- 1Department of Marine Biotechnology, National Kaohsiung University of Science and Technology, Kaohsiung, Taiwan
- 2The Integrative Evolutionary Galliforms Genomics Research (iEGG) and Animal Biotechnology Center, National Chung Hsing University, Taichung, Taiwan
- 3Department of Life Science, National Taiwan University, Taipei, Taiwan
- 4Planning and Information Division, Fisheries Research Institute, Keelung City, Taiwan
- 5Institute of Cellular and Organismic Biology, Academia Sinica, Taipei, Taiwan
Maintenance of an acid-base balance is essential for normal physiological processes in vertebrates. Freshwater fishes live in an aquatic environment with variable pH, and their buffering capacity for acid-base balance in body fluids is weak. Thus, after acid exposure, fishes secrete excess acid to prevent internal acidosis. Acid-secreting ionocytes present in the adult gills and embryonic skin are primarily responsible for acid secretion, and H+-ATPase and Na+/H+ exchanger 3 (NHE3) are the two main transporters responsible for apical acid secretion. Vitamin D is a well-known hormone involved in the maintenance of Ca2+ homeostasis and is suggested to be involved in acid-base regulation by modulating the activity and/or mRNA expression of NHE3 in mammalian models. It remains unclear whether vitamin D is involved in acid secretion in fishes. The aim of the present study was to use zebrafish as a model to determine whether vitamin D and its receptors influence acid secretion. Our results indicated that the levels of 1α, 25-dihydroxyvitamin D3 (1α,25(OH)2D3), the bioactive vitamin D, were significantly increased in 3 days post-fertilization zebrafish larvae after exposure to acidic freshwater (AFW, pH 4.0). Exogenous 1α,25(OH)2D3 (20 μg/L) incubation substantially enhanced the mRNA expression of acid-secreting transporters and acid secretion at the skin of the entire body and each H+-ATPase-rich cell (HRC), a type of acid-secreting ionocyte. Furthermore, the expression of vitamin D receptors (VDRs) was identified in HRCs of zebrafish. When both VDRa and VDRb were knocked down, acid secretion and the mRNA expression of acid-secreting transporters were significantly decreased. Moreover, double knockdown of VDRa/b prevented the increase in acid secretion induced by AFW and 1α,25(OH)2D3 treatment. This study is the first to indicate that vitamin D is involved in acid secretion in fish.
Introduction
Fish living in freshwater environments frequently experience aquatic acidification, which is caused by natural and anthropogenic ambient factors. Maintenance of an internal pH homeostasis is vital for normal physiological processes (Karet, 2002). The acid-base buffering capacity of fish body fluids is low; therefore, fish easily have internal acidosis under ambient acidity and need to excrete excess acid (Evans et al., 2005; Perry and Gilmour, 2006; Hwang et al., 2011). While the gills are responsible for approximately 90% of the acid-base movement in adult fish, this function is mainly performed by the yolk skin during early embryonic stages (Evans et al., 2005; Hwang and Perry, 2010). Acid secretion at the cellular level in fish gills/skin is primarily accomplished by the excretion of protons across the apical membrane of ionocytes (Evans et al., 2005; Hwang et al., 2011). A specific subtype of ionocytes for acid secretion has been identified in the gills and embryonic skin of teleosts (Hwang et al., 2011). In acid-secreting ionocytes, apical H+-ATPase (HA) and Na+/H+ exchanger 3 (NHE3) are the two pathways for transepithelial proton secretion (Hwang et al., 2011; Guh et al., 2015). In addition, basolateral anion exchanger 1 (AE1) transports from ionocytes to body fluid that is also involved in proton secretion in teleosts (Lee et al., 2011; Hsu et al., 2014).
Previous studies have combined electrophysiological, gene knockdown, and pharmacological experiments to determine HA and NHE3b mediated proton secretion at the apical membrane of H+-ATPase-rich cells (HRCs), the acid-secreting ionocytes, in zebrafish (Shih et al., 2008; Shih et al., 2012). In zebrafish, HA and NHE3b in HRCs are responsible for approximately 70% and 30% of the apical proton secretion, respectively (Shih et al., 2008; Shih et al., 2012). However, NHE3 is the major route for apical proton secretion in acid-secreting ionocytes of medaka (Lin et al., 2012; Lin et al., 2021). The function of other acid secretion-related transporters in ionocytes is homologous in fishes (Hsu et al., 2014; Liu et al., 2016; Yan and Hwang, 2019). Hormonal control plays an essential role in the maintenance of the acid-base balance in fishes (Guh et al., 2015; Yan and Hwang, 2019). Some hormones such as cortisol and endothelin-1 are induced after acid exposure in fishes (Guh et al., 2014; Lin et al., 2015; Lin et al., 2021). Overexpression and pharmacological approaches suggest that increased hormone levels can regulate the capacity for acid secretion by stimulating the mRNA expression and activity of acid-secreting transporters and/or ionocyte differentiation (Cruz et al., 2013; Guh et al., 2014; Lin et al., 2015; Lin et al., 2021).
Acid exposure simultaneously causes a decrease in Ca2+ absorption and increase in Ca2+ loss rate in zebrafish (Kwong and Perry, 2014). Moreover, acid exposure increases the gene expression and secretion of cortisol, a hypercalcemic hormone, but decreases the gene expression of stanniocalcin-1, a hypocalcemic hormone, in zebrafish (Kumai et al., 2012; Lin et al., 2015; Chou et al., 2015). Decreased whole-body Ca2+ levels in zebrafish after acid exposure may be compensated by an increase in the mRNA expression level of epithelial Ca2+ channels and the density of ecac-expressing ionocytes (Kwong and Perry, 2014). Thus, the different changes in cortisol and stanniocalcin-1 levels after acid exposure may reflect a compensatory mechanism for Ca2+ homeostasis. However, incubation and overexpression experiments have indicated that cortisol and stanniocalcin-1 are not only involved in the regulation of ecac transcripts but also ha and nhe3b transcripts in zebrafish (Lin et al., 2015; Chou et al., 2015). Furthermore, cortisol can affect the acid secretion capacity at each HRC in zebrafish (Lin et al., 2015). In contrast, knockdown of hypercalcemic parathyroid hormone 1 (PTH1) decreases HRC differentiation in zebrafish (Kwong and Perry, 2015). Calciotropic hormones may have multiple effects on ion regulation in fishes.
Vitamin D is a well-known hormone for Ca2+ homeostasis in mammals and has also been reported to elevate the body-fluid Ca2+ levels in carp, cod, and zebrafish (Swarup et al., 1991; Sundell et al., 1993; Lin et al., 2012). The vitamin D precursor is initially synthesized in the skin. Through a series of reactions, it is converted to 1α, 25-dihydroxyvitamin D3 (1α,25(OH)2D3), the active form of vitamin D, by renal 1α-OHase (CYP27B1) (Holick, 2007). Previous studies have indicated that renal tissues and the liver are vital for 1α,25(OH)2D3 production (Hayes et al., 1986; du Bois et al., 1988; Takeuchi et al., 1991), and cyp27b1 has also been identified in teleosts (Cheng et al., 2006; Lin et al., 2012). Vitamin D regulates target gene expression by binding to vitamin D receptors (VDRs) (Holick, 2007). There are two forms of VDR in teleosts (Taylor et al., 2001; Howarth et al., 2008). VDRa and VDRb are two paralogous VDRs in zebrafish. For Ca2+ uptake, vitamin D bound to VDRa has been proposed to regulate the gene expression of epithelial Ca2+ channels in zebrafish (Lin et al., 2012). However, in mammalian cell lines, teleost VDRs have been found to induce transcription of the expression construct with 1α,25(OH)2D3 (Reschly et al., 2007; Howarth et al., 2008; Krasowski et al., 2011). Although the two isoforms of medaka VDRs show different responses to 1α,25(OH)2D3, they still induce the transcripts of VDR responsive element-containing expression constructs in cell lines (Howarth et al., 2008). In mammals, metabolic acids are mainly excreted by the proximal tubules of the kidneys. NHE3 plays an essential role in apical proton secretion in the proximal tubules, and its activity provides the necessary driving force for bicarbonate reabsorption (Wagner et al., 2004; Bobulescu and Moe, 2006). Mice with targeted NHE3 disruption exhibit metabolic acidosis and decreased renal absorption of Na+ and Ca2+ (Bobulescu and Moe, 2006; Pan et al., 2012). In addition, increased serum 1α,25 (OH)2D3 levels have been found in NHE3 knockout mice (Pan et al., 2012). Treatment with 1α,25 (OH)2D3 stimulates apically located sodium-hydrogen exchange (NHE) activity in renal epithelial cell lines of mammals (Binswanger et al., 1993). Vitamin D has been suggested to be involved in the regulation of the acid-base homeostasis by enhancing urinary acid excretion in mammals (Stim et al., 1994). In contrast, 1α,25(OH)2D3 treatment inhibits the NHE activity in a Caco-2 colonic carcinoma cell line (Wali et al., 1992) and the rat ileum by downregulating the mRNA levels of NHE3 (Gill et al., 2002). The divergent responses of NHE to 1α,25(OH)2D3 in tissues and cell lines suggest the involvement of different cell-specific mechanism(s) in the regulation of NHE (Gill et al., 2002). To date, the major understanding of vitamin D in the ion regulation of fishes has focused on Ca2+ absorption (Lin and Hwang, 2016). Our understanding of whether acid exposure affects vitamin D synthesis in fishes is limited. Moreover, the effects of vitamin D on acid-secreting transporters and acid secretion in fishes remain unknown.
The zebrafish is an emerging model frequently used to explore the endocrine control on ion regulation in fishes because of its advantages in molecular, cellular, bioinformatic, and in vivo electrophysiological approaches (Guh et al., 2015; Yan and Hwang, 2019). In addition, acid-secreting transporters have been well identified in HRCs, and zebrafish can survive in acidic freshwater (AFW) with a pH as low as 4 (Guh et al., 2015). Thus, the present study used zebrafish as a model to investigate the following questions: (1) Does AFW (pH 4.0) change the mRNA expression of cyp27b1 that encodes an enzyme for 1α,25(OH)2D3 synthesis and 1α,25(OH)2D3 levels in zebrafish larvae? (2) Is vitamin D involved in regulating the mRNA expression of HA, NHE3b, and AE1b, a set of acid secretion-related transporters are expressed in HRC, and acid secretion in zebrafish larvae? (3) Does VDRa and/or VDRb mediate the effects of vitamin D on the regulation of acid secretion? Elucidating these questions would enhance our knowledge regarding the role of vitamin D in the acid-base regulation in fishes.
Materials and methods
Experimental animals
Zebrafish (Danio rerio) were kept in local tap water at 28.5°C under a 14:10 h light:dark photoperiod at the Department of Marine Biotechnology, National Kaohsiung University of Science and Technology, Kaohsiung, Taiwan. The experimental protocols were approved by the Institutional Animal Care and Utilization Committee of the university (approval no. 0109-AAAP-006).
Acclimation experiments
Freshwater (FW, pH 7.0) and acidic freshwater (AFW, pH 4.0) prepared from local tap water were used to determine the effects of an acidic medium. In accordance with previous studies (Lin et al., 2015; Lin et al., 2021), the acidic medium was prepared by adding H2SO4 to the FW; the concentrations of other ions in the AFW were same as those in the FW (see Supplemental Table S1). Fertilized zebrafish eggs were transferred to either FW or AFW and then incubated until sampling at 3 days post-fertilization (dpf). The media were changed twice daily. The pH values of all experimental media were verified using a pH meter (FP20; OHAUS, Parsippany, NJ, USA), and the ion concentrations were determined using an atomic absorption spectrometer (U-2000; Hitachi, Tokyo, Japan).
Vitamin D measurement
Following the method previously described by Lin et al., 2012, the amount of whole-body 1α,25(OH)2D3, the bioactive form of vitamin D, in zebrafish larvae was measured using an ELISA kit (E0467GE; EIAlab, Wuhan, China). Three dpf zebrafish larvae with FW and AFW treatments were anesthetized with 0.03% MS-222 and then washed several times with 1X phosphate-buffered saline (PBS). Twenty larvae were pooled in one vial as a single sample. The zebrafish larvae in vials were homogenized in 500 μL of 1X PBS. Next, diethyl ether (Merck, Darmstadt, Germany) was added to the homogenates and the mixtures were vortexed for 1 min. Then, the mixtures were centrifuged at 2000 × g at 4°C for 10 min. The diethyl ether phase was collected and dried under a nitrogen flux at room temperature. Finally, the dried samples were resuspended in the standard/sample solution of the ELISA kit, and 1α,25(OH)2D3 was quantified according to the manufacturer’s instructions.
Vitamin D incubation experiment
Following the method previously described by Lin et al., 2012, 0 (control) and 20 μg/L of 1α,25(OH)2D3 (CAS No. 32222-06-3, Sigma, USA) were used for an incubation experiment. Zebrafish eggs were incubated in 1α,25(OH)2D3 media immediately after fertilization and were sampled at 3 dpf for subsequent analysis. The incubation media were changed with new 1α,25(OH)2D3 solutions daily to maintain constant levels of 1α,25(OH)2D3. During incubation, no significant mortality or abnormal behavior was observed.
RNA extraction
After anesthetization with 0.03% MS-222, 20 zebrafish larvae were collected and homogenized in 1 mL TRIzol reagent (Invitrogen, Carlsbad, CA, USA) and then mixed with 0.2 mL chloroform and thoroughly shaken. After centrifugation at 4°C and 12,000 × g for 30 min, the supernatants were collected. The samples were then mixed with an equal volume of isopropanol. Pellets were precipitated by centrifugation at 4°C and 12,000 × g for 30 min, washed with 70% alcohol, and stored at -20°C until use.
Reverse-transcription polymerase chain reaction
Complementary DNA (cDNA) was prepared according to the manufacturer’s protocol of SuperScript Reverse Transcriptase (Invitrogen). Briefly, 5 μg of total RNA was reverse-transcribed in a final volume of 20 μL containing 0.5 mM dNTPs, 2.5 μM oligo(dT)20, 250 ng random primers, 5 mM dithiothreitol, 40 units RNase inhibitor, and 200 units reverse transcriptase (SuperScript Reverse Transcriptase, Invitrogen) for 1 h at 50°C, followed by a 70°C incubation for 15 min.
Quantitative real-time PCR
qPCR was performed using a LightCycler real-time PCR system (Roche, Penzberg, Germany) in a 10 μL mixture containing 5 μL 2X SYBR Green I Master (Roche), 300 nM of the primer pairs, and 20–30 ng cDNA. The standard curve for each gene was checked in a linear range with β-actin as an internal control. The present study followed previous studies (Chang et al., 2009; Lin et al., 2012; Lin et al., 2015) for the qPCR primer design (Supplemental Table S2). To show each gene expression at the same scale, the expression value was multiplied by different fold, 1000x for cyp27b1, 100x for ha, 5000x for nhe3b, 2000x for ae1b and 1000x for gcm2, finally.
In situ hybridization
Zebrafish vdra or vdrb fragments were obtained by PCR using a PCR primer design. The PCR fragment was inserted into pGEM-T Easy Vector (Promega, Madison, WI, USA). The inserted fragments were amplified with T7 and SP6 primers by PCR, and the products were used as templates for in vitro transcription with T7 and SP6 RNA polymerase (Roche) in the presence of digoxigenin-11-uridine triphosphate (Roche) to synthesize the probes. Three dpf zebrafish larvae were anesthetized on ice and fixed with 4% paraformaldehyde in a PBS (1.4 mM NaCl, 0.2 mM KCl, 0.1 mM Na2HPO4, and 0.002 mM KH2PO4; pH 7.4) solution at 4 °C overnight. Subsequently, we performed in situ hybridization as previously described (Lin et al., 2012).
Immunocytochemistry
After in situ hybridization, the samples were immediately subjected to immunocytochemistry. The zebrafish larvae were washed with PBS and then incubated with 3% bovine serum albumin for 2 h to block non-specific binding. The samples were then incubated overnight at 4°C with a polyclonal antibody against the α subunit of zebrafish H+-ATPase (dilution at 1:100; synthetic peptide: AEMPADSGYPAYLGARLA). After washing with PBS for 30 min, the samples were further incubated with Alexa Fluor 488 goat anti-rabbit IgG antibodies (Molecular Probes; diluted at 1:200 with PBS) for 2 h at room temperature. Images were acquired using an Axioplan 2 microscope (Zeiss, Oberkochen, Germany).
Morpholino oligonucleotide knockdown
As previously described (Lin et al., 2012), zebrafish vdra morpholino oligonucleotide (MO) (5’- AACGGCACTATTTTCCGTAAGCATC-3’), vdrb MO (5’- AACGTTCCGGTCGAACTCATCTGGC-3’), and a standard control MO (5’-CCTCTTACCTCAGTTACAATTTATA-3’) were used for the MO knockdown experiment. A 4 ng/embryo (MO) dosage was used for the injection. The MOs (4 ng/embryo) were injected into the embryos at the 1–2 cell stage using an IM-300 microinjector system (Narishige Scientific Instrument Laboratory, Tokyo, Japan). At this dose, the MO knockdown did not cause embryos either significant abnormal development or mortality. The MO-injected larvae at 3 dpf were sampled for subsequent analyses.
Scanning ion-selective electrode technique, skin surface H+ gradients, and H+ flux in ionocytes of zebrafish larvae
Scanning ion-selective electrode technique (SIET), recording of the H+ gradients at the skin surface, and H+ flux at the surface of ionocytes in zebrafish larvae were performed as previously described (Lin et al., 2006). Briefly, SIET was performed at room temperature (26–28°C) in a small plastic recording chamber filled with 1 mL of a “recording medium” that contained 300 µM 3-(N-morpholino)propanesulfonic acid buffer (Sigma) and 0.1 mg/L ethyl 3-aminobenzoate (Tricaine, pH 7.0) (Sigma), as described previously (Lin et al., 2006). To record the H+ gradients at the skin surface of a larva, a microelectrode was moved to a target position 10–20 µm from the skin. After recording the target point, the microelectrode was moved to record the background. In the present study, Δ[H+] represents the difference between the measured H+ gradients of the skin surface and background.
To record the H+ flux at the surface of the ionocytes, the microelectrode was moved to a position 2 µm above the cell surface. At every position, the voltage difference in microvolts was measured by orthogonally probing the surface at 10-µm intervals. The recording was performed in 10 replicates, and the median of the repeats was used to calculate the ion flux of the cell. To calculate the ionic flux, the voltage differences were first converted into a concentration gradient ΔC (µmol·L-1·cm-3). ΔC was subsequently converted into ionic flux using Fick’s law of diffusion in the following equation: J = D(ΔC)/ΔX, where J (pmol·cm-2·s-1) is the net flux of the ion, D is the diffusion coefficient of the ion (2.09 × 10-5 cm2/s for H+), and ΔX (cm) is the distance between the two points.
Statistical analysis
The values were expressed as mean ± standard error of the mean (SEM). The results were compared using one-way analysis of variance (ANOVA) with Tukey’s pairwise test and Student’s t-test. The significance level was set at p < 0.05.
Results
Effects of AFW on the cyp27b1 transcripts and whole-body 1α,25(OH)2D3 levels in 3 dpf zebrafish larvae
To determine the effects of AFW (pH 4.0) treatment on the mRNA expression of cyp27b1, the key enzyme in the synthesis of 1α,25(OH)2D3, and whole-body 1α,25(OH)2D3 levels in zebrafish larvae, the zebrafish fertilized eggs were exposed to FW (pH 7.0) or AFW for 3 days. Zebrafish larvae exposed to AFW exhibited significantly elevated expression of cyp27b1 and 1α,25(OH)2D3 level compared with zebrafish larvae exposed to FW (Figures 1A, B).
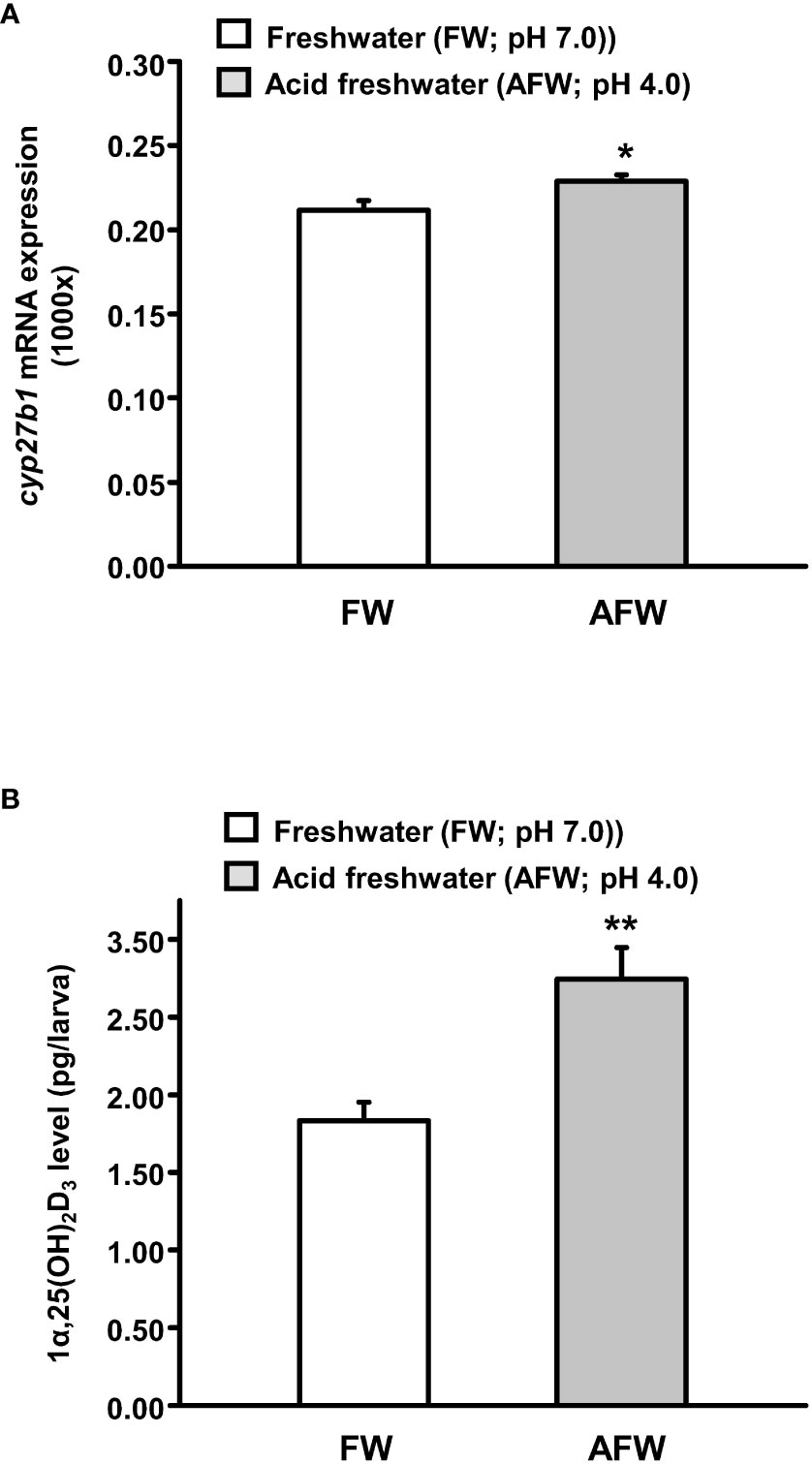
Figure 1 Effects of freshwater (FW, pH 7.0) and acidic freshwater (AFW, pH 4.0) on (A) the mRNA expression of cyp27b1 and (B) whole body 1α, 25-dihydroxyvitamin D3 (1α,25(OH)2D3) level in 3 days post-fertilization (dpf) zebrafish larvae. The mRNA expression was analyzed by qPCR, and the values were normalized to β-actin. Values were expressed as the mean ± SEM (n = 6). Student’s t-test, * p < 0.05; ** p < 0.01.
Effects of exogenous 1α,25(OH)2D3 treatment on the mRNA expression of genes related to acid secretion and proton secretion in 3 dpf zebrafish larvae
The mRNA expression of cyp27b1 and whole-body 1α,25(OH)2D3 levels were significantly upregulated in zebrafish larvae in AFW (Figure 1). Therefore, we further investigated whether exogenous 1α,25(OH)2D3 (20 μg/L) treatment causes changes in the mRNA expression of transporters related to acid secretion and the proton secretion. Compared with the control, exogenous 1α,25(OH)2D3 treatment predominantly increased the mRNA expression of the acid-secreting transporters, HA, NHE3b, and anion exchanger 1b (AE1b), and proton secretion on the skin surface of 3 dpf zebrafish larvae (Figures 2A, B).
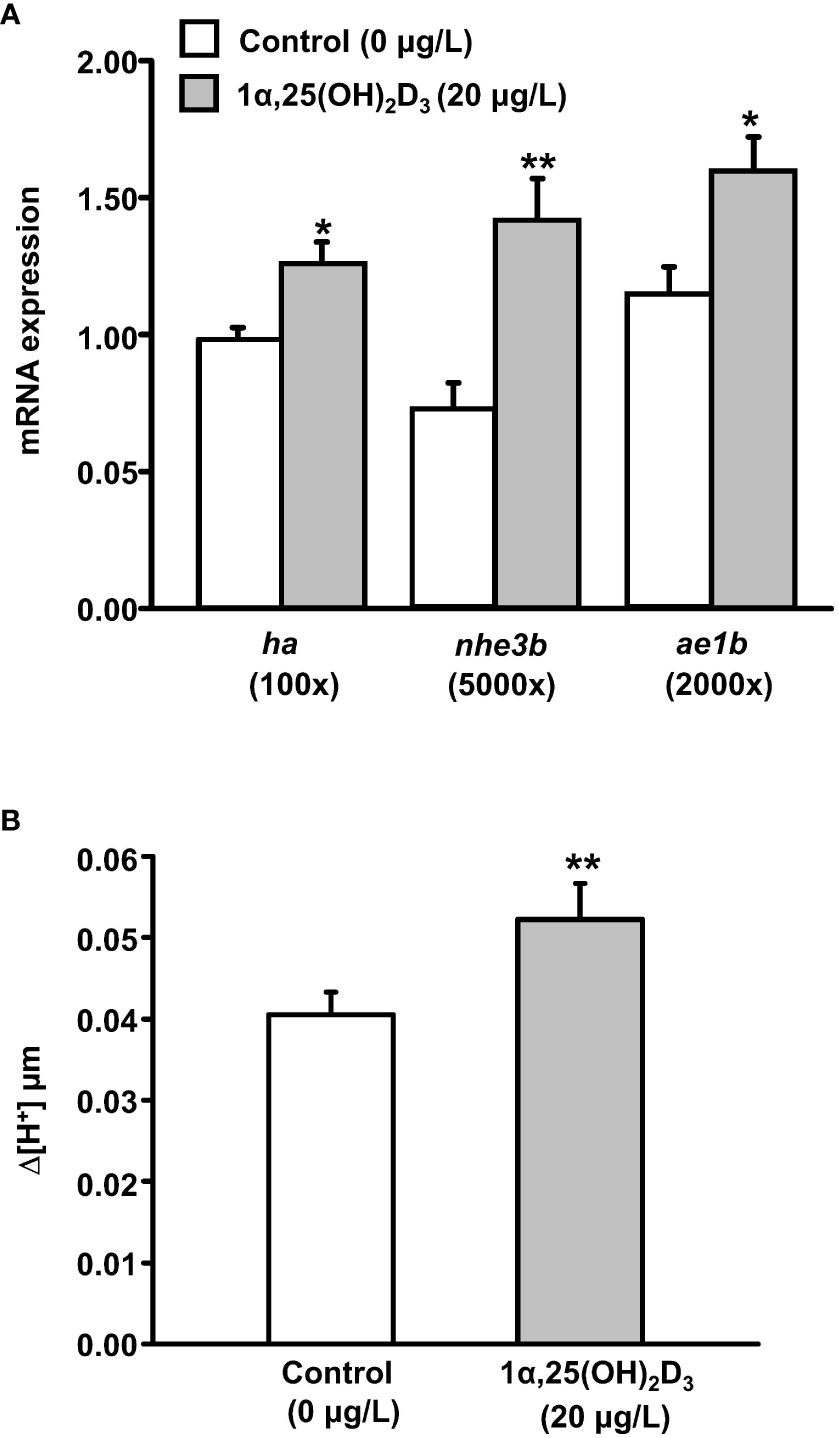
Figure 2 Effects of exogenous 1α,25(OH)2D3 treatment on (A) the mRNA expression of transporter genes and (B) proton secretion in the skin surface of 3 dpf zebrafish larvae. The mRNA expressions were analyzed by qPCR, and the values were normalized to β-actin. Scanning ion-selective electrode technique (SIET) was used to measure the H+ activity on the skin. Values were expressed as the mean ± SEM (n = 6–12). Student’s t-test, * p < 0.05; ** p < 0.01.
Colocalization of VDR mRNA with acid-secreting ionocyte in zebrafish larvae
To further confirm the role of vitamin D in acid secretion, we examined whether the mRNA expression of vdra and/or vdrb is expressed in HRCs, the acid-secreting ionocytes, by double in situ hybridization and immunocytochemistry against VDR mRNA and H+-ATPase, respectively, in 3 dpf zebrafish larvae. As shown in Figures 3A, B, partial vdra signals were localized in the HRCs but all vdrb signals were identified in the HRCs (Figures 3C, D).
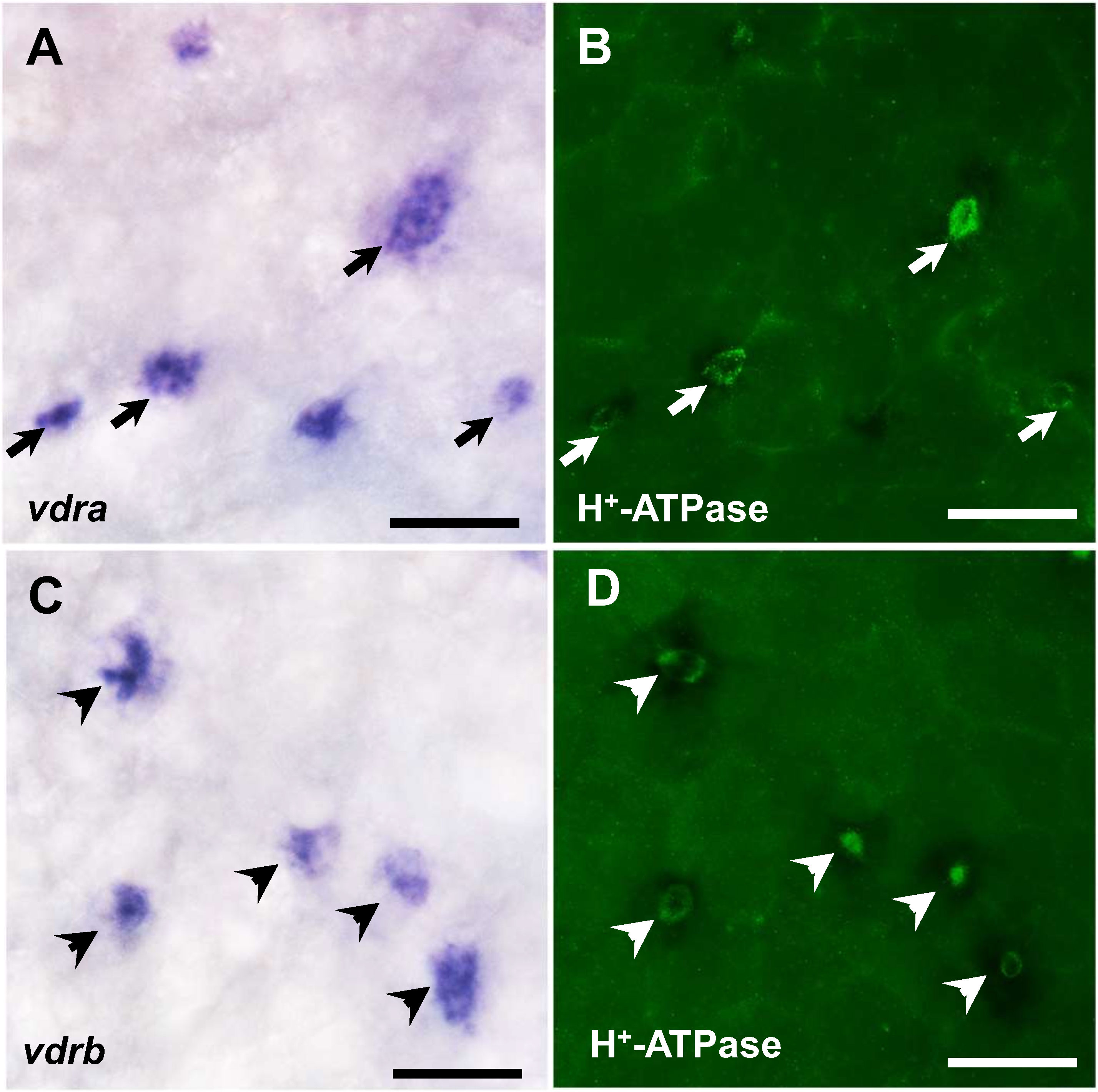
Figure 3 In situ hybridization staining of vdra and vdrb (A, C) and immunocytochemical staining of H+-ATPase (B, D) in 3 dpf zebrafish larvae. Co-localization of vdra mRNA and H+-ATPase protein signals in the same cell is indicated by arrows. Scale bar: 20 μm.
Effects of vdra and/or vdrb knockdown on the mRNA expression of genes related to acid secretion and proton secretion in 3 dpf zebrafish larvae
Exogenous 1α,25(OH)2D3 treatment significantly increased the mRNA expression of transporters related to acid secretion and the proton secretion on the skin surface of zebrafish larvae (Figure 2). Moreover, vdra and vdrb expressions were identified in HRCs (Figure 3). Thus, we further explored the effects of VDRa and/or VDRb on acid secretion in zebrafish larvae. Fertilized zebrafish eggs were injected with vdra or/and vdrb MO, and transporter expression and proton secretion were analyzed in 3 dpf larvae. The mRNA expression of ha, nhe3b, and ae1b and proton secretion from the skin surface did not change in vdra or vdrb morphants (Figure 4A). In contrast, double vdra and vdrb (vdra/b) knockdown caused a significant decrease in mRNA expression of acid-secreting transporters as well as the proton secretion from the skin surface (Figures 4A, B).
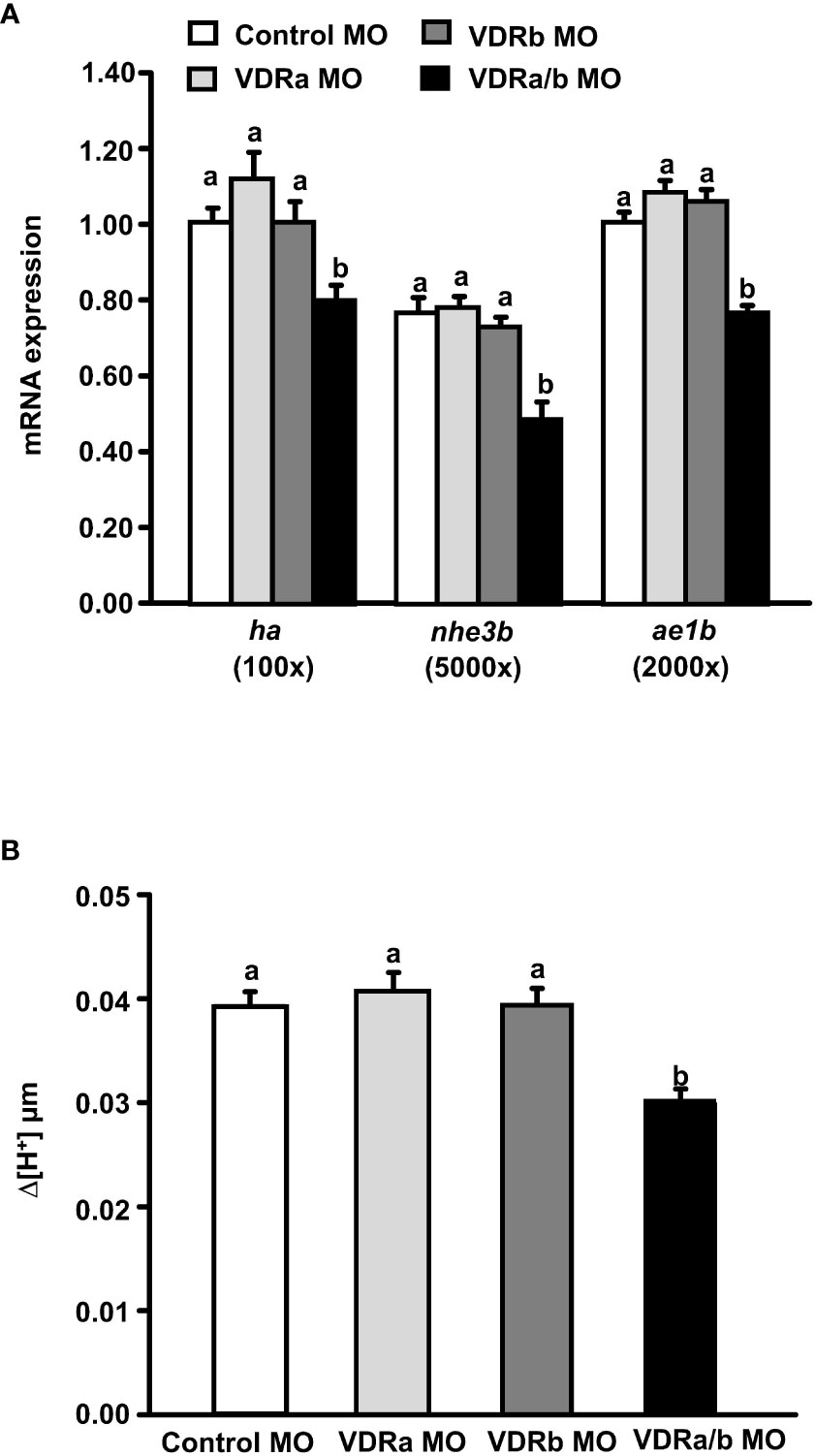
Figure 4 Effects of vitamin D receptor (VDR) morpholino oligonucleotides (MOs) on (A) the mRNA expression of transporters and (B) proton secretion in the skin surface of 3 dpf zebrafish larvae. The mRNA expressions were analyzed by qPCR, and the values were normalized to β-actin. SIET was used to measure the H+ activity on the skin. Different letters indicate a significant difference (p < 0.05), as determined using one-way ANOVA followed by Tukey’s multiple-comparison test. Values were expressed as the mean ± SEM (n = 6–12).
Effect of exogenous 1α,25(OH)2D3 treatment on gcm2 expression in 3 dpf zebrafish larvae with vdra/b knockdown
To determine whether vitamin D can potentially promote the differentiation of acid-secreting ionocytes, we examined the effect of exogenous 1α,25(OH)2D3 (20 μg/L) treatment on the expression of gcm2, a key transcription factor controlling the differentiation of HRCs (Chang et al., 2009). Exogenous 1α,25(OH)2D3 treatment significantly stimulated gcm2 transcripts, while the stimulation was prevented in 3 dpf zebrafish larvae with vdra/b knockdown (Figure 5).
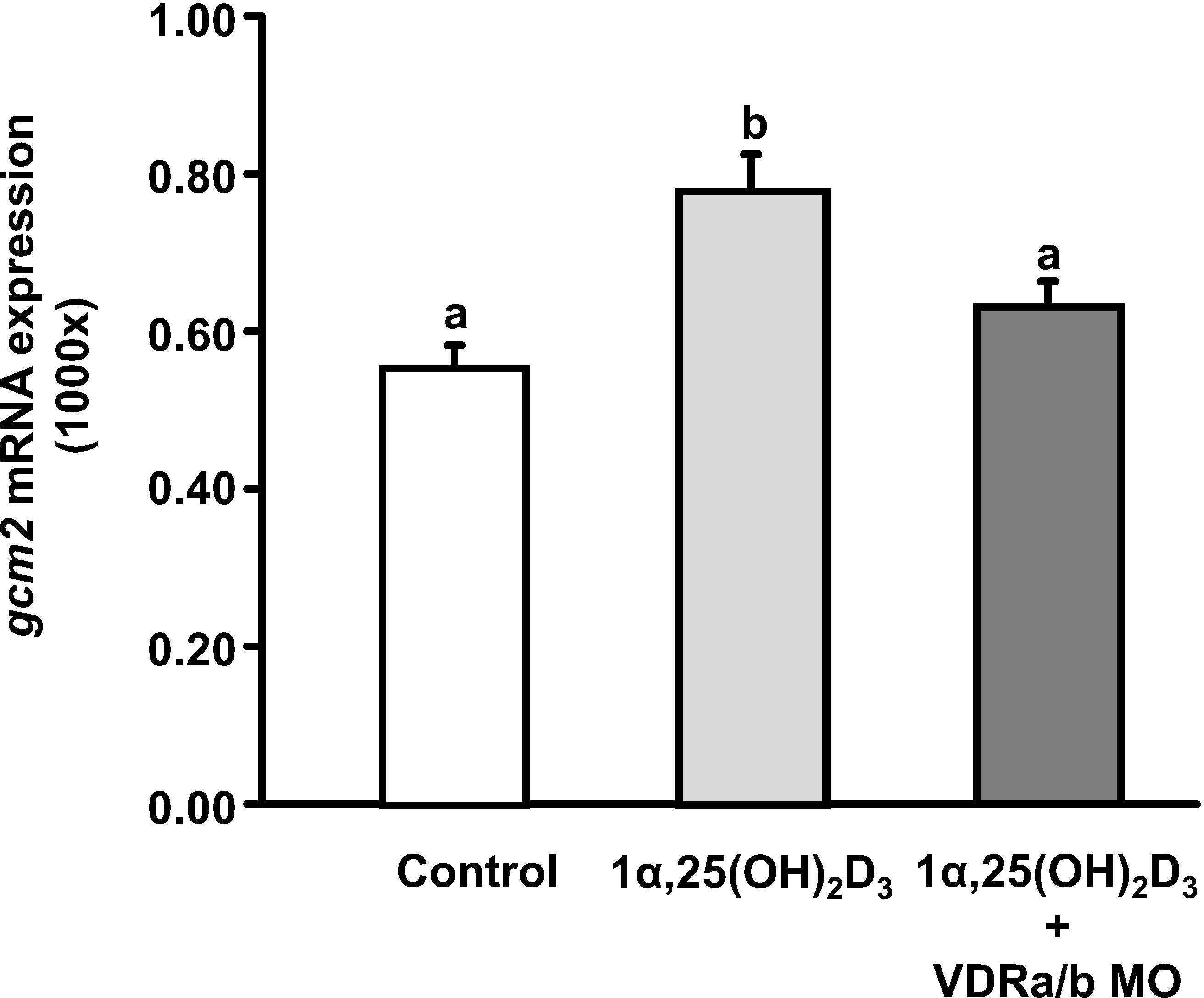
Figure 5 The effect of VDR MOs on gcm2 expression in 3 dpf zebrafish larvae treated with 1α,25(OH)2D3. The mRNA expression was analyzed by qPCR, and the values were normalized to β-actin. Different letters indicate a significant difference (p < 0.05), as determined using one-way ANOVA followed by Tukey’s multiple-comparison test. Values were expressed as the mean ± SEM (n = 6).
Effects of exogenous 1α,25(OH)2D3 or AFW treatment on proton secretion of acid-secreting ionocytes in 3 dpf zebrafish larvae with vdra/b knockdown
Double vdra/b knockdown impaired proton secretion from the skin surface in zebrafish larvae (Figure 4B). To establish whether vdra/b knockdown mediated the effect of vitamin D and AFW on proton secretion of HRCs, vdra/b MO were co-injected into fertilized zebrafish eggs. Knockdown of vdra/b prevented the increase in proton secretion in HRCs induced by exogenous 1α,25(OH)2D3 (20 μg/L) treatment in 3 dpf zebrafish larvae (Figure 6A). In addition, the increased proton secretion in HRCs induced by AFW treatment was also blocked in vdra/b zebrafish morphants (Figure 6B).
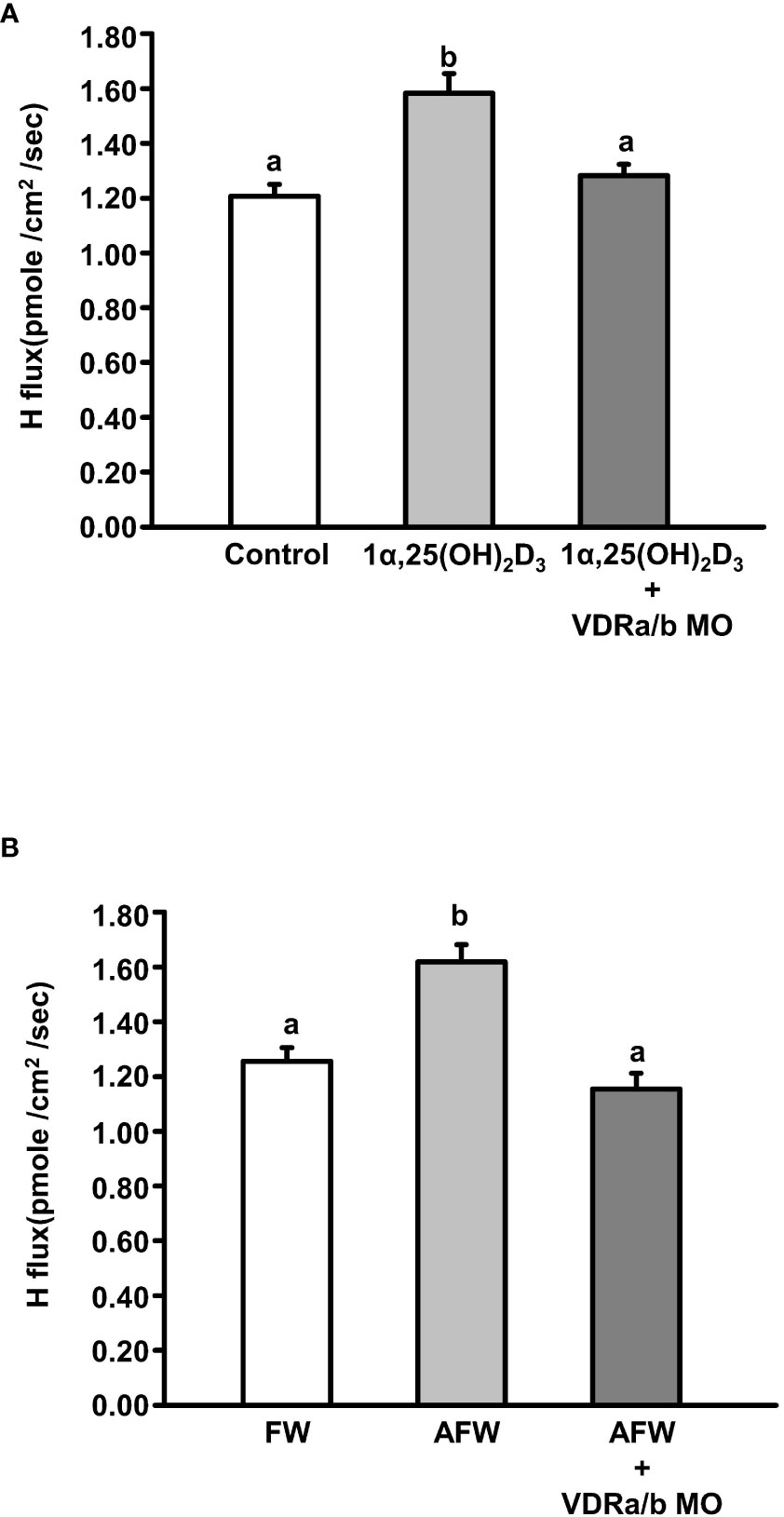
Figure 6 The effect of VDR MOs on proton secretion of acid-secreting ionocytes in 3 dpf zebrafish larvae treated with (A) 1α,25(OH)2D3 and (B) AFW. SIET was used to measure H+ flux on the skin. Different letters indicate a significant difference (p < 0.05), as determined using one-way ANOVA followed by Tukey’s multiple-comparison test. Values were expressed as the mean ± SEM (n = 12).
Discussion
In the present study, the level of 1α,25(OH)2D3, the bioactive vitamin D, was increased in 3 dpf zebrafish larvae after exposure to AFW (pH 4.0). AFW exposure also elevated the mRNA expression of cyp27b1, which encodes the enzyme for 1α,25(OH)2D3 synthesis. These findings suggest that AFW treatment stimulates cyp27b1 transcription, thereby enhancing the synthesis of 1α,25(OH)2D3 in zebrafish. In zebrafish larvae, acid secretion is stimulated after 3 and 4 days of AFW treatment (Horng et al., 2009; Lin et al., 2015). In addition, AFW treatment significantly stimulates HRC differentiation and the mRNA expression of acid-secreting transporters in HRCs of zebrafish larvae (Horng et al., 2009; Chang et al., 2009; Lin et al., 2015). The increased HRC differentiation and mRNA expression of acid-secreting transporters have been suggested to contribute to increased acid secretion in zebrafish (Lin et al., 2015). In the present study, 1α,25(OH)2D3 treatment substantially stimulated the gene expression of HA, NHE3b, and AE1b, the transporters related to acid secretion in HRCs. Furthermore, it significantly enhanced the capacity of acid secretion at the skin surface and each HRC. Therefore, we conclude that increased 1α,25(OH)2D3 level contributes to the enhanced acid secretion ability of zebrafish after exposure to AFW. This is the first study to demonstrate that 1α,25(OH)2D3 treatment increases acid secretion and the mRNA expression of acid-secreting transporters in fish.
1α,25(OH)2D3 treatment elevated the acid secretion capacity at each HRC. HA and NHE3b are the major routes for apical acid secretion and responsible for approximately 70% and 30% apical acid secretion in HRCs of zebrafish, respectively (Shih et al., 2008; Shih et al., 2012). Previous results have revealed that 1α,25(OH)2D3 treatment significantly increased the mRNA expression of both ha and nhe3b, which was approximately 0.25-fold for ha expression and 1-fold for nhe3b expression. 1α,25(OH)2D3 treatment had a greater effect on nhe3b expression. However, Lin et al. (2015) reported that the mRNA expression level of ha was far greater than nhe3b—approximately 50-fold—in 3 dpf zebrafish larvae, and the present study also found a similar result. Thus, the increased ha expression by 1α,25(OH)2D3 treatment predominantly contributed to the increased acid secretion in zebrafish larvae. The mRNA expression of ae1b also increased in zebrafish larvae treated with 1α,25(OH)2D3. ae1b is expressed in the basolateral membrane of acid-secreting ionocytes in zebrafish and medaka (Lee et al., 2011; Hsu et al., 2014). Knockdown of ae1b decrease acid secretion in zebrafish, and ae1b is suggested to extrude cytosolic out of cells to fulfill the function of epithelial acid secretion (Lee et al., 2011). Therefore, 1α,25(OH)2D3 treatment may increase the capacity for acid secretion at each HRC by enhancing the expression of apical and basolateral acid-secreting transporters in zebrafish larvae.
Acid secretion at skin surface of the entire body and at each HRC of zebrafish was elevated by 1α,25(OH)2D3 treatment. Moreover, 1α,25(OH)2D3 treatment induced the mRNA expression of acid-secreting transporters. Therefore, vitamin D may exert a genomic effect on mRNA expression and subsequently increase the capacity for acid secretion at each HRC. This is done via VDR, a ligand-activated transcription factor (Omdahl et al., 2002). Unlike mammals, there are two paralogous VDR forms in fishes because of genome duplication (Taylor et al., 2001; Howarth et al., 2008). By immunohistochemistry with a mammalian VDR antibody, VDR signals were detected in most tissues including the gills in zebrafish (Craig et al., 2008). However, this approach could not identify the respective expressions of paralogous VDRs. To reinforce our hypothesis, we performed double in situ hybridization and immunocytochemistry to determine the mRNA expression of VDRa and/or VDRb in HRCs. The mRNA expression signals of both VDRa and VDRb were identified in HRCs. However, when we knocked down either VDRa or VDRb, the mRNA expression of acid-secreting transporters and acid secretion were not affected. In contrast, double knockdown of VDRa and VDRb significantly decreased the mRNA expression and acid secretion. Knockdown of VDRa induced VDRb expression and vice versa (Supplemental Figure S1). The single knockdown of a VDR may be compensated by the expression of the other VDR form and the actual influence of a knocked-down VDR is neutralized. The transcription activity of VDR responsive element-containing expression constructs with medaka VDRα or VDRβ and steroid receptor coactivator co-expression is significantly induced by 1α,25(OH)2D3 treatment (Howarth et al., 2008). The present study demonstrated that the mRNA expression signals of both VDRa and VDRb were localized in HRCs. However, the knockdown of both VDRa and VDRb prevented 1α,25(OH)2D3- and AFW-induced acid secretion at each HRC. Under in vivo conditions, vitamin D may affect the mRNA expression of acid-secreting transporters and acid secretion via both VDRa and VDRb in zebrafish. However, a previous study has indicated that medaka VDRα and VDRβ respond differently to 1α,25(OH)2D3 treatment (Howarth et al., 2008). Lin et al., 2012 reported that zebrafish VDRa and VDRb show an amino acid sequence similarity of ~97% in the DNA binding domain and ~92% in the ligand binding domain. A slight change in the amino acid composition may result in a difference in transactivation (Lin et al., 2012). Thus, VDRa and VDRb may contribute differently to the regulation of acid secretion.
The present study demonstrated that VDRb knockdown increased VDRa mRNA expression. Lin et al., 2012 reported that VDRa but not VDRb knockdown is involved in the regulation of Ca2+ uptake and mRNA expression of Ca2+ transporters in zebrafish larvae. vdra and vdrb are universally expressed in tissues of zebrafish and the vdra expression is more dominant than vdrb expression in most tissues (Lin et al., 2012). The present data revealed that vdrb expression signals were present in HRCs. vdrb may not be expressed in Na+/K+-ATPase-rich cells, the subtype of ionocytes for Ca2+ absorption in zebrafish. Knockdown of vdrb caused increased vdra expression in most tissues but may not influence the vdra expression in Ca2+ absorption-related cells in zebrafish. Therefore, increased vdra mRNA expression following vdrb knockdown did not affect Ca2+ uptake. However, further evidence is required to support this hypothesis. Our results revealed that VDRa and VDRb are involved in acid regulation. Lin et al., 2012 reported that only VDRa is involved in Ca2+ absorption in zebrafish. VDRa and VDRb may have divergent roles in ion regulation in fishes. This is consistent with a previous study that reported that approximately 20%–50% of paralogous genes from gene duplication are conserved as one of the duplicates acquires a new function or subfunction (Lynch and Force, 2000).
Hormones can modulate the capacity for acid secretion through genomic and/or cytogenic pathways in fish (Guh et al., 2015; Yan and Hwang, 2019). For example, cortisol has been suggested to increase acid secretion at HRCs by stimulating the mRNA expression of acid-secreting transporters (Lin et al., 2015). Additionally, cortisol enhances ionocyte differentiation and elevates the systemic capacity for acid secretion (Cruz et al., 2013). Vitamin D and VDR have been shown to regulate the gene expression related to cellular differentiation (Gil et al., 2018). In the present study, treatment with 1α,25(OH)2D3 considerably stimulated the gcm2 expression, but knockdown of VDRs prevented this increase. gcm2 is a transcription factor specifically expressed in HRCs but not in Na+/K+-ATPase-rich cells or other ionocytes of zebrafish and is essential for the differentiation and functional regulation of HRCs (Chang et al., 2009). In addition, our preliminary result showed exogenous 1α,25(OH)2D3 treatment significantly increased HRCs density in 3 dpf zebrafish larvae (Supplemental Figure S2). Thus, vitamin D may increase the gcm2 expression and promote HRC differentiation in zebrafish. A previous study indicated that PTH1 knockdown reduces the gcm2 expression and the number of HRCs; however, when gcm2 is overexpressed in zebrafish after PTH1 knockdown, the decreased number of HRCs is recovered (Kwong and Perry, 2015). In mammals, parathyroid hormone is a major stimulator of vitamin D synthesis (Khundmiri et al., 2016). Therefore, PTH1 may increase vitamin D synthesis in zebrafish, and then, the increased vitamin D levels may enhance ionocyte differentiation. However, further studies are required to determine the role of vitamin D in ionocyte differentiation.
In summary, the present study reported that the synthesis and secretion levels of 1α,25(OH)2D3 were induced in zebrafish after AFW exposure, and the increased 1α,25(OH)2D3 levels may increase the expression of acid-secreting transporters in HRCs via VDRa and VDRb. This results in an increase in the acid secretion capacity of zebrafish larvae to prevent acidosis of body fluids. The present study is the first attempt to demonstrate a relationship between vitamin D and the acid-base balance in fish. This study extends our understanding of the role of vitamin D in ion regulation of fishes.
Data availability statement
The original contributions presented in the study are included in the article/Supplementary Materials. Further inquiries can be directed to the corresponding author.
Ethics statement
The animal study was reviewed and approved by NKUST Institutional Animal Care and Utilization Committee.
Author contributions
C-HL provided the conception and study design; C-HL, S-TL, Y-CW, Y-LT, and H-JH carried out the experiments and data collection; C-HL and S-TL performed the data analysis and interpretation; C-HL wrote the manuscript. All authors contributed to the article and approved the submitted version.
Funding
This work was partly supported by research grants from the Ministry of Science and Technology of Taiwan (MOST109-2313-B-992-003-MY3) and in part by the iEGG and Animal Biotechnology Center from The Feature Area Research Center Program within the framework of the Higher Education Sprout Project by the Ministry of Education (MOE), Taiwan (MOE 109-S-0023-A) to C-H Lin.
Conflict of interest
The authors declare that the research was conducted in the absence of any commercial or financial relationships that could be construed as a potential conflict of interest.
Publisher’s note
All claims expressed in this article are solely those of the authors and do not necessarily represent those of their affiliated organizations, or those of the publisher, the editors and the reviewers. Any product that may be evaluated in this article, or claim that may be made by its manufacturer, is not guaranteed or endorsed by the publisher.
Supplementary material
The Supplementary Material for this article can be found online at: https://www.frontiersin.org/articles/10.3389/fmars.2022.990502/full#supplementary-material
Abbreviations
1α,25(OH)2D3, 1α, 25-dihydroxyvitamin D3; AE1b, anion exchanger 1b; AFW, acidic freshwater; ANOVA, analysis of variance; dpf, days post-fertilization; FW, freshwater; HA, H+-ATPase; HRCs, H+-ATPase-rich cells; NHE3, Na+/H+ exchanger 3; PBS, phosphate-buffered saline; PTH1, parathyroid hormone 1; SEM, standard error of the mean; SIET, Scanning ion-selective electrode technique; VDR, vitamin D receptor.
References
Binswanger U., Helmle-Kolb C., Forgo J., Mrkic B., Murer H. (1993). Rapid stimulation of Na+/H+ exchange by 1,25-dihydroxyvitamin D3; interaction with parathyroid-hormone-dependent inhibition. Pflügers Arch. 424, 391–397. doi: 10.1007/BF00374899
Bobulescu I. A., Moe O. W. (2006). Na+/H+ exchangers in renal regulation of acid-base balance. Semin. Nephrol. 26, 334–344. doi: 10.1016/j.semnephrol.2006.07.001
Chang W. J., Horng J. L., Yan J. J., Hsiao C. D., Hwang P. P. (2009). The transcription factor, glial cell missing 2, is involved in differentiation and functional regulation of h+-ATPase-rich cells in zebrafish (Danio rerio). Am. J. Physiol. Regul. Integr. Comp. Physiol. 296, R1192–R1201. doi: 10.1152/ajpregu.90973.2008
Cheng W., Guo L., Zhang Z., Soo H. M., Wen C., Wu W., et al. (2006). HNF factors form a network to regulate liver-enriched genes in zebrafish. Dev. Biol. 294 (2), 482–496. doi: 10.1016/j.ydbio.2006.03.018
Chou M. Y., Lin C. H., Chao P. L., Hung J. C., Cruz S. A., Hwang P. P. (2015). Stanniocalcin-1 controls ion regulation functions of ion-transporting epithelium other than calcium balance. Int. J. Biol. Sci. 11 (2), 122–132. doi: 10.7150/ijbs.10773
Craig T. A., Sommer S., Sussman C. R., Grande J. P., Kumar R. (2008). Expression and regulation of the vitamin d receptor in the zebrafish, Danio rerio. J. Bone Miner. Res. 23 (9), 1486–1496. doi: 10.1359/jbmr.080403
Cruz S. A., Lin C. H., Chao P. L., Hwang P. P. (2013). Glucocorticoid receptor, but not mineralocorticoid receptor, mediates cortisol regulation of epidermal ionocyte development and ion transport in zebrafish (Danio rerio). PloS One 8, e77997. doi: 10.1371/journal.pone.0077997
du Bois M. B., Milet C., Garabedian M., Guillozo H., Martelly E., Lopez E., et al. (1988). Calcium-dependent metabolism of 25-hydroxycholecalciferol in silver eel tissues. Gen. Comp. Endocrinol. 71 (1) 1–9. doi: 10.1016/0016-6480(88)90288-2
Evans D. H., Piermarini P. M., Choe K. P. (2005). The multifunctional fish gill: Dominant site of gas exchange, osmoregulation, acid-base regulation, andexcretion of nitrogenous waste. Physiol. Rev. 85, 97–177. doi: 10.1152/physrev.00050.2003
Gill R., Nazir T. M., Wali R., Sitrin M., Brasitus T. A., Ramaswamy K., et al. (2002). Regulation of rat ileal NHE3 by 1,25(OH)2-vitamin D3. Dig. Dis. Sci. 47 (5), 1169–1174. doi: 10.1023/a:1015071014584
Gil Á., Plaza-Diaz J., Mesa M. D. (2018). Vitamin d: Classic and novel actions. Ann. Nutr. Metab. 72 (2), 87–95. doi: 10.1159/000486536
Guh Y. J., Lin C. H., Hwang P. P. (2015). Osmoregulation in zebrafish: Ion transport mechanisms and functional regulation. EXCLI J. 14, 627–659. doi: 10.17179/excli2015-246
Guh Y. J., Tseng Y. C., Yang C. Y., Hwang P. P. (2014). Endothelin-1 regulates H+-ATPase-dependent transepithelial H+ secretion in zebrafish. Endocrinology 155 (5), 1728–1737. doi: 10.1210/en.2013-1775
Hayes M. E., Guilland-Cumming D. F., Russell R. G., Henderson I. W. (1986). Metabolism of 25-hydroxycholecalciferol in a teleost fish, the rainbow trout (Salmo gairdneri). Gen. Comp. Endocrinol. 64, 143–150. doi: 10.1016/0016-6480(86)90040-7
Horng J. L., Lin L. Y., Hwang P. P. (2009). Functional regulation of h+- ATPase-rich cells in zebrafish embryos acclimated to an acidic environment. Am. J. Physiol. Cell Physiol. 296, C682–C692. doi: 10.1152/ajpcell.00576.2008
Howarth D. L., Law S. H., Barnes B., Hall J. M., Hinton D. E., Moore L., et al. (2008). Paralogous vitamin d receptors in teleosts: Transition of nuclear receptor function. Endocrinology 149 (5), 2411–2422. doi: 10.1210/en.2007-1256
Hsu H. H., Lin L. Y., Tseng Y. C., Horng J. L., Hwang P. P. (2014). A new model for fish ion regulation: Identification of ionocytes in freshwater- and seawater-acclimated medaka (Oryzias latipes). Cell. Tissue. Res. 357, 225–243. doi: 10.1007/s00441-014-1883-z
Hwang P. P., Lee T. H., Lin L. Y. (2011). Ion regulation in fish gills: Recent progress in the cellular and molecular mechanisms. Am. J. Physiol. Regul. Integr. Comp. Physiol. 301, R28–R47. doi: 10.1152/ajpregu.00047.2011
Hwang P. P., Perry S. F. (2010). “Ionic and acid-base regulation,” in Fish physiology vol. 29. zebrafish. Eds. Perry S. F., Ekker M., Farrell A. P., Brauner C. J. (San Diego, CA: Academic Press), p.311–p.343.
Karet F. E. (2002). Inherited distal renal tubular acidosis. J. Am. Soc Nephrol. 13, 2178–2184. doi: 10.1097/01.ASN.0000023433.08833.88
Khundmiri S. J., Murray R. D., Lederer E. (2016). PTH and vitamin D. Comp. Physiol. 6 (2), 561–601. doi: 10.1002/cphy.c140071
Krasowski M. D., Ai N., Hagey L. R., Kollitz E. M., Kullman S. W., Reschly E. J., et al. (2011). The evolution of farnesoid X, vitamin d, and pregnane X receptors: Insights from the green-spotted pufferfish (Tetraodon nigriviridis) and other non-mammalian species. BMC Biochem. 12, 5. doi: 10.1186/1471-2091-12-5
Kumai Y., Nesan D., Vijayan M. M., Perry S. F. (2012). Cortisol regulates na+ uptake in zebrafish, Danio rerio, larvae via the glucocorticoid receptor. Mol. Cell. Endocrinol. 364, 113–125. doi: 10.1016/j.mce.2012.08.017
Kwong R. W. M., Perry S. F. (2015). An essential role for parathyroid hormone in gill formation and differentiation of ion-transporting cells in developing zebrafish. Endocrinology 156 (7), 2384–2394. doi: 10.1210/en.2014-1968
Kwong R. W. M., Perry S. F.. (2014). The physiology of fish at low pH: The zebrafish as a model system. J. Exp. Biol. 217, 651–62. doi: 10.1242/jeb.091603
Lee Y. C., Yan J. J., Cruz S., Horng J. L., Hwang P. P. (2011). Anion exchanger 1b, but not sodium-bicarbonate cotransporter 1b, plays a role in transport functions of zebrafish h+-ATPase-rich cells. Am. J. Physiol. Cell Physiol. 300, C295–C307. doi: 10.1152/ajpcell.00263.2010
Lin L. Y., Horng J. L., Kunkel J. G., Hwang P. P. (2006). Proton pump-rich cell secretes acid in skin of zebrafish larvae. Am. J. Physiol. Cell Physiol. 290, C371–C378. doi: 10.1152/ajpcell.00281.2005
Lin C. H., Hu H. J., Chuang H. J., Tsou Y. L., Hwang P. P. (2021). Cortisol and glucocorticoid receptor 2 regulate acid secretion in medaka (Oryzias latipes) larvae. J. Comp. Physiol. B. 191 (5), 855–864. doi: 10.1007/s00360-021-01390-w
Lin C. H., Hwang P. P. (2016). The control of calcium metabolism in zebrafish (Danio rerio). Int. J. Mol. Sci. 17, 1783. doi: 10.3390/ijms17111783
Lin C. C., Lin L. Y., Hsu H. H., Thermes V., Prunet P., Horng J. L., et al. (2012). Acid secretion by mitochondrion-rich cells of medaka (Oryzias latipes) acclimated to acidic freshwater. Am. J. Physiol. Regul. Integr. Comp. Physiol. 302 (2), R283–R291. doi: 10.1152/ajpregu.00483.2011
Lin C. H., Shih T. H., Liu S. T., Hsu H. H., Hwang P. P. (2015). Cortisol regulates acid secretion of h+-ATPase-richionocytes in zebrafish (Danio rerio) embryos. Front. Physiol. 6. doi: 10.3389/fphys.2015.00328
Lin C. H., Su C. H., Tseng D. Y., Ding F. C., Hwang P. P. (2012). Action of vitamin d and the receptor, VDRa, in calcium handling in zebrafish (Danio rerio). PloS One 7 (9), e45650. doi: 10.1371/journal.pone.0045650
Liu S. T., Horng J. L., Chen P. Y., Hwang P. P., Lin L. Y. (2016). Salt secretion is linked to acid-base regulation of ionocytes in seawater-acclimated medaka: New insights into the salt-secreting mechanism. Sci. Rep. 6, 31433. doi: 10.1038/srep31433
Lynch M., Force A. (2000). The probability of duplicate gene preservation by subfunctionalization. Genetics 154 (1), 459–473. doi: 10.1093/genetics/154.1.459
Omdahl J. L., Morris H. A., May B. K. (2002). Hydroxylase enzymes of the vitamin d pathway: Expression, function, and regulation. Annu. Rev. Nutr. 22, 139–166. doi: 10.1146/annurev.nutr.22.120501.150216
Pan W., Borovac J., Spicer Z., Hoenderop J., Bindels R., Shull G., et al. (2012). The epithelial sodium/proton exchanger, NHE3, is necessary for renal and intestinal calcium (re)absorption. Am. J. Physiol. Renal. Physiol. 302 (8), F943–F956. doi: 10.1152/ajprenal.00504.2010
Perry S. F., Gilmour K. M. (2006). Acid-base balance and CO2 excretion in fish: unanswered questions and emerging models. Respir. Physiol. Neurobiol. 154, 199–215. doi: 10.1016/j.resp.2006.04.010
Reschly E. J., Bainy A. C., Mattos J. J., Hagey L. R., Bahary N., Mada S. R., et al. (2007). Functional evolution of the vitamin d and pregnane X receptors. D BMC Evol. Biol. 7, 222. doi: 10.1186/1471-2148-7-222
Shih T. H., Horng J. L., Hwang P. P., Lin L. Y. (2008). Ammonia excretion by the skin of zebrafish (Danio rerio) larvae. Am. J. Physiol. Cell Physiol. 295, C1625–C1632. doi: 10.1152/ajpcell.00255.2008
Shih T. H., Horng J. L., Liu S. T., Hwang P. P., Lin L. Y. (2012). Rhcg1 and NHE3b are involved in ammonium-dependent sodium uptake by zebrafish larvae acclimated to low-sodium water. Am. J. Physiol. Regul. Integr. Comp. Physiol. 302, R84–R93. doi: 10.1152/ajpregu.00318.2011
Stim J. A., Bernardo A. A., Arruda J. A. (1994). The role of parathyroid hormone and vitamin d in acid excretion and extrarenal buffer mobilization. Miner. Electrolyte. Metab. 20 (1-2), 60–71.
Sundell K., Norman A. W., Björnsson B. T. (1993). 1,25(OH)2 vitamin D3 increases ionized plasma calcium concentrations in the immature Atlantic cod. Gadus morhua. Gen. Comp. Endocrinol. 91 (3), 344–351. doi: 10.1006/gcen.1993.1135
Swarup K., Das V. K., Norman A. W. (1991). Dose-dependent vitamin D3 and 1,25-dihydroxyvitamin D3-induced hypercalcemia and hyperphosphatemia in male cyprinoid Cyprinus carpio. Comp. Biochem. Physiol. A. Physiol. 100 (2), 445–447. doi: 10.1016/0300-9629(91)90497-Z
Takeuchi A., Okano T., Kobayashi T. (1991). The existence of 25-hydroxyvitamin D3–1 alpha-hydroxylase in the liver of carp and bastard halibut. Life. Sci. 48, 275–282. doi: 10.1016/0024-3205(91)90355-f
Taylor J. S., Van de Peer Y., Braasch I., Meyer A. (2001). Comparative genomics provides evidence for an ancient genome duplication event in fish. philos. Trans. R. Soc Lond. B. Biol. Sci. 356, 1661–1679. doi: 10.1098/rstb.2001.0975
Wagner C. A., Finberg K. E., Breton S., Marshansky V., Brown D., Geibel J. P. (2004). Renal vacuolar-ATPase. Physiol. Rev. 84, 1263–1314. doi: 10.1152/physrev.00045.2003
Wali R. K., Baum C. L., Bolt M. J., Brasitus T. A., Sitrin M. D. (1992). 1,25-dihydroxyvitamin D3 inhibits na+-h+ exchange by stimulating membrane phosphoinositide turnover and increasing cytosolic calcium in caco-2 cells. Endocrinology 131 (3), 1125–1133. doi: 10.1210/endo.131.3.1324151
Keywords: zebrafish, vitamin D, acid secretion, H+-ATPase, ionocyte
Citation: Lin C-H, Liu S-T, Wang Y-C, Tsou Y-L and Hu H-J (2022) Vitamin D regulates transepithelial acid secretion in zebrafish (Danio rerio) larvae. Front. Mar. Sci. 9:990502. doi: 10.3389/fmars.2022.990502
Received: 10 July 2022; Accepted: 20 September 2022;
Published: 12 October 2022.
Edited by:
Mathilakath Vijayan, University of Calgary, CanadaReviewed by:
Srijit Chakravarty, Central Institute of Fisheries Education (ICAR), IndiaJiun-Lin Horng, Taipei Medical University, Taiwan
Copyright © 2022 Lin, Liu, Wang, Tsou and Hu. This is an open-access article distributed under the terms of the Creative Commons Attribution License (CC BY). The use, distribution or reproduction in other forums is permitted, provided the original author(s) and the copyright owner(s) are credited and that the original publication in this journal is cited, in accordance with accepted academic practice. No use, distribution or reproduction is permitted which does not comply with these terms.
*Correspondence: Chia-Hao Lin, Y2gxMjNAbmt1c3QuZWR1LnR3