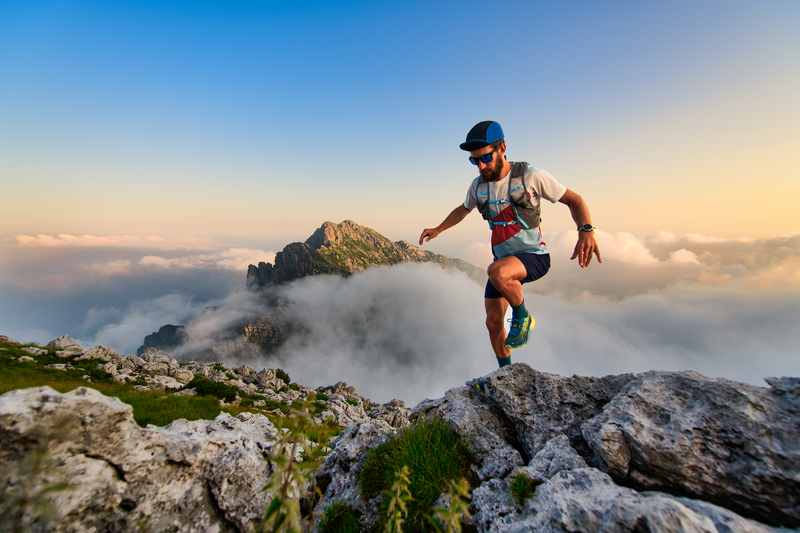
95% of researchers rate our articles as excellent or good
Learn more about the work of our research integrity team to safeguard the quality of each article we publish.
Find out more
ORIGINAL RESEARCH article
Front. Mar. Sci. , 22 August 2022
Sec. Marine Biogeochemistry
Volume 9 - 2022 | https://doi.org/10.3389/fmars.2022.986415
This article is part of the Research Topic Carbon Dynamics in Freshwater, Coastal and Oceanic Ecosystems in Response to the SDG Goals View all 12 articles
Seagrass ecosystems rank among the most effective blue carbon sinks in climate change mitigation and greenhouse gas removal. Nutrient pollution has emerged as a leading threat to seagrass decline and has diminished the carbon sequestration potential in recent decades. Changes in the nutrient regime can also impact the organic carbon compositions (labile and refractory organic carbon compositions) of seagrass tissues, with important implications for determining the quantity and quality of carbon sequestration. However, there is still little information about the impact of nutrient loading on seagrass plant refractory organic carbon composition (ROC), which hinders our ability to reveal the driving mechanisms of anthropogenic factors that decrease seagrass organic carbon sequestration capability. Here, a multidisciplinary approach was employed to investigate the organic carbon variations of Halophila beccarii at five seagrass meadows with contrasting nutrient loading levels. The results showed that H. beccarii plant nitrogen (N) content ranged from 2.21% to 5.65%, which well reflected the external nutrient loading levels. High nutrient loading elevated labile organic carbon content, like free amino acids and soluble sugars. Nevertheless, ROC content (cellulose-associated organic matter) decreased with increasing nutrient loading, which presented a significant negative linear correlation with plant N content. These results provide evidence that eutrophic conditions enhance H. beccarii plant quality (high N and labile organic carbon) and consequently decrease plant ROC sequestration potential. This suggests that reducing nitrogen input to seagrass meadows would aid in increasing seagrass carbon storage.
Seagrass ecosystems are one of the coastal blue carbon ecosystems and have great potential to mitigate global climate change (Macreadie et al., 2019). Although only occupying<0.2% of the global ocean surface, seagrass ecosystems contribute ~10% of marine organic carbon sequestration (Duarte et al., 2013). Seagrass contributes to more than half of all organic carbon storage (Kennedy et al., 2010), and in some seagrass meadows, it even accounts for 90% (Reef et al., 2017), which is mostly due to the abundance of refractory chemical compositions of organic matter (>50%) in seagrass plants (Trevathan-Tackett et al., 2017). Refractory carbon like lignocellulose is selectively preserved in sediments to avoid decomposition (Klap et al., 2000), while polysaccharides are continuously reduced during the diagenetic process through complex biochemical reactions (Kaal et al., 2019). Therefore, seagrass plant organic carbon compositions, particularly their refractory compositions, are the foundation of the high accumulation and preservation potential of organic carbon in seagrass ecosystems (Kaal et al., 2019).
Organic carbon in seagrass plants can be generally divided into labile organic carbon (LOC) compositions and refractory organic carbon (ROC) compositions (Kaal et al., 2016). Amino acids, non-structural carbohydrate compounds (NSC, such as, soluble sugar, and starch), and hemicellulose are the main components of seagrass LOC, while ROC is always represented as cellulose and lignin (Zieman et al., 1984; Peduzzi and Herndl, 1991; Vichkovitten and Holmer, 2004; Trevathan-Tackett et al., 2017; Cragg et al., 2020). Many common chemical methods are used for measuring the contents of plant organic carbon compounds. For example, the phenol–sulfuric acid colorimetric method was used to determine soluble sugar and starch contents (Dubois, 1956), and the vanillin/HCl method, near-infrared reflectance spectroscopy, and filter-bag technique were applied for measuring the contents of tannin, fiber, and lignin, respectively (Lawler et al., 2006; Torbatinejad et al., 2007; Siegalwillott et al., 2010). Thermogravimetric analysis (TGA) is a quantitative technique that uses the increase or decrease in the mass of substances with temperature to characterize physical and chemical changes and has been widely used for plant chemical composition analysis recently (Dimitrakopoulos, 2001). TGA provides a lower material mass (10 mg) and the ability to provide accurate and rapid component characterization (Dimitrakopoulos, 2001). The organic carbon composition of marine macroalgae and vascular plants was assessed by TGA. For example, previous reports have suggested that the lignocellulose content of seagrass rhizomes is 50%–60% of dry weight (Trevathan-Tackett et al., 2017), and marine macroalgae do contain refractory compounds including xylans and sulfated polysaccharides (Trevathan-Tackett et al., 2016).
Coastal nutrient loading is a leading threat to seagrass meadow decline (Jiang et al., 2020), which is mainly due to algal overgrowth and reduction of light availability, as well as ammonium toxicity (Burkholder et al., 2007). Meanwhile, nutrient loading can affect the carbon metabolism in seagrass plants (Touchette and Burkholder, 2000), and consequently change the organic carbon composition of seagrass plants (Pazzaglia et al., 2020; Helber et al., 2021a). Nutrient addition can always increase the content of amino acids in seagrass tissues (Udy and Dennison, 1997; Invers et al., 2004), while the response of sucrose content in seagrass to nutritional loading shows variation. For example, nitrogen and phosphorus enrichment were found to increase sucrose contents in Enhalus acoroides leaf (Artika et al., 2020), but sucrose contents of Halophila stipulacea and Posidonia oceanica leaf were observed unchanged and decreased after nitrogen and phosphorus additions, respectively (Pazzaglia et al., 2020; Helber et al., 2021b). Previous studies reported that nutrient loading could change the LOC content of seagrass plants (Brun et al., 2002; Touchette and Burkholder, 2007; Jiménez-Ramos et al., 2017; Artika et al., 2020; Hernán et al., 2021). However, there is still little information on seagrass ROC changes in response to nutrient loading.
Halophila beccarii is a small monoecious seagrass mainly distributed throughout the tropical Indo-Pacific region (Udagedara and Dahanayaka, 2020). The blue carbon stock of H. beccarii meadows ranged from 2.655 Mg C/ha to 12 Mg C/ha (Jiang et al., 2017; Kaladharan et al., 2021). The organic carbon concentration in the sediment within the H. beccarii seagrass meadow is 110%–134% greater than nearby bare sediment (Kaladharan et al., 2021), possibly due to abundant H. beccarii plant organic carbon inputs into in situ sediment (Premarathne et al., 2021). H. beccarii is widely distributed in tropical and subtropical regions of China, which are at a risk of further nutrient loading pressure due to intense human activities (urban runoffs, fishing, dredging) (Jiang et al., 2017; Jiang et al., 2020). This trend could accelerate the loss of seagrass ecosystems and a reduction in carbon contribution. In this study, we investigated H. beccarii LOC and ROC compositions in five different nutrient-loaded seagrass meadows in the Guangdong and Hainan provinces, Southern China. We hypothesized that nutrient loading would increase the LOC content but decrease the ROC of H. beccarii. The goal of this study was to further determine the relationship between eutrophication and carbon storage capacity of H. beccarii and, with this result, help resource managers maximize the organic carbon sequestration potential in seagrass ecosystems.
During October 2021, we collected the H. beccarii plants from five seagrass meadows: Yifengxi (YFX, 23.54N, 116.92E), Hailing Island (HL, 21.68N, 111.91E), Liusha Bay (LS, 20.39N, 109.98E), Tangjia Bay (TJ, 22.33N, 113.59E), and Qiaotou (QT, 19.948N, 110.093 E) (Figure 1). Three 25 cm × 25 cm quadrats (distance between quadrats is >5 m) were randomly thrown into each seagrass meadow, and H. beccarii plants were dug out together with underground roots in the quadrats to ensure the integrity of the plants. After being rinsed with seawater, they were put into polyethylene sealing bags. Meanwhile, seawater around each quadrat was collected and quickly filtered onto the GF/F filter. The seagrass plants and filtered seawater samples were stored at −20 °C until they were back in the laboratory.
The SEAL AutoAnalyzer 3 High Resolution (Seal Analytical, BRAN+LUEBBE, Germany) was used to analyze dissolved inorganic nitrogen (DIN = nitrate + nitrite + ammonium) and dissolved inorganic phosphate (DIP) in filtered seawater. The Seal AA3 HR is a completely automated analysis system for colorimetric analysis of dissolved nutrients in environmental samples (Zhang et al., 2019).
The whole body of seagrass plants was first dried at 60 °C in an oven to constant weight, and then the dry sample was fully ground to a homogenized state by a grinding instrument (mortar grinder ST-B220, SKTEM, China). Powdered samples were analyzed in duplicate for carbon and nitrogen content using a CHN analyzer (Vario EL, Elemental Analyser System GmbH, Germany). Elemental content was calculated on a dry weight basis (mass of element/dry weight of sample ∗ 100%), while elemental ratios were calculated on a mole: mole basis. All isotopic analyses were measured using a standard isotope ratio mass spectrometer (Thermo Scientific MAT 253) procedures. All isotopic data were expressed in the conventional delta notation (‰): δ13Csampleor δ15Nsample= (Rsample/Rreference− 1) × 1,000 where R = 13C/12C or 15N/14N. Soluble sugars (sucrose, glucose, and fructose) from ground samples were measured by the anthrone colorimetric method (Jan and Roel, 1993). Free amino acid was measured using the Ninhydrin colorimetric method with an absorbance of 570 nm (Lee et al., 2003).
The remaining dry samples of the whole body of the seagrass were subsequently analyzed by thermogravimetric analysis (TGA, TG209F1, 0.1 μg balance sensitivity, Netzsch, Germany). About 10 mg of the ground sample was placed in a platinum cup and heated under N2(gas flow = 100 ml min−1) with a heating ramp rate of 10°C min−1, ranging from 28 to 600°C (Liu et al., 2020). The rate-of-change derivative (% mass loss °C−1) can be used to describe the thermal intervals (TI) and consequently indicates distinct temperatures of organic matter (OM) loss. The TI1 (labile; soluble carbohydrates, hemicellulose) ranged from 180°C to 300°C. TI2 (cellulose-associated recalcitrant organic matter) extended from 300°C to 400°C, then from 400°C to 600°C was determined as TI3 (lignin-associated recalcitrant organic matter and insoluble polysaccharide residues). The range of mass loss (TI1-3) was recalculated as a portion of total organic matter (OM) from 180°C to 600°C. The proportions of TI1, TI2, and TI3 in total OM are represented as TI1/OM, TI2/OM, and TI3/OM.
One-way analysis of variance (ANOVA) was used to determine if there were any statistically significant changes in environmental nutrients, elemental content, soluble sugars, amino acids, TI1, TI2, TI3, and OM of H. beccarii among the five seagrass meadows. A Tukey post hoc test was applied to identify the significantly different components of the above parameters. The effect, with p<0.05, was considered statistically significant. Before this test, the homogeneity of variance was tested with Levene’s test. Data were log-transformed to achieve the homogeneity of variance where the heterogeneity of variance was found. TI1, TI2, TI3, TI1/OM, TI2/OM, and TI3/OM are converted into arcsine square roots before ANOVA. The relationships among seawater nutrients and seagrass nitrogen content with organic carbon compositions were examined by the Pearson’s correlation coefficient, respectively. The above-mentioned statistical analyses were performed using Excel 2010, SPSS 22.0, and R 4.1.3, respectively.
DIN and DIP of seawater ranged from 14.89–162.94 μmol/L to 0.18–7.79 μmol/L, respectively, with significant differences among the five seagrass meadows (Table 1, DIN: F = 15.050, p<0.001; DIP: F = 5.541, p = 0.013). , , and accounted for 1.67%–5.09%, 31.79%–75.11%, and 22.55%–63.12% of DIN, respectively. There were significant differences in all the above-mentioned environmental variables among the five seagrass meadows (Table 1). The DIN of YFX was 4-fold higher than that of LS, and the DIN contents of HL, TJ, and QT did not differ significantly (F = 0.202, p = 0.823).
Table 1 Seawater nutrients parameters in HI, LS, TJ, YFX, and QT (Mean ± SE). The different capital letters indicate significant differences among the five seagrass meadows. Identical superscripts indicate non-significantly different means.
Biochemical parameters of H. beccarii were observed to be significantly different among the sampling sites, with the exception of carbon content (Table 2). We recorded a broad range of nitrogen content values and C/N ratios of H. beccarii. The nitrogen content and C/N ratios for H. beccarii ranged from 2.21% to 5.65% and from 7.24 to 15.57, respectively (Table 2). The highest and lowest nitrogen content and C/N ratio values were both observed in YFX and QT, respectively (Table 2). However, the carbon content of plants in the five seagrass meadows tended to be similar (Table 2; F = 1.964, p = 0.176).
Table 2 Carbon, Nitrogen Content, Stable Isotope Analysis (δ13C and δ15N) of seagrass tissue in five meadows (Mean ± SE).
The δ13C signature of H. beccarii in the five seagrass meadows ranged from −20.764‰ to −14.802‰, with HL and LS as the most depleted and richest values, respectively (Table 2). Additionally, there was a significantly higher δ15N value in H. beccarii of YFX than in other seagrass meadows (Table 2). The soluble sugar content of YFX was triple-fold higher than that of LS (Figure 2A), while the amino acid content of H. beccarii at YFX was higher than that at other sites (Figure 2B).
Figure 2 Souble sugars (A) and free amino acid (B) content of seagrass plant in five areas. Different letters above bars indicate significant differences (p<0.05) among regions. Values represent mean ± S.E. Due to the lack of sample size of QT, variables just have one or two values.
TGA showed that the decomposition resulted in an obvious loss of organic matter for H. beccarii in five seagrass meadows (Figure 3). All samples manifested similar Z-shaped TG curves and the rate of change indicated that most of the differences among the areas occurred during losses between 100 °C–180 °C and 200 °C–400 °C (Figure 3). Figure 4 shows the specific mass loss of organic matter for H. beccarii. Although the total OM content of H. beccarii in HL was ~10% higher than that in LS, no significant differences were observed in the OM content of H. beccarii among the different seagrass meadows (Figure 4D; F = 1.518, p = 0.269). Overall, TI1 generally decreased as the nutrient level decreased (Figure 4A), while the opposite trend was observed in TI2 (Figure 4B). Furthermore, there was no significant difference in the TI3 content among the five seagrass meadows (Figure 4C; F = 1.064, p = 0.423). According to the proportion of different TIs in OM, the proportion of TI1/OM and TI2/OM differed significantly among the five seagrass meadows (TI1/OM: F = 47.336, p<0.001; TI2/OM: F = 38.749, p<0.001) (Figure 5). Similar to the TI3, the TI3/OM was homogeneous among the three seagrass meadows (F = 3.106, p = 0.066) (Figure 5).
Figure 3 Thermograms for the seagrass among five seagrass meadows, (A) % mass remaining with increasing temperature and (B) derivate rate-of-change (% mass loss per °C) with increasing pyrolysis temperature.
Figure 4 TI1 (A), TI2 (B), TI3 (C), and OM (D) as percent of total mass (Mean ± S.E.). TIs represent distinct organic matter components percent in total mass from TGA (TI1: labile, amino acid, carbohydrates, hemicellulose, 180–300°C; TI2: cellulose-associated recalcitrant organic matter, 300–400°C; and TI3: refractory, lignin-associated recalcitrant organic matter and residues, 400–600°C).
Figure 5 Thermal intervals (TIs) in total organic matter (OM) across all location was represented as TIs/OM. TIs represent distinct organic matter components from TGA. Values represent mean ± S.E.
The heatmap based on Pearson’s correlation analysis revealed that the amino acids, soluble sugars, TI1, and TI1/OM of H. beccarii had significantly positive correlations with N content (Figure 6A). In contrast, TI2/OM showed a significantly negative correlation with N content (Figure 6A). TI2 was negatively correlated with N content, but not significantly (Figure 6A). Furthermore, there was no positive or negative correlation between TI3 or TI3/OM and N content (Figure 6A). Additionally, amino acids, soluble sugars of H. beccarii had significantly positive correlations with seawater while TI2/OM had a significantly negative correlation with seawater (Figure 6B).
Figure 6 Relationship between nitrogen content and thermal organic matter of seagrass (A). Relationship between seawater nutrient concentrations and thermal organic matter of seagrass (B). Blue represents a positive correlation between variables and red represents a negative correlation (*p<0.05, **p<0.01, ***p<0.001).
The seawater nutrients in our study seagrass meadows (DIN: 14.89–162.94 μmol/L, DIP: 0.18–7.79 μmol/L) were higher than those in other seagrass meadows in Florida Bay (Median DIN = 3.56 µmol/L, Median TP = 0.31 µmol/L) (Fourqurean et al., 2003), Alfaques Bay, Mediterranean ( <10 µmol/L, DIP: 0.1–0.5 µmol/L) (Pérez and Romero, 1994), Cau Hai lagoon, Vietnam (DIN: 77.45 μg/L) (Dang et al., 2020), and Akrotiri Bay, Cyprus, Red Sea (DIN: 0.03–0.65 µmol/L) (Alexandre et al., 2014), which suggested that the H. beccarii seagrass meadows distributed along the southern China coastal areas were exposed to a higher nutrient loading pressure. The DIN and DIP concentrations among five seagrass meadows indicated distinct nutrient loading pressure that H. beccarii suffered. TJ had the lowest concentration of DIP, which can be attributed to its open water conditions and intense hydrodynamic exchange. External inorganic nutrients (nitrogen and phosphorus) can be absorbed by seagrass leaves and roots, with absorption kinetics that depend on external inorganic nutrient concentrations (Brun et al., 2002). The nutrient content of seagrass plants has been widely used as a good indicator for seagrass health monitoring and can well reflect the external nutrient loading (Yang et al., 2018). Plants from low nutrient environments have significantly lower N-content and higher C:N ratios than those from high-nutrient environments (Lee and Dunton, 1999). In this study, the N content of H. beccarii plants had significantly positive relationships with, and DIN, but not with (Supplementary Figure 1). Although both and can be absorbed by H. beccarii, content is generally low in the water column and uptake by seagrass is a more energy-saving process than (Turpin, 2010). In the study area, it can be assumed that represents a substantial source of nitrogen for H. beccarii. However, H. beccarii plant nitrogen content in LS was much higher than that in TJ and QT, although LS has the lowest external nutrient load. This should be ascribed to macroalgae bloom (Ulva lactuca) at LS during our sampling period (Supplementary Figure 2), resulting in a decrease in nutrient levels in the water column due to macroalgae absorption. Additionally, variation in δ15N can be used as an indicator of eutrophication as well (Cole et al., 2004). More enriched H. beccarii δ15N in LS than in QT suggested that there were more human wastewater inputs (Helber et al., 2021b). Therefore, H. beccarii plant nitrogen content well reflected the external nutrient loading.
Carbon and nitrogen metabolism are interconnected. An alteration in one process is likely to set off cascading responses affecting other biochemical mechanisms. Variations in external nutrient loading may cause the change of carbohydrate storage reserves in seagrass through nitrogen metabolism. TI1 represented the total content of soluble carbohydrates (soluble sugar and starch), amino acids, and hemicellulose. does not accumulate in healthy seagrass leaves due to its toxicity in chloroplasts, so energy-dependent rapid assimilation into amino acids is catalyzed by the glutamine synthetase/glutamate synthase pathway (Touchette and Burkholder, 2000), which should be responsible for the higher amino acid content in H. beccarii at high nutrient loading seagrass meadows (Invers et al., 2004). In our study, a significant positive correlation was found between amino acid content and N content, seawater , , and DIN. Soluble sugar is the main component of non-structural carbohydrate (TNC) (Vichkovitten et al., 2007), acting as a carbon reserve for ammonium assimilation via the tricarboxylic acid cycle (Brun et al., 2008). Under nutrient enrichment, the internal carbohydrate concentrations decreased significantly, which was mainly due to the nitrogen assimilation process (Brun et al., 2008) and the lack of an ammonium uptake feedback mechanism (Burkholder et al., 1992). In contrast to previous studies (Campbell et al., 2012; Helber et al., 2021b), soluble sugar content was higher at the high nutrient seagrass meadow in this study. This might be due to keeping a high energy metabolism to cope with high nutrient loading conditions (Brun et al., 2002). Ammonium enrichment improved photosynthetic efficiency, allowing the tissues to meet the increased carbon demands caused by ammonium assimilation into organic nitrogen compounds (Brun et al., 2002). Additionally, H. beccarii soluble sugar at LS was observed to have the lowest content, which might be due to light deficiency during the macroalgae bloom period. Seagrass photosynthetic rate declines and mobilizes carbohydrate reserves to maintain metabolic processes under low light conditions (Ralph et al., 2007), resulting in a significant decrease in the soluble sugar content (Burke et al., 1996; Schmidt et al., 2012). Hemicellulose is a structural carbohydrate that plays a vital role in the strength of plant cell walls (Xu et al., 2020). Hemicellulose accounts for the major part of TI1, so the hemicellulose content should follow the same trend as TI1. Therefore, nutrient loading increased the quantity of labile components like amino acid and soluble sugar contents in the living H. beccarii plant. On the other hand, appropriate nutrient loading can promote seagrass production through changes in maximum rate of photosynthesis and increased chlorophyll concentrations as well (Agawin et al., 1996; Lee and Dunton, 1999), thus allowing for greater seagrass carbon fixation (Lee and Dunton, 1999). Nevertheless, only the seagrass plant organic carbon compositions were measured in this study, and the seagrass production in response to eutrophication should be conducted in future research.
Cellulose strands form microfibrils that act as a framework for other wall components such as hemicelluloses, proteins, and lignin (Ogden et al., 2018). The overall cellulose content of vascular plants varies substantially depending on the thermogravimetric interval, ranging from 24% to 50% (Trevathan-Tackett et al., 2016), with seagrass having a cellulose content of 33%–46% (Trevathan-Tackett et al., 2017). In this study, the mass loss of TI2 decreased as N content increased, and TI2/OM was negatively correlated with N content and seawater indicating that cellulose depletion under high nutrient loading conditions occur. In terrestrial ecosystems, high N leads to a reduction of cellulose in angiosperms, which is due to decreased expression of genes involved in cellulose production in response to elevated nitrogen levels (Zhang et al., 2017). Under high nutrient loading conditions, seagrass diverts carbon and energy produced by photosynthesis toward nitrogen uptake and assimilation, which consequently leads to less carbon and energy being allocated to the production of cellulose (Shan et al., 2018). This explains the difference in cellulose content of H. beccarii at different nitrogen content gradients. Furthermore, even though our study discovered no significant correlation between nitrogen and TI3 content, it does not suggest that nutrient loading had no impact on lignin biosynthesis in H. beccarii. Lignin is a refractory biomacromolecule composed of three phenylropanoid units: p-hydroxyphenyl (H), guaiacyl (G), and syringyl (S) (Mottiar et al., 2016), with determining the refractory degree of lignin (Bonawitz et al., 2014). The study of terrestrial ecosystems showed that nutrient loading significantly influenced the composition of these three monomers (Pitre et al., 2007). As a matter of future research, we recommend elucidating the effects of nutrient loading on the unit compositions of lignin in seagrass tissues, with important implications for seagrass blue carbon potential. Additionally, we did not distinguish the differences in organic carbon compositions between tissues by only using the whole body of H. beccarii. According to previous studies, the ratios of aboveground (leaf) to belowground biomass (rhizome and root) of H. beccarii were similar in different sites of Southern China (ranging from 0.71 to 1) (Jiang et al., 2020). We assumed that the samples collected in different sites had similar ratios of aboveground to belowground biomass.
Our study revealed that eutrophication could increase the content of labile organic carbon but reduce the refractory components by 4.19%–6.23% (Figure 7). It is necessary to point out that the effects of nutrient load on seagrass production and biomass were complex. Moderate nutrient loads may enhance the total production of seagrass (Connell et al., 2017). Extreme nutrient loads would hamper the production and biomass of seagrass (increased shading by epiphytic algae) (Schmidt et al., 2012). However, we did not measure the parameters of H. beccarii production or the biomass in this study, which limited us to estimating the trade-off of the plant carbon stock. In the future research, field investigation and laboratory simulation should be conducted to study the effects of eutrophication on H. beccarii primary production and organic carbon compositions. It has been widely reported that eutrophication has been identified as a leading threat to seagrass worldwide (Burkholder et al., 2007). Our study demonstrated that eutrophication would decrease the seagrass plant refractory carbon contribution to carbon sequestration. Therefore, better management of fish farming, shoreline rehabilitation, and sewage discharge and restoration measurements are required to regulate anthropogenic nutrient inputs and maintain their natural carbon sinks.
Figure 7 Impact of eutrophication on seagrass meadow. The dashed wave line and blue wave line represents high tide and low tide, respectively.
Interactions among seagrass organic carbon compositions and eutrophication were discussed in our study. Our results supported our initial hypothesis: eutrophication improves the content of labile organic carbon such as soluble sugars and amino acids while decreasing refractory organic carbon through the carbon allocation of H. beccarii. However, H. beccarii in southern China is threatened by accelerating eutrophication, which might weaken the contribution of seagrass plants to refractory carbon stock. One important subject is to determine nutrient thresholds for a maximum of seagrass carbon stock. In addition, the carbon and nitrogen cycles of seagrass, as well as the mechanisms by which labile and refractory carbon respond to eutrophication, need to be investigated further.
The original contributions presented in the study are included in the article/Supplementary Material. Further inquiries can be directed to the corresponding authors.
HL: Conceptualization, methodology, and writing—original draft. SL: Validation, methodology, writing—review and editing, and data curation. YR: Investigation, validation, and software. ZJ: Project administration and investigation. YW: Formal analysis and software. XZ: Resources and data curation. JL: Formal analysis. XH: Supervision, writing—review and editing, and funding acquisition. All authors contributed to the article and approved the submitted version.
This work was supported by the National Natural Science Foundation of China (U1901221, 42176155), the Key Special Project for Introduced Talents Team of Southern Marine Science and Engineering Guangdong Laboratory (Guangzhou) (GML2019ZD0405), Hainan Province Science and Technology Special Fund (ZDYF2020180, ZDYF2021SHFZ254), the Innovation Academy of South China Sea Ecology and Environmental Engineering, Chinese Academy of Sciences (ISEE2021PY06 and ISEE2021ZD03), and the Science and Technology Planning Project of Guangdong Province, China (2020B1212060058).
The authors declare that the research was conducted in the absence of any commercial or financial relationships that could be construed as a potential conflict of interest.
All claims expressed in this article are solely those of the authors and do not necessarily represent those of their affiliated organizations, or those of the publisher, the editors and the reviewers. Any product that may be evaluated in this article, or claim that may be made by its manufacturer, is not guaranteed or endorsed by the publisher.
The Supplementary Material for this article can be found online at: https://www.frontiersin.org/articles/10.3389/fmars.2022.986415/full#supplementary-material
Agawin N. S. R., Duarte C. M., Fortes M. D. (1996). Nutrient limitation of Philippine seagrasses (Cape bolinao, NW philippines): In situ experimental evidence. Mar. Ecol. Prog. Ser. 138 (1-3), 233–243. doi: 10.3354/meps138233
Alexandre A., Georgiou D., Rui S. (2014). Inorganic nitrogen acquisition by the tropical seagrass halophila stipulacea. Mar. Ecology. 35, 387–394. doi: 10.1111/maec.12128
Artika S. R., Ambo-Rappe R., Teichberg M., Moreira-Saporiti A., Viana I. G. (2020). Morphological and physiological responses of enhalus acoroides seedlings under varying temperature and nutrient treatment. Front. Mar. Science. 7. doi: 10.3389/fmars.2020.00325
Bonawitz N. D., Kim J. I., Tobimatsu Y., Ciesielski P. N., Anderson N. A., Ximenes E., et al. (2014). Disruption of mediator rescues the stunted growth of a lignin-deficient arabidopsis mutant. Nature 509, 376–380. doi: 10.1038/nature13084
Brun F. G., Hernández I., Vergara J. J., Peralta G., Pérez-Lloréns J. (2002). Assessing the toxicity of ammonium pulses to the survival and growth of zostera noltii. Mar. Ecol. Progress. 225, 177–118. doi: 10.3354/meps225177
Brun F., Olive I., Malta E. J., Vergara J., Pérez-Lloréns J. (2008). Increased vulnerability of zostera noltii to stress caused by low light and elevated ammonium levels under phosphate deficiency. Mar. Ecol. Prog. Series. 365, 67–75. doi: 10.3354/meps07512
Burke M. K., Dennison W. C., Moore K. A. (1996). Non-structural carbohydrate reserves of eelgrass zostera marina. Mar. Ecol. Prog. Series. 137, 195–201. doi: 10.3354/meps137195
Burkholder J., Mason K., Glasgow H. (1992). Water-column nitrate enrichment promotes decline of eelgrass zostera manna: evidence from seasonal mesocosm experiments. Mar. Ecol. Prog. Series. 81, 163–178. doi: 10.3354/meps081163
Burkholder J., Tomasko D. A., Touchette B. W. (2007). Seagrasses and eutrophication. J. Exp. Mar. Biol. Ecology. 350, 46–72. doi: 10.1016/j.jembe.2007.06.024
Campbell J. E., Yarbro L. A., Fourqurean J. W. (2012). Negative relationships between the nutrient and carbohydrate content of the seagrass thalassia testudinum. Aquat. Botany. 99, 56–60. doi: 10.1016/j.aquabot.2012.02.002
Cole M. L., Valiela I., Kroeger K. D., Tomasky G. L., Cebrian J., Wigand C., et al. (2004). Assessment of a delta15N isotopic method to indicate anthropogenic eutrophication in aquatic ecosystems. J. Environ. Quality. 33, 124–132. doi: 10.2134/jeq2004.012
Connell S. D., Fernandes M., Burnell O. W., Doubleday Z. A., Griffin K. J., Irving A. D., et al. (2017). Testing for thresholds of ecosystem collapse in seagrass meadows. Conserv. Biol. 31 (5), 1196–1201. doi: 10.1111/cobi.12951
Cragg S. M., Friess D. A., Gillis L. G., Trevathan-Tackett S. M., Terrett O. M., Watts J. E., et al. (2020). Vascular plants are globally significant contributors to marine carbon fluxes and sinks. Annu. Rev. Mar. science. 12, 469–497. doi: 10.1146/annurev-marine-010318-095333
Dang T., Hoang N. T., Lieu P. K., Harada H., Venterink H. O. (2020). Interspecific variation in foliar nutrients and isotopes of submerged macrophytes in the cau hai lagoon, the typical brackish lagoon in vientam. Botanica Pacifica. 9, 61–72. doi: 10.17581/bp.2020.09207
Dimitrakopoulos A. P. (2001). Thermogravimetric analysis of Mediterranean plant species. J. Analytical Appl. Pyrolysis. 60, 123–130. doi: 10.1016/S0165-2370(00)00164-9
Duarte C. M., Losada I. J., Hendriks I. E., Mazarrasa I., Marbà N. (2013). The role of coastal plant communities for climate change mitigation and adaptation. Nat. Climate Change. 3, 961–968. doi: 10.1038/NCLIMATE1970
Dubois M. (1956). Colorimetric method for determination of sugars and related substances. Analytical Chem. 28, 350–356. doi: 10.1021/ac60111a017
Fourqurean J. W., Boyer J. N., Durako M. J., Hefty L. N., Peterson B. J. (2003). Forecasting responses of seagrass distributions to changing water quality using monitoring data. Ecol. Applications. 13, 474–489. doi: 10.1890/1051-0761(2003)013[0474:Frosdt]2.0.Co;2
Helber S. B., Procaccini G., Belshe E. F., Santillan-Sarmiento A., Cardini U., Broehl S., et al. (2021a). Unusually warm summer temperatures exacerbate population and plant level response of posidonia oceanica to anthropogenic nutrient stress. Front. Plant Science. 12. doi: 10.3389/fpls.2021.662682
Helber S. B., Winters G., Stuhr M., Belshe E., Bröhl S., Schmid M., et al. (2021b). Nutrient history affects the response and resilience of the tropical seagrass halophila stipulacea to further enrichment in its native habitat. Front. Plant science. 12. doi: 10.3389/fpls.2021.678341
Hernán G., Ortega M. J., Henderson J., Alós J., Boyer K., Cimon S., et al. (2021). Latitudinal variation in plant defence against herbivory in a marine foundation species does not follow a linear pattern: The importance of resource availability. Global Ecol. Biogeography. 30, 220–234. doi: 10.1111/geb.13217
Invers O., Kraemer G. P., Pérez M., Romero J. (2004). Effects of nitrogen addition on nitrogen metabolism and carbon reserves in the temperate seagrass posidonia oceanica. J. Exp. Mar. Biol. Ecology. 303, 97–114. doi: 10.1016/j.jembe.2003.11.005
Jan B., Roel M. (1993). An improved colorimetric method to quantify sugar content of plant tissue. J. Exp. Botany. 44, 1627–1629. doi: 10.1093/jxb/44.10.1627
Jiang Z., Cui L., Liu S., Zhao C., Wu Y., Chen Q., et al. (2020). Historical changes in seagrass beds in a rapidly urbanizing area of guangdong province: Implications for conservation and management. Global Ecol. Conserv. 22, e01035. doi: 10.1016/j.gecco.2020
Jiang Z., Liu S., Zhang J., Zhao C., Wu Y., Yu S., et al. (2017). Newly discovered seagrass beds and their potential for blue carbon in the coastal seas of hainan island, south China Sea. Mar. pollut. Bulletin. 125, 513–521. doi: 10.1016/j.marpolbul.2017.07.066
Jiménez-Ramos R., Egea L. G., Ortega M. J., Hernández I., Vergara J. J., Brun F. G. (2017). Global and local disturbances interact to modify seagrass palatability. PloS One 12, e0183256. doi: 10.1371/journal.pone.0183256
Kaal J., Serrano O., Cortizas A. M., Baldock J. A., Lavery P. S. (2019). Millennial-scale changes in the molecular composition of posidonia australis seagrass deposits: Implications for blue carbon sequestration. Organic Geochemistry. 137, 103898. doi: 10.1016/j.orggeochem.2019.07.007
Kaal J., Serrano O., Nierop K. G., Schellekens J., Cortizas A. M., Mateo M.-Á. (2016). Molecular composition of plant parts and sediment organic matter in a Mediterranean seagrass (Posidonia oceanica) mat. Aquat. Botany. 133, 50–61. doi: 10.1016/j.aquabot.2016.05.009
Kaladharan P., Lavanya R., Akshara C. (2021). Capture and storage of carbon in seagrass beds of a vulnerable species halophila beccarii (Asch.) at the kadalundi estuary, kerala, India. J. Mar. Biol. Assoc. India 63 (2), 126–129. doi: 10.6024/jmbai.2021.63.2.2284-19
Kennedy H., Beggins J., Duarte C. M., Fourqurean J. W., Holmer M., Marbà N., et al. (2010). Seagrass sediments as a global carbon sink: Isotopic constraints. Global Biogeochemical Cycles. 24, GB4026. doi: 10.1029/2010GB003848
Klap A. V., Hemminga M. A., Boon J. J. (2000). Retention of lignin in seagrasses: angiosperms that returned to the sea. Mar. Ecol. Prog. Series. 194, 1–11. doi: 10.3354/meps194001
Lawler I. R., Aragones L., Berding N., Marsh H., Foley W. (2006). Near-infrared reflectance spectroscopy is a rapid, cost-effective predictor of seagrass nutrients. J. Chem. Ecology. 32, 1353–1365. doi: 10.1007/s10886-006-9088-x
Lee K. S., Dunton K. H. (1999). Influence of sediment nitrogen-availability on carbon and nitrogen dynamics in the seagrass thalassia testudinum. Mar. Biol. 134, 217–226. doi: 10.1007/s002270050540
Lee S. W., Lim J. M., Bhoo S. H., Paik Y. S., Hahn T. R. (2003). Colorimetric determination of amino acids using genipin from gardenia jasminoides. Analytica Chimica Acta 480, 267–274. doi: 10.1016/S0003-2670(03)00023-0
Liu S., Trevathan-Tackett S. M., Ewers Lewis C. J., Huang X., Macreadie P. I. (2020). Macroalgal blooms trigger the breakdown of seagrass blue carbon. Environ. Sci. Technol. 54, 14750–14760. doi: 10.1021/acs.est.0c03720
Macreadie P. I., Anton A., Raven J. A., Beaumont N., Duarte C. M. (2019). The future of blue carbon science. Nat. Commun. 10, 3998. doi: 10.1038/s41467-019-11693-w
Mottiar Y., Vanholme R., Boerjan W., Ralph J., Mansfield S. D. (2016). Designer lignins: harnessing the plasticity of lignification. Curr. Opin. Biotechnol. 37, 190–200. doi: 10.1016/j.copbio.2015.10.009
Ogden M., Hoefgen R., Roessner U., Persson S., Khan G. A. (2018). Feeding the walls: How does nutrient availability regulate CellWall composition? Int. J. Mol. Sci. 19, 2691. doi: 10.3390/ijms19092691
Pazzaglia J., Santillán-Sarmiento A., Helber S. B., Ruocco M., Procaccini G. (2020). Does warming enhance the effects of eutrophication in the seagrass posidonia oceanica? Front. Mar. Science. 7. doi: 10.3389/fmars.2020.564805
Peduzzi P., Herndl G. J. (1991). Decomposition and significance of seagrass leaf litter(Cymodocea nodosa) for the microbial food web in coastal waters(Gulf of Trieste, northern Adriatic Sea). Mar. Ecol. Prog. series. Oldendorf. 71, 163–174. doi: 10.3354/meps071163
Pérez M., Romero J. (1994). Growth dynamics, production, and nutrient status of the seagrass cymodocea nodosa in a Mediterranean semi-estuarine environment. Mar. Ecology. 15, 51–64. doi: 10.1111/j.1439-0485.1994.tb00041.x
Pitre F. E., Pollet B., Lafarguette F., Cooke J. E. K., MacKay J. J., Lapierre C. (2007). Effects of increased nitrogen supply on the lignification of poplar wood. J. Agric. Food Chem. 55, 10306–10314. doi: 10.1021/jf071611e
Premarathne C., Jiang Z., He J., Fang Y., Huang X. (2021). Low light availability reduces the subsurface sediment carbon content in halophila beccarii from the south China Sea. Front. Plant Science. 12. doi: 10.3389/fpls.2021.664060
Ralph P. J., Durako M. J., Enríquez S., Collier C. J., Doblin M. A. (2007). Impact of light limitation on seagrasses. J. Exp. Mar. Biol. Ecology. 350, 176–193. doi: 10.1016/j.jembe.2007.06.017
Reef R., Atwood T. B., Samper-Villarreal J., Adame M. F., Sampayo E. M., Lovelock C. E. (2017). Using eDNA to determine the source of organic carbon in seagrass meadows. Limnology Oceanography. 62, 1254–1265. doi: 10.1002/lno.10499
Schmidt A. L., Wysmyk J. K. C., Craig S. E., Lotze H. K. (2012). Regional-scale effects of eutrophication on ecosystem structure and services of seagrass beds. Limnology Oceanography 57, 1389–1402. doi: 10.4319/lo.2012.57.5.1389
Shan L., Song C., Zhang X., Ren J. (2018). Effects of long-term nitrogen and phosphorus addition on plant defence compounds in a freshwater wetland. Ecol. Indicators. 94, 1–6. doi: 10.1016/j.ecolind.2018.06.017
Siegalwillott J. L., Harr K., Hayek L., Scott K. C., Gerlach T., Sirois P., et al. (2010). Proximate nutrient analyses of four species of submerged aquatic vegetation consumed by Florida manatee (Trichechus manatus latirostris) compared to romaine lettuce (Lactuca sativa var. longifolia). J. Zoo Wildlife Med. 41, 594–602. doi: 10.1638/2009-0118.1
Torbatinejad N. M., Annison G., Rutherfurd-Markwick K., Sabine J. R. (2007). Structural constituents of the seagrass posidonia australis. J. Agric. Food Chem. 55, 4021–4026. doi: 10.1021/jf063061a
Touchette B. W., Burkholder J. M. (2000). Review of nitrogen and phosphorus metabolism in seagrasses. J. Exp. Mar. Biol. Ecology. 250, 133–167. doi: 10.1016/S0022-0981(00)00195-7
Touchette B. W., Burkholder J. M. (2007). Carbon and nitrogen metabolism in the seagrass, zostera marina l.: Environmental control of enzymes involved in carbon allocation and nitrogen assimilation. J. Exp. Mar. Biol. Ecology. 350, 216–233. doi: 10.1016/j.jembe.2007.05.034
Trevathan-Tackett S. M., Kelleway J., Macreadie P. I., Beardall J., Ralph P., Bellgrove A. (2016). Comparison of marine macrophytes for their contributions to blue carbon sequestration. Ecology 96, 3043–3057. doi: 10.1890/15-0149.1
Trevathan-Tackett S. M., Macreadie P. I., Sanderman J., Baldock J., Howes J. M., Ralph P. J. (2017). A global assessment of the chemical recalcitrance of seagrass tissues: Implications for long-term carbon sequestration. Front. Plant Science. 8. doi: 10.3389/fpls.2017.00925
Turpin D. H. (2010). Effects of inorganic n availability on algal photosynthesis and carbon metabolism. J. Phycology. 27, 14–20. doi: 10.1111/j.0022-3646.1991.00014.x
Udagedara S., Dahanayaka D. D. G. L. (2020). Current status and checklist of seagrass in Sri Lanka. Int. J. Aquat. Biology-IJAB. 8, 317–326. doi: 10.22034/ijab.v8i5.619
Udy J. W., Dennison W. C. (1997). Growth and physiological responses of three seagrass species to elevated sediment nutrients in moreton bay, Australia. J. Exp. Mar. Biol. Ecology. 217, 253–277. doi: 10.1016/S0022-0981(97)00060-9
Vichkovitten T., Holmer M. (2004). Contribution of plant carbohydrates to sedimentary carbon mineralization. Organic geochemistry 35, 1053–1066. doi: 10.1016/j.orggeochem.2004.04.007
Vichkovitten T., Holmer M., Frederiksen M. S. (2007). Spatial and temporal changes in non-structural carbohydrate reserves in eelgrass (Zostera marina l.) in Danish coastal waters. Botanica Marina 50 (2), 75–87. doi: 10.1515/bot.2007.009
Xu L., Zhou J., Ni J., Li Y., Long Y., Huang R. (2020). Investigating the pyrolysis kinetics of pinus sylvestris using thermogravimetric analysis. Bioresources 15, 5577–5592. doi: 10.15376/biores.15.3.5577-5592
Yang X., Zhang P., Li W., Hu C., Zhang X., He P. (2018). Evaluation of four seagrass species as early warning indicators for nitrogen overloading: Implications for eutrophic evaluation and ecosystem management. Sci. Total Environment. 635, 1132–1143. doi: 10.1016/j.scitotenv.2018.04.227
Zhang P. Y., Grutters B. M. C., van Leeuwen C. H. A., Xu J., Petruzzella A., van den Berg R. F., et al. (2019). Effects of rising temperature on the growth, stoichiometry, and palatability of aquatic plants. Front. Plant Sci. 9. doi: 10.3389/fpls.2018.01947
Zhang W., Wu L., Ding Y., Yao X., Wu X., Weng F., et al. (2017). Nitrogen fertilizer application affects lodging resistance by altering secondary cell wall synthesis in japonica rice (Oryza sativa). J. Plant Res. 130, 859–871. doi: 10.1007/s10265-017-0943-3
Keywords: Halophila beccarii, nutrient loading, organic carbon, labile, recalcitrant
Citation: Luo H, Liu S, Ren Y, Jiang Z, Wu Y, Zhang X, Li J and Huang X (2022) Eutrophication decreases Halophila beccarii plant organic carbon contribution to sequestration potential. Front. Mar. Sci. 9:986415. doi: 10.3389/fmars.2022.986415
Received: 05 July 2022; Accepted: 02 August 2022;
Published: 22 August 2022.
Edited by:
Jeng-Wei Tsai, China Medical University, TaiwanReviewed by:
Kenta Watanabe, Port and Airport Research Institute (PARI), JapanCopyright © 2022 Luo, Liu, Ren, Jiang, Wu, Zhang, Li and Huang. This is an open-access article distributed under the terms of the Creative Commons Attribution License (CC BY). The use, distribution or reproduction in other forums is permitted, provided the original author(s) and the copyright owner(s) are credited and that the original publication in this journal is cited, in accordance with accepted academic practice. No use, distribution or reproduction is permitted which does not comply with these terms.
*Correspondence: Xiaoping Huang, eHBodWFuZ0BzY3Npby5hYy5jbg==; Songlin Liu, bGl1c29uZ2xpbkBzY3Npby5hYy5jbg==
Disclaimer: All claims expressed in this article are solely those of the authors and do not necessarily represent those of their affiliated organizations, or those of the publisher, the editors and the reviewers. Any product that may be evaluated in this article or claim that may be made by its manufacturer is not guaranteed or endorsed by the publisher.
Research integrity at Frontiers
Learn more about the work of our research integrity team to safeguard the quality of each article we publish.