- 1National Oceanic and Atmospheric Administration (NOAA) Pacific Islands Fisheries Science Center, National Marine Fisheries Service, Honolulu, HI, United States
- 2Cooperative Institute for Marine and Atmospheric Research, University of Hawai’i, Honolulu, HI, United States
- 3Pacific Islands Ocean Observing System, University of Hawai’i, Honolulu, HI, United States
- 4Red Sea Research Center, King Abdullah University of Science & Technology, Thuwal, Saudi Arabia
The U.S. Pacific Islands span a dramatic natural gradient in climate and oceanographic conditions, and benthic community states vary significantly across the region’s coral reefs. Here we leverage a decade of integrated ecosystem monitoring data from American Samoa, the Mariana Archipelago, the main and Northwestern Hawaiian Islands, and the U.S. Pacific Remote Island Areas to evaluate coral reef community structure and reef processes across a strong natural gradient in pH and aragonite saturation state (Ωar). We assess spatial patterns and temporal trends in carbonate chemistry measured in situ at 37 islands and atolls between 2010 and 2019, and evaluate the relationship between long-term mean Ωar and benthic community cover and composition (benthic cover, coral genera, coral morphology) and reef process (net calcium carbonate accretion rates). We find that net carbonate accretion rates demonstrate significant sensitivity to declining Ωar, while most benthic ecological metrics show fewer direct responses to lower-Ωar conditions. These results indicate that metrics of coral reef net carbonate accretion provide a critical tool for monitoring the long-term impacts of ocean acidification that may not be visible by assessing benthic cover and composition alone. The perspectives gained from our long-term, in situ, and co-located coral reef environmental and ecological data sets provide unique insights into effective monitoring practices to identify potential for reef resilience to future ocean acidification and inform effective ecosystem-based management strategies under 21st century global change.
Introduction
Increasing concentrations of atmospheric carbon dioxide (CO2) are driving unprecedented shifts in global ocean carbon systems (Hoegh-Guldberg et al., 2007). Absorption of excess CO2 gas in ocean surface waters decreases seawater pH, carbonate ion concentration (CO32-), and the saturation state of the calcium carbonate mineral aragonite (Ωar), a process known as ocean acidification (Doney et al., 2009). In Pacific open ocean waters, significant decreasing trends in surface seawater pH have already been observed in the North Pacific Subtropical Gyre (-0.0019 yr-1 to -0.0016 yr-1 at Station ALOHA, Dore et al., 2009; Bates et al., 2014) and in the central equatorial Pacific (-0.0026 to -0.0018 yr-1, Sutton et al., 2014) over the past several decades. Dependent on future emissions scenarios, global climate models predict an additional decline in seawater pH of approximately 0.3 to 0.4 units by the end of the century (Kwiatkowski et al., 2020).
The impacts of ocean acidification are projected to have wide ranging effects across many marine ecosystems. Coral reefs are largely defined by calcium carbonate structure and engineered by organisms with known sensitivity to low pH and Ωar; therefore, they are likely to show major effects both in rates of organismal carbonate production as well as bioerosion and dissolution of existing carbonate structures (Kroeker et al., 2010; Kroeker et al., 2013; Hoegh-Guldberg et al., 2017). Most of our current understanding of coral reef ecosystem sensitivities to ocean acidification is derived from laboratory manipulation studies. Single-species and mesocosm perturbation experiments can provide direct evidence of coral reef taxa responses to shifts in carbonate chemistry in controlled environments, and numerous studies have shown that calcification rates of some (but not all) coral species, crustose coralline algae (CCA), and other calcifying marine taxa decline significantly with decreasing Ωar (Kuffner et al., 2008; Kroeker et al., 2010; Chan and Connolly, 2013; Kroeker et al., 2013; Manning et al., 2019). Paired with observations of accelerating rates of calcium carbonate dissolution and bioerosion under acidified conditions (Wisshak et al., 2012; Schönberg et al., 2017), many predictions for coral reef ecosystems under ocean acidification therefore converge on a paradigm of future reef communities that are algal-dominated, structurally simplified, and net eroding (Hoegh-Guldberg et al., 2017).
However, laboratory studies cannot fully replicate dynamic coral reef communities, where co-varying environmental, ecological, biogeographic, and anthropogenic factors may potentially magnify or mitigate the impacts of ocean acidification. Instead, multiple studies over the past decade have leveraged unique coral reef systems where natural processes produce sustained lower-pH/Ωar conditions, providing a glimpse over space of the possible trajectory of reef ecosystem states through time (Manzello et al., 2008; Fabricius et al., 2011; Crook et al., 2012; Inoue et al., 2013; Barkley et al., 2015; Enochs et al., 2015; Enochs et al., 2020). The expanding global portfolio of these natural “ocean acidification analog” sites has painted a more nuanced picture of coral reef ecosystems under acidified conditions, as the negative responses to decreasing Ωar frequently observed in laboratory experiments are not consistently realized on reefs as significant declines in the most commonly monitored benthic health metrics, including cover and diversity of hard corals, CCA, and other calcifying reef taxa. While perhaps demonstrative of the reef-scale variability in community sensitivity, this makes it challenging for long-term monitoring programs and coral reef managers to select, track, and manage the most appropriate indicators of ocean acidification impacts in coral reef ecosystems.
The coral reefs of the U.S-affiliated Pacific Islands provide a geographically expansive view of reef communities that exist across a large spatial gradient in carbonate chemistry conditions. The islands and atolls of the five U.S. Pacific Islands regions — American Samoa, the Mariana Archipelago, the main Hawaiian Islands, the Northwestern Hawaiian Islands, and the Pacific Remote Island Areas — are widely scattered across the western and central Pacific Ocean (~15° S to 30° N, ~140° E to 150° W) and thus experience a diversity of climate, oceanographic, and biogeochemical conditions. These range from the dynamic upwelling zones of the central equatorial Pacific Remote Island Areas to the more stable, oligotrophic Hawaiian and American Samoan reefs within the North and South Pacific Subtropical Gyres (Figure 1). Due in part to the range of climate and environmental conditions experienced, benthic community states also vary from coral-dominated to algal-dominated cover (Smith et al., 2016), in the composition of coral genera, and in rates of net calcium carbonate production (Vargas-Ángel et al., 2015). However, spatial and temporal patterns in carbonate chemistry and relationships with these benthic community metrics have, to date, remained more poorly constrained.
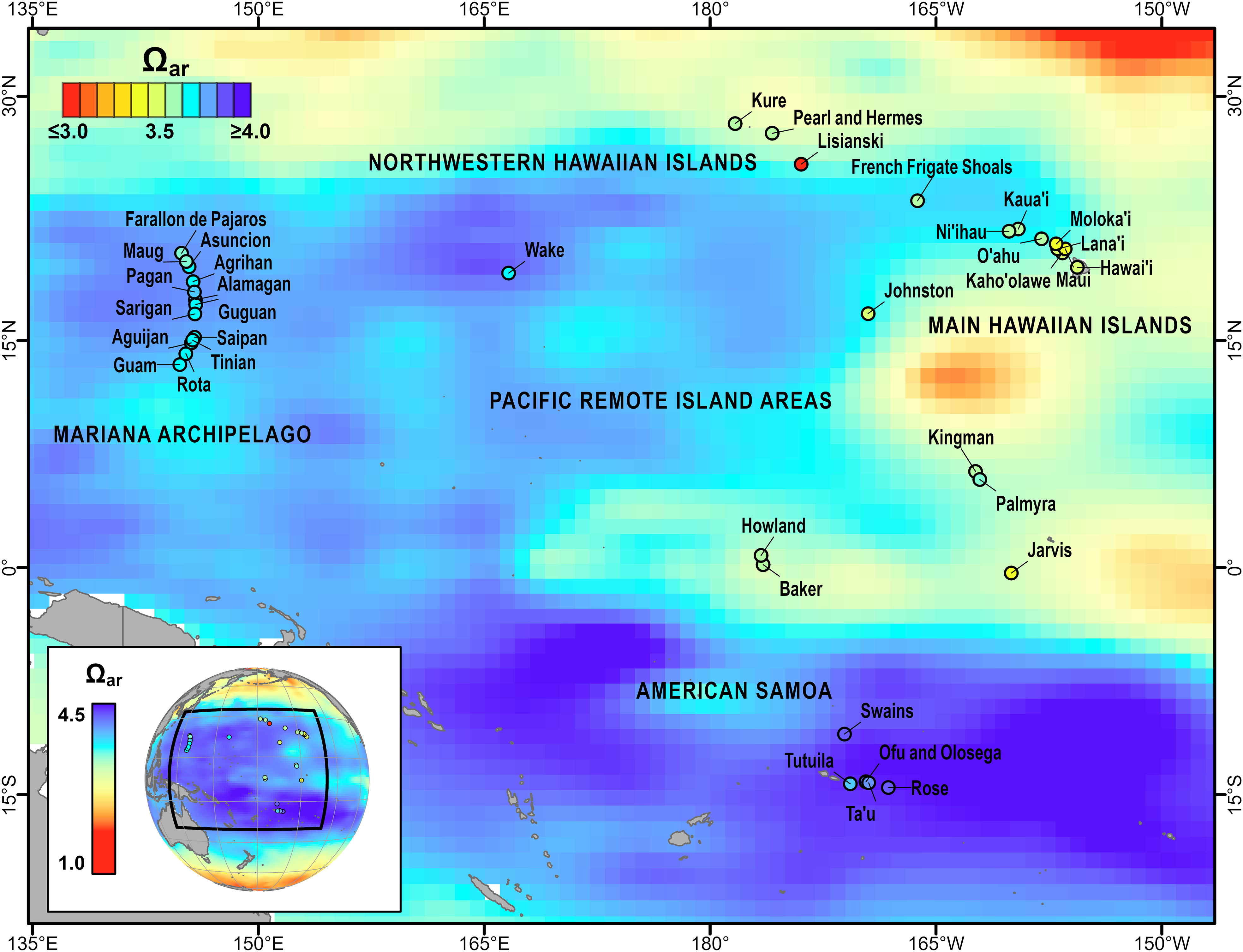
Figure 1 Climatological aragonite saturation state (Ωar) for the U.S. Pacific Islands region from the GLobal Ocean Data Analysis Project (GLODAP, 1973–2013) v2 (Lauvset et al., 2016), shown with global climatological Ωar (inset). Islands and atolls surveyed as part of NOAA’s Pacific RAMP/NCRMP are shown as points, with shading corresponding to the average 2010–2019 mean in situ Ωar. Modified and updated from Figure 6.3 in Jewett et al., 2020.
Here we employ the past decade (2010–2019) of National Oceanic and Atmospheric Administration (NOAA) integrated coral reef ecosystem monitoring data in the U.S. Pacific Islands to conduct a baseline analysis of acidification impacts on benthic community structure and function. We examine spatial and temporal patterns in carbonate chemistry measured in situ across 37 U.S. Pacific Islands and then evaluate the impacts of observed basin-scale Ωar variability on co-located 1) benthic cover, 2) genera and morphology of coral communities, and 3) rates of net carbonate accretion (production of calcium carbonate material). Our results allow us to identify the coral reef ecosystem metrics that co-vary most strongly with gradients in Ωar, to begin to project Pacific coral reef ecosystem sensitivities to future ocean acidification, and to evaluate the most relevant reef response metrics for ongoing monitoring and assessment of ocean acidification in reef environments.
Methods
Survey dates and sampling design
The NOAA Pacific Islands Fisheries Science Center has collected multi-disciplinary coral reef ecosystem monitoring data at U.S.-affiliated Pacific islands and atolls since 2000, initially as part of the NOAA Pacific Reef Assessment Monitoring Program (Pacific RAMP, 2000–2012) and currently under the NOAA National Coral Reef Monitoring Program (NCRMP, 2013–present). Robust, co-located monitoring of carbonate chemistry, benthic ecology, and carbonate accretion rates was initiated in 2010; thus, data collected between 2010 and 2019 were included in this study. Regions were surveyed during the following years: American Samoa: 2010, 2012, 2015, 2018; Mariana Archipelago: 2011, 2014, 2017; main Hawaiian Islands: 2010, 2013, 2016, 2019; Northwestern Hawaiian Islands: 2010, 2013, 2015, 2016, 2019; and the Pacific Remote Island Areas: 2010, 2012, 2015, 2016, 2017, 2018 (Wake only: 2011, 2014, 2017). Access to study sites, particularly remote islands, is dependent on ship availability, logistics, mission length, and suitable weather conditions. As a result, not every island was surveyed and/or data stream collected during a mission (see Supplementary Tables 1–11 for a full list of islands, sampling periods, and data streams collected).
NCRMP’s climate and ecological monitoring design integrates both permanent sites (surveyed during every sampling period) and sites selected using a stratified-random sampling design (surveyed only once). Permanent survey sites were established at approximately 15 m depth on the fore reef, with oceanographic and ecological instruments deployed on the benthos to establish long-term, site-specific time series. Stratified random sites for benthic ecological surveys were stratified based on reef zone and depth (0–30 m), with more sites randomly selected within strata that had larger hard bottom substrate area relative to the total domain. Most permanent and stratified random sites were located on the fore reef; however, sampling and surveys were also conducted at sites in lagoon, back reef, and protected reef slope environments where present. Full descriptions of NCRMP development, survey design, methods, and indicators are available in NOAA’s National Coral Reef Monitoring Plans (NOAA Coral Program, 2014; NOAA Coral Program, 2021) and Ocean Acidification Research Plans (Feely et al., 2010; Jewett et al., 2020).
Carbon system chemistry
Discrete water samples were collected at both permanent and stratified random NCRMP survey sites following the Carbon Dioxide Information Analysis Center Guide to Best Practices for Ocean CO2 Measurements (Dickson et al., 2007). Coral reef-associated water samples were collected during daylight hours (08:00–16:00) using a 5-L Niskin bottle deployed from a small boat or by divers at the surface (1 m depth) and at the benthos (~15 m depth). Samples were stored in 500 mL borosilicate bottles, where the sample bottle was allowed to overflow with seawater for at least one full volume and ~1% sample volume was removed to create a calibrated headspace. Samples were immediately poisoned with 200 µL of saturated mercuric chloride to inhibit biological activity and sample bottles sealed with glass stoppers that were lightly covered in Apiezon-L grease. Temperature, salinity, and pressure measurements were recorded by a co-located CTD profiler (2010–2018: Seabird 19+, 2019: RBR Concerto3). A total of 1,788 nearshore carbonate chemistry samples were collected between 2010 and 2019 (see Supplementary Figure 1 and Supplementary Tables 1–5 for a summary of water sample locations and years).
Seawater samples were stored indoors at room temperature for the duration of each field mission and then shipped to the NOAA Pacific Marine Environmental Laboratory in Seattle, WA, for dissolved inorganic carbon (DIC) and total alkalinity (TA) analysis. Coulometric titration for DIC was conducted using two Single Operator Multiparameter Metabolic Analyzer (SOMMA) systems, and two-stage, potentiometric, open-cell titration for TA was conducted using an instrument custom-built by the Dickson lab at Scripps Institution of Oceanography. Ωar was calculated from TA, DIC, temperature, salinity, and pressure using the R package seacarb (Gattuso et al., 2020) with the dissociation constants of Lueker et al. (2000). Concentrations of total phosphate and total silicate were assumed to be zero. Additional information on carbonate chemistry data collection and analysis can be found at Barkley et al. (2021).
Benthic cover and community composition
Stratified random benthic cover data were collected at each island or atoll using standard NCRMP sampling protocols (see Ayotte et al., 2015 and Winston et al., 2020 for full description of survey methodology). In brief, 30 photographs per site were collected at 1 m increments along a 30 m transect with a high-resolution digital camera (Cannon PowerShot 1200, S110 and G9 X) with underwater housings from 1 m above the benthos. Benthic data were extracted from photographs using fully manual human annotation in Coral Point Count with Excel extensions, CPCe (Kohler and Gill, 2006), from 2010–2014 and the web-based image annotation tool, CoralNet (Beijbom et al., 2015), from 2015–2019. For each image, the organism (genus/morphology for corals, genus/functional group for algae) or type of substrate was identified beneath each of ten randomly overlaid points. Points were pooled across all imagery to generate a total of 300 annotations per site (Lozada-Misa et al., 2017). A total of 6,136 surveys were conducted across all islands between 2010 and 2019 and included in this analysis (Supplementary Figure 2 and Supplementary Tables 6–10).
Site-level percent cover was calculated at the functional level, by coral morphology and by coral genera. The functional level included total hard coral, CCA, encrusting macroalgae (including calcifying Peyssonnelia sp.), Halimeda algae, macroalgae, sediment, and turf. Coral morphologies were categorized into branching, columnar, encrusting, foliose, free-living, massive, and table groups. Coral genera were subset to the five most common genera observed across the Pacific Islands region (Acropora, Montipora, Pavona, Pocillopora, and Porites). Stratified random surveys are not designed to be analyzed at the site level; therefore, site-level data were averaged to calculate mean cover across strata, then weighted by strata area, and summed for each island and year.
Carbonate accretion rates
Carbonate accretion rates were estimated using Calcification Accretion Units (CAUs) deployed at 220 permanent sites in 31 NCRMP islands between 2010 and 2019 (n = 1446 units; see Supplementary Figure 3 and Supplementary Table 11 for details on CAU deployment islands and years). Each CAU assembly consists of two 10 cm × 10 cm PVC plates separated by a 1 cm plastic spacer and fastened on a stainless steel rod (see Johnson et al., 2022). CAUs were installed on stainless steel stakes hammered into the reef substrate, secured with epoxy, and positioned at approximately 10–20 cm above the benthos. Five CAUs were installed per site, with typically 4–5 sites deployed per island and year (range: 1–13 sites) at approximately 15 m depth (range: 2.1–19.8 m depth). CAUs were recovered and replaced after a 2–3 year period (e.g., CAUs deployed in 2010 were recovered in 2012) and stored frozen at -5°C until prepared for processing.
Laboratory analysis was conducted at the NOAA Pacific Islands Fisheries Science Center in Honolulu, HI, following protocols outlined in Price et al. (2012) and Vargas-Ángel et al. (2015). CAU plates were defrosted, disassembled, and dried at 60°C until plate weight had stabilized, and then decalcified in 5% HCl until all calcified material had fully dissolved. Any remaining fleshy, non-calcified material was then scraped onto filter paper, vacuum-filtered, dried, and weighed. The weight of calcified material was calculated by subtracting the weight of the PVC plate and fleshy material from the total weight of the recovered unit and converted to an accretion rate based on time in water. CAU data were averaged to the site-level for statistical analysis. The cover and composition of organisms occupying recovered CAUs were not quantified as part of this study, although previous work has shown that CCA represents about two-thirds of recruited calcifying taxa, with small contributions from corals, algal crusts, and carbonate sediment (<5% each; Vargas-Ángel et al., 2015).
Data and statistical analysis
Carbonate chemistry and ecological data from five islands in the Mariana Archipelago with ≤ 3 water samples collected across all survey years were dropped from statistical analysis (Aguijan, Alamagan, Farallón de Pájaros, Guguan, and Sarigan; n = 10 samples). In addition, extreme outliers in the carbonate chemistry data (values more than three times the interquartile range below the first quartile or above the third quartile for each island and year; n = 26 samples) were removed. Variance component analysis was then conducted using the VCA package in R (Schuetzenmeister and Dufey, 2020) to attribute observed spatial and temporal variance in annual Ωar calculated from discrete water samples to the explanatory variables of depth, time of day (solar hour), region, island (nested within region), sampling period, and their interaction terms. Sampling period was represented by year groups (2010–2011, 2012–2014, 2015–2017, 2018–2019) rather than by numerical year.
Due to the relatively temporally sparse nature of NCRMP surveys, single carbonate chemistry data points collected at each site represent only a very brief snapshot of highly dynamic coral reef biogeochemical environments. Therefore, to more robustly characterize climatological carbonate chemistry as a driver that could meaningfully impact benthic communities over longer time periods, mean Ωar values were calculated across all depths for each island and year and compared to annual, island-level means for benthic composition. Beta regression models were constructed to evaluate the links between Ωar and the functional benthic cover categories (coral, CCA, turf, and macroalgae) using the R package betareg (Cribari-Neto and Zeileis, 2010). Cover data were converted to proportion data (constrained between 0 and 1) and square-root transformed. To account for mortality following the bleaching events that occurred at many islands, predominantly between 2013 and 2017, and to explore the contribution of potential outliers to statistical results, separate beta regression models were constructed for all years (2010–2019), the first sampling period for each island (2010–2012), the last sampling period for each island (2016–2019), and with and without the inclusion of the equatorial and near-equatorial Pacific Remote Islands (Baker, Howland, Jarvis, Kingman, and Palmyra). These upwelling-influenced islands were considered as possible outliers due to the more extreme mean values and inter-annual variability in carbonate chemistry, temperature, salinity, and biogeochemical conditions that could influence benthic community structure and function (Barkley et al., 2018). Multivariate benthic composition, coral morphology, and coral genera data were analyzed using distance based redundancy analysis (db-RDA) on dissimilarity matrices of square-root transformed data, and the significance of the relationship Ωar evaluated using permutation tests with 9999 permutations (R package vegan, Oksanen et al., 2020). Non-transformed and log-transformed 2010–2019 mean accretion rate data were fit to generalized linear models with Gaussian error distributions and a gamma error distribution with log link function. The generalized linear model using log-transformed accretion data and a Gaussian error distribution was found to best describe the relationship between Ωar and accretion rates based on quantile-quantile plots of the model residuals.
Results
Spatial and temporal patterns in carbonate chemistry
Carbonate chemistry showed significant spatial variability across the five U.S. Pacific Island regions (Figures 1, 2 and Supplementary Figures 4–6). Variance component analysis revealed that the largest component of observed variance in Ωar was spatially driven, with 51.1% of observed variance attributed to region and 18.6% of observed variance attributed to island nested within region (Supplementary Table 12). The highest Ωar levels were consistently observed at the five islands within American Samoa (2010–2019 island-mean range = 3.80–4.12). Lower mean Ωar values were observed in the Mariana Archipelago (3.60–3.86) and main Hawaiian Islands (3.30–3.65), and Ωar values did not deviate substantially from regional means across islands within these archipelagoes. In the Northwestern Hawaiian Islands, regional Ωar values were relatively low (2.55–3.80), with particularly low and variable Ωar conditions consistently observed at Lisianski (2.93 ± 0.10). The range in carbonate chemistry conditions was also fairly large across islands within the Pacific Remote Island Areas (3.14–3.90); long-term Ωar was lowest at Jarvis (3.33 ± 0.06) and Johnston (3.44 ± 0.04), slightly higher at Baker (3.55 ± 0.11), Howland (3.57 ± 0.10), Kingman (3.59 ± 0.08), and Palmyra (3.59 ± 0.07), and highest at Wake (3.78 ± 0.02).
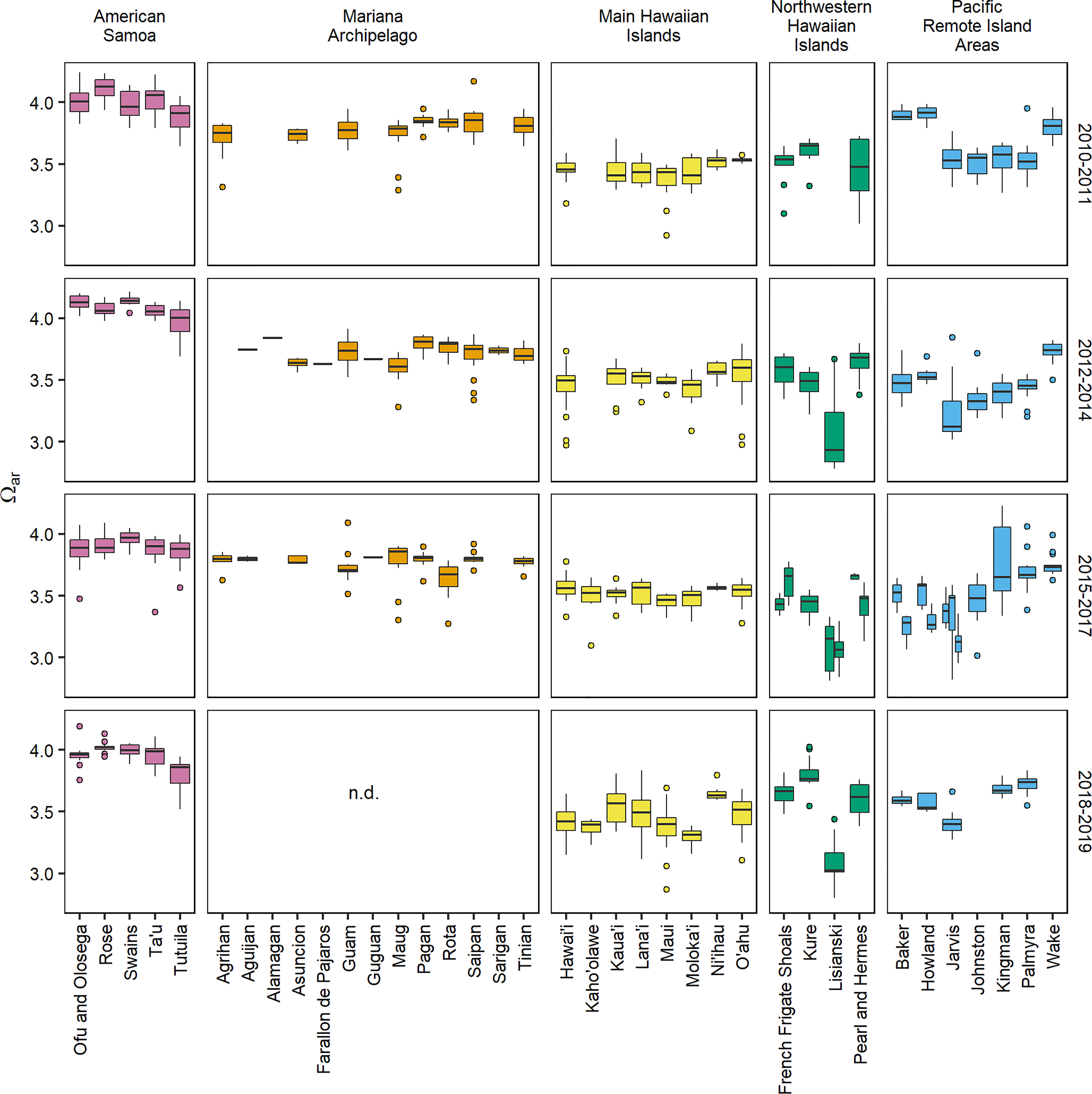
Figure 2 Aragonite saturation state (Ωar) data for 37 U.S. Pacific islands and atolls collected during four sampling periods (2010–2011, 2012–2014, 2015–2017, and 2018–2019). 2018–2019 data are not available for the Mariana Archipelago due to COVID-19 (“n.d.” = no data), and additional islands missing data were not sampled during the specified sampling period. Islands that were surveyed more than once during the 2015–2017 sampling period (French Frigate Shoals and Lisianski: 2015 and 2016, Howland and Baker: 2015 and 2017, Jarvis: 2015, 2016, and 2017) are shown with individual boxplots for each year. See Supplementary Figures 1–5 and Supplementary Tables 1–5 for a full description of sample collection years and carbonate chemistry parameter data.
Regional spatial patterns in carbonate chemistry were stable in most regions and islands through the time periods analyzed. Inter-annual change (year group) explained none (0%) of the variance across the full data set, with only small percentages of variance attributed to inter-annual change within region (interaction of year group and region, 4.6%), within island (interaction of island within region, 7.4%), sample depth (1.2%), and time of day (solar hour, 0.4%; Supplementary Table 12). The notable exceptions to this large-scale temporal stability were the equatorial islands of the Pacific Remote Island Areas (Jarvis, Howland, and Baker), where significant inter-annual swings in Ωar were observed across sampling periods. Particularly high Ωar values were observed at Howland and Baker in 2010 (3.90–3.91), and lower-Ωar conditions occurred at Howland, Baker, and Jarvis in 2017 (3.12–3.29).
Benthic responses to Ωar
Across the U.S. Pacific Islands, benthic cover and composition varied substantially across regions and islands (Supplementary Figures 7–10 and Supplementary Tables 6–10). Turf algae was the dominant type of benthic cover at 28 of the 37 Pacific islands surveyed. Only four islands in American Samoa (Swains, Ofu and Olosega, Tā‘u, and Tutuila) and five in the Pacific Remote Island Areas (Jarvis, Howland, Baker, Kingman, Palmyra) had greater percent cover of calcifiers than of turf. These patterns were generally consistent across years. Coral and CCA percent cover was highest in American Samoa and lower across the Mariana Archipelago, main Hawaiian Islands, and Northwestern Hawaiian Islands, although coral cover was notably high at Lisianski and French Frigate Shoals. In the Pacific Remote Island Areas, particularly low mean coral cover (7.5 ± 0.5%) and high CCA cover (26.2 ± 1.1%) were observed at Jarvis, as the 2010–2019 mean data include the cover data collected both before and after the severe 2015–2016 coral bleaching and mortality event (Barkley et al., 2018; Brainard et al., 2018; Vargas-Ángel et al., 2019).
The Pacific-wide gradient in Ωar was not meaningfully correlated with any benthic cover component across all sampling years (2010–2019) and all islands (Figure 3, Supplementary Figure 11, and Supplementary Table 13). Similar results were observed from analyses on the first (2010–2012) and last (2016–2019) sampling period for each island, with the exception of a negative relationship between Ωar and CCA that became significant during the last sampling period. With the equatorial and near-equatorial Pacific Remote Islands (Jarvis, Howland, Baker, Kingman, and Palmyra) removed from analysis, increasing Ωar was linked with significant increases in CCA cover and decreases in turf cover across all three sampling windows and with decreasing macroalgae cover in the last sampling period (2016–2019). Variability in Ωar was also not significantly related to multivariate benthic cover composition (db-RDA, pseudo-F = 1.45, p = 0.18), coral morphology (pseudo-F = 1.47, p = 0.20), or the composition of major coral genera (pseudo-F = 1.99, p = 0.08; Figure 4).
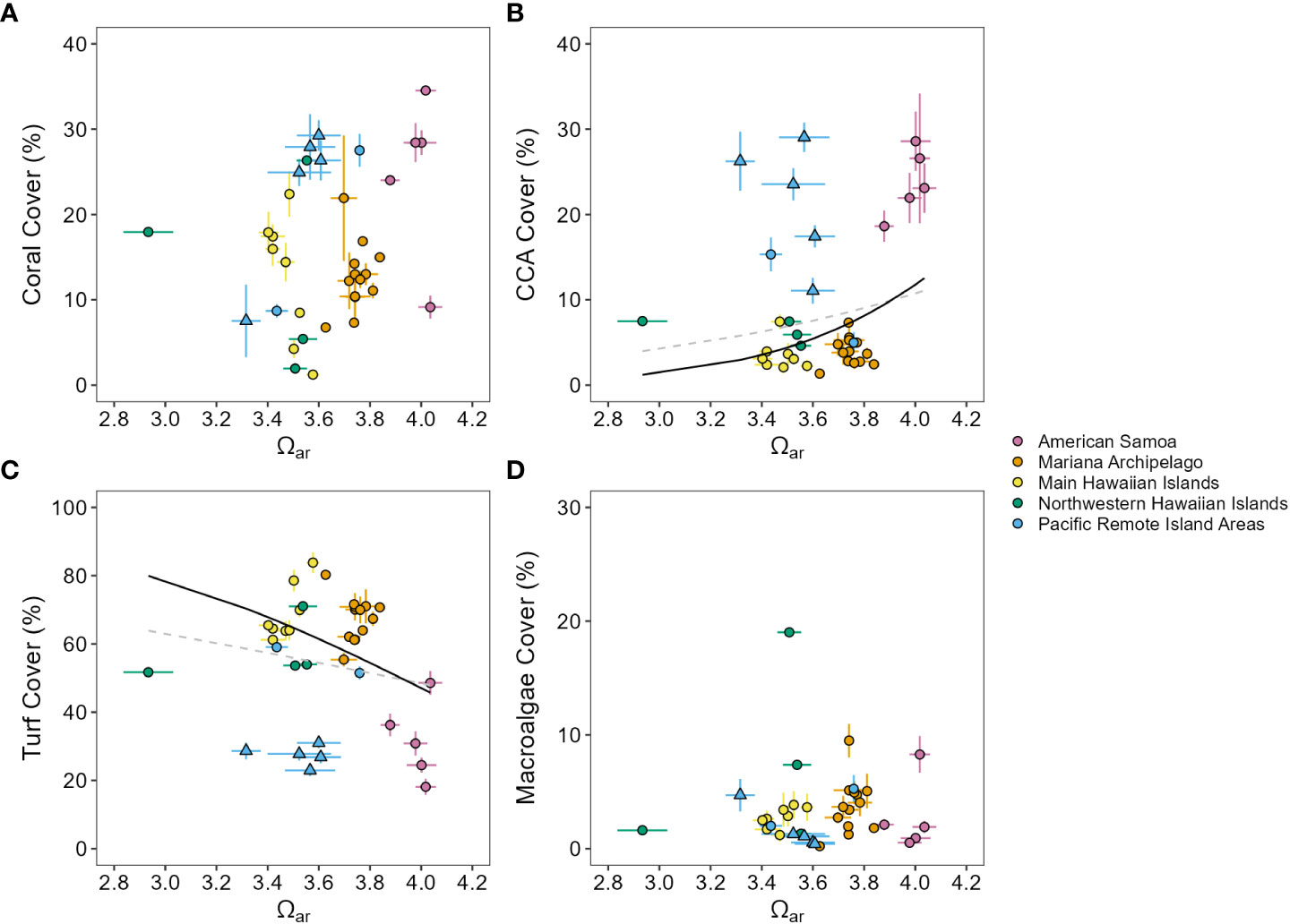
Figure 3 Benthic community responses across a Pacific-wide gradient in Ωar. Mean (± standard error) percent cover of (A) hard coral, (B) crustose coralline algae (CCA), (C) turf, and (D) macroalgae plotted against mean (± standard error) Ωar for each island (2010–2019 means). (B, C) Gray dashed lines show non-significant beta regression model fit for all data, and black lines show significant (solid, p ≤ 0.05) and non-significant (dashed, p > 0.05) beta regression model fit with equatorial and near-equatorial Pacific Remote Island Areas (triangles) removed; neither fit was significant for (A, D).
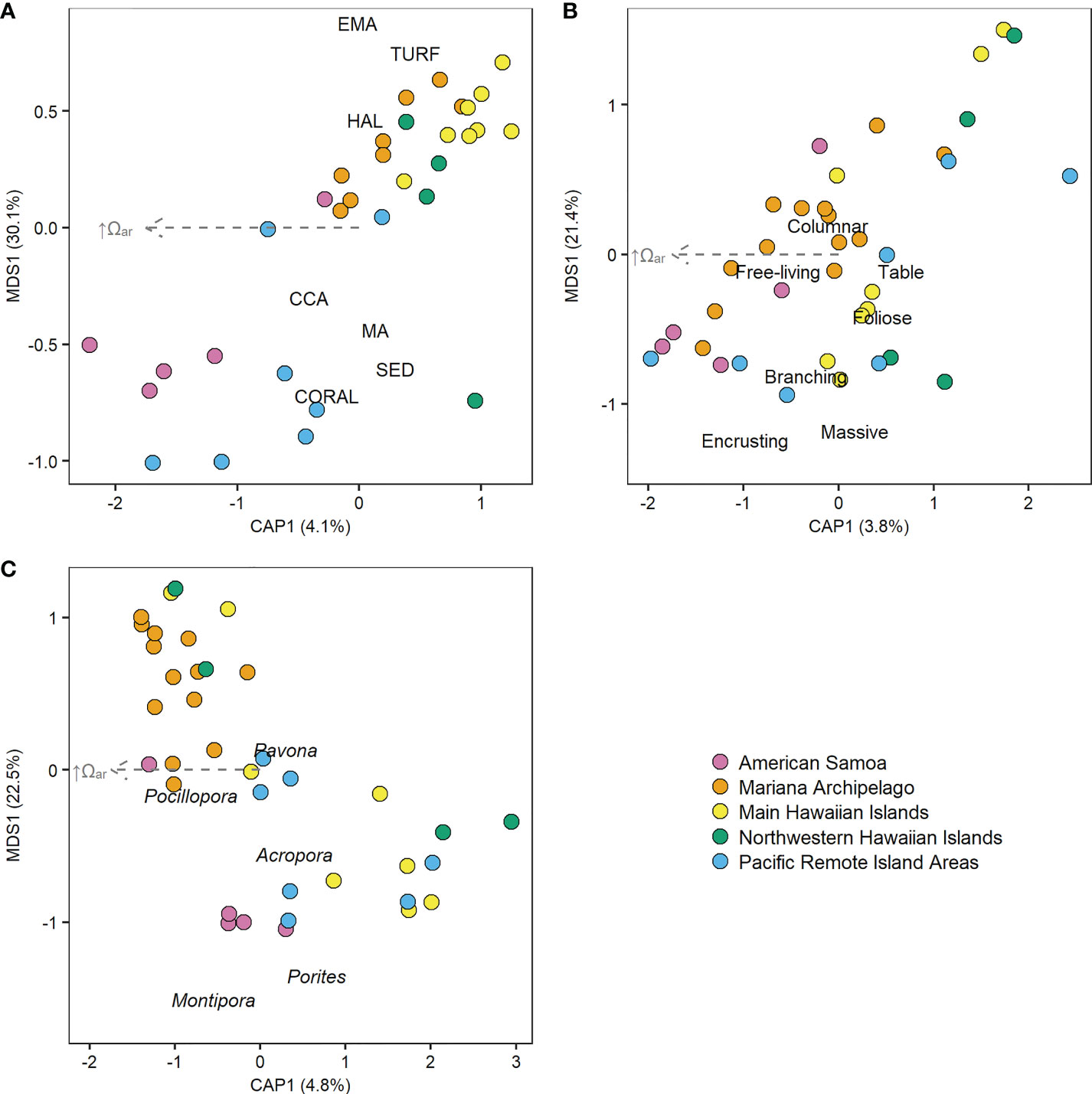
Figure 4 Distance-based redundancy analysis (db-RDA) plots of relationship between Ωar and (A) benthic percent cover, (B) coral morphology, and (C) composition of the five most common Pacific Islands coral genera. Points show 2010–2019 mean benthic data for each island colored by region, and gray arrows show the non-significant linear vectors of increasing Ωar. (A) CCA, crustose coralline algae; EMA, encrusting macroalgae; HAL, Halimeda algae; MA, macroalgae; SED, sediment.
Ωar impacts on net carbonate accretion rates
Reef carbonate accretion rates measured from CAU plates varied across the U.S. Pacific Islands region and showed significant sensitivity to spatial differences in carbonate chemistry conditions (Supplementary Figures 12, 13). Carbonate accretion rates increased exponentially with increasing Ωar (GLM with log-transformed response variable, estimate = 0.45, SE = 0.16, t = 2.89, R2 = 0.20, p = 0.007; Figure 5), and the model fit improved with the equatorial and near-equatorial Pacific Remote Island Areas (Jarvis, Howland, Baker, Kingman, and Palmyra) removed as outliers (estimate = 0.57, SE = 0.15, t = 3.93, R2 = 0.24, p < 0.0001). The fastest accretion rates (2010–2019 mean ± standard error = 121.2 ± 8.9 mg cm-2 yr-1) were observed at the highest Ωar site (Rose, mean Ωar = 4.05 ± 0.01), and were consistently high across the other four high-Ωar islands of American Samoa (53.2–99.8 mg cm-2 yr-1). The slowest accretion rates were observed in the Northwestern Hawaiian Islands at Pearl and Hermes (15.6 ± 1.2 mg cm-2 yr-1; Ωar = 3.51 ± 0.03). Accretion rates were also very low at Lisianski (24.2 ± 1.21 mg cm-2 yr-1), the island with lowest mean Ωar (Ωar = 2.96 ± 0.03). Accretion rates in the Mariana Archipelago (22.2–47.6 mg cm-2 yr-1) and main Hawaiian Islands (28.6–51.5 mg cm-2 yr-1) were intermediate. Carbonate accretion patterns in the Pacific Remote Island Areas were divided by latitude: the central equatorial Pacific Remote Island Areas (Jarvis, Howland, Baker, Kingman, and Palmyra) were among the highest-accreting islands assessed in the Pacific Islands (55.7–71.5 mg cm-2 yr-1), while carbonate accretion rates at the higher-latitude islands (Johnston: 19.1 ± 2.6 mg cm-2 yr-1 and Wake: 17.7 ± 2.4 mg cm-2 yr-1) were among the lowest. CCA cover was also significantly correlated with net carbonate accretion rates (Pearson’s ρ = 0.71, p < 0.001; Supplementary Figure 14).
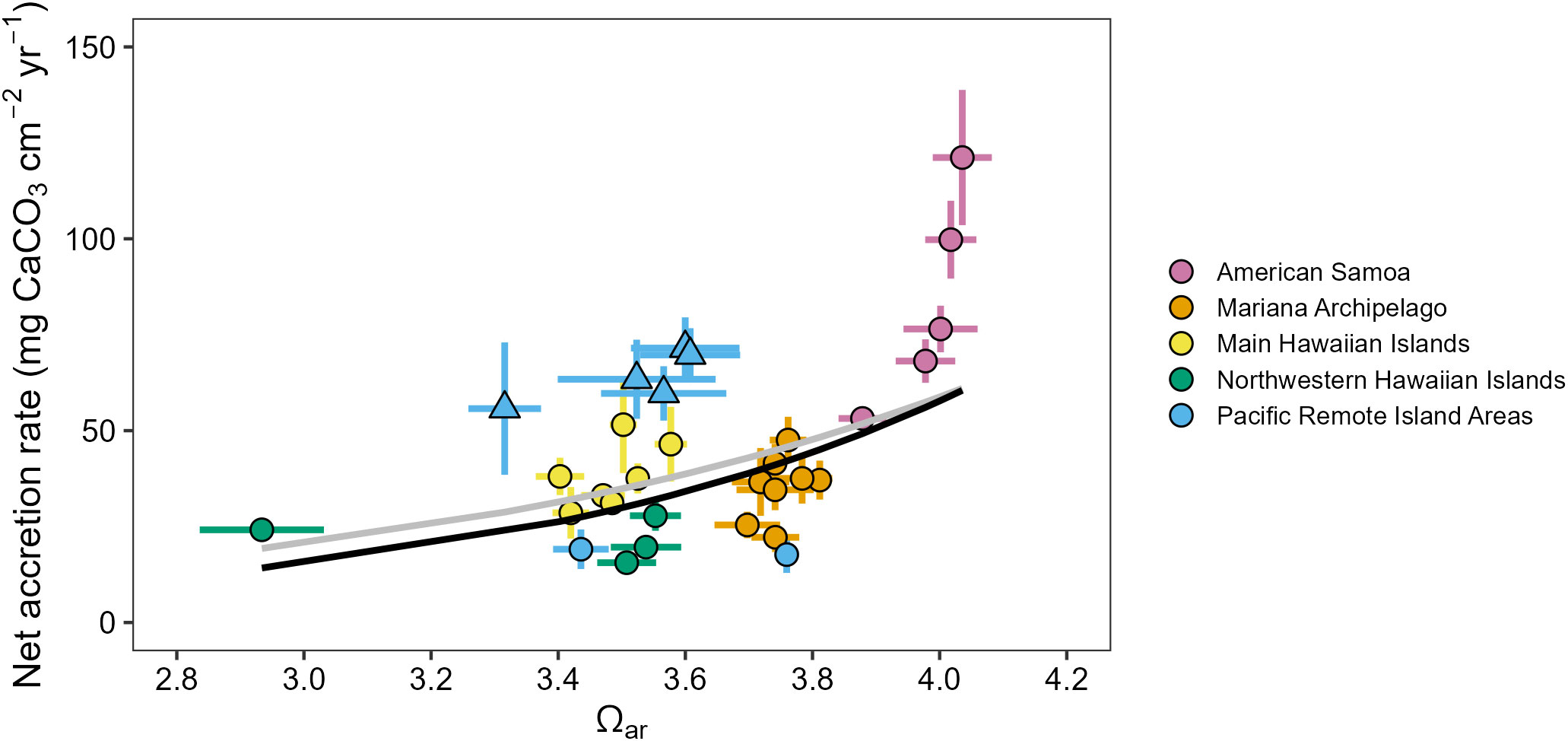
Figure 5 Mean (± standard error) in situ Ωar plotted against mean net calcium carbonate accretion rates measured from Calcification Accretion Units (CAUs) for 31 Pacific Islands from 2010–2019, shown with significant fitted exponential relationships between Ωar and accretion rates for all islands (gray line) and with equatorial and near-equatorial Pacific Remote Island Areas (triangles) removed (black line).
Discussion
A decade of co-located biogeochemical and benthic data collected in the U.S. Pacific Islands reveal stable, regional-scale spatial patterns in the mean carbonate chemistry of nearshore coral reef environments. These data also show a strong gradient in mean Ωar (Ωar = 2.96–4.05) that spans the high-Ωar reefs of American Samoa to the lower-Ωar conditions present in the Hawaiian Archipelago and Pacific Remote Island Areas. This gradient in Ωar is driven by spatial variability in temperature, salinity, total alkalinity, and dissolved inorganic carbon, which previous basin-scale analyses have shown are in turn influenced by regional-scale differences in physical (precipitation/evaporation, air-sea exchange of CO2), oceanographic (upwelling, vertical mixing), and biological (photosynthesis/respiration, calcification/dissolution) processes (Feely et al., 2002; Kuchinke et al., 2014). Regional spatial patterns in Ωar derived from in situ samples are broadly consistent with Pacific climatology (e.g., the Global Ocean Data Analysis Project, Lauvset et al., 2016), although nearshore Ωar values are generally depressed relative to offshore climatological estimates due to coral reef biological activity (Figure 1).
Most of the samples and surveys conducted as part of this study occurred on the fore reef; the steep bathymetric slopes of most Pacific Islands (Gove et al., 2016) lead to shallow waters (0–30 m) on the fore reef that are generally well-flushed, well-mixed, and minimally variable during diurnal hours. However, NCRMP water chemistry monitoring data highlight the reef systems where localized oceanographic, hydrodynamic, and/or biological processes uniquely impact nearshore carbonate chemistry. For example, in the Northwestern Hawaiian Islands, upwelling and internal waves drive low and variable Ωar, particularly at Pearl and Hermes (Vroom and Braun, 2010). In addition, long residence times of seawater pooled on Lisianski’s shallow Neva Shoals likely magnify calcification and respiration driven draw down of Ωar, producing unusually low Ωar values within the Northwestern Hawaiian Islands (e.g., Shamberger et al., 2014; Silbiger et al., 2017). In the equatorial Pacific Remote Island Areas of Jarvis, Howland, and Baker, combined equatorial upwelling and topographic upwelling of the Equatorial Undercurrent produce cool, low-Ωar, and high-nutrient nearshore environments (Gove et al., 2006). Inter-annual variability in the El Niño Southern Oscillation (ENSO) dynamically changes oceanographic conditions on these reefs, shifting between low-Ωar during La Niña periods when upwelling of cool, low-Ωar deeper water intensifies (e.g., 2017) to higher-Ωar during El Niño events (e.g., 2010) when upwelling weakens and temperatures and Ωar rise (Barkley et al., 2018).
Over the ten years and four sampling periods covered in this analysis, only minimal variance in carbonate chemistry was attributable to temporal factors. This demonstrates that the strong spatial patterns in carbonate chemistry were relatively stable inter-annually in most regions between 2010 and 2019, with the exception of the ENSO-influenced Pacific Remote Island Areas. NCRMP discrete water chemistry data — collected at each island over a few days once every one to three years — represent a snapshot of reef conditions during daylight hours and can be used to estimate climatological conditions at each island when averaged across multiple sampling years. Critically, however, these data cannot be used as robust evidence for or against a significant linear change in Ωar during this time period, such as a decline in Ωar predicted under ocean acidification. Longer, higher-resolution time series, such as those from pCO2 moorings, are required to accurately detect and resolve ocean acidification amidst the tidal, diel, seasonal, and inter-annual variability that is present in highly dynamic nearshore coral reef waters (Sutton et al., 2014; Sutton et al., 2019). Currently, Moored Autonomous pCO2 (MAPCO2) buoys are deployed at two NCRMP permanent sites in the U.S. Pacific (Kāne‘ohe Bay, O‘ahu, Hawai‘i and Fagatele Bay, Tutuila, American Samoa), and time series data generated from these moorings will provide important insights into the progression of pH change in nearshore waters over the next several decades (Terlouw et al., 2019).
Despite the gradient in Ωar across the U.S. Pacific Islands, most of the island-scale benthic cover metrics assessed in this study were not strongly driven by spatial differences in Ωar. Island-scale benthic community composition, including cover of reef calcifiers (corals and CCA) and algae, coral morphological composition, and the composition of major coral genera were not significantly related to mean Ωar levels across all regions. Removing the equatorial and near-equatorial Pacific Remote Island Areas from analysis did result in significant relationships between Ωar and cover of CCA and turf algae, where CCA cover increased and turf algae cover decreased in areas of lower Ωar. This may be indicative of a potential transition from higher CCA cover at higher-Ωar toward more abundant turf algae at lower-Ωar in some non-equatorial regions. Both coral and fleshy macroalgae cover remained unresponsive to Ωar across all analyses considered, suggesting that the cover of these benthic groups is less responsive to decreasing Ωar.
Together, these observed responses provide some evidence of a possible link between carbonate chemistry and benthic composition. However, it is more likely that Ωar is not a simple, dominant driver of most benthic metrics over this range in Ωar and at the spatial and temporal scales analyzed here. Furthermore, other environmental and ecological factors (e.g., temperature, productivity, light, wave exposure, anthropogenic impacts) likely have a stronger influence — particularly in the upwelling-influenced Pacific Remote Island Areas — in shaping coral reef communities across the U.S. Pacific Islands region.
Considered across a basin-scale carbonate chemistry gradient, the coral reefs of the U.S. Pacific Islands exhibit few of the benthic community responses that are often reported across other natural acidification gradients. Although not observed here, decreasing Ωar has been linked to lower hard coral cover in some (Manzello et al., 2008; Crook et al., 2012; Inoue et al., 2013; Enochs et al., 2015), but not all (Fabricius et al., 2011; Barkley et al., 2015; Enochs et al., 2020), locations. Declines in CCA cover (Fabricius et al., 2011; Fabricius et al., 2015; Smith et al., 2020), increasing macroalgae cover (Fabricius et al., 2011; Enochs et al., 2015; Smith et al., 2020), and decreasing abundance of complex coral morphologies (Fabricius et al., 2011; Strahl et al., 2016) at low-Ωar are also common, but these benthic community trends are not consistently present in the U.S. Pacific Islands during the 2010–2019 time period. Evidence of Porites coral tolerance of lower-Ωar levels (Fabricius et al., 2011; Crook et al., 2012; Barkley et al., 2015; Enochs et al., 2015; Strahl et al., 2016) was the only benthic community response variable that emerged as a shared benthic community response across this and other gradient studies. None of these systems are perfect analogs for future ocean acidification, and differences in spatial and temporal scales analyzed, divergent mechanisms for localized acidification, co-variance with other environmental factors, and variation in adaptive capacity likely confound a unified benthic community signal to acidified conditions. Moreover, because the range of the Ωar gradient (Ωar = 2.96–4.05) is still less extreme than at most of these sites, it is also possible that current Ωar levels across the U.S. Pacific Islands have not yet exceeded the threshold beyond which these major benthic community shifts are expected to occur.
In contrast to the few significant changes in coral reef community composition, net carbonate accretion rates decreased exponentially over the range of mean Ωar experienced by benthic communities, showing the most sensitivity of any ecosystem response we observed across the U.S. Pacific Islands carbonate chemistry gradient. Net accretion rates derived from CAUs largely reflect calcification rates of CCA (Vargas-Ángel et al., 2015). Our field observations thus support the results of many experimental studies that have demonstrated that CCA is likely to be among the coral reef taxa most susceptible to ocean acidification. This elevated sensitivity is due to the calcified algae’s highly soluble, high Mg-calcite skeletons that dissolve more rapidly under acidified conditions than aragonitic coral skeletons, which can in turn reduce productivity, calcification, recruitment, and abundance (Kuffner et al., 2008; Diaz-Pulido et al., 2014; Ordoñez et al., 2014; Fabricius et al., 2015; Dutra et al., 2016; Manning et al., 2019). As CCA plays an important ecological role in both reef cementation and coral recruitment (Price, 2010; Manning et al., 2019), their vulnerability to ocean acidification will likely also threaten future reef ecosystem persistence.
Carbonate accretion rate data derived from CAUs serve as a proxy for rates of reef ecosystem net carbonate production. Caution should be applied in scaling up these observations, as calcifier recruits to CAU settlement plates may not reflect community structure in the adjacent benthos and reef accretion rates can vary across depths and reef zones (Vargas-Ángel et al., 2015; Johnson et al., 2022; Kench et al., 2022). Nevertheless, reef-scale rates of net ecosystem calcification have been shown to similarly track with Ωar (DeCarlo et al., 2017), demonstrating that net carbonate production may be a leading indicator of coral reef community responses to ocean acidification. Across the Pacific Islands, accretion rates were generally higher at islands with higher cover of CCA. Yet, the lower net accretion rates observed with decreasing Ωar were not directly reflected in the cover of reef calcifiers. Together, these observations suggest that patterns in benthic cover and composition potentially lag or are decoupled from trends in reef carbonate accretion rates (Page et al., 2017).
The analysis presented here focuses specifically on carbonate chemistry as a driver of coral reef benthic composition and function. However, Ωar is one of several environmental and anthropogenic drivers, including sea surface temperature (and thermal stress), productivity, and human population density, that co-vary across the U.S. Pacific Islands region and impact coral reef communities (Williams et al., 2015; Smith et al., 2016). Given the lack of a strong relationship between carbonate chemistry and most benthic response variables over the carbonate chemistry gradient examined, it is likely that these factors have a greater impact than Ωar in shaping benthic community composition than Ωar alone, either independently or in combination. This is especially likely in the equatorial Pacific Remote Island Areas, where carbonate accretion rates are higher than might be expected based solely on Ωar levels and where calcification rates are likely enhanced by the upwelling of high-nutrient water (Gove et al., 2006; Silverman et al., 2007; Johnson et al., 2020).
Bleaching events occurred in all U.S. Pacific Islands regions, but not at all islands, between 2010 and 2019. Particularly severe impacts were documented in the Pacific Remote Island Areas (Vargas-Ángel et al., 2011; Barkley et al., 2018; Brainard et al., 2018; Vargas-Ángel et al., 2019), Hawaiian Archipelago (Kramer et al., 2016; Couch et al., 2017; Winston et al., 2022), Guam (Raymundo et al., 2019), and the Northern Mariana Islands (Carilli et al., 2020). The stable, insignificant relationship between benthic cover and Ωar during the past decade suggests that these recent heat stress events have not yet influenced the relationship between carbonate chemistry and the benthos. However, this state is unlikely to persist. Increasingly frequent and severe episodic marine heatwaves over the next few decades are predicted to directly drive declines in both coral cover and net carbonate production (Cornwall et al., 2021). While ocean acidification may not ultimately be the dominant driver of future reef states, it may act chronic stressor that reduces coral calcification rates, calcifier productivity, and reef structural integrity, thus amplifying reef sensitivity to warming and/or hindering ecosystem recovery potential following bleaching events (Klein et al., 2022).
Overall, these results suggest that the most immediate and significant impacts of ocean acidification may become evident first in reef community processes (e.g., calcification and accretion rates) rather than in benthic cover and composition. Especially when considered at the island scale, these common ecological metrics are not as clearly linked to shifts in carbonate chemistry. Future targeted monitoring efforts should consider prioritizing metrics of coral reef ecosystem sensitivity to ocean acidification that include estimates of net community carbonate production and erosion — using networks of CAUs, hydrochemical approaches, and/or census-based carbonate budgets (Courtney et al., 2016; Lange et al., 2020) — to more robustly characterize reef function under changing carbonate chemistry conditions. Tracking these more sensitive reef community processes can help identify areas of vulnerability or resilience in coral reef communities. In addition, strategic and sustained monitoring of these parameters can provide early warning of progressive ocean acidification impacts on coral reefs, and thus increase the number of potential interventions and time available to initiate proactive management prior to major shifts in benthic community structure.
Data availability statement
The data included in this study are summarized in the Supplementary Material and archived in online repositories at NOAA’s National Centers for Environmental Information (https://www.ncei.noaa.gov/) under the following accession numbers: 0157714, 0157715, 0157716, 0159169, 0159171, 0160330, 0175227, 0175228, 0175229, 0175230, 0225980, 0226794, 0226976, 0232262 (carbonate chemistry); 0157752, 0159140, 0159148, 0159158, 0159165, 0164295, 0176560, 0176574, 0217940 (benthic composition); 0157722, 0157758, 0159149, 0159151, 0159156, 0175165, 0175166, 0175167, 0231439, 0233019, 0255269 (carbonate accretion).
Author contributions
Over the period of the project, HB and TO acted as successive Principal Investigators of the synthesis project. As a team, they conceived, designed and performed initial synthesis analyses. HB further acted as primary author of the manuscript and led data analysis and writing. TO secured funding and contributed to and edited the manuscript. CY and RB conceived the project survey and sampling design. HB, TO, AH, NP, CY, and CC collected field data. HB and JNS were responsible for the processing, analysis, management, and archival of carbonate chemistry data. CC provided benthic data. AH and RW were responsible for laboratory analysis and management of carbonate accretion data. HB and TO conducted statistical analysis. RB and JCS assisted with overall project management. All authors contributed to the article and approved the submitted version.
Funding
This work was funded by the NOAA Ocean Acidification Program and the NOAA Coral Reef Conservation Program (project #743), as part of the NOAA National Coral Reef Monitoring Program.
Acknowledgments
We thank the officers and crew of the NOAA Ships Hiʻialakai, Oscar Elton Sette, and Rainier for providing operational support for Pacific RAMP/NCRMP missions, as well as the numerous NOAA scientific staff and partners who have collected field data over a decade of long-term coral reef monitoring surveys. We thank Kevin Trick, Annette DesRochers, Michael Akridge, and Brooke Olenski for extensive assistance with the management, quality control, and archival of data; Dana Greeley, Morgan Ostendorf, Julian Herndon, and Simone Alin for conducting laboratory analysis of carbonate chemistry samples; and Brittany Huntington and Bernardo Vargas-Ángel for advice on benthic data analysis and interpretation. Pacific RAMP/NCRMP ocean acidification sampling design, survey methodologies, and early data collection were designed, developed, and implemented with input from Richard Feely, Christopher Sabine, Elizabeth Jewett, Adrienne Sutton, Simone Alin, and in close collaboration with the NOAA Ocean Acidification Program and NOAA Coral Reef Conservation Program. We also acknowledge the many partner agencies — including the Papahānaumokuākea Marine National Monument, Pacific Remote Islands Marine National Monument, Rose Atoll Marine National Monument, Marianas Trench Marine National Monument, US Fish and Wildlife Service, US National Park Service, American Samoa Department of Marine and Wildlife Resources, National Marine Sanctuary of American Samoa, State of Hawaii Department of Land and Natural Resources, Commonwealth of the Northern Mariana Islands (CNMI) Division of Fish and Wildlife, CNMI Division of Coastal Resources Management, and Guam Division of Aquatic and Wildlife Resources — who have supported field operations, provided input on research priorities, and granted research permits to collect the data presented here.
Conflict of interest
The authors declare that the research was conducted in the absence of any commercial or financial relationships that could be construed as a potential conflict of interest.
Publisher’s note
All claims expressed in this article are solely those of the authors and do not necessarily represent those of their affiliated organizations, or those of the publisher, the editors and the reviewers. Any product that may be evaluated in this article, or claim that may be made by its manufacturer, is not guaranteed or endorsed by the publisher.
Supplementary material
The Supplementary Material for this article can be found online at: https://www.frontiersin.org/articles/10.3389/fmars.2022.991685/full#supplementary-material
References
Ayotte P., McCoy K., Heenan A., Williams I., Zamzow J. (2015). Coral reef ecosystem program standard operating procedures: Data collection for rapid ecological assessment fish surveys. Honolulu: U.S. Department of Commerce, National Oceanic and Atmospheric Administration. doi: 10.7289/v5sn06zt.
Barkley H. C., Cohen A. L., Golbuu Y., Starczak V. R., DeCarlo T. M., Shamberger K. E. F. (2015). Changes in coral reef communities across a natural gradient in seawater pH. Sci. Adv. 1, e1500328. doi: 10.1126/sciadv.1500328
Barkley H. C., Cohen A. L., Mollica N. R., Brainard R. E., Rivera H. E., DeCarlo T. M., et al. (2018). Repeat bleaching of a central pacific coral reef over the past six decades, (1960–2016). Commun. Biol. 1, 1–10. doi: 10.1038/s42003-018-0183-7
Barkley H. C., Halperin A. A., Smith J. N., Weible R., Pomeroy N. (2021). National coral reef monitoring program: Dissolved inorganic carbon, total alkalinity, pH and other variables collected from surface discrete measurements using coulometer, alkalinity titrator and other instruments from the Hawaiian archipelago, from 2019-04-21 to 2019-09-05 (NCEI Accession 0226794). National Oceanic and Atmospheric Administration, National Centers for Environmental Information. Available at: https://www.ncei.noaa.gov/data/oceans/ncei/ocads/metadata/0226794.html.
Bates N. R., Astor Y. M., Church M. J., Currie K., Dore J. E., Gonzalez-Davila M., et al. (2014). A time-series view of changing surface ocean chemistry due to ocean uptake of anthropogenic CO₂ and ocean acidification. Oceanography 27, 126–141. doi: 10.5670/oceanog.2014.16
Beijbom O., Edmunds P. J., Roelfsema C., Smith J., Kline D. I., Neal B. P., et al. (2015). Towards automated annotation of benthic survey images: Variability of human experts and operational modes of automation. PLoS One 10, e0130312. doi: 10.1371/journal.pone.0130312
Brainard R. E., Oliver T., McPhaden M. J., Cohen A., Venegas R., Heenan A., et al. (2018). Ecological impacts of the 2015/16 El niño in the central equatorial pacific. Bull. Amer. Meteor. Soc. 99, S21–S26. doi: 10.1175/BAMS-D-17-0128.1
Carilli J. E., Bolick L., Marx D. E., Smith S. H., Fenner D. (2020). Coral bleaching variability during the 2017 global bleaching event on a remote, uninhabited island in the western pacific: Farallon de medinilla, commonwealth of the northern Mariana islands. Bull. Mar. Sci. 96, 785–802. doi: 10.5343/bms.2019.0083
Chan N. C. S., Connolly S. R. (2013). Sensitivity of coral calcification to ocean acidification: A meta-analysis. Glob Change Biol. 19, 282–290. doi: 10.1111/gcb.12011
Cornwall C. E., Comeau S., Kornder N. A., Perry C. T., van Hooidonk R., DeCarlo T. M., et al. (2021). Global declines in coral reef calcium carbonate production under ocean acidification and warming. Proc. Natl. Acad. Sci. 118, e2015265118. doi: 10.1073/pnas.2015265118
Couch C. S., Burns J. H. R., Liu G., Steward K., Gutlay T. N., Kenyon J., et al. (2017). Mass coral bleaching due to unprecedented marine heatwave in papahānaumokuākea marine national monument (Northwestern Hawaiian islands). PLoS One 12, e0185121. doi: 10.1371/journal.pone.0185121
Courtney T. A., Andersson A. J., Bates N. R., Collins A., Cyronak T., de Putron S. J., et al. (2016). Comparing chemistry and census-based estimates of net ecosystem calcification on a rim reef in Bermuda. Front. Mar. Sci. 3, 181. doi: 10.3389/fmars.2016.00181
Cribari-Neto F., Zeileis A. (2010). Beta regression in r. J. Stat. Softw 34, 1–24. doi: 10.18637/jss.v034.i02
Crook E. D., Potts D., Rebolledo-Vieyra M., Hernandez L., Paytan A. (2012). Calcifying coral abundance near low-pH springs: Implications for future ocean acidification. Coral Reefs 31, 239–245. doi: 10.1007/s00338-011-0839-y
DeCarlo T. M., Cohen A. L., Wong G. T. F., Shiah F.-K., Lentz S. J., Davis K. A., et al. (2017). Community production modulates coral reef pH and the sensitivity of ecosystem calcification to ocean acidification. J. Geophys Res. Oceans 122, 745–761. doi: 10.1002/2016JC012326
Diaz-Pulido G., Nash M. C., Anthony K. R. N., Bender D., Opdyke B. N., Reyes-Nivia C., et al. (2014). Greenhouse conditions induce mineralogical changes and dolomite accumulation in coralline algae on tropical reefs. Nat. Commun. 5, 3310. doi: 10.1038/ncomms4310
Dickson A. G., Sabine C. L., Christian J. R. (2007). Guide to best practices for ocean CO2 measurements. PICES Spec. Publ. 3, 191.
Doney S. C., Fabry V. J., Feely R. A., Kleypas J. A. (2009). Ocean acidification: The other CO2 problem. Annu. Rev. Mar. Sci. 1, 169–192. doi: 10.1146/annurev.marine.010908.163834
Dore J. E., Lukas R., Sadler D. W., Church M. J., Karl D. M. (2009). Physical and biogeochemical modulation of ocean acidification in the central north pacific. Proc. Natl. Acad. Sci. 106, 12235–12240. doi: 10.1073/pnas.0906044106
Dutra E., Koch M., Peach K., Manfrino C. (2016). Tropical crustose coralline algal individual and community responses to elevated pCO2 under high and low irradiance. ICES J. Mar. Sci. 73, 803–813. doi: 10.1093/icesjms/fsv213
Enochs I. C., Formel N., Manzello D., Morris J., Mayfield A. B., Boyd A., et al. (2020). Coral persistence despite extreme periodic pH fluctuations at a volcanically acidified Caribbean reef. Coral Reefs 39, 523–528. doi: 10.1007/s00338-020-01927-5
Enochs I. C., Manzello D. P., Donham E. M., Kolodziej G., Okano R., Johnston L., et al. (2015). Shift from coral to macroalgae dominance on a volcanically acidified reef. Nat. Clim Change 5, 1083–1088. doi: 10.1038/nclimate2758
Fabricius K. E., Kluibenschedl A., Harrington L., Noonan S., De’ath G. (2015). In situ changes of tropical crustose coralline algae along carbon dioxide gradients. Sci. Rep. 5, 9537. doi: 10.1038/srep09537
Fabricius K. E., Langdon C., Uthicke S., Humphrey C., Noonan S., De’ath G., et al. (2011). Losers and winners in coral reefs acclimatized to elevated carbon dioxide concentrations. Nat. Clim Change 1, 165–169. doi: 10.1038/nclimate1122
Feely R. A., Boutin J., Cosca C. E., Dandonneau Y., Etcheto J., Inoue H. Y., et al. (2002). Seasonal and interannual variability of CO2 in the equatorial pacific. Deep Sea Res. Part II Top. Stud. Oceanogr 49, 2443–2469. doi: 10.1016/S0967-0645(02)00044-9
Feely R. A., Wanninkhof R., Stein J. E., Sigler M. F., Jewett E. B., Arzayus L. F., et al. (2010). NOAA Ocean and Great Lakes Acidification Research Plan. Washington DC, U.S. Department of Commerce, National Oceanic and Atmospheric Administration.
Gattuso J.-P., Epitalon J.-M., Lavigne H., Orr J. (2020). Seacarb: Seawater Carbonate Chemistry. R Package Version 3.2.13. Available at: https://cran.r-project.org/web/packages/seacarb/index.html.
Gove J. M., McManus M. A., Neuheimer A. B., Polovina J. J., Drazen J. C., Smith C. R., et al. (2016). Near-island biological hotspots in barren ocean basins. Nat. Commun. 7, 10581. doi: 10.1038/ncomms10581
Gove J. M., Merrifield M. A., Brainard R. E. (2006). Temporal variability of current-driven upwelling at Jarvis island. J. Geophys Res. Oceans 111, C12011. doi: 10.1029/2005JC003161
Hoegh-Guldberg O., Mumby P. J., Hooten A. J., Steneck R. S., Greenfield P., Gomez E., et al. (2007). Coral reefs under rapid climate change and ocean acidification. Science 318, 1737–1742. doi: 10.1126/science.1152509
Hoegh-Guldberg O., Poloczanska E. S., Skirving W., Dove S. (2017). Coral reef ecosystems under climate change and ocean acidification. Front. Mar. Sci. 4, 158. doi: 10.3389/fmars.2017.00158
Inoue S., Kayanne H., Yamamoto S., Kurihara H. (2013). Spatial community shift from hard to soft corals in acidified water. Nat. Clim Change 3, 683–687. doi: 10.1038/nclimate1855
Jewett E. B., Osbourne E. B., Arzayus K. M., Osgood K., DeAngelo J., Mintz J. M. (2020) NOAA Ocean, coastal, and great lakes acidification research plan: 2020-2029. Available at: https://oceanacidification.noaa.gov/ResearchPlan2020.
Johnson M. D., Fox M. D., Kelly E. L. A., Zgliczynski B. J., Sandin S. A., Smith J. E. (2020). Ecophysiology of coral reef primary producers across an upwelling gradient in the tropical central pacific. PLoS One 15, e0228448. doi: 10.1371/journal.pone.0228448
Johnson M. D., Price N. N., Smith J. E. (2022). Calcification accretion units (CAUs): A standardized approach for quantifying recruitment and calcium carbonate accretion in marine habitats. Methods Ecol. Evol. 13, 1436–1446. doi: 10.1111/2041-210X.13867
Kench P. S., Beetham E. P., Turner T., Morgan K. M., Owen S. D., McLean R. F. (2022). Sustained coral reef growth in the critical wave dissipation zone of a maldivian atoll. Commun. Earth Environ. 3, 1–12. doi: 10.1038/s43247-021-00338-w
Klein S. G., Geraldi N. R., Anton A., Schmidt-Roach S., Ziegler M., Cziesielski M. J., et al. (2022). Projecting coral responses to intensifying marine heatwaves under ocean acidification. Glob. Change Biol. 28, 1753–1765. doi: 10.1111/gcb.15818
Kohler K. E., Gill S. M. (2006). Coral point count with excel extensions (CPCe): A visual basic program for the determination of coral and substrate coverage using random point count methodology. Comput. Geosci 32, 1259–1269. doi: 10.1016/j.cageo.2005.11.009
Kramer K. L., Cotton S. P., Lamson M. R., Walsh W. J. (2016). Bleaching and catastrophic mortality of reef-building corals along west hawai’i island: findings and future directions. In Proceedings of the 13th International Reef Symposium. Honolulu. 219–232.
Kroeker K. J., Kordas R. L., Crim R., Hendriks I. E., Ramajo L., Singh G. S., et al. (2013). Impacts of ocean acidification on marine organisms: quantifying sensitivities and interaction with warming. Glob Change Biol. 19, 1884–1896. doi: 10.1111/gcb.12179
Kroeker K. J., Kordas R. L., Crim R. N., Singh G. G. (2010). Meta-analysis reveals negative yet variable effects of ocean acidification on marine organisms. Ecol. Lett. 13, 1419–1434. doi: 10.1111/j.1461-0248.2010.01518.x
Kuchinke M., Tilbrook B., Lenton A. (2014). Seasonal variability of aragonite saturation state in the Western pacific. Mar. Chem. 161, 1–13. doi: 10.1016/j.marchem.2014.01.001
Kuffner I. B., Andersson A. J., Jokiel P. L., Rodgers K. S., Mackenzie F. T. (2008). Decreased abundance of crustose coralline algae due to ocean acidification. Nat. Geosci 1, 114–117. doi: 10.1038/ngeo100
Kwiatkowski L., Torres O., Bopp L., Aumont O., Chamberlain M., Christian J. R., et al. (2020). Twenty-first century ocean warming, acidification, deoxygenation, and upper-ocean nutrient and primary production decline from CMIP6 model projections. Biogeosciences 17, 3439–3470. doi: 10.5194/bg-17-3439-2020
Lange I. D., Perry C. T., Alvarez-Filip L. (2020). Carbonate budgets as indicators of functional reef “health”: A critical review of data underpinning census-based methods and current knowledge gaps. Ecol. Indic 110, 105857. doi: 10.1016/j.ecolind.2019.105857
Lauvset S. K., Key R. M., Olsen A., van Heuven S., Velo A., Lin X., et al. (2016). A new global interior ocean mapped climatology: the 1° × 1° GLODAP version 2. Earth Syst. Sci. Data 8, 325–340. doi: 10.5194/essd-8-325-2016
Lozada-Misa P., Schumacher B., Vargas-Angel B. (2017). Analysis of benthic survey images via CoralNet: A summary of standard operating procedures and guidelines. Honolulu: U.S. Department of Commerce, National Oceanic and Atmospheric Administration. doi: 10.7289/V5/AR-PIFSC-H-17-02
Lueker T., Dickson A., Keeling C. (2000). Ocean pCO2 calculated from dissolved inorganic carbon, alkalinity, and equations for K1 and K2: Validation based on laboratory measurements of CO2 in gas and seawater at equilibrium. Mar. Chem. 70, 105–119. doi: 10.1016/S0304-4203(00)00022-0
Manning J. C., Carpenter R. C., Miranda E. A. (2019). Ocean acidification reduces net calcification and wound healing in the tropical crustose coralline alga, porolithon onkodes (Corallinales, rhodophyta). J. Exp. Mar. Biol. Ecol. 520, 151225. doi: 10.1016/j.jembe.2019.151225
Manzello D. P., Kleypas J. A., Budd D. A., Eakin C. M., Glynn P. W., Langdon C. (2008). Poorly cemented coral reefs of the eastern tropical pacific: Possible insights into reef development in a high-CO2 world. Proc. Natl. Acad. Sci. 105, 10450–10455. doi: 10.1073/pnas.0712167105
NOAA Coral Program (2014). National Coral Reef Monitoring Plan (Silver Spring, MD, NOAA Coral Reef Conservation Program).
NOAA Coral Program (2021). National Coral Reef Monitoring Plan. Silver Spring, MD, NOAA Coral Reef Conservation Program. doi: 10.25923/fqkq-w497
Oksanen J., Guillaume Blanchet F., Friendly M., Kindt R., Legendre P., McGlinn D., et al. (2020). Vegan: Community Ecology Package. R Package Version 2.5-7. Available at: https://cran.r-project.org/web/packages/vegan/index.html.
Ordoñez A., Doropoulos C., Diaz-Pulido G. (2014). Effects of ocean acidification on population dynamics and community structure of crustose coralline algae. Biol. Bull. 226, 255–268. doi: 10.1086/BBLv226n3p255
Page H. N., Courtney T. A., Collins A., De Carlo E. H., Andersson A. J. (2017). Net community metabolism and seawater carbonate chemistry scale non-intuitively with coral cover. Front. Mar. Sci. 4, 161. doi: 10.3389/fmars.2017.00161
Price N. (2010). Habitat selection, facilitation, and biotic settlement cues affect distribution and performance of coral recruits in French Polynesia. Oecologia 163, 747–758. doi: 10.1007/s00442-010-1578-4
Price N. N., Martz T. R., Brainard R. E., Smith J. E. (2012). Diel variability in seawater pH relates to calcification and benthic community structure on coral reefs. PLoS One 7, e43843. doi: 10.1371/journal.pone.0043843
Raymundo L. J., Burdick D., Hoot W. C., Miller R. M., Brown V., Reynolds T., et al. (2019). Successive bleaching events cause mass coral mortality in Guam, Micronesia. Coral Reefs 38, 677–700. doi: 10.1007/s00338-019-01836-2
Schönberg C. H. L., Fang J. K. H., Carreiro-Silva M., Tribollet A., Wisshak M. (2017). Bioerosion: the other ocean acidification problem. ICES J. Mar. Sci. 74, 895–925. doi: 10.1093/icesjms/fsw254
Schuetzenmeister A., Dufey F. (2020). VCA: Variance Component Analysis. R Package Version 1.4.3. Available at: https://cran.r-project.org/web/packages/VCA/index.html.
Shamberger K. E. F., Cohen A. L., Golbuu Y., McCorkle D. C., Lentz S. J., Barkley H. C. (2014). Diverse coral communities in naturally acidified waters of a Western pacific reef. Geophys Res. Lett. 41, 499–504. doi: 10.1002/2013GL058489
Silbiger N. J., Donahue M. J., Brainard R. E. (2017). Environmental drivers of coral reef carbonate production and bioerosion: A multi-scale analysis. Ecology 98, 2547–2560. doi: 10.1002/ecy.1946
Silverman J., Lazar B., Erez J. (2007). Effect of aragonite saturation, temperature, and nutrients on the community calcification rate of a coral reef. J. Geophys Res. Oceans 112, C05004. doi: 10.1029/2006JC003770
Smith J. E., Brainard R., Carter A., Grillo S., Edwards C., Harris J., et al. (2016). Re-evaluating the health of coral reef communities: baselines and evidence for human impacts across the central pacific. Proc. R Soc. B Biol. Sci. 283, 20151985. doi: 10.1098/rspb.2015.1985
Smith J. N., Mongin M., Thompson A., Jonker M. J., De’ath G., Fabricius K. E. (2020). Shifts in coralline algae, macroalgae, and coral juveniles in the great barrier reef associated with present-day ocean acidification. Glob Change Biol. 26, 2149–2160. doi: 10.1111/gcb.14985
Strahl J., Francis D. S., Doyle J., Humphrey C., Fabricius K. E. (2016). Biochemical responses to ocean acidification contrast between tropical corals with high and low abundances at volcanic carbon dioxide seeps. ICES J. Mar. Sci. 73, 897–909. doi: 10.1093/icesjms/fsv194
Sutton A. J., Feely R. A., Maenner-Jones S., Musielwicz S., Osborne J., Dietrich C., et al. (2019). Autonomous seawater pCO2 and pH time series from 40 surface buoys and the emergence of anthropogenic trends. Earth Syst. Sci. Data 11, 421–439. doi: 10.5194/essd-11-421-2019
Sutton A. J., Feely R. A., Sabine C. L., McPhaden M. J., Takahashi T., Chavez F. P., et al. (2014). Natural variability and anthropogenic change in equatorial pacific surface ocean pCO2 and pH. Glob Biogeochem Cycles 28, 131–145. doi: 10.1002/2013GB004679
Terlouw G. J., Knor L. A. C. M., De Carlo E. H., Drupp P. S., Mackenzie F. T., Li Y. H., et al. (2019). Hawaii Coastal seawater CO2 network: A statistical evaluation of a decade of observations on tropical coral reefs. Front. Mar. Sci. 6, 226. doi: 10.3389/fmars.2019.00226
Vargas-Ángel B., Huntington B., Brainard R. E., Venegas R., Oliver T., Barkley H., et al. (2019). El Niño-associated catastrophic coral mortality at Jarvis island, central equatorial pacific. Coral Reefs 38, 731–741. doi: 10.1007/s00338-019-01838-0
Vargas-Ángel B., Looney E. E., Vetter O. J., Coccagna E. F. (2011). Severe, widespread El niño–associated coral bleaching in the US phoenix islands. Bull. Mar. Sci. 87, 623–638. doi: 10.5343/bms.2010.1095
Vargas-Ángel B., Richards C. L., Vroom P. S., Price N. N., Schils T., Young C. W., et al. (2015). Baseline assessment of net calcium carbonate accretion rates on U.S. Pacific Reefs. PLoS One 10, e0142196. doi: 10.1371/journal.pone.0142196
Vroom P. S., Braun C. L. (2010). Benthic composition of a healthy subtropical reef: Baseline species-level cover, with an emphasis on algae, in the northwestern Hawaiian islands. PLoS One 5, e9733. doi: 10.1371/journal.pone.0009733
Williams I. D., Baum J. K., Heenan A., Hanson K. M., Nadon M. O., Brainard R. E. (2015). Human, oceanographic and habitat drivers of central and Western pacific coral reef fish assemblages. PLoS One 10, e0120516. doi: 10.1371/journal.pone.0120516
Winston M., Couch C., Huntington B., Vargas-Ángel B. (2020). Ecosystem Sciences Division Standard Operating Procedures: Data Collection for Rapid Ecological Assessment Benthic Surveys, 2019 Update. Honolulu: U.S. Department of Commerce, National Oceanic and Atmospheric Administration. doi: 10.25923/ws5s-km69
Winston M., Oliver T., Couch C., Donovan M. K., Asner G. P., Conklin E., et al. (2022). Coral taxonomy and local stressors drive bleaching prevalence across the Hawaiian archipelago in 2019. PLoS One 17, e0269068. doi: 10.1371/journal.pone.0269068
Keywords: coral reefs, carbonate chemistry, ocean acidification, carbonate accretion, U.S. Pacific, climate change, reef monitoring
Citation: Barkley HC, Oliver TA, Halperin AA, Pomeroy NV, Smith JN, Weible RM, Young CW, Couch CS, Brainard RE and Samson JC (2022) Coral reef carbonate accretion rates track stable gradients in seawater carbonate chemistry across the U.S. Pacific Islands. Front. Mar. Sci. 9:991685. doi: 10.3389/fmars.2022.991685
Received: 11 July 2022; Accepted: 03 November 2022;
Published: 24 November 2022.
Edited by:
Maria Cristina Gambi, Istituto Nazionale di Oceanografia e di Geofisica Sperimentale, ItalyReviewed by:
Guillermo Diaz-Pulido, Griffith University, AustraliaWei-Jen Huang, National Sun Yat-sen University, Taiwan
Copyright © 2022 Barkley, Oliver, Halperin, Pomeroy, Smith, Weible, Young, Couch, Brainard and Samson. This is an open-access article distributed under the terms of the Creative Commons Attribution License (CC BY). The use, distribution or reproduction in other forums is permitted, provided the original author(s) and the copyright owner(s) are credited and that the original publication in this journal is cited, in accordance with accepted academic practice. No use, distribution or reproduction is permitted which does not comply with these terms.
*Correspondence: Hannah C. Barkley, aGFubmFoLmJhcmtsZXlAbm9hYS5nb3Y=