- 1Ocean School, Yantai University, Yantai, China
- 2Key Laboratory of Experimental Marine Biology, Center for Ocean Mega-Science, Institute of Oceanology, Chinese Academy of Sciences, Qingdao, China
- 3Laboratory for Marine Biology and Biotechnology, Qingdao National Laboratory for Marine Science and Technology, Qingdao, China
- 4Algal and Microbial Biotechnology Division, Faculty of Biosciences and Aquaculture, Nord University, Bodø, Norway
- 5Faculty of Core Research, Natural Science Division, Ochanomizu University, Tokyo, Japan
- 6Faculty of Biological Science, Wonkwang University, Iksan, South Korea
- 7Department of Benthic Ecology, GEOMAR Helmholtz-Zentrum für Ozeanforschung Kiel, Kiel, Germany
- 8Centre of Marine Sciences, University of Algarve, Campus de Gambelas, Faro, Portugal
Ocean warming is one of the most important factors in shaping the spatial distribution and genetic biodiversity of marine organisms worldwide. The northwest Pacific has been broadly illustrated as an essential seaweed diversity hotspot. However, few studies have yet investigated in this region on whether and how past and ongoing climate warming impacted the distribution and genetic pools of coastal seaweeds. Here, we chose the invasive species Gracilaria vermiculophylla as a model, and identified multiple genetic lineages in the native range through genome-scale microsatellite genotyping. Subsequently, by reconstructing decadal trends of sea surface temperature (SST) change between 1978 and 2018, we found that SST in northern Japan and the East China Sea indeed increased broadly by 0.25-0.4°C/decade. The projections of species distribution models (SDMs) under different future climate change scenarios (RCP 2.6, RCP 4.5, RCP 6.0 and RCP 8.5) indicated that a unique genetic pool of G. vermiculophylla at its current southern range limit (i.e. the South China Sea) is at high risk of disappearance, and that the populations at its current northern range limit (i.e. in Hokkaido region) will undergo poleward expansions, particularly by the year 2100. Such responses, along with this species’ limited dispersal potential, may considerably alter the contemporary distribution and genetic composition of G. vermiculophylla in the northwest Pacific, and ultimately threaten ecological services provided by this habitat-forming species and other associated functional roles.
Introduction
The northwest Pacific, comprising both cold- and warm-temperate marine biogeographic provinces (Briggs and Bowen, 2012), is a global seaweed biodiversity hotspot with high species richness and endemism (Keith et al., 2014; Fragkopoulou et al., 2022). For example, approximately 1500, 1300 and 900 species of seaweeds have been reported in Japan, China and Korea, respectively (Ding et al., 2011; Kim et al., 2013; Yoshida et al., 2015), including 515 endemics in the Southern South China Sea and 85 endemics in the Jeju Island (Lee, 2008; Ding et al., 2011). These seaweeds not only provide food, habitat and nursery services to many marine organisms such as fish and invertebrates, but also have been used as raw materials for food, feed and fertilizer ingredients, leading to successful practices of commercial cultivar selection, genetic breeding and aquaculture over the past decades (i.e. the kelps Saccharina japonica and Undaria pinnatifida in East Asia, Hwang et al., 2019; Hu et al., 2021).
Global marine biodiversity patterns have been relatively stable over the last millennium but are now confronted with rapid changes due to climate warming (Molinos et al., 2015). This trend is believed to apply for coastal seaweeds, as well. The thermal regime, by regulating a variety of life activities such as life-histories and photosynthetic performances, has long been listed as one of the key factors determining the survival and distribution of intertidal and benthic seaweeds (Hoek, 1982; Jueterbock et al., 2013). In the north Atlantic, long-term field monitoring, ecological experiments and phylogeographic surveys showed that ocean warming, mediated by climate change, is threatening the genetic diversity and spatial distribution of seaweeds (Lima et al., 2007; Jueterbock et al., 2013; Zardi et al., 2015; Neiva et al., 2015), including the potential loss of unique gene pools of some habitat-forming species (i.e. Fucus vesiculosus, Nicastro et al., 2013; Fucus guiryi, Lourenco et al., 2016). Furthermore, species distribution models (SDMs) progressively forecast that ocean warming will cause severe contractions at species’ rear edges of distribution and poleward expansions (Jueterbock et al., 2013; Neiva et al., 2015; Song et al., 2021; Assis et al., 2022). This warming-induced range shift of seaweeds also stimulated a few parallel studies in the northwest Pacific and south Pacific. For instance, the kelp Saccharina flourishes in northern Japan in winter, at sea surface temperatures (SST) below 5°C, but ocean warming caused a considerable reduction of standing biomass (>70%) in the Shakotan Peninsula in Hokkaido in the 1990s (Akaike, 2000). In addition, on the coast of Cape Muroto (Kochi, Shikoku, Japan), the mean SST increased by 1.24°C between 1970 and 2010. In response, the tropical species Sargassum ilicifolium gradually expanded its range and replaced temperate Sargassum species, and also the kelp Ecklonia cava (Tanaka et al., 2012). Likewise, in temperate Australia, ocean warming has been found to cause large-scale range contraction in the kelp Macrocystis pyrifera (Wernberg et al., 2011), including the deleterious effects on the habitat-forming kelp E. radiata across its range (Flukes et al., 2015). These research efforts provide only an initial glimpse of climate-mediated impacts on seaweeds in the northwest Pacific. Recently, phylogeographic screening and SDM projections showed that S. thunbergii expanded its range by 18 degrees of latitude in the northwest Pacific since the Last Glacial Maximum (LGM, c. 21,000 years ago), and predicted that some unique gene lineages will be lost under future climate warming (Song et al., 2021). However, these studies cannot present generalized patterns about seaweeds’ responses to ocean warming, because taxonomically and/or physiologically (i.e. warm-temperate vs. cold temperate) different species usually exhibit variable functional responses to climatic and non-climatic environmental shifts, including the location of sheltered sites such as coastal upwelling climatic refugia (Lima et al., 2007; Martínez et al., 2012; Piñeiro-Corbeira et al., 2018). Studies, with a biogeographic scale across different marine provinces, may provide vigorous evidence on how seaweeds responded to past and contemporary climate warming, and hence may shed light on conserving and managing natural seaweed resources in a long-term sustainability perspective.
The agar-producing red seaweed Gracilaria vermiculophylla (Ohmi) Papenfuss is an intertidal habitat-forming species endemic to the northwest Pacific, and usually assemblages on natural hard substrata, muddy and sandy habitats. Gracilaria vermiculophylla is able to reproduce sexually and vegetatively (Guillemin et al., 2008), and its spermatia (male gametes) show extremely limited dispersal in space and time (Engel et al., 1999). This species had been previously renamed as Agarophyton vermiculophyllum based on phylogenetic data, but a more recent phylogenomic analysis re-classified it back to Gracilaria vermiculophylla (Lyra et al., 2021). Gracilaria vermiculophylla can provide superior nursey habitat and compensate for severe declines of coastal seagrass nurseries, and facilitate the emergence of a novel ecosystem (Johnston and Lipcius, 2012). The high rate of uptake of ammonium () and nitrate () makes G. vermiculophylla an ideal biofilter component of Integrated Multi-Trophic Aquaculture (IMTA) systems in estuarine environments (Abreu et al., 2011). Some unique ecological traits, such as euryhaline, eurytherm, and high tolerance to biotic and abiotic factors (for a review, see Hu and Lopez-Bautista, 2014), contributed to this species’ rapid colonization of new habitats along the north American and European coasts over the past 100 years (Krueger-Hadfield et al., 2017). Recent studies found that compared to the native range, the invaded G. vermiculophylla populations usually exhibited exceptional anti-epiphyte defence capability (Wang et al., 2017), microbial epibiont diversity (Saha et al., 2016; Bonthond et al., 2020), and heat-shock resistance (Hammann et al., 2016). These lines of evidence suggest that microbial diversity is vital for the growth, spatial persistence and expansion of G. vermiculophylla. However, climate warming has been illustrated to reduce microbial composition and stability (Hutchins and Fu, 2017), which in turn can affect microbial network complexity (Bonthond et al., 2020), potentially leading G. vermiculophylla in the native northwest Pacific at a high risk of distributional contraction and genetic diversity loss.
In the native northwest Pacific, population-level molecular investigations (i.e. amplified fragment length polymorphism and sequence variation at the mitochondrial cox1 gene) revealed multiple genetic lineages in G. vermiculophylla scattered along the South China Sea, the Yellow-Bohai Sea and the Japan-Pacific coasts, respectively, potentially driven by historical climate scenarios (i.e. the late Quaternary ice ages) and contemporary environmental conditions (i.e. the Yangtze diluted water) (Hu et al., 2018a; Zhong et al., 2020). From 1982 to 2010, the northwest Pacific had witnessed a dramatic increase in coastal summer-autumn SST, particularly along the coasts of the Yellow-Bohai Sea, East and South China Seas, where the warming rate exceeded 0.5°C per decade (Lima and Wethey, 2012). Similarly, SST around Japan has increased by an average of 1.1°C over the past 100 years (Japan Meteorological Agency, 2018). However, whether such intense ocean warming has already or will threaten the distribution range of G. vermiculophylla in the northwest Pacific, including the putative risk of diversity loss under future climate scenarios, remains unknown.
In this study, we firstly used 10 microsatellite loci to screen the fine-scale genetic structure of 38 G. vermiculophylla populations sampled across the northwest Pacific. We then assessed whether the chosen representative sampling sites displayed obvious coastal SST warming during the recent period of 1978–2018. Finally, we used SDMs to evaluate how the past climate fluctuations (i.e. the LGM) and future climate scenarios (Representative Concentration Pathway: RCP 2.6, RCP 4.5, RCP 6.0 and RCP 8.5) may have produced/will produce changes in the distribution range of G. vermiculophylla. These combined results such as the identification of unique genetic lineages and species’ distribution range shifts in response to climate changes, will provide valuable baselines information for monitoring and conserving G. vermiculophylla resources in the wild, including the ecosystem services it provided in the northwest Pacific.
Materials and methods
Sampling, DNA extraction and microsatellite genotyping
Thirty-eight populations were sampled in the period 2014–2019, from Hokkaido, Japan (44.05°N) to Beihai, China (21.49°N) (Table 1). Detailed field sampling methods are described in Zhong et al. (2020). In total, we obtained 538 attached specimens of G. vermiculophylla. Genomic DNA was isolated using the methods reported in Hu et al. (2004) and Zhong et al. (2020) with minor modifications (potassium acetate (5.0 M, pH 7.5) was used twice for extraction).
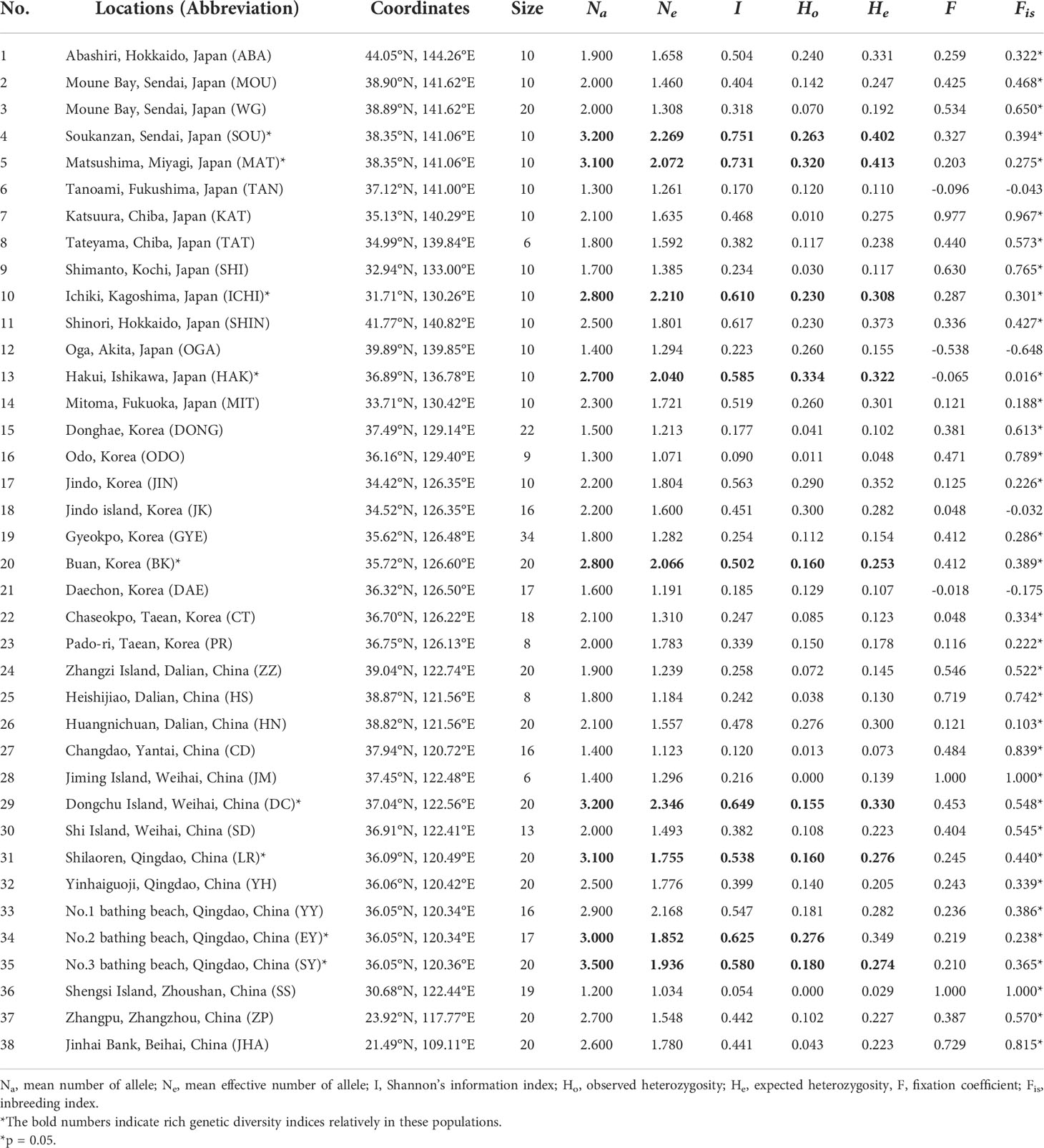
Table 1 Sampling information of G. vermiculophylla in the northwest Pacific and genetic diversity indices.
Based on a previous genetic screening of G. vermiculophylla (Krueger-Hadfield et al., 2016), 10 species-specific polymorphic microsatellite loci (Supplementary Table S1) were chosen to genotype all sampled specimens from all 38 populations. Each locus was amplified by polymerase chain reaction (PCR) as described in Song et al. (2021). The genotyping of 10 microsatellite loci was achieved by Beijing Qingke Biotechnology Co., Ltd (Qingdao, China). Allelic scores were checked manually for quality and consistency in STRAND (Toonen and Hughes, 2001) using the 350 ROX™ size standard (Applied Biosystems). We tested for linkage disequilibrium between each pair of loci with 20 batches and 5000 iterations per batch, and for deviations from Hardy-Weinberg equilibrium at each locus, using GENEPOP 4.1 (Raymond and Rousset, 1995; Rousset, 2008). We further checked for null alleles using MICROCHECKER (van Oosterhout et al., 2004) to avoid bias in estimating genetic indices. Genetic estimates, including Shannon’s information index (I), observed and expected heterozygosity (Ho and He, respectively), fixation coefficient (F), and inbreeding index (Fis) were calculated with GENALEX 6.5 (Peakall and Smouse, 2006) and FASTAT 2.9.3 (Goudet, 1995), respectively. The level of genetic differentiation (FST) was estimated with ARLEQUIN 3.5.1.3 (Excoffier and Lischer, 2010), and gene flow (Nm) among populations was measured with POPGENE 1.32 (Yeh et al., 1999).
The number of genetic clusters in G. vermiculophylla was inferred using Bayesian analysis in STRUCTURE 2.3.4 (Pritchard et al., 2000) with the admixture model and correlated allele frequencies. To estimate the optimal number of clusters (K = 1–10), the analysis ran with 10 independent simulations using a full-length of 106 Markov Chain Monte Carlo iterations and a burn-in of 105 steps. The best K value was determined using the log probability of the data Pr(x/K) and the ΔK criterion (Evanno et al., 2005) in Structure Harvester (Earl and Vonholdt, 2012). We subsequently calculated and summarized the average result over 10 runs in CLUMPP 1.1.2 (Jakobsson and Rosenberg, 2007) to obtain the final admixture results and viewed them in DISTRUCT 1.1 (Rosenberg, 2004).
Temperature trends in the northwest Pacific
SST along the northwest Pacific coast was retrieved between January 1978 and December 2018 at a 4-km resolution from the Moderate Resolution Imaging Spectroradiometer-Aqua (MODISAqua) dataset available from the National Aeronautics Space Administration (NASA) Goddard Earth Sciences (GES) Data and Information Services Center (DISC). The generation of the decadal average SST anomaly over the last 40 years followed the method described by Lima et al. (2007). To obtain a more precise picture of the warming trend from 1978 to 2018, decadal averages of SST were gathered for 17 stations (11 dispersed in latitudes 20.85°N–30.71°N and 6 dispersed in latitudes 41.86°N–42.32°N, Figure 1) spaced along the northwest Pacific.
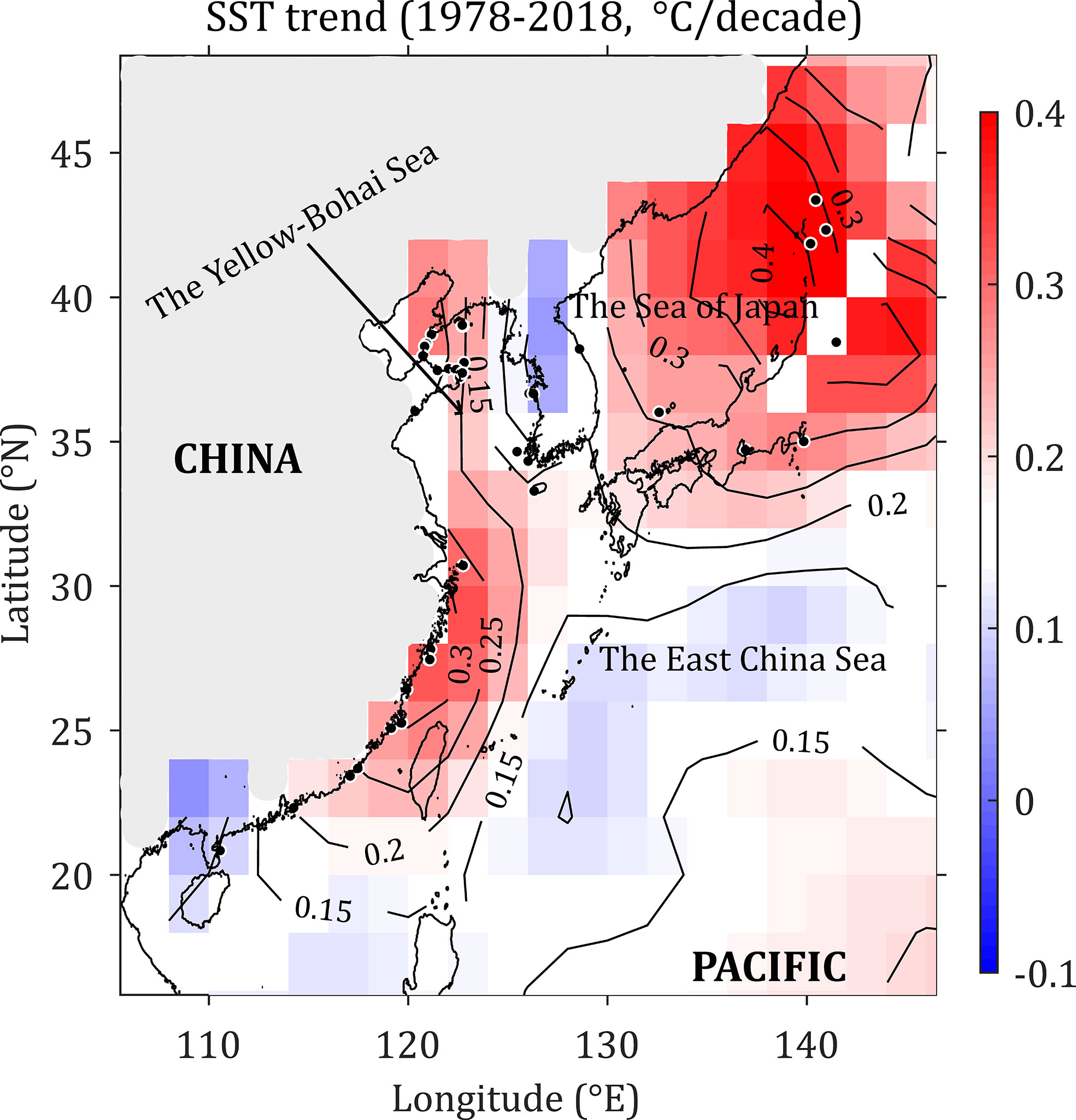
Figure 1 Decadal sea surface temperature (SST) rate of change (°C) in the northwest Pacific from January 1978 to December 2018. The light grey indicates the land part.
SDM projection: Occurrence records
Niche modeling was based on 100 literature-based occurrence records of G. vermiculophylla (Supplementary Figure S1 and Table S2). We thinned the occurrence records to avoid the model from misinterpreting areas with high local occurrence densities as such presenting highest habitat suitability (Phillips et al., 2009). Areas of high occurrence density were identified with the bkde2D function of the R package ‘KernSmooth’ 2.23-15 (Wand and Ripley, 2015), using a bandwidth of 3.0 in longitudinal and 1.5 in latitudinal direction. From these areas, 26 occurrence records were randomly removed by the java program ‘Occurrence Thinner’ v.1.04 (Verbruggen, 2012), using thresholds t1 = 0.6 and t2 = 1.0) (Figure S1; Table S2).
SDM projection: Background points
Environmental conditions in the distributional range of G. vermiculophylla were captured by 1,000 background (pseudo-absence) locations distributed randomly along the northwest Pacific coast within 10°N-60°N latitude and 95°E-160°E longitude with the R package ‘raster’ v.3.0.2 (Hijmans et al., 2011).
SDM projection: Variable selection
To avoid overfitting the models to the occurrence records, we reduced the full set of 62 environmental variables from the Bio-ORACLE database (Assis et al., 2018). Long-term mean and maximum Bio-ORACLE variables were excluded before variable selection given that no corresponding values were available for past or future conditions. Using the R package ‘MaxentVariableSelection’ (Jueterbock et al., 2016), we selected a set of uncorrelated variables (Pearson’s product moment correlation <0.8) with relative contribution scores >5% and a betamultiplier (between 0.5 and 10) that resulted in a model of lowest AICc [sample-size-adjusted Akaike information criterion (Akaike, 1974)]. We only tested models built with hinge features, as these generally provide good performance when there are at least 15 occurrence sites (Philipps and Dudík, 2009). In comparison with models selected by maximum AUC.Test (area under the receiver operating characteristic estimated from test data, (Fielding and Bell, 1997), models selected by minimum AICc better predict a species’ fundamental niche and better allow to transfer habitat suitability to novel environmental conditions (Warren and Seifert, 2011; Jiménez-Valverde, 2012; Warren et al., 2014). Model-overfitting was estimated by the difference between AUC values from test and training data (Warren and Seifert, 2011).
SDM projections of habitat suitability
We projected habitat suitability for G. vermiculophylla in the West Pacific under past, present, and future conditions using MAXENT 3.4.1 (Phillips et al., 2004; Phillips et al., 2017) with the settings and variables that characterized the model of highest performance (lowest AICc, see results). Projections into the past and future differed from the present-day projection in the mean ice thickness, and mean SST, the two environmental variables that could best discriminate suitable from non-suitable habitat. Mean SST projections for 21kya (LGM) and 6kya (mid Holocene, MH) were obtained from the PaleoMARSPEC data layers (Sbrocco, 2014). Mean ice thickness projections for the LGM and MH were compiled. Mean SST projections and ice thickness projections into years 2050 and 2100 under representative concentration pathway (RCP) scenarios (Collins et al., 2013). RCP 2.6 (low emissions peaking in 2010-2020), RCP 4.5 (medium emissions peaking in 2040), RCP 6.0 (medium emissions peaking in 2080), and RCP 8.5 (high emissions continuing to rise throughout the 21st century) were obtained from the Bio-ORACLE database (Assis et al., 2018). All projections were based on complementary log-log (cloglog) output grids (Fithian et al., 2015) averaged over 10 replicated MAXENT runs.
Results
Genetic diversity and lineage differentiation
We identified 126 allelic loci in 38 populations using 10 microsatellites, and the average number of loci was 12.6. Null alleles were observed, but they were not overrepresented in each locus or population. MICROCHECKER analysis did not identify genotyping errors of microsatellites. After false discovery rate (FDR) correction for multiple tests and Bonferroni correction, significant linkage disequilibrium for each pair of loci and deviation from Hardy-Weinberg equilibrium for each locus at all populations were 2.3% and 38% (p < 0.05) (Table S3), respectively. Diversity indices were the highest in the two populations from Sendai in Japan (SOU: Na=3.2, I=0.751, Ho=0.263; MAT: Na=3.1, I=0.731, Ho=0.320), besides the populations from Dongchu Island, Weihai (DC: Na=3.2, I=0.649, Ho=0.155), and Shilaoren, Qingdao (LR: Na=3.1, I=0.538, Ho=0.160), China that showed comparable estimates (Table 1). Diversity did not show a clear spatial gradient, but G. vermiculophylla populations in China were genetically more diverse (the average Na=2.35) than those in Korea (the average Na=1.99) and Japan (the average Na=2.2) (Table 1). In addition, Fis estimates showed that most populations were characterized by heterozygote deficiency (Fis ≥ 0.016), except for the four populations (TAN, OGA, JK and DAE: -0.648≤ Fis ≤-0.032, Table 1).
Pairwise FST values based on microsatellites revealed high levels of genetic differentiation among populations of G. vermiculophylla (93.5% of FST values > 0.25) with most estimates being statistically significant (p=0.05) (Table S4). Extremely low levels of genetic differentiations were observed among populations from Weihai and Qingdao, China (FST = 0.017–0.271, population numbers 29-35 in Table S4). In particular, the three populations from southern China were strongly differentiated (the average FST = 0.772, population numbers 36-38 in Table S4) from all other populations. Nm estimates showed very limited gene flow among most of the 38 G. vermiculophylla populations, and only 3.7% estimates suggested frequent genetic exchange (Nm >1.0) among populations (Table S4).
Bayesian clustering of the 38 populations indicated 4 distinct genetic lineages (the optimal value of ΔK = 4, Figures 2, 3). Namely, all 14 populations from Japan and 4 populations from the east and southwest coasts of Korea (DONG, ODO, JIN and JK) formed a ‘blue’ lineage, 5 populations from the west coast of Korea and 5 populations sampled north of 37.40°N in the Yellow-Bohai Sea formed a ‘green’ lineage, and the ‘red’ and ‘yellow’ lineages that consisted of 10 populations were distributed in the Yellow and South China Seas, respectively (Figures 2, 3). When K was set to 6, the ‘blue’ lineage was divided into two sub-clades, and the three populations (SS, ZP and JHA) from southern China formed a distinct ‘brown’ lineage (Figures 2, 3).
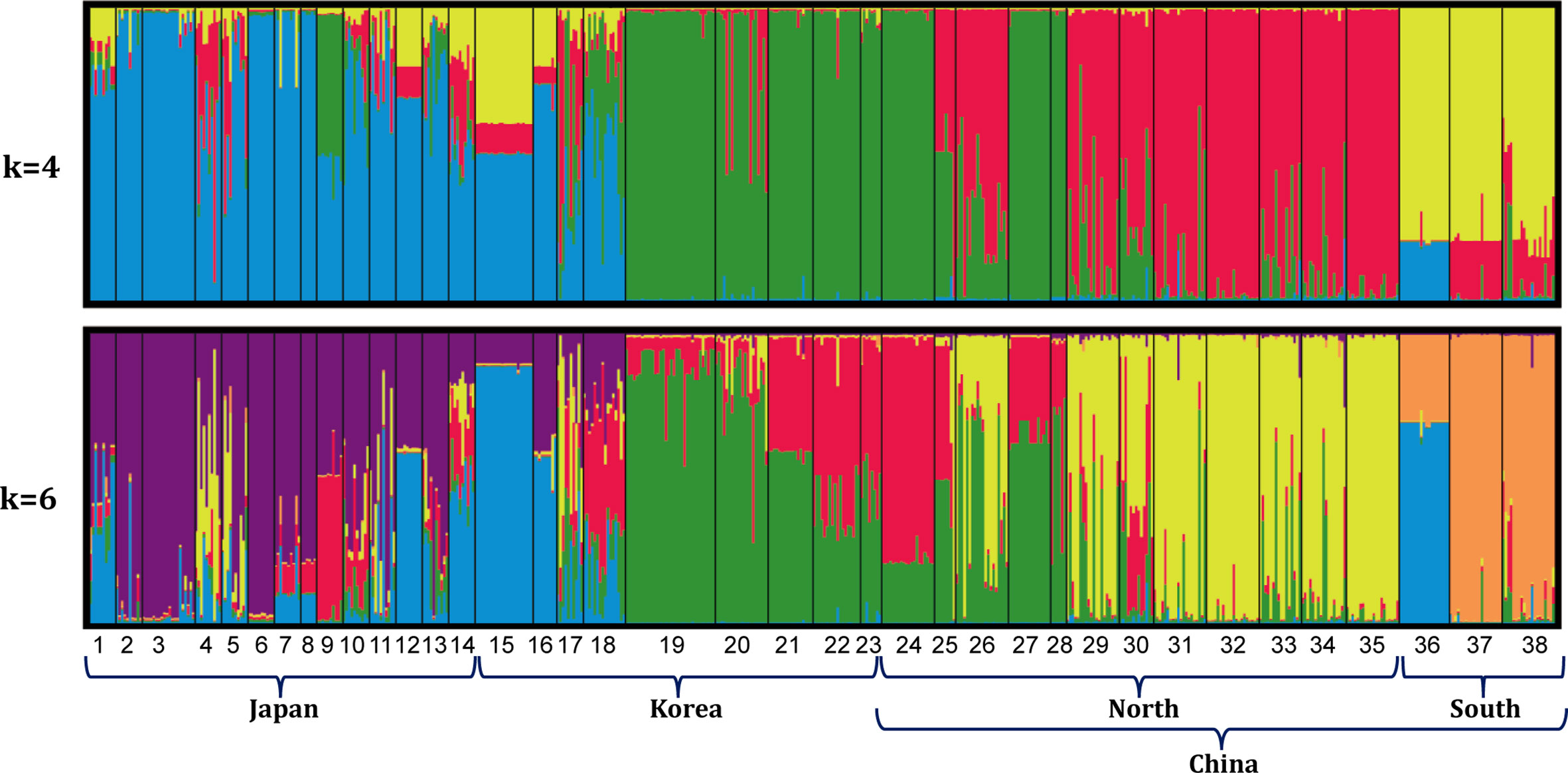
Figure 2 Genetic grouping of 38 G. vermiculophylla populations in the northwest Pacific based on STRUCTURE analysis (K = 4 and K = 6).
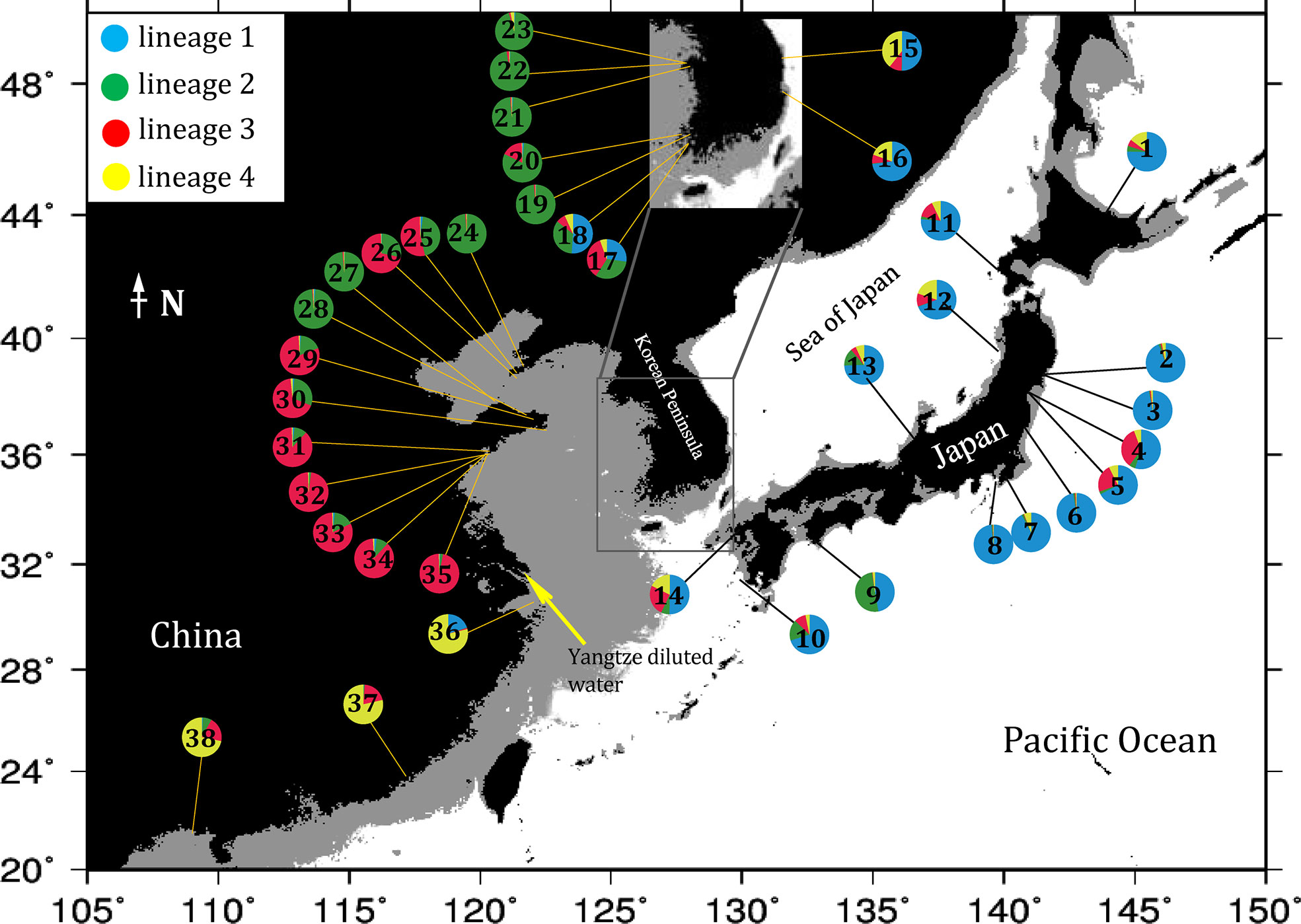
Figure 3 Geographical distribution of genetic lineages of G. vermiculophylla in the northwest Pacific identified by microsatellite genotyping. Numbers in pie charts correspond to sampling localities in Table 1. Colors corresponds to the lineages indicated in the structuring analysis (K = 4). The black areas represent the elevation >0 m, the gray areas represent the depth of water <130 m, and the light blue areas represent the depth of water >130 m (sea levels during the LGM).
Temperature trends
SST increased throughout the entire northwest Pacific from 1978 to 2018 (Figure 1). SST increased at the highest rate of 0.3–0.4°C decade-1 in the northern Sea of Japan and the Japan-Pacific coast, corresponding to a SST increase of 1.2–1.6°C over the past 40-year period. In comparison, the SST warming rate was approximately 0.15°C per decade in the Yellow-Bohai Sea, 0.25–0.3°C per decade in the East China Sea, and. 0.15–0.2°C per decade in the South China Sea (Figure 1). In general, higher latitudes are warming faster than lower latitudes (Figure 1).
Environmental variables determining habitat suitability
Of all models built with only uncorrelated variables that contributed with >5% to the model gain, the model of lowest AICc (2206) was built with a betamultiplier of 3 (Figure S2A) and three predictor variables: mean sea surface temperature (SST), mean sea ice thickness, and minimum nitrate concentration, with model contributions of 61.7%, 28.5%, and 9.8%, respectively (Table S5). Habitat suitability was predicted to be highest from c. 15°C to 23°C mean SST (Figure S3A), and in absence of sea ice (Figure S3B) and nitrate (Figure S3C).
The average AUC.Test value of 0.806 (Figure S2B; Table S5) suggests that the present-day model could discriminate well between presence and absence sites. The low AUC.Diff value of 0.02 (Figure S2C; Table S5) suggests that the model was not overfitting to the occurrence locations and, thus, suitable to be transferred to past and future climate conditions (Warren and Seifert, 2011).
Habitat suitability projections
The habitat suitability projected for present-day conditions (Figure 4) agreed well with the occurrence records of the species (Figure S1). While the species’ southern distribution limit currently stretches no further south than northern Vietnam, cooler water temperatures during the LGM may have provided suitable conditions to the southern tip of Vietnam and to the Philippines (Figure 4A). All emission scenarios, however, predicted the species disappearance within this century from regions south of Taiwan (Figure 5). The species’ northern distribution limit shifted northwards from southern shores of Hokkaido during the LGM to northern shores of Hokkaido in the Mid Holocene (Figures 4A, B). Within this century, rising temperatures and melting sea ice will likely open up suitable habitat in the north along the coast of the Kamchatka Peninsula (all emission scenarios), and in the entire Okhotsk Sea (RCP 4.5, RCP 6.0, and RCP 8.5) (Figure 5). In the southern range limit, habitat suitability for the contemporary observed ‘brown’ lineage in G. vermiculophylla will largely contract or potentially disappear, particularly by the year 2100 under RCP85 (Figure 5).
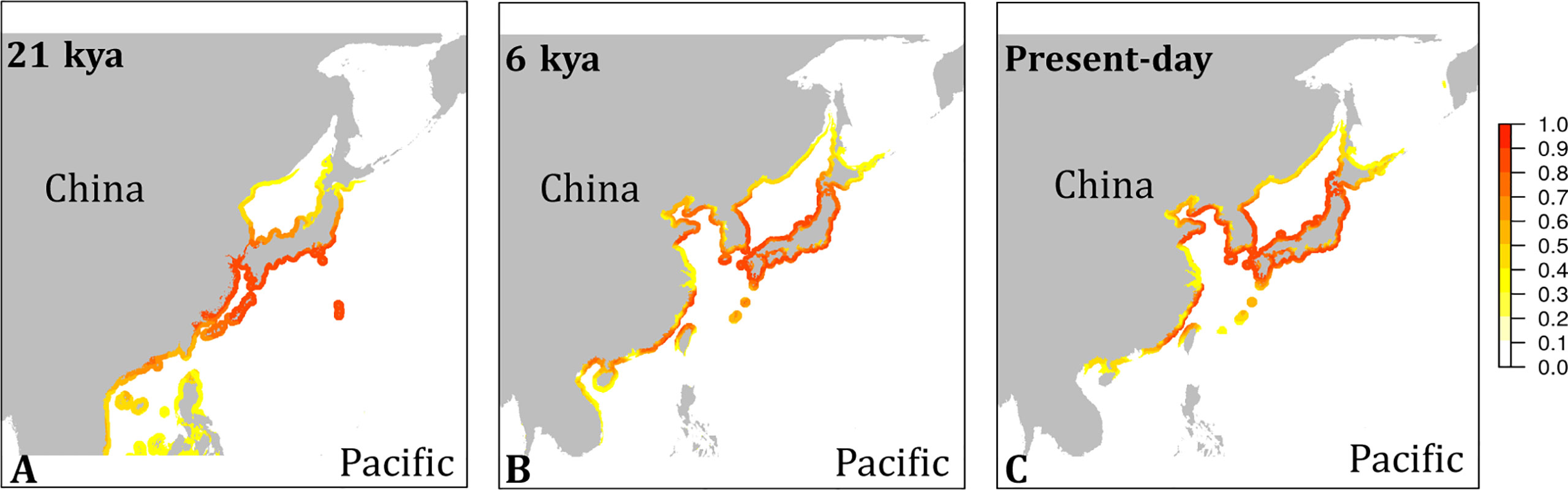
Figure 4 Niche model projections of G. vermiculophylla in the northwest Pacific. Habitat suitability in cloglog representation at the last glacial maximum (LGM, 21 kya) (A), the Mid-Holocene (6 kya) (B), and present time (C).
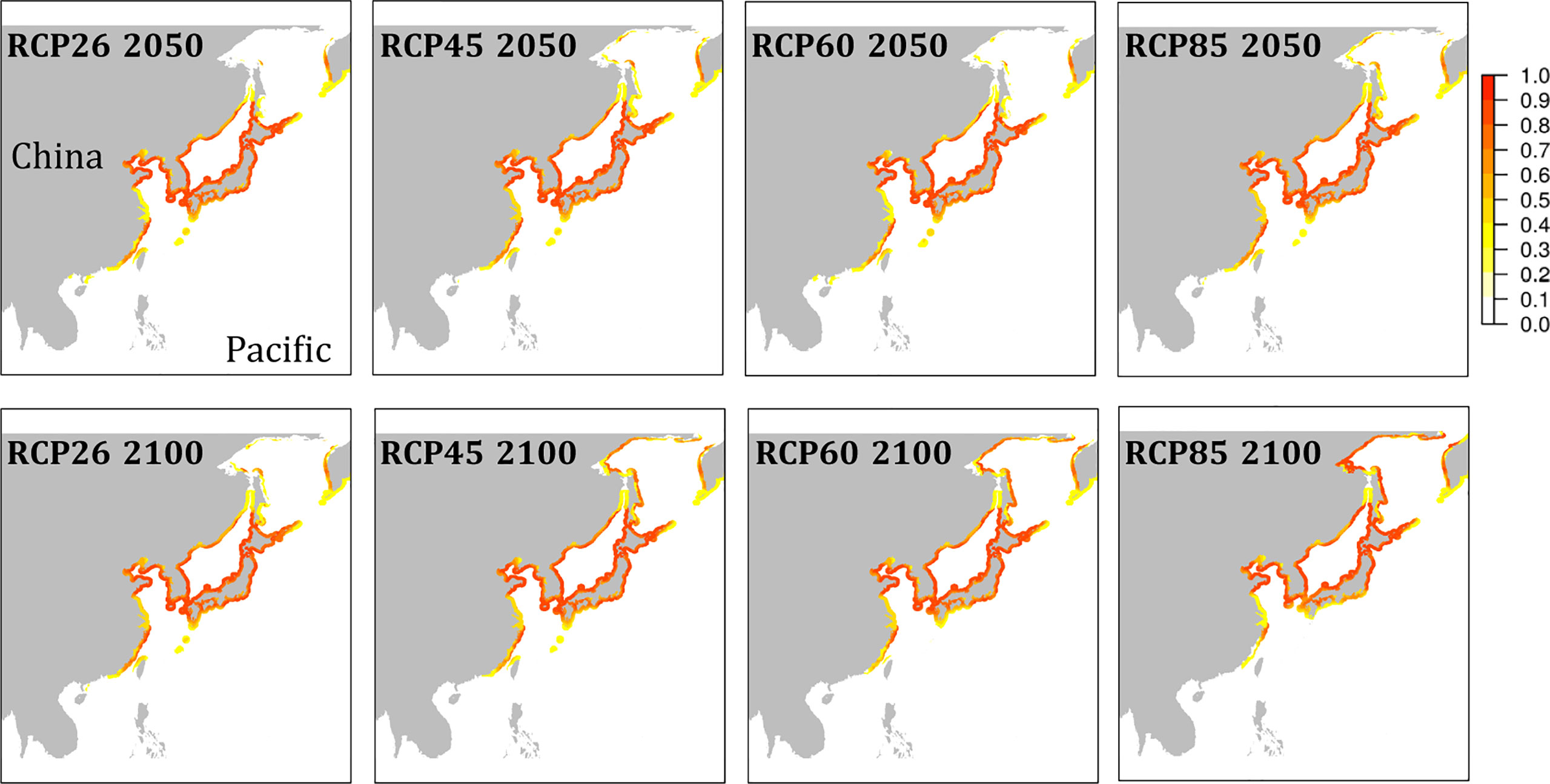
Figure 5 Niche model projections of G. vermiculophylla in the northwest Pacific. Future habitat suitability by the year 2050 (upper) and by the year 2100 (lower) in cloglog representation under different emission scenarios (RCP26, RCP45, RCP60, and RCP85).
Discussion
Climate warming can alter the distribution ranges of intertidal seaweeds (Hoek, 1982; Lima et al., 2007; Wernberg et al., 2011; Jueterbock et al., 2013; Assis et al., 2017). In this study, the analysis of SST patterns off the northwest Pacific coast from 1978 to 2018 revealed a clear warming trend, particularly in the Sea of Japan, the Yellow-Bohai and the East China Seas (Figure 1). Under future climate warming, G. vermiculophylla is likely to expand its northern range limit poleward and to lose a unique genetic lineage at its southern range edge, despite the presence of coastal upwelling waters and several late-Pleistocene glacial refugia in the northern South China Sea (Hu et al., 2011; Wang et al., 2012; Hu et al., 2018b).
The SST variability in the northwest Pacific is linked to large-scale climate variability. Herein, SST increased significantly in the northwest Pacific between 1978 and 2018. In accordance, Pei et al. (2017) found an increasing SST trend at a hydrographic section transecting the Yellow and East China Seas. Importantly, the increasing rate of SST, which decreased with water depth, leads to increased vertical stratification and decreased vertical mixing, and hence largely contributes to the warming of the Yellow and East China Seas (Pei et al., 2017). In addition, the warming rate reported here also matches the increase in SST observed in other marine provinces and ecoregions over the past decades (Lima and Wethey, 2012). SDM projections, in situ field observations and empirical evidence congruently show that significant changes in distribution ranges of intertidal seaweeds have occurred worldwide from the northeast Atlantic (Lima et al., 2007; Nicastro et al., 2013; Jueterbock et al., 2013; Neiva et al., 2015; Lourenco et al., 2016; Assis et al., 2017), to the Arctic (Jueterbock et al., 2016), to the northwest Pacific (Tanaka et al., 2012; Song et al., 2021) and the sub-Antarctic (Murcia et al., 2020). Our study is thus consistent with these previous findings, showing that coastal seaweed beds worldwide, including the northwest Pacific, are facing biodiversity loss and range shifts driven by ongoing and future climate change.
Factors in coastal marine environments such as SST and nitrate concentrations are usually tightly correlated (Ayers and Strutton, 2013). For example, population persistence and recruitment success of the kelps usually decrease with rising SST, as with low levels of nutrient availability (Muth et al., 2019). Ocean warming can enhance the stratification of the upper mixed layer of coastal seawaters (Behrenfeld et al., 2006), and oceanographic processes (i.e. the intensity and frequency of upwelling events) can affect dynamic nutrient availability that is indispensable for the productivity of seaweeds (Lobban and Harrison, 2012). In this study, poleward range expansion of G. vermiculophylla seems to be induced mainly by temperature, but nutrient availability is also a necessary element for its metabolism and optimal physiological performance (Gordillo, 2012; Franco et al., 2018). Mean SST has been projected as a direct range-limiting factor for G. vermiculophylla, followed by mean ice thickness and minimum nitrate (Table S5), implying that increasing SST and depleted nutrient likely limit spore recruitment, development, growth, reproduction and survival of this kind of habitat-forming seaweed (Flukes et al., 2015; Muth et al., 2019; Williamson et al., 2019). This, in turn, suggests that G. vermiculophylla has potentially higher physio-evolutionary adaption in response to ocean warming, and accordingly high efficiency of nutrient metabolism, than other marine macroalgae (Murcia et al., 2020).
Intertidal sessile seaweeds are generally sensitive and susceptible to climate-driven environmental changes because they are usually characterized by a short life-span and often live close to their physiological tolerance limits. While G. vermiculophylla is eurythermal and can tolerate a wide range of temperature from 5°C to 30°C in field (i.e. Shikoku Island, Japan), the optimum temperature for the growth of the tetrasporophyte and gametophyte is 15–25°C and 20-30°C, respectively (Yokoya et al., 1999). The SST increase of 0.2–0.4°C/decade during the past 4 decades in the northwest Pacific (Figure 1) is not lethal for G. vermiculophylla (van den Hoek, 1982; Davison and Pearson, 1996), but by the year 2050 or 2100 SST is expected to be high enough to act as a limiting factor by imposing non-lethal effects and increasing the susceptibility to diseases, or indirectly setting limits on distribution by determining the outcome of biotic interactions (Sanford, 2002). When the non-lethal temperature increase meets photoperiod requirement (day length and number of cycles), it can potentially advance the timing of reproductive events of G. vermiculophylla (de Bettignies et al., 2018). This effect can be amplified where critical day lengths are modified by temperature, and may eventually lead to reproduction failure, growth cessation, and potential death.
The faster warming rates of SST in higher latitudes than those in lower latitudes observed in the northwest Pacific (Figure 1) are in accordance with the reports of the Intergovernmental Panel on Climate Change (IPCC, 2018). In this study, the northern range limit is projected to expand to the north of the Sea of Okhotsk (c. 58°N) (Figure 5). However, the poleward expansion of the warm-water G. vermiculophylla could be limited by the absence of suitable high temperatures during the summer (van den Hoek, 1982). The long-term SST trend from 1978 to 2018 shows a consistent increase, but it is not known whether this increase was homogeneous between the summer and winter seasons (Lima et al., 2007). A seasonal picture of SST trends may help to explain why warming SST had not hindered the northward range extension of G. vermiculophylla in summer seasons.
The contemporary southernmost distribution limit of G. vermiculophylla is at around 21°N (Table 1). SDMs showed the species’ southern distribution limit retracted 15 degrees northward since the LGM (Figure 4). By the year 2100, the southern range limit is forecasted to further contract to the southern East China Sea (c. 23°N) (Figure 5). Such sequential retraction in distribution range driven by increasing temperature since the LGM, including the recorded SST rise over the past 4 decades, suggest that a specific ‘brown’ lineage (Figure 2) is likely to disappear under projected ocean warming. Geographically, this unique ‘brown’ lineage occurring at the species’ southern rear-edge (Figure 2) is characterized by low gene flow and high genetic differentiation among populations (Table S4) likely due to a harsher environment, higher genetic drift, higher isolation and selection as compared with the species’ range center (Hampe and Petit, 2005). The Yangtze diluted water, which has been illustrated as a physical barrier for trans-regional dispersal and genetic exchange of intertidal species (Hu et al., 2018a) and proposed to move northward to c. 33°-34°N due to the changed habitat continuity and oceanographic circulation (Hu and Dong, 2022), will most likely hinder the expansion of the geographically isolated ‘brown’ lineage of G. vermiculophylla across the Yangtze River under future climate change scenarios. Yet, the capacity of G. vermiculphylla to adapt to rising temperature at the southern range limit has not been estimated empirically. In addition, phenotypic plasticity, as one of the mechanisms to help marine organisms to cope with the changing environments, has been recently documented effective for seaweeds to perform well and survive the increases in temperature (Flukes et al., 2015; Rugiu et al., 2018). It is thus noteworthy to comparatively explore eco-physiological performances and phenological traits between central and marginal populations (here refers to the ‘brown’ lineage) of G. vermiculophylla (Usandizaga et al., 2020), including energy allocation between sexual and asexual (vegetative) reproduction, and resilience to environmental extremes (e.g. temperature and nutrients) (Hu et al., 2018a).
In the case of G. vermiculophylla with a haplo-diplontic life cycle, both gametogenesis and tetrasporogenesis are controlled by the combination of temperature and day length, leading to a strict reproductive time window (Dring, 1984). The ‘brown’ lineage of G. vermiculophylla in the southern range limit is not only a genetically temperate unit for growth and survival, but also reproduction (de Bettignies et al., 2018). Indeed, temperature can overcome photoperiod thresholds as the main driver of reproduction (Molenaar et al., 1996). Temperature has been found to significantly affect tetraspore yield of species in the order Gracilariales, through temperature-dependent photosynthesis and associated vegetative reproduction and tetraspore formation (Wang et al., 2010). More importantly, the ‘brown’ lineage often occurs in sandy or muddy habitats (authors’ field observation), characterized by soft and unstable substrata that prevents normal spore (tetraspore and carpospores) settlement and germination (Simonetti et al., 1970; Guillemin et al., 2008), as compared with the rocky bottoms where other northern lineages normally occur. This characteristic habitat is an important signature of predominant vegetative reproduction of G. vermiculophylla in its distributional rear-edge (Krueger-Hadfield et al., 2016). In order to maintain a constant population size and fitness under the scenario of ongoing and future SST warming, G. vermiculophylla can likely shift its reproductive mode from a mixture of sexual and vegetative reproduction to vegetative reprodution by fragmentation of erect thalli which can recruit in soft bottom by sediment burial (Simonetti et al., 1970). However, this mainly vegetative reproduction can lead to a bottleneck effect with reduced genetic diversity in the long term (Guillemin et al., 2008).
Species’ vulnerability to anthropogenic climate change depends on the degree of climate shifts, and its sensitivity and adaptive capacity to environmental variables (Fortini and Schubert, 2017). Climate-driven distributional shifts of the commercially important and habitat-forming G. vermiculophylla can impose severe socio-ecological impacts at both local and eco-regional scales in the northwest Pacific. Relative to the species’ range center, G. vermiculophylla populations at lower latitudes dwell in habitats close to its optimum growth temperature and are likely at high risk of extinction due to its sensitivity to climate change and anthropogenic interferences (Gibson et al., 2009). However, selection-induced adaption to the local environment can result in the development of distinct ecotypes in the periphery (Castric and Bernatchez, 2003), similarly to the unique ‘brown’ lineage comprised of the three southern peripheral populations of G. vermiculophylla (Figure 2). Zhong et al. (2020) recently reported that the ancestors of G. vermiculophylla in the northwest Pacific originated from multiple areas, including the Japan-Pacific coast, East and South China Sea. This implies that the unique ‘brown’ lineage of G. vermiculophylla is particularly important in a conservation context, because the three southern peripheral populations have not contributed to postglacial recolonization of the central and northern range in the northwestern Pacific (Hampe and Petit, 2005). These southern populations with ancient private diversity, together with their functional roles as typical ecosystem engineers of coastal communities (Thomsen et al., 2013), raise the need of management and preservation under ongoing ocean warming. Ex situ conservation of germplasm banks of southern G. vermiculophylla populations in the northwest Pacific is feasible in practice (Hu et al., 2018), and this activity will retard the threat to species persistence and ecological roles, including the commercial and ecosystem services provided by G. vermiculophylla in East Asia.
Data availability statement
The datasets presented in this study can be found in online repositories. The names of the repository/repositories and accession number(s) can be found in the article/Supplementary Material.
Author contributions
Z-MH conceived the project. Y-JL performed temperature trend analysis, prepared figures and tables and dominated the writing. K-LZ conducted molecular experiments, data collection and analysis. AJ carried out SDM analysis and results interpretation. SS, H-GC, and FW provided partial samples, assisted interpretation and writing. AJ, FW, JA and Z-MH commented the results and modified the manuscript to final version. All authors contributed to the article and approved the submitted version.
Funding
This study was supported by the National Natural Science Foundation of China (31971395 and 41761144057) and the Foundation for Science and Technology (FCT) of Portugal through projects UIDB/04326/2020, UIDB/04326/2020, LA/P/0101/2020 and PTDC/BIA- CBI/6515/2020 and the transitional norm DL57/2016/CP1361/CT0035.
Acknowledgments
We thank Dr. Zhong-Min Sun and Mr. Ruo-Yu Liu at Institute of Oceanology, Chinese Academy of Sciences (IOCAS) for providing assistance in field collections. We also thank Dr. Prof. Shi-Jian Hu at IOCAS for providing guidelines about temperature trend analysis in the northwest Pacific, two anonymous reviewers and the handing editor for providing valuable comments to improve the manuscript.
Conflict of interest
The authors declare that the research was conducted in the absence of any commercial or financial relationships that could be construed as a potential conflict of interest.
Publisher’s note
All claims expressed in this article are solely those of the authors and do not necessarily represent those of their affiliated organizations, or those of the publisher, the editors and the reviewers. Any product that may be evaluated in this article, or claim that may be made by its manufacturer, is not guaranteed or endorsed by the publisher.
Supplementary material
The Supplementary Material for this article can be found online at: https://www.frontiersin.org/articles/10.3389/fmars.2022.983685/full#supplementary-material
References
Abreu M. H., Pereira R., Buschmann A. H., Sousa-Pinto I., Yarish C. (2011). Nitrogen uptake responses of Gracilaria vermiculophylla (Ohmi) papenfuss under combined and single addition of nitrate and ammonium. J. Exp. Mar. Biol. Ecol. 407, 190–199. doi: 10.1016/j.jembe.2011.06.034
Akaike H. (1974). A new look at the statistical model identification. IEEE T. Automat. Contr. 19, 716–723. doi: 10.1109/TAC.1974.1100705
Akaike S. (2000). Spatial and temporal variations of areas of”Isoyake” and macroalgal communities interpreted from color aerial photographs and SCUBA surveys along the western coast of shakotan peninsula, hokkkaido, Japan. Sci. Rep. Hokkaido Nucl. Energy Environ. Res. Center 6, 1–119.
Assis J., Araújo M. B., Serrão E. A. (2017). Projected climate changes threaten ancient refugia of kelp forests in the north Atlantic. Glob. Change Biol. 24, 1365–2486. doi: 10.1111/gcb.13818
Assis J., Serrão E. A., Duarte C. M., Fragkopoulou E., Krause-Jensen D. (2022). Major expansion of marine forests in a warmer arctic. Front. Mar. Sci. 9. doi: 10.3389/fmars.2022.850368
Assis J., Tyberghein L., Bosch S., Verbruggen H., Serrão E. A., De Clerck O. (2018). Bio-ORACLE v2.0: Extending marine data layers for bioclimatic modelling. Glob. Ecol. Biogeogr. 27, 277–284. doi: 10.1111/geb.12693
Ayers J. M., Strutton P. G. (2013). Nutrient variability in subantarctic mode waters forced by the southern annular mode and ENSO. Geophys. Res. Lett. 40, 3419–3423. doi: 10.1002/grl.50638
Behrenfeld M. J., O’Malley R. T., Siegel D. A., McClain C. R., Sarmiento J. L., Feldman G. C., et al. (2006). Climate-driven trends in contemporary ocean productivity. Nature 444, 752–755. doi: 10.1038/nature05317
Bonthond G., Bayer T., Krueger-Hadfield S. A., Barboza F. R., Nakaoka M., Valero M., et al. (2020). How do microbiota associated with an invasive seaweed vary across scales? Mol. Ecol. 29, 2094–2108. doi: 10.1111/mec.15470
Briggs J. C., Bowen B. W. (2012). A realignment of marine biogeographic provinces with particular reference to fish distributions. J. Biogeogr. 39, 12–30. doi: 10.1111/j.1365-2699.2011.02613.x
Castric V., Bernatchez L. (2003). The rise and fall of isolation by distance in the anadromous brook charr (Salvelinus fontinalis mitchill). Genetics 163, 983–996. doi: 10.1093/genetics/163.3.983
Collins M., Knutti R., Arblaster J., Dufresne J. L., Fichefet T., Friedlingstein P., et al. (2013). Long-term climate change, projections, commitments and irreversibility in Climate Change 2013, The Physical Science Basis. Contribution of Working Group I to the Fifth Assessment Report of the Intergovernmental Panel on Climate Change, eds Stocker T. F., Qin D., Plattner G.-K., Tignor M., Allen S. K., Boschung J., et al (New York, NY: Cambridge University Press) 1029–1136.
Davison I. R., Pearson G. A. (1996). Stress tolerance in intertidal seaweeds. J. Phycol. 32, 197–211. doi: 10.1111/j.0022-3646.1996.00197.x
de Bettignies T., Wernberg T., Guigel F. D. (2018). Exploring the influence of temperature on aspects of the reproductive phenology of temperate seaweeds. Front. Mar. Sci. 5. doi: 10.3389/fmars.2018.00218
Ding L. P., Huang B. X., Xie Y. Q. (2011). Advances and problems with the study of marine macroalgae of China seas. Biodiv. Sci. 19, 798–804. doi: 10.3724/SP.J.1003.2011.07128
Earl D. A., Vonholdt B. M. (2012). STRUCTURE HARVESTER: a website and program for visualizing STRUCTURE output and implementing the evanno method. Conserv. Genet. Resour. 4, 359–361. doi: 10.1007/s12686-011-9548-7
Engel C. R., Wattier R., Destombe C., Valero M. (1999). Performance of non-motile male gametes in the sea: analysis of paternity and fertilization success in a natural population of a red seaweed, Gracilaria gracilis. Proc. R. Soc Lond. B. 266, 1879–1886. doi: 10.1098/rspb.1999.0861
Evanno G., Regnaut S., Goudet J. (2005). Detecting the number of clusters of individuals using the software structure: A simulation study. Mol. Ecol. 14, 2611–2620. doi: 10.1111/j.1365-294X.2005.02553.x
Excoffier L., Lischer H. E. L. (2010). Arlequin 3.5: A new series of programs to perform population genetics analyses under Linux and windows. Mol. Ecol. Res. 10, 564–567. doi: 10.1111/j.1755-0988.2010.02847.x
Fielding A. H., Bell J. F. (1997). A review of methods for the assessment of prediction errors in conservation presence/absence models. Environ. Conserv. 24, 38–49. doi: 10.1017/S0376892997000088
Fithian W., Elith J., Hastie T., Keith D. A. (2015). Bias correction in species distribution models: Pooling survey and collection data for multiple species. Methods Ecol. Evol. 6, 424–438. doi: 10.1111/2041-210X.12242
Flukes E. B., Wright J. T., Johnson C. R. (2015). Phenotypic plasticity and biogeographic variation in physiology of habitat-forming seaweed: response to temperature and nitrate. J. Phycol. 51, 896–909. doi: 10.1111/jpy.12330
Fortini L., Schubert O. (2017). Beyond exposure, sensitivity and adaptive capacity: a response based ecological framework to assess species climate change vulnerability. Clim. Change Res. 4, 2. doi: 10.1186/s40665-017-0030-y
Fragkopoulou E., Serrão E. A., De Clerck O., Costello M. J., Araújo M. B., Duarte C. M., et al. (2022). Global biodiversity patterns of marine forests of brown macroalgae. Glob. Ecol. Biogeogr. 31, 636–648. doi: 10.1111/geb.13450
Franco J. N., Tuya F., Bertocci I., Rodriguez L., Martinez B., Sousa-Pinto I., et al. (2018). The ‘golden kelp’ Laminaria ochroleuca under global change: Integrating multiple eco-physiological responses with species distribution models. J. Ecol. 106, 47–58. doi: 10.1111/1365-2745.12810
Gibson S. Y., van der Marel R. C., Starzomski B. M. (2009). Climate change and conservation of leading-edge peripheral populations. Conserv. Biol. 23, 1369–1373. doi: 10.1111/j.1523-1739.2009.01375.x
Gordillo F. J. L. (2012). “Environment and algal nutrition,” in Seaweed biology. Eds. Wiencke C., Bischof K. (Berlin: Springer), 471–493.
Goudet J. (1995). FSTAT (version 1.2): a computer program to calculate f-statistics. J. Hered. 86, 485–486. doi: 10.1093/oxfordjournals.jhered.a111627
Guillemin M. L., Faugeron S., Destombe C., Viard F., Correa J. A., Valero M. (2008). Genetic variation in wild and cultivated populations of the haploid-diploid red alga gracilaria chilensis: how farming practices favor asexual reproduction and heterozygosity. Evolution 62, 1500–1519. doi: 10.1111/j.1558-5646.2008.00373.x
Hammann M., Wang G. G., Boo S. M., Aguilar-Rosas L. E., Weinberger F. (2016). Selection of heat-shock resistance traits during the invasion of the seaweed Gracilaria vermiculophylla. Mar. Biol. 163, 104. doi: 10.1007/s00227-016-2881-3
Hampe A., Petit R. J. (2005). Conserving biodiversity under climate change: the rear edge matters. Ecol. Lett. 8, 461–467. doi: 10.1111/j.1461-0248.2005.00739.x
Hijmans R. J., van Etten J., Mattiuzzi M., Sumner M., Greenberg J. A., Lamigueiro O. P., et al. (2011). Raster: raster: Geographic data analysis and modeling. r package, version: 2.0, Vienna.
Hoek C. V. D. (1982). The distribution of benthic marine algae in relation to the temperature regulation of their life histories. Biol. J. Linn. Soc 18, 81–144. doi: 10.1111/j.1095-8312.1982.tb02035.x
Hu L. S., Dong Y. W. (2022). Northward shift of a biogeographical barrier on china’s coast. Diversity Distrib. 28 (2), 318–330. doi: 10.1111/ddi.13463
Hu Z. M., Liu R. Y., Zhang J., Duan D. L., Wang G. G., Li W. H. (2018a). A unique genetic lineage at the southern coast of China in the agar-producing Gracilaria vermiculophylla (Gracilariales. florideophyceae). Algae 33, 269–278. doi: 10.4490/algae.2018.33.8.30
Hu Z. M., Kantachumpoo A., Liu R. Y., Sun Z. M., Yao J. T., Komatsu T., et al. (2018b). A late pleistocene marine glacial refugium in the south-west of hainan island, China: Phylogeographical insights from the brown alga Sargassum polycystum. J. Biogeogr. 45, 355–366. doi: 10.1111/jbi.13130
Hu Z. M., Lopez-Bautista J. (2014). Adaptation mechanisms and ecological consequences of seaweed invasions: a review case of agarophyte Gracilaria vermiculophylla. Biol. Invas. 16, 967–976. doi: 10.1007/s10530-013-0558-0
Hu Z. M., Shan T. F., Zhang J., Zhang Q. S., Critchley A. T., Choi H. G., et al. (2021). Kelp aquaculture in China: a retrospective and future prospects. Rev. Aquacult. 13, 1324–1351. doi: 10.1111/raq.12524
Hu Z. M., Uwai S., Yu S. H., Komatsu T., Ajisaka T., Duan D. L. (2011). Phylogeographic heterogeneity of the brown macroalga Sargassum horneri (Fucaceae) in the northwestern pacific in relation to late pleistocene glaciation and tectonic configurations. Mol. Ecol. 20, 3894–3909. doi: 10.1111/j.1365-294X.2011.05220.x
Hu Z. M., Zeng X. Q., Wang A. H., Shi C. J., Duan D. L. (2004). An efficient method for DNA isolation from red algae. J. Appl. Phycol. 16, 161–166. doi: 10.1023/B:JAPH.0000048456.26639.1a
Hutchins D. A., Fu F. X. (2017). Microorganisms and ocean global change. Nat. Microbiol. 2, 17058. doi: 10.1038/nmicrobiol.2017.58
Hwang E. K., Yotsukura N., Pang S. J., Su L., Shan T. F. (2019). Seaweed breeding programs and progress in eastern Asian countries. Phycologia 58, 484–495. doi: 10.1080/00318884.2019.1639436
IPCC (2018). Global warming of 1.5°C. an IPCC special report on the impacts of global warming of 1.5°C above pre-industrial levels and related global greenhouse gas emission pathways, in the context of strengthening the global response to the threat of climate change, sustainable development, and efforts to eradicate poverty. Eds. Masson-Delmotte V. P., Zhai H.-O., Pörtner D., Roberts J., Skea P. R., Shukla A., et al. (Geneva, Switzerland: World Meteorological Organization).
Jakobsson M., Rosenberg N. A. (2007). CLUMPP: A cluster matching and permutation program for dealing with label switching and multimodality in analysis of population structure. Bioinformatics 23, 1801–1806. doi: 10.1093/bioinformatics/btm233
Japan Meteorological Agency (2018) Home page. Available at: https://www.jma.go.jp/jma/indexe.html.
Jiménez-Valverde A. (2012). Insights into the area under the receiver operating characteristic curve (AUC) as a discrimination measure in species distribution modelling. Glob. Ecol. Biogeogr. 21, 498–507. doi: 10.1111/j.1466-8238.2011.00683.x
Johnston C. A., Lipcius R. N. (2012). Exotic macroalga Gracilaria vermiculophylla provides superior nursery habitat for native blue crab in Chesapeake bay. Mar. Ecol. Prog. Ser. 467, 137–146. doi: 10.3354/meps09935
Jueterbock A., Smolina I., Coyer J. A., Hoarau G. (2016). The fate of the Arctic seaweed Fucus distichus under climate change: An ecological niche modeling approach. Ecol. Evol. 6, 1712–1724. doi: 10.1002/ece3.2001
Jueterbock A., Tyberghein L., Verbruggen H., Coyer J. A., Olsen J. L., Hoarau G. (2013). Climate change impact on seaweed meadow distribution in the north Atlantic rocky intertidal. Ecol. Evol. 3, 1356–1373. doi: 10.1002/ece3.541
Keith S. A., Kerswell A. P., Connolly S. R. (2014). Global diversity of marine macroalgae: environmental conditions explain less variation in the tropics. Global. Ecol. Biogeogr. 23, 517–529. doi: 10.1111/geb.12132
Kim H. S., Boo S. M., Lee I. K., Sohn C. H. (2013). National list of species of Korea “Marine algae” (Incheon: National Institute of Biological Resources Ministry of Environment Korea), 366.
Krueger-Hadfield S. A., Kollars N. M., Byers J. E., Greig T. W., Hammann M., Murray D. C., et al. (2016). Invasion of novel habitats uncouples haplo-diplontic life cycles. Mol. Ecol. 25, 3801–3816. doi: 10.1111/mec.13718
Krueger-Hadfield S. A., Kollars N. M., Strand A. E., Byers J. E., Shainker S. J., Terada R., et al. (2017). Genetic identification of source and likely vector of a widespread marine invader. Ecol. Evol. 7, 4432–4447. doi: 10.1002/ece3.3001
Lima F. P., Ribeiro P. A., Queiroz N., Hawkins S. J., Santos A. M. (2007). Do distributional shifts of northern and southern species of algae match the warming pattern? Glob. Change Biol. 13, 2592–2604. doi: 10.1111/j.1365-2486.2007.01451.x
Lima F. P., Wethey D. S. (2012). Three decades of high-resolution coastal sea surface temperatures reveal more than warming. Nat. Commun. 3, 704. doi: 10.1038/ncomms1713
Lobban C. S., Harrison P. J. (2012). Seaweed ecology and physiology (UK: Cambridge University Press).
Lourenco C. R., Zardi G. I., McQuaid C. D., Serrão E. A., Pearson G. A., Jacinto R., et al. (2016). Upwelling areas as climate change refugia for the distribution and genetic diversity of a marine macroalga. J. Biogeogr. 43, 1595–1607. doi: 10.1111/jbi.12744
Lyra G. M., Iha C., Grassa C. J., Cai L. M., Zhang H. R., Lane C., et al. (2021). Phylogenomics, divergence time estimation and trait evolution provide a new look into the gracilariales (Rhodophyta). Mol. Phylogenet. Evol. 165, 107294. doi: 10.1016/j.ympev.2021.107294
Martínez B., Viejo R. M., Carreñno F., Aranda S. C. (2012). Habitat distribution models for intertidal seaweeds: responses to climatic and non-climatic drivers. J. Biogeogr. 39, 1877–1890. doi: 10.1111/j.1365-2699.2012.02741.x
Molenaar F. J., Breeman A. M., Venekamp L. A. H. (1996). Ecotypic variation in Cystoclonium purpureum (Rhodophyta) synchronizes life history events in different regions. J. Phycol. 32, 516–525. doi: 10.1111/j.0022-3646.1996.00516.x
Molinos J. G., Halpern B. S., Schoeman D. S., Brown C. J., Kiessling W., Moore P. J., et al. (2015). Climate velocity and the future global redistribution of marine biodiversity. Nat. Clim. Change 6, 83–88. doi: 10.1038/nclimate2769
Murcia S., Riul P., Mendez F., Rodriguez J. P., Rosenfeld S., Ojeda J., et al. (2020). Predicting distributional shifts of commercially important seaweed species in the subantarctic tip of south America under future environmental changes. J. Appl. Phycol. 32, 2105–2114. doi: 10.1007/s10811-020-02084-6
Muth A. F., Graham M. H., Lane C. E., Harley C. D. (2019). Recruitment tolerance to increased temperature present across multiple kelp clades. Ecology 100, e02594. doi: 10.1002/ecy.2594
Neiva J., Assis J., Coelho N. C., Fernandes F., Pearson G. A., Serrão E. A. (2015). Genes left behind: climate change threatens cryptic genetic diversity in the canopy-forming seaweed Bifurcaria bifurcata. PloS One 10, e0131530. doi: 10.1371/journal.pone.0131530
Nicastro K. R., Zardi G. I., Teixeira S., Neiva J., Serrão E. A., Pearson G. A. (2013). Shift happens: trailing edge contraction associated with recent warming trends threatens a distinct genetic lineage in the marine macroalga Fucus vesiculosus. BMC Biol. 11, 6. doi: 10.1186/1741-7007-11-6
Peakall R., Smouse P. E. (2006). GENALEX 6: Genetic analysis in excel. population genetic software for teaching and research. Mol. Ecol. Notes 6, 288–295. doi: 10.1111/j.1471-8286.2005.01155.x
Pei Y. H., Liu X. H., He H. L. (2017). Interpreting the sea surface temperature warming trend in the yellow Sea and East China Sea. Sci. China Earth. Sci. 160, 1558–1568. doi: 10.1007/s11430-017-9054-5
Philipps S. J., Dudík M. (2009). Modeling of species distributions with maxent: new extentions and a comprehensive evaluation. Ecography 31, 161–175. doi: 10.1111/j.0906-7590.2008.5203.x
Phillips S. J., Anderson R. P., Dudík M., Schapire R. E., Blair M. E. (2017). Opening the black box: an open-source release of maxent. Ecography 40, 887–893. doi: 10.1111/ecog.03049
Phillips S. J., Dudík M., Elith J., Graham C. H., Lehmann A., Leathwick J., et al. (2009). Sample selection bias and presence-only distribution models: Implications for background and pseudo-absence data. Ecol. Appl. 19, 181–197. doi: 10.1890/07-2153.1
Phillips S. J., Dudík M., Schapire R. E. (2004). A maximum entropy approach to species distribution modeling. Proceedings of the twenty-first international conference on Machine learning. Banff, 4-8 July 2004, 655–662.
Piñeiro-Corbeira C., Barreiro R., Cremades J., Arenas F. (2018). Seaweed assemblages under a climate change scenario: Functional responses to temperature of eight intertidal seaweeds match recent abundance shifts. Sci. Rep. 8, 12978. doi: 10.1038/s41598-018-31357-x
Pritchard J. K., Stephens M., Donnelly P. (2000). Inference of population structure using multilocus genotype data. Genetics 155, 945–959. doi: 10.1093/genetics/155.2.945
Raymond M., Rousset F. (1995). GENEPOP (version 3.1): Population genetics software for exact tests and ecumenism. J. Hered. 86, 248–249. doi: 10.1093/oxfordjournals.jhered.a111573
Rosenberg N. A. (2004). DISTRUCT: a program for the graphical display of population structure. Mol. Ecol. Notes 4, 137–138. doi: 10.1046/j.1471-8286.2003.00566.x
Rousset F. (2008). Genepop´007: a complete re-implementation of the genepop software for windows and Linux. Mol. Ecol. Res. 8, 103–106. doi: 10.1111/j.1471-8286.2007.01931.x
Rugiu L., Manninen I., Rothäusler E., Jormalainen V. (2018). Tolerance to climate change of the clonally reproducing endemic Baltic seaweed, Fucus radicans: is phenotypic plasticity enough? J. Phycol. 54, 888–898. doi: 10.1111/jpy.12796
Saha M., Wiese J., Weinberger F., Wahl M. (2016). Rapid adaptation to controlling new microbial epibionts in the invaded range promotes invasiveness of an exotic seaweed. J. Ecol. 104, 969–978. doi: 10.1111/1365-2745.12590
Sanford E. (2002). Water temperature, predation, and the neglected role of physiological rate effects in rocky intertidal communities. Integr. Comp. Biol. 42, 881–891. doi: 10.1093/icb/42.4.881
Sbrocco E. (2014). Paleo-MARSPEC: gridded ocean climate layers for the mid- Holocene and last glacial maximum. Ecology 95, 2014. doi: 10.1890/14-0443.1
Simonetti G., Giaccone G., Pignatti. S. (1970). The seaweed Gracilaria confervoides, an important object for autecologic and cultivation research in northern Adriatic Sea. Helgoländer Wiss. Meeresunters 20, 89–96. doi: 10.1007/BF01609890
Song X. H., Assis J., Zhang J., Gao X., Choi H. G., Duan D. L., et al. (2021). Climate-induced range shifts shaped the present and threaten the future genetic variability of a marine brown alga in the Northwest pacific. Evol. Appl. 14, 1867–1879. doi: 10.1111/eva.13247
Tanaka K., Taino S., Haraguchi H., Prendergast G., Hiraoka M. (2012). Warming off southwestern Japan linked to distributional shifts of subtidal canopy-forming seaweeds. Ecol. Evol. 2, 2854–2865. doi: 10.1002/ece3.391
Thomsen M. S., Stæhr P. A., Nejrup L., Schiel D. R. (2013). Effects of the invasive macroalgae Gracilaria vermiculophylla on two co-occurring foundation species and associated invertebrates. Aquat. Invas. 8, 133–145. doi: 10.3391/ai.2013.8.2.02
Toonen R. J., Hughes S. (2001). Increased throughput for fragment analysis on an ABI PRISM (R) automated sequencer using a membrane comb and STRand software. BioTechniques 31, 1320–1324.
Usandizaga S., Buschmann A. H., Camus C., Kappes J. L., Arnaud-Haond S., Mauger S., et al. (2020). Better off alone? compared performance of monoclonal and polyclonal stands of a cultivated red alga growth. Evol. Appl. 13, 905–917. doi: 10.1111/eva.12908
van Oosterhout C., Hutchinson W. F., Wills D. P., Shipley P. (2004). MICRO-CHECKER: Software for identifying and correcting genotyping errors in microsatellite data. Mol. Ecol. Notes 4, 535–538. doi: 10.1111/j.1471-8286.2004.00684.x
Wand M., Ripley B. KernSmooth. In Wand M. P., Jones M. C.. (1995). Kernel Smoothing, London, Chapman & Hall.
Wang Z. Y., Wang G. C., Niu J. F., Wang W. J., Peng G. (2010). Optimization of conditions for tetraspore release and assessment of photosynthetic activities for different generation branches of Gracilaria lemaneiformis bory. Chin. J. Oceanol. Limnol. 28, 738–748. doi: 10.1007/s00343-010-9908-2
Wang S. S., Wang G. G., Weinberger F., Bian D. P., Nakaoka M., Lenz M. (2017). Anti-epiphyte defences in the red seaweed Gracilaria vermiculophylla: non-native algae are better defended than their native conspecifics. J. Ecol. 105, 445–457. doi: 10.1111/1365-2745.12694
Wang D. X., Zhuang W., Xie S. P., Hu J. Y., Shu Y. Q., Wu R. S. (2012). Coastal upwelling in summer 2000 in the northeastern south China Sea. J. Geophy. Res. 117. doi: 10.1029/2011JC007465
Warren D. L., Seifert S. N. (2011). Ecological niche modeling in maxent: The importance of model complexity and the performance of model selection criteria. Ecol. Appl. 21, 335–342. doi: 10.1890/10-1171.1
Warren D. L., Wright A. N., Seifert S. N., Shaffer H. B. (2014). Incorporating model complexity and spatial sampling bias into ecological niche models of climate change risks faced by 90 California vertebrate species of concern. Divers. Distrib. 20, 334–343. doi: 10.1111/ddi.12160
Wernberg T., Russell B. D., Moore P. J., Ling S. D., Smale D. A., Campbell A., et al. (2011). Impacts of climate change in a global hotspot for temperate marine biodiversity and ocean warming. J. Exp. Mar. Biol. Ecol. 400, 7–16. doi: 10.1016/j.jembe.2011.02.021
Williamson C. E., Neale P. J., Hylander S., Rose K. C., Figueroa F. L., Robinson S. A., et al. (2019). The interactive effects of stratospheric ozone depletion, UV radiation, and climate change on aquatic ecosystems. Photochem. Photobiol. Sci. 18, 717–746. doi: 10.1039/c8pp90062k
Yeh F. C., Yang R. C., Boile T. (1999). POPGENE version 1.31 Microsoft window-based freeware for population genetic analysis. Edmonton, AB Canada.
Yokoya N. S., Kakita H., Obika H., Kitamura T. (1999). Effects of environmental factors and plant growth regulators on growth of the red alga Gracilaria vermiculophylla from Shikoku island, Japan. Hydrobiologia 398, 339–347. doi: 10.1023/A:1017072508583
Yoshida T., Suzuki M., Yoshinaga K. (2015). Checklist of marine algae of Japan (Revised in 2015). Jpn. J. Phycol. (Sôrui) 63, 129–189.
Zardi G. I., Nicastro K. R., Serrão E. A., Jacinto R., Monteiro C. A., Pearson G. A. (2015). Closer to the rear edge: ecology and genetic diversity down the core-edge gradient of a marine macroalga. Ecosphere 6, 1–25. doi: 10.1890/ES14-00460.1
Keywords: biodiversity conservation, climate change, microsatellite genotyping, peripheral populations, range limits, genetic lineage loss
Citation: Liu Y-J, Zhong K-L, Jueterbock A, Satoshi S, Choi H-G, Weinberger F, Assis J and Hu Z-M (2022) The invasive alga Gracilaria vermiculophylla in the native northwest Pacific under ocean warming: Southern genetic consequence and northern range expansion. Front. Mar. Sci. 9:983685. doi: 10.3389/fmars.2022.983685
Received: 01 July 2022; Accepted: 06 September 2022;
Published: 26 September 2022.
Edited by:
Didier Alain Jollivet, Centre National de la Recherche Scientifique (CNRS), FranceReviewed by:
Alejandro H. Buschmann, University of Los Lagos, ChileLee Wei-Kang, Codon Genomics, Malaysia
Copyright © 2022 Liu, Zhong, Jueterbock, Satoshi, Choi, Weinberger, Assis and Hu. This is an open-access article distributed under the terms of the Creative Commons Attribution License (CC BY). The use, distribution or reproduction in other forums is permitted, provided the original author(s) and the copyright owner(s) are credited and that the original publication in this journal is cited, in accordance with accepted academic practice. No use, distribution or reproduction is permitted which does not comply with these terms.
*Correspondence: Zi-Min Hu, aHV6aW1pbjk3MTJAMTYzLmNvbQ==; aHV6bUB5dHUuZWR1LmNu
†These authors have contributed equally to this work