provided the original author(s) and the copyright owner(s) are credited and that the original publication in this journal is cited, in accordance with accepted academic practice. No use, distribution or reproduction is permitted which does not comply with these terms.
*Correspondence: M. Emilia Bravo, mebravo@gl.fcen.uba.ar