- 1Ocean Biogeosciences, National Oceanography Centre, Southampton, United Kingdom
- 2Ocean and Earth Science, University of Southampton, Southampton, United Kingdom
- 3Marine Systems Modelling, National Oceanography Centre, Southampton, United Kingdom
- 4Environmental and Biochemical Sciences, The James Hutton Institute, Aberdeen, United Kingdom
- 5Scottish Association for Marine Science, Oban, United Kingdom
- 6Observatoire des Sciences de l'Univers, Pythéas Institute, OSU, Marseille, France
- 7Faculté des sciences, Aix-Marseille Université, OSU, Marseille, France
Calanoid copepods comprise around 90% of Arctic zooplankton biomass and are fundamental to the ecological and biogeochemical functioning of high-latitude pelagic ecosystems. They accumulate lipid reserves during the productive months and represent an energy-rich food source for higher trophic levels. Rapidly changing climate in the Arctic may alter the quantity and composition of the food environment for one of the key copepod species, Calanus finmarchicus, with as yet unquantified effects on its production. Here we present rates of feeding and egg production in female C. finmarchicus exposed to the range of feeding conditions encountered across the Fram Strait in May/June 2018. Carbon (C) budgets were constructed and used to examine the relationship between feeding and growth (= egg production) in these animals. C-specific ingestion rates (mean ± standard deviation) were highly variable, ranging from 0.015 ± 0.004 to 0.645 ± 0.017 day-1 (mean = 0.295 ± 0.223 day-1), and were positively correlated with food availability. C-specific egg production rates ranged from 0.00 to 0.049 day-1 (mean = 0.012 ± 0.011) and were not correlated with either food availability or ingestion rate. Calculated gross growth efficiencies (GGE: growth/ingestion) were low, 0.12 ± 0.13 (range = 0.01 to 0.39). The assembled C budgets indicate that the average fraction of ingested food that was surplus to the requirements for egg production, respiration and losses to faecal pellets was 0.17 ± 0.42. We suggest that this excess occurred, at least in part, because many of the incubated females were still undergoing the energetically (C-) expensive process of gonad maturation at the time of sampling, an assertion that is supported by the relatively high C:N (nitrogen) ratios of the incubated females, the typically low egg production rates, and gonad maturation status. Ontogenetic development may thus explain the large variability seen in the relationship between egg production and ingestion. The apparently excessive ingestion rates may additionally indicate that recently moulted females must acquire additional N via ingestion to complete the maturation process and begin spawning. Our results highlight the need for improved fundamental understanding of the physiology of high-latitude copepods and its response to environmental change.
Introduction
Copepods are among the most numerous multicellular animals in the world, dominating zooplankton biomass in the Arctic (Mauchline et al., 1998; Nöthig et al., 2015). In the Fram Strait, 70-92% of zooplankton biomass is in the subclass Copepoda (Hop et al., 2006) where the biomass is generally dominated by three key species in the genus Calanus (Hop et al., 2006; Blachowiak-Samolyk et al., 2007). These animals are important grazers of phytoplankton and represent high-quality prey for higher trophic levels (Gatten and Sargent, 1973) including the larvae of commercially important fish (Sakshaug, 2004). Calanus spp. also play an important role in ocean biogeochemistry due to their dense faecal pellets, their daily vertical migrations, and their ontogenetic migration to depth to over winter, all of which lead to sequestration of carbon in the deep ocean (Jónasdóttir et al., 2015).
The Arctic Ocean (Figure 1) is experiencing rapid, human-led change (Thomas et al., 2022). It is warming at three times the global mean rate (Dai et al., 2019; AMAP, 2021) leading to a cycle of sea ice loss, decreasing ocean albedo, increasing poleward ocean heat transport and increasing polar cloud cover (Holland and Bitz, 2003). The Fram Strait is both the main inflow and outflow gateway between the Arctic and the Atlantic and so has variable physicochemical conditions across its width. The relatively warm, salty West Spitsbergen Current is the main inflow, and the colder, fresher East Greenland Current the main outflow (Figure 1). The ice-covered East Greenland current has a low standing stock of phytoplankton dominated by flagellates, whereas chain-forming diatoms dominate further East (Gradinger and Baumann, 1991). The mixing of the two water bodies and their rapidly changing physiochemical properties affects the stocks of nutrients and organisms in the Fram Strait: the freshening, warming waters are increasing stratified, reducing nutrient cycling and allowing different organisms to thrive (Gluchowska et al., 2017; Basedow et al., 2018). Additionally, the western Fram Strait is thought the be experiencing ‘Atlantification’, the increasing influence of Atlantic water in the Arctic (Karpouzoglou et al., 2022). For one key species in the Calanus genus – Calanus finmarchicus – recent Atlantification seems to have allowed a range expansion, with them now completing their life cycles further North in the Arctic (Tarling et al., 2022). The changing physical and chemical ocean environment is expected to change the composition, distribution, timing and magnitude of primary production (Li et al., 2009; Kahru et al., 2011; Yool et al., 2015; Neukermans et al., 2018; Lewis et al., 2020) – the food on which copepods rely. With a different food environment comes the potential for changes to the productivity of Calanus spp., and in turn their population success. Understanding how Calanus spp. will respond to changing food environment is essential for predicting how the ecological and biogeochemical functioning of Arctic pelagic ecosystems will change in the future.
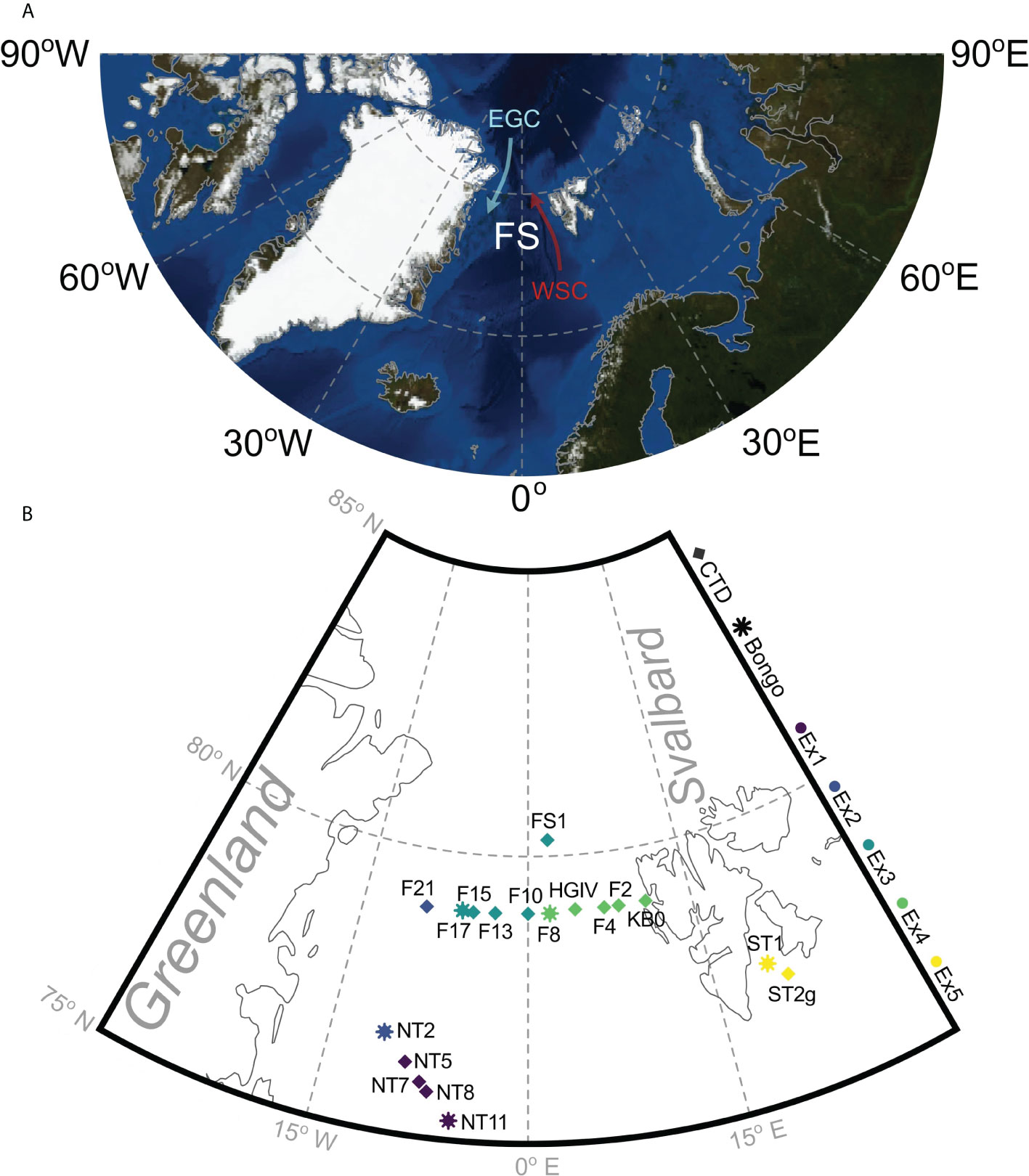
Figure 1 The location of the Fram Strait (FS) in the Arctic Ocean (A) and the stations that were sampled on cruise JR17005 in May-June 2018, showing the West Spitsbergen Current (WSC) and the East Greenland Current (EGC). Generated using Blue Marble, NASA Earth Observatory (B). The Arctic Ocean map is coloured bathymetrically showing the deep channel of the Fram Strait, where most water enters and exits the Arctic Ocean. Sequential long-term grazing experiments (1-5) are marked by Ex1-Ex5. Bongo and CTD show the locations where animals for experimental incubations and the natural plankton assemblage were collected, respectively.
Egg production in Calanus is often positively correlated with temperature (Pasternak et al., 2013) and also typically increases with food availability (Runge, 1984; Hirche and Bohrer, 1987; Hirst and Bunker, 2003; Mayor et al., 2009b). Indeed, the effects of temperature and food availability on copepod reproduction likely interact because, as poikilotherms, their physiological rates increase with temperature. Ingestion rates may therefore increase with warming, providing the animals with more food to fuel increased reproductive rates, but only when sufficient resources are available (Anderson et al., 2021). When food is scarce, reproductive demands cannot be met by ingested food alone, and may instead be met from maternal biomass (Smith, 1990; Niehoff, 2004; Mayor et al., 2009a). This is termed capital breeding, as opposed to income breeding, where reproductive demands are met by ingested food only. Without capital resources, when food concentrations are not saturating, egg production, which is considered to be equivalent to growth in adult females (Poulet et al., 1995), may therefore decline with warming because of the higher metabolic costs associated with higher temperatures (Anderson et al., 2021).
The relationship between reproduction and ingestion in Calanus is further complicated by prey selection and how associated feeding behaviour influences the degree to which the available food is ingested. There is evidence for and against selective feeding by calanoid copepods, both dependent and independent of food availability (Kleppel, 1993; Koski and Wexels Riser, 2006). For example, there are numerous examples of dietary selection by food type: for large conic ciliates (Mayor et al., 2006; Leiknes et al., 2014), for diatoms (Kiørboe et al., 1996; Nejstgaard et al., 2008; Kiørboe, 2011; Peter and Sommer, 2012; Ray et al., 2016a; Ray et al., 2016b), directly by nutritional content (Cowles et al., 1988; Carroll et al., 2019), by size (Hansen et al., 1990; Meyer et al., 2002), by toxicity (Teegarden et al., 2008), motility or chemical cues. In contrast, other studies have suggested that Calanus shows little or no prey selectivity (Castellani et al., 2008; Mayor et al., 2009a; Djeghri et al., 2018). Prey preference is rooted in achieving nutritional balance - copepods that ingest food which does not meet their stoichiometric demands can face decreased growth, egg production and hatching success (Jónasdóttir et al., 2002). Diatoms are thought to be key in the diet of Calanus (Irigoien et al., 2002; Kohlbach et al., 2021), positively correlating with both ingestion and production. Understanding patterns of prey selection by Arctic Calanus is a fundamental precursor to determining how the changing food environment will impact their ability to obtain the necessary resources to reproduce.
Our aim was to investigate the relationship between reproductive output and the food environment in female C. finmarchicus across a range of food environments in the Fram Strait in May - June 2018. We conducted a series of experiments in which rates of ingestion, prey selection, and egg production were measured for replicate groups of animals, and determined the elemental content of the experimental animals. Metabolic budgets, which compare C intake to that lost via egg production, respiration and egestion of faecal matter, are used to examine how the reproductive physiology of C. finmarchicus varies in response to the local food environment.
Methods
Experimental procedure
Ingestion and egg production rates of female Calanus finmarchicus were measured simultaneously at 18 stations across the Fram Strait in May-June 2018 (Figure 1; Table 1; RRS James Clark Ross cruise JR17005). The natural plankton assemblage was collected daily from the chlorophyll maximum via 20 L Niskin bottles. Two 200 mL water samples were collected at each station and preserved with 1% acidified Lugol’s iodine for subsequent microplankton analysis. Copepods were collected using a motion-compensated bongo net fitted with a 200 µm mesh hauled vertically from 200 m and subsequently transferred into buckets containing surface seawater. Female C. finmarchicus were picked using a dissection microscope (Wilde M5), and swan-necked forceps under gentle illumination. The identities of the animals collected at each station were verified using molecular analysis of the 16S rDNA barcode (Lindeque et al., 2022). All experimental work was conducted in a temperature-controlled room at 1.6 ± 1.1°C.
Ingestion and egg production rates were determined simultaneously using a series of sequential 24-hour particle removal experiments, as previously described (Mayor et al., 2009a). These were planned to last for a total of 5 days to allow the robust measurement of the change in biomass over that period, but experiments 1, 2 and 5 were curtailed due to adverse weather conditions and logistics. At the outset of each experiment, groups of 10 healthy and active female C. finmarchicus were transferred into replicate (n = 6) 2.2 L glass bottles containing natural seawater from the chlorophyll maximum and incubated for 24 hours on a plankton wheel at ~1 rpm. Three additional control bottles were incubated without the addition of copepods to account for microplankton growth during the incubations. Microplankton samples (100 mL) were collected from the control and grazed bottles at the start and end of each 24-hour incubation period and preserved with 1% acidified Lugol’s iodine. The remaining water from the grazed bottles was gently passed through a 63 µm mesh sieve to collect and enumerate any eggs produced by the experimental females during the incubation. Any eggs found in the microplankton samples were added to the respective sample’s egg total. Nauplii were excluded as they were unlikely to have hatched from experimental eggs – hatching of Calanus eggs at these temperatures takes around five days (Corkett et al., 1986). This procedure was repeated for up to 5 consecutive days, with experimental females being gently transferred into fresh seawater every day via a wide-bore (10 mm internal diameter) plastic dip tube. Replicate groups (n = 6) of 5 copepods were frozen in tin cups at -80°C at the start and end of each experiment to determine any changes in the C and nitrogen (N) content of their biomass over the duration of the experiment. Elemental analysis of the freeze-dried experimental animals was conducted using a Flash EA 1112 Series Elemental Analyser (Thermo Fisher). The gonad maturation stage (GS) of ≥10 females from each station where the experimental animals were collected was determined following the description of Niehoff and Runge (2003).
Microplankton analysis
Samples were gently agitated for one minute before being transferred to 25 mL Utermöhl sedimentation chambers and left to settle for 48 hours (Lund et al., 1958). Cells were identified and enumerated with a Brunel SP95I inverted microscope at × 250 and × 400 magnification for cells >2 µm and small flagellates, respectively (Båmstedt et al., 2000; Mayor et al., 2006). Small and large diatom categories refer to centric cells with diameters <20 µm (e.g. Chaetoceros spp.) and ≥20 µm (e.g. Thalassiosira spp.), respectively. Cell dimensions were measured for each genus present using an ocular micrometer and their volumes were calculated by applying appropriate geometric formulae as is common practice (Hillebrand et al., 1999; Menden-Deuer and Lessard, 2000; Mayor et al., 2009b). Measurements of representative cells were repeated until the cell volumes for each group were normally distributed. Cell volumes were converted to carbon biomass using published conversion factors specific to the cell type (Menden-Deuer and Lessard, 2000; Malzahn and Boersma, 2012). Chlorophyll-a (CAS 479-61-8) was measured with an in-situ Chelsea Aqua 3 Fluorometer.
Carbon budgets
Metabolic C budgets were constructed for copepods according to (Equation 1):
where the terms, all expressed as biomass-specific rates per day, are ingestion (I), egg production (E), respiration (R), production of faecal matter (W) and a balancing term (Ω). The balancing term captures processes not specified in the simple budget. C-specific ingestion rates (day-1) were estimated using the mean C content of the females within each experiment and the ingestion rate per experimental bottle.
Ingestion rates of individual animals (II; μmol C ind-1 day-1) were calculated using established equations (Frost, 1972) and converted to biomass-specific rates (I; day-1) as described above. Egg production (EI; μmol C ind-1 day-1) was measured and converted to C units assuming 20.9 nmol C egg-1 (Mayor et al., 2009a); Mayor, unpublished data). Gross growth efficiency (GGE) was then calculated as E/I, i.e., egg production as a fraction of intake. The respiration rate of individual animals was estimated from the globally-used equations of Ikeda et al. (Ikeda et al., 2001) (Equation 2):
where B is body weight (mg N ind.-1) and T is temperature (°C) of the laboratory in which our experiments were conducted.
Values were converted to C units, RI, (µg CO2-C ind-1 hr-1) by assuming a respiratory quotient (RQ) of 0.97 and multiplying by 12/22.4 (Ikeda et al., 2000). Conversion of RI to the corresponding specific rate, R, was as described above. Production of faecal matter (W), i.e. the fraction of ingested food that does not pass across the gut wall, was calculated as I × 1- absorption efficiency (AE), using an assumed AE of 0.47 (Mayor et al., 2011). Excess (surplus) C, Ω, was calculated by difference using Equation 1. The sensitivity of the budget to the assumed values for RQ and AE was examined by changing these values to 0.7 (Mayzaud, 1976) and 0.74 (Anderson et al., 2017), respectively.
Statistical and computational analysis
Prey preference was examined by comparing the abundance of a cell type in the food environment relative to the abundance of that cell type ingested. Parametric statistical tests were used (ANOVA and Pearson’s). Results were considered significant at the 0.05 level. When assumptions of normality, linearity and variance homogeneity were not maintained, non-parametric tests were used (Spearman’s rho, ρ). Averages shown are mean average followed by standard deviation. Statistical analysis and data visualisation were done using the R programming environment (R Core Team, 2021) using the packages ggplot2 (Wickham, 2016) and viridis (Garnier, 2018).
Results
Dominant water masses
Water temperatures at stations NT7, NT2, F21, F17, F15, F13 and F10 were all < 0.0°C (Table 1) showing the water body was the southerly moving East Greenland Current. At F8 there was a sharp increase in temperature showing the front between the East Greenland Current and the West Spitsbergen Current, where temperatures ranged from 0.58 to 4.31°C. Station KB0, near the mouth of Kongsfjorden, and stations ST1 and ST2g in Storfjorden, also had temperatures < 0.0°C due to their proximity to coastal meltwater runoff. Across all the stations, the average temperature at the chlorophyll-a maximum was 0.14 ± 1.9°C (Table 1).
Microplankton
The composition and biomass of the microplankton varied considerably across the Fram Strait (Figure 2; Supplementary Table 1). Total biomass ranged from 18.0 to 187.0 µmol C L-1, averaging 61.6 ± 46.6 µmol C L-1. Stations in the area of East Greenland, NT2, F21, F17 and F15, were generally below this average, with 18.0, 21.0, 69.5, and 30.0 µmol carbon L-1, respectively. Biomass was high at the two stations in the south of the study area, NT8 and NT5, where the water column contained 109.3 µmol C L-1 and 187.0 µmol C L-1, respectively. Biomass was also high at station F13, where it reached 143.0 µmol C L-1.
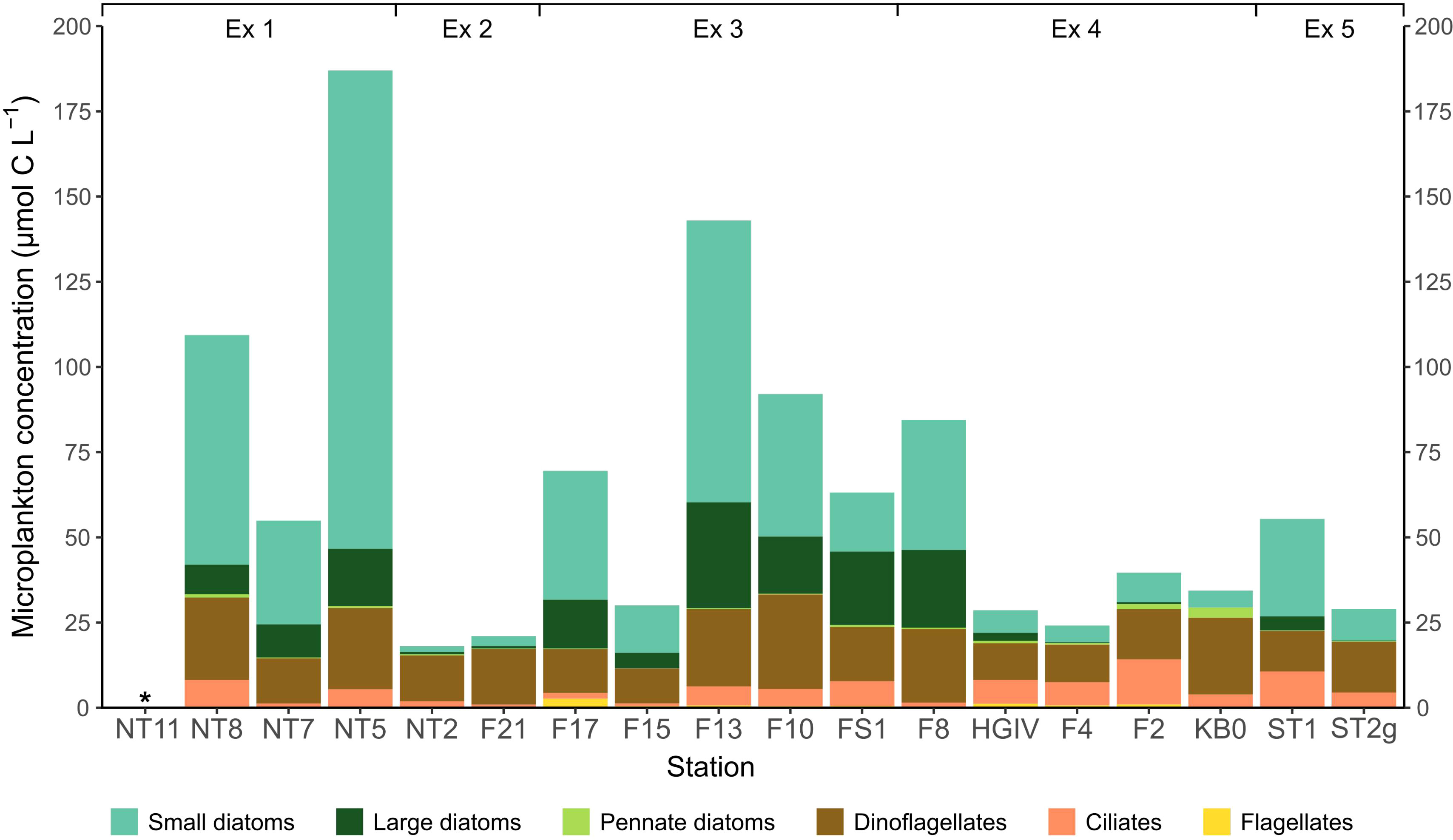
Figure 2 The microplankton food environment for Calanus finmarchicus across the Fram Strait, calculated from inverted microscopy of water sampled at the chlorophyll maximum at each station. *indicates where the parameter was not measured.
Small chain-forming diatoms, and to a lesser extent large diatoms and dinoflagellates, were the dominant microplankton type at the majority of stations sampled (Figure 2). The stations close to Svalbard and the West Spitsbergen Current (HGIV, F4, F2, and KB0) had a lower proportion of both small and large diatoms. The westward stations (NT2, F21, F17, and F15) had a lower proportion of ciliates than those eastward (F13, F10, FS1, HGIV, F4, F2, except for F8 and KB0). The small and large diatom peaks in the mid stations (F13, F10, FS1, F8) suggest a diatom bloom. The microplankton communities along the southerly transect (NT8, NT7 and NT5) also denote a bloom of small chain-forming diatoms. Flagellates were numerous, with colonies of Phaeocystis spp. found at many of the stations sampled, but they did not contribute significantly to the community biomass.
Elemental composition of Calanus finmarchicus
The average C and N contents of the experimental animals were variable (Figure 3, Supplementary Table 2). The average C content of C. finmarchicus females was 15.0 ± 2.9 µmol female-1, ranging from 11.2 to 22.9 µmol female-1, and the average N content was 2.2 ± 0.4 µmol female-1, ranging from 1.5 to 3.0 µmol female-1. The molar C:N ratio of the females averaged 6.8 ± 1.0 and ranged from 5.6 to 8.7. There was more variability in the ratio between individual animals than there was between average values at the start and the end of the experiments. The C and N content of the experimental females did not vary significantly between the different the five experiments (F(4, 20) ≤ 2.318, p ≥ 0.09 in both cases) or change between the start and end of the incubations (F(1, 20) ≤ 0.093, p ≥ 0.76) (Supplementary Figure 1).
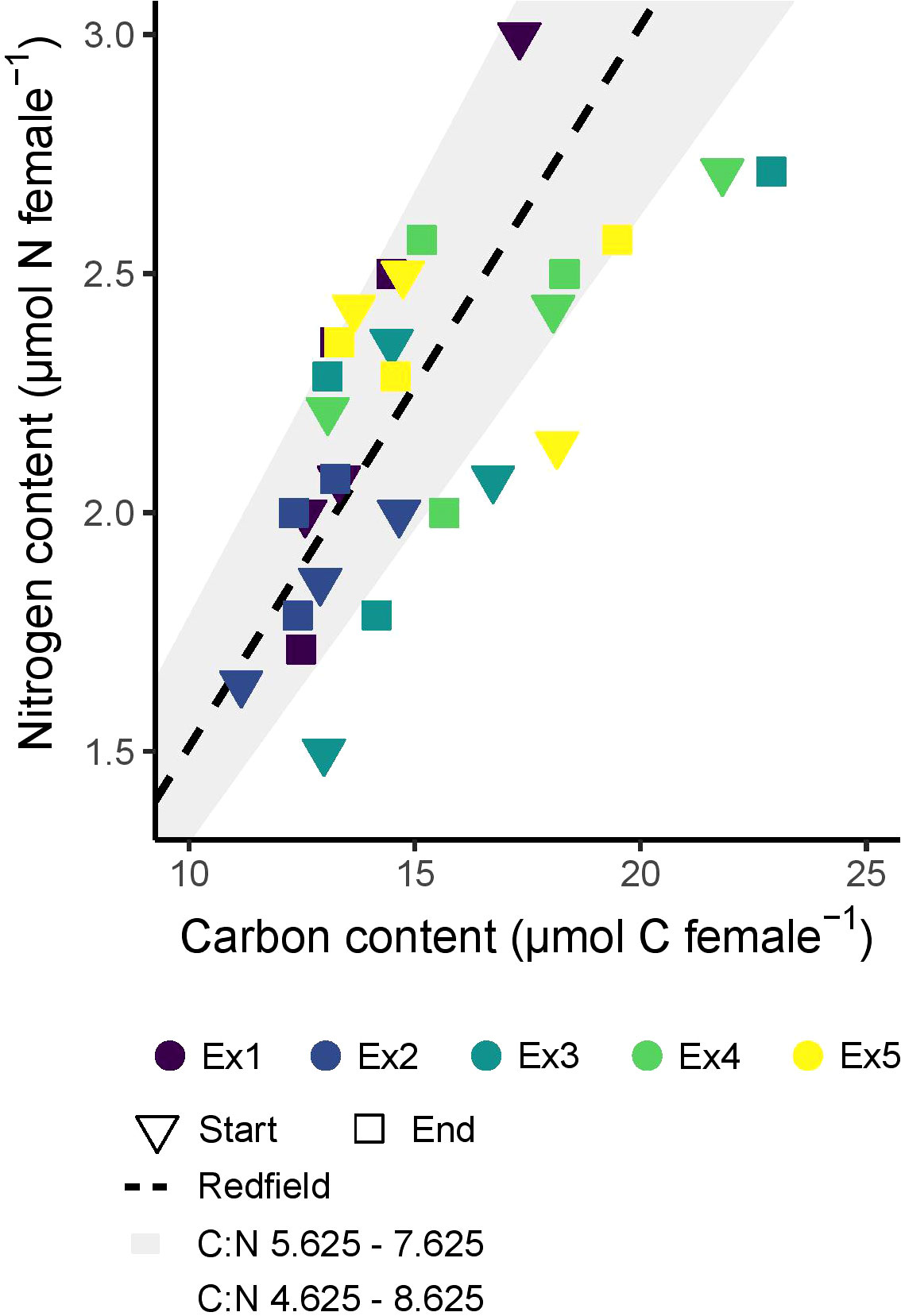
Figure 3 The elemental content of females across experiments 1-5 (Ex1-5), before and after experimentation. Start indicates representative adults sampled at the beginning of each experiment, and end represents the composition of the same cohort of adults used in the experiments. ‘Redfield’ is the Redfield ratio (Redfield, 1958) found between carbon and nitrogen in phytoplankton (6.625 by atoms).
Ingestion and egg production
Total daily ingestion rates ranged from 0.3 to 12.3 µmol C female-1 day-1 and averaged 4.7 ± 3.6 µmol C female-1 day-1 (Figure 4; Supplementary Table 1). The station with the highest average ingestion was F10 (10.1 ± 0.3 µmol C female-1 day-1) and the station with the lowest was KB0 (0.3 ± 0.1 µmol C female-1 day-1). C-specific ingestion rates ranged from 0.015 ± 0.004 to 0.645 ± 0.017 day-1 and averaged 0.295 ± 0.223 day-1.
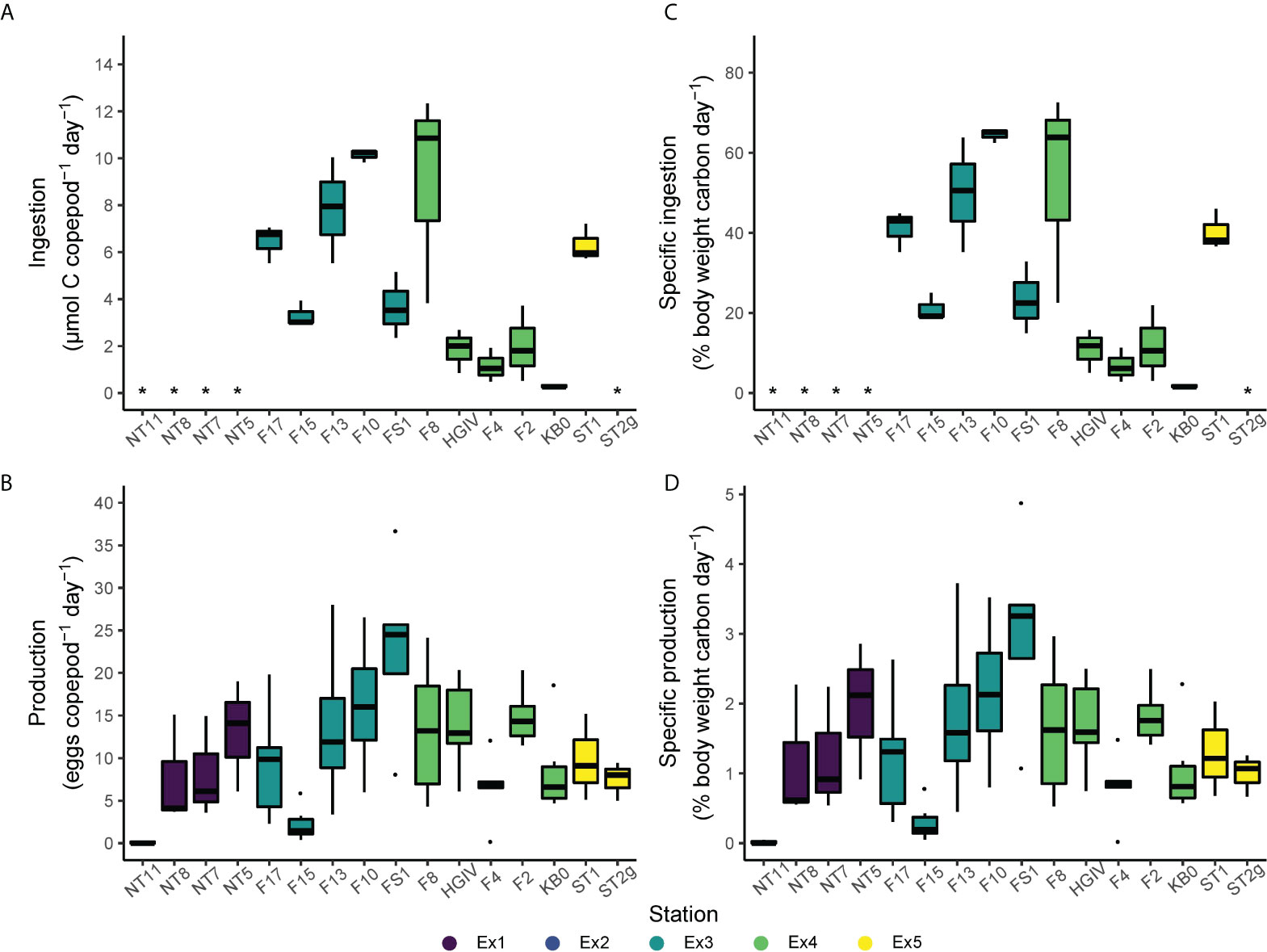
Figure 4 Total ingestion (A), specific ingestion (B), total egg production (C), and specific egg production (D) by Calanus finmarchicus across the Fram Strait. The mid-line represents the median, the box the upper and lower quartiles, whiskers the range, and black points the outliers. Specific ingestion and production were converted from a fraction to a percentage for these figures. * indicates where the parameter was not measured.
Egg production in the grazing experiments ranged from 0.0 to 36.7 eggs female-1 day-1, and averaged 8.9 ± 8.1 eggs female-1 day-1 across all experiments (Figure 4). This corresponds to C-specific egg production rates ranging from 0.00 to 0.049 day-1, and an average of 0.012 ± 0.011 day-1. Egg production rates did not correlate with female C content, N content, or the C:N ratio of the experimental animals (Supplementary Figure 1). At the stations where the experimental animals were collected, between 5-50% of the females were ≤GS3, and many of those within GS4 were developing relatively small clutches of eggs (= GS4B and GS4C) (Table 2).
Prey was ingested in approximately similar proportions to that which was available. The proportions of all diatom types that were ingested correlated positively, albeit weakly, with the proportions available in the prey field (small diatoms: ρ = 0.31; large diatoms: ρ = 0.52; pennate diatoms: ρ = 0.72, p < 0.05 in all cases). The proportion of ciliates ingested also correlated with the proportion available (ρ = 0.48, p = 0.004). By contrast, there was a negative relationship between available and ingested dinoflagellates (ρ = -0.56, p < 0.001) and no significant correlation between the proportions of flagellates available and those ingested (Figure 5).
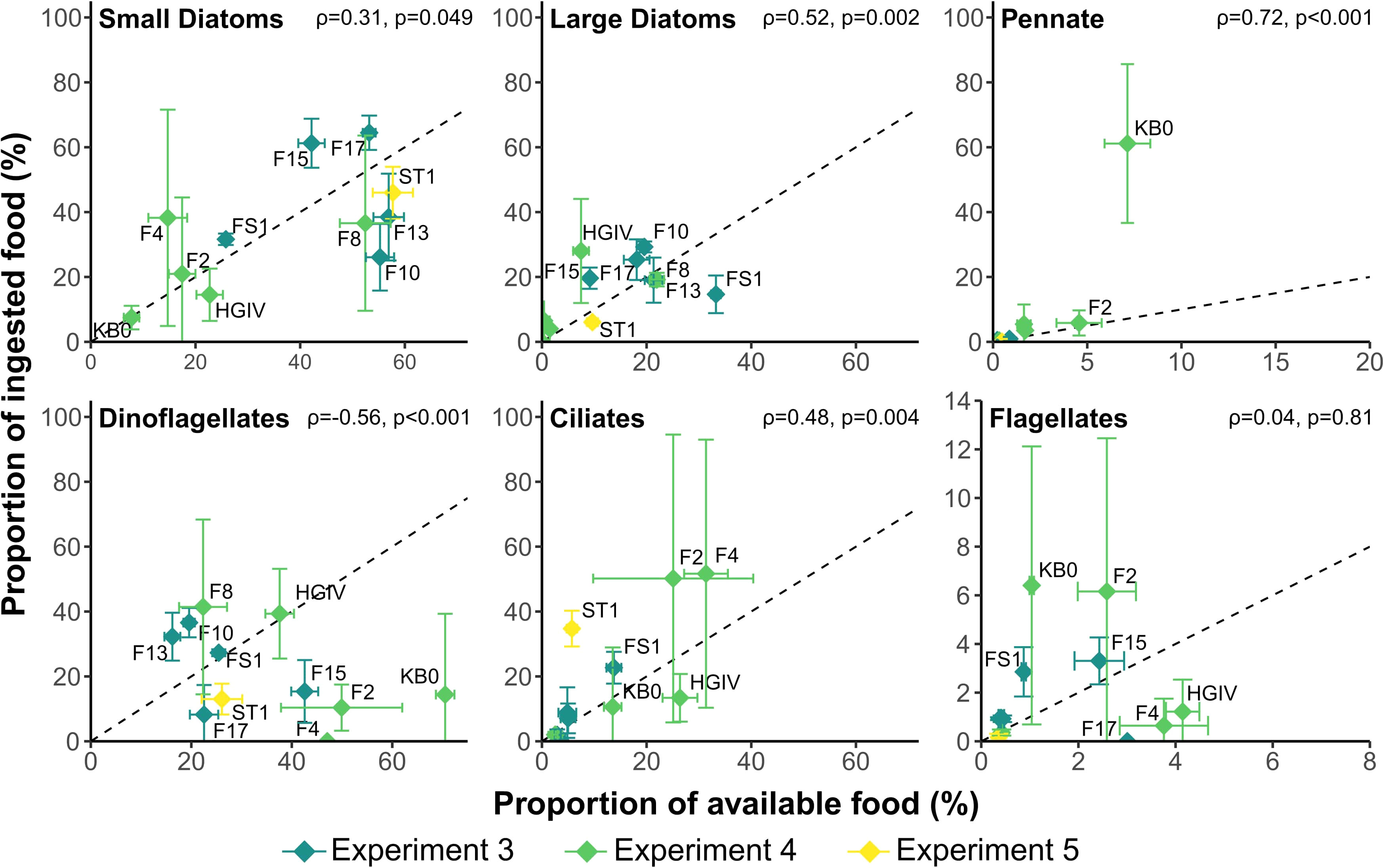
Figure 5 The contribution of food types to the diet of Calanus finmarchicus against the contribution of the food types to the available food environment. The dotted line represents the 1:1 line where ingestion is proportional to available food, i.e. non-selective feeding. Above this, a greater proportion is ingested than is available, so the food is selected for, and below, the food is selected against. Note the variable scales on the x- and y-axes.
The specific rate of ingestion increased significantly with the concentration of microplankton carbon (Figure 6B). This relationship appeared to be driven largely by the concentrations of small and large diatoms (Figure 6C), was reflected by the concentration of dinoflagellates (Figure 6D), and was not reflected in the chlorophyll concentrations (Figure 6A). Specific egg production, in contrast to ingestion, showed no relationship with any measure of food availability (Figures 6E–H; all p > 0.05). Similar relationships were seen between total rates of ingestion and production and measures of food availability (Supplementary Figure 2). Specific rates of egg production were generally higher when specific ingestion rates were higher, but the correlation between these variables was not significant when examined across all stations (ρ = 0.21, p > 0.2; Supplementary Figure 3). There was a significant positive relationship between the specific egg production rate measured here and the and the proportion of females that were spawning (Cook et al., unpublished; ρ = 0.39, p = 0.027; Supplementary Figure 4). There was no relationship between ingestion or egg production and environmental temperature before the experiment began (r = -0.2, p > 0.1 and r = 0.2, p > 0.1, respectively).
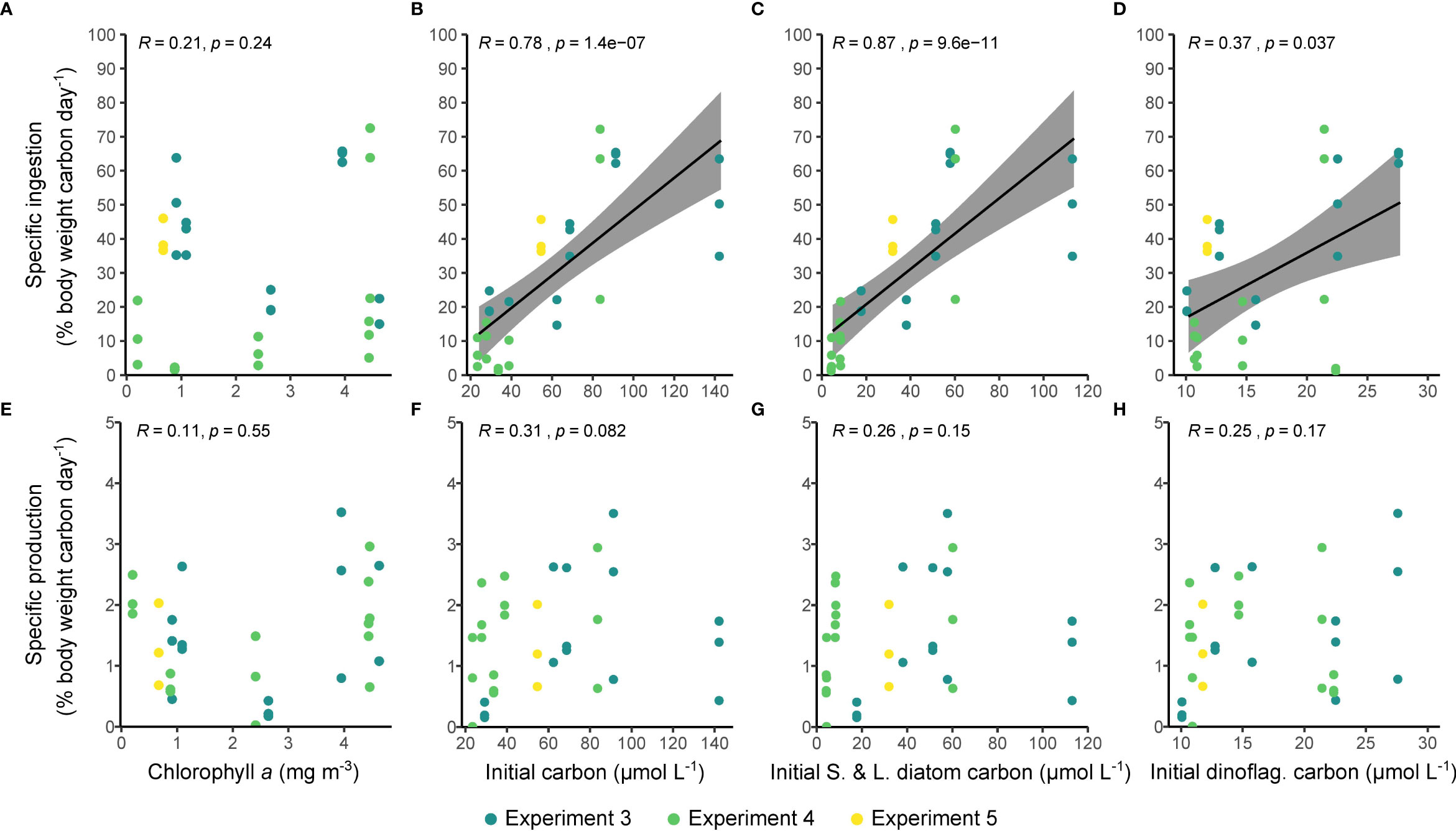
Figure 6 The relationships between the quantity of available food and ingestion (A–D) and egg production rate (E–H). The quantity of available food is estimated from using the chlorophyll a concentration as a proxy (A, E), and calculated using inverted microscopy for all cell types (B, F), for small (S) and large (L) diatoms (C, G), and for dinoflagellates (D, H). Shaded areas show 95% confidence intervals. R is Spearman’s rho.
Carbon budgets
The daily metabolic C budgets for the experimental animals are shown in Table 3. The calculated gross growth efficiencies of the animals ranged from 0.01 to 0.39, averaging 0.12 ± 0.13 (Table 3). Ingestion was consistently higher than the total C needed for egg production, respiration, and egestion combined. In all but one station, C. finmarchicus had an excess of C. The surplus was on average 1.6 ± 1.6 µmol C individual-1 day-1 or 19.2 µg C individual-1 day-1. As a proportion of intake, this corresponds to 0.17 ± 0.42 (Table 3), which is more than needed for egg production and only slightly less than used for respiration. Stations F8, F10 and F13 had the greatest amounts of excess, where the fractions of C intake (C individual-1 day-1) were 0.40, 0.40 and 0.41, respectively. By contrast station KB0 had a C-deficit of 1.04 as a fraction of C intake individual-1 day-1.
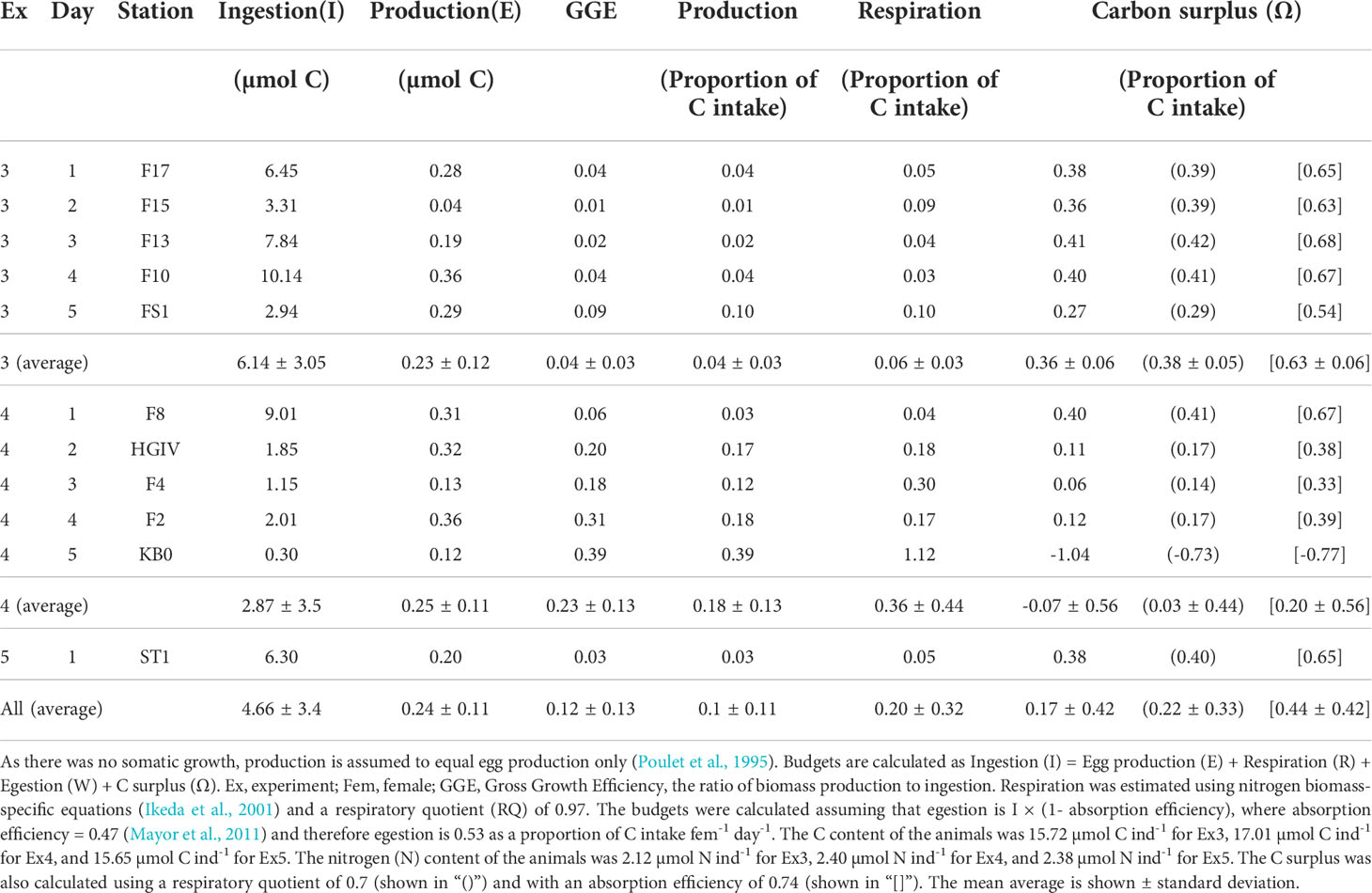
Table 3 Daily metabolic carbon (C) budgets for Calanus finmarchicus (female-1 day-1) [, showing measured ingestion and production].
Increasing the AE to 0.74 had the greatest effect on the budget of all the non-measured terms, with higher efficiencies adding to the C surplus (Table 3). Changing the assumed metabolic substrate by adjusting the RQ had only a small effect on the surplus. Assuming complete lipid metabolism, i.e. RQ = 0.7 (Ikeda et al., 2000), the surplus would again be increased (Table 3).
Discussion
Our study quantified feeding and reproduction in female Calanus finmarchicus and examined how these varied in response to the food environment across the Fram Strait in May-June 2018. We show that ingestion rates typically increased as the total amount of microplankton food available increased. By contrast, egg production showed no obvious relationship with food availability. The incubated animals mostly displayed low gross growth efficiencies (GGE <0.20). This suggests that a large fraction of the ingested C was used for physiological processes other than egg production.
Ingestion
The stations sampled across the Fram Strait were typically characterised by elevated microplankton concentrations, and thus feeding conditions were almost always favourable. This was particularly evident in Experiments 1 (NT8, NT7, NT5) and 3 (F17, F15, F13, F10, FS1), where concentrations were > 50 µmol C L-1 on all but one day. Food concentrations > 42 µmol C L-1 have been found to be saturating for C. finmarchicus (Båmstedt et al., 1999). At stations NT5 and F13 microplankton C concentrations were ≥ 142 µmol L-1, and many of the other stations sampled (NT8, NT7, NT5, F17, F13, F10, FS1, F8, KB0, ST1) had food concentrations > 42 µmol C L-1 (500 µg C L-1). Diatoms were abundant at the southerly stations sampled during Experiment 1 (NT8, NT7, NT5), and at the stations in Experiment 3 (F17, F15, F13, F10, FS1). In contrast, much lower diatom concentrations were encountered at the stations sampled during Experiment 2 (NT2, F21), and at several of those sampled during Experiment 4 (HGIV, F4, F2, and KB0).
C. finmarchicus typically consumed prey in proportion to their availability in the plankton (Figure 5). This pattern of intake is similar to what has been found in other areas, such as North Atlantic (Mayor et al., 2006; Castellani et al., 2008; Mayor et al., 2009a) and the English Channel (Djeghri et al., 2018), and supports the understanding that C. finmarchicus are less selective than other calanoid copepods (Teegarden et al., 2008). Diatoms dominated the diet of C. finmarchicus at most stations examined, as is often reported (Irigoien et al., 2002; Søreide et al., 2008; Cleary et al., 2017; Kohlbach et al., 2021). This intake simply reflects the predominance of diatoms in the microplankton, rather than these cells being positively selected for. There was some evidence for positive selection towards ciliates at stations F2, F4 and ST1, where ciliate biomass was high and diatom biomass, particularly that of large diatoms, was low. It seems that the copepods actively selected for ciliates at these stations in order to compensate for the reduction in diatom biomass, as has been observed previously (Mayor et al., 2006). By contrast, the proportion of dinoflagellates in the ingested ration was negatively correlated with their availability in the microplankton (Figure 5), suggesting that there were increasingly selected against. A range of dinoflagellates are known to be capable of producing toxins that reduce food absorption, egg production rates and egg hatching success in C. finmarchicus (Roncalli et al., 2016) and the apparent avoidance of dinoflagellates may indicate the presence of one or more toxin-producing species. However, the absence of a negative relationship between ingested dinoflagellate C and egg production (Figure 6H) suggests that any potential negative effect of consuming dinoflagellates was insufficient to cause a noticeable effect.
Ingestion by C. finmarchicus was on average 4.7 ± 3.6 µmol C female-1 day-1, well within the previously reported range for this species when feeding on natural plankton assemblages [0.04 – 7.33 µmol C female-1 day-1 (Jónasdóttir et al., 2008; Mayor et al., 2009a)]. C-specific ingestion rates were generally close to 0.3 day-1, which also fits well within the published range (Gamble, 1978; Ohman and Runge, 1994; Nejstgaard et al., 1997), matching with similar ingestion rates found during bloom periods in the Norwegian Sea (Irigoien et al., 1998). The maximum value for C-specific ingestion, 0.645 ± 0.017 day-1, was high, but again, consistent with the 0.80 day-1 previously reported for C. finmarchicus when feeding during periods of elevated food availability (Smith and Lane, 1988). Indeed, it is probable that the elevated ingestion rates reported herein were because of the high concentration of food available, as suggested by the strong positive correlations between ingestion and both the total concentration of microplankton and that of diatoms (Figures 6B, C); the weakly significant correlation between ingestion and dinoflagellate concentrations likely reflects the collinearity between dinoflagellate- and diatom C, rather than a causal relationship.
Interestingly, there was no relationship between chlorophyll a concentration, a common proxy for food availability, and ingestion (Figure 6A). This could be because the chlorophyll a data were just a snapshot of the sampling location and therefore not representative of the food available to the incubated copepods. This suggestion is supported by the lack of a relationship between available food and chlorophyll a (add details of correlation (or lack thereof) here). This disparity is also potentially attributable to the high abundances of picoplankton (≤2 µm cell diameter) that have been reported to occur in the Fram Strait, in particular the Micromonas genus of prasinophytes (Bachy et al., 2022), that were not enumerated in our study.
Egg production
The reproductive strategy of C. finmarchicus can vary in response to food supply, likely a necessity of its one-year life cycle (Falk-Petersen et al., 2009). Maximum egg production rates occur during the spring bloom, but the timing of this differs between areas and is controlled by hydrography, light conditions and climate (Niehoff, 2004). Egg production is subject to prior gonad maturation and oocyte development.
The measured egg production rates were within the range 0.3 – 36.7 eggs female-1 day-1 previously observed for C. finmarchicus in the Arctic (Hirche, 1990; Hirche and Kosobokova, 2007; Møller et al., 2016). C-specific egg production rates were within the range of 0.00 to 0.049 day-1, in good agreement with the published range for C. finmarchicus (Hirche et al., 1997; Mayor et al., 2006; Møller et al., 2016; Jónasdóttir et al., 2022). The observed rates did not correlate with any measure of ingested food quantity or prey type. This either suggests that the link between recent feeding history and egg production rate is weak, or that the rate at which eggs are produced is not directly limited by the available food. However, many studies have found a strong link between food quantity and egg production rate (Marshall and Orr, 1958; Hirche, 1990; Ohman and Runge, 1994; Hirche et al., 1997; Harris et al., 2000; Hirst and Bunker, 2003; Jónasdóttir et al., 2022). Two of these studies were conducted in the Arctic, but the animals were either kept under identical feeding conditions for some time before experimentation (Hirche et al., 1997), or their eggs were only counted after 48 hours (Hirche, 1990), rather than 24 hours used herein, to allow for a longer spawning interval. A time ‘lag’ of > 24 hours between ingestion and the production of eggs could potentially explain the absence of a relationship between egg production rates and both food availability and ingestion rates; C. finmarchicus has previously been observed to display a spawning interval of > 24 hours in the Arctic at 0°C (Hirche, 1990). However, egg production did not correlate with the amount of food ingested during the preceding day (p ≥ 0.88 in all cases). Furthermore, independent egg production experiments conducted in parallel to those presented herein revealed that, on average, 66% (ranging from 0-94%) of the 20 individual females incubated at each station produced eggs within the first 24 hours (Cook et al., unpublished), indicating that the spawning interval was generally < 24 hours throughout the period of our study.
The disconnect between ingestion and egg production seen in our experiments could, alternatively, indicate that the animals were using maternal reserves, rather than, or in addition to, the ingested food to produce eggs. C. finmarchicus has previously been observed to adopt a capital breeding reproductive mode in the North Atlantic when feeding conditions are poor, losing significant quantities of maternal biomass C and N to fuel continued egg production (Niehoff, 2004; Mayor et al., 2006; Mayor et al., 2009a). The C and N contents of the experimental animals in the present study did not, however, change significantly throughout the experiments (Figure 3; Supplementary Figure 1), indicating that they were not using biomass reserves to fuel reproduction. Indeed, the metabolic budgets show that the copepods ingested C in excess of that required for egg production and other physiological processes (discussed below). Regardless of the underlying mechanism, the lack of a relationship between the observed rates of ingestion and reproductive output indicates that accurately predicting how C. finmarchicus will respond to projected changes in their food environment is complex and likely requires information beyond simple metrics of food concentration.
Carbon budgets
Carbon budgets were constructed for each experiment by combining measured rates of C intake and egg production rates with empirically-estimated rates of respiration and faecal pellet production. In all but one instance (Experiment 4, day 5; Table 3), C intake could not be fully accounted for by respiration and the production of eggs and faecal matter. Indeed, across all experiments, the average fraction of ingested C that was in excess of requirements was 0.17 ± 0.42.
The fractions of intake allocated to respiration and egestion were approximately 0.20 and 0.531, respectively. Changing the respiratory quotient towards lipid-fueled metabolism (RQ = 0.7) only reduced the estimated C required for respiration by a small amount, causing the apparent excess of C to increase slightly (Table 3). The chosen value of AE, 0.47, was selected because it relates specifically to C. finmarchicus feeding on diatoms (Mayor et al., 2011), the main prey item in our experiments. Increasing AE to 0.74, which is commonly assumed when modelling marine copepods (Anderson et al., 2017; Anderson et al., 2020; Anderson et al., 2021), decreases the fraction of ingested C released as faecal matter to 0.26, further increasing the excess of C in the metabolic budget. We therefore suggest that our estimated C excesses are conservative.
Calculated GGEs were low, usually well below the expected range of 0.2 – 0.3 observed for copepods (Straile, 1997), meaning that egg production accounted for a relatively small fraction of the consumed food. Cannibalism of eggs could potentially explain the relatively low GGEs (Bonnet et al., 2004) and the C surpluses. However, previous work using the same experimental design as used here concluded that the effects of cannibalism in these experiments were negligible (Mayor et al., 2006; Mayor et al., 2009a). Furthermore, if the apparent C excesses were simply caused by egg cannibalism, the actual EPRs would have been 89.1 ± 76.8 (with a maximum EPR of 212.8) – towards or beyond the upper end of field-reported values (Hirche, 1990; Hirche et al., 1997; Niehoff et al., 1999; Richardson et al., 1999; Swalethorp et al., 2011; Møller et al., 2016, Supplementary Table 3), and well in excess of the rates determined in parallel experiments with individual females that were excluded from their eggs to prevent cannibalism (24.1 ± 14.4 eggs female-1 day-1; Cook et al., unpublished). This suggests that egg cannibalism cannot explain the apparent C excesses.
Like many polar copepods, all of those in the genus Calanus are well-known for their ability to produce and store lipids (Lee et al., 2006). It is therefore possible that the excess C reflects lipid biosynthesis and accumulation by these animals. However, this seems unlikely, given that a) the experiments were conducted in May at the very start of the growth season, b) C. finmarchicus typically undertake a 1-year life cycle (Falk-Petersen et al., 2009), and thus females are not thought to re-enter diapause, and, most importantly, c) the C content of the experimental animals did not increase significantly over the course of the experiments (Figure 3; Supplementary Figure 1). We therefore propose that the observed metabolic C surpluses may, at least in part, be explained by the significant energetic costs associated with gonad maturation. In C. finmarchicus, this process generally starts in stage V copepodites (CVs) several months before the animals emerge from their over-wintering period and progresses in newly moulted females during the following spring (Tande, 1982; Hirche, 1996; Jónasdóttir, 1999; Niehoff et al., 2002; Niehoff, 2007). The rates at which newly moulted females produce eggs increases from zero to maximal rates over 15 days at 5°C (Rey et al., 1999), and likely takes longer at colder temperatures (Melle and Skjoldal, 1998). The observed gonad maturation stages (Table 2) further supports the suggestion that a proportion of the experimental females were still in the process of developing their ovaries.
Gonad maturation in C. finmarchicus is known to require large amounts of energy, ~ 5.8 µmol C individual-1 (Rey-Rassat et al., 2002). At times these animals are able to provide the resources for gonad maturation and/or egg production from their own biomass (Irigoien et al., 1998; Niehoff, 2004; Mayor et al., 2006; Mayor et al., 2009a). Females that have just undergone gonad maturation therefore often exhibit depleted lipid reserves (Sargent and Falk-Petersen, 1988; Rey-Rassat et al., 2002; Anderson et al., 2022) with biomass C:N ratios declining as low as ~5 by atoms when spawning begins (Tande, 1982; Mayor et al., 2009b). However, the lack of a clear decline in biomass C and N content over the duration of our experiments (Figure 3) suggests that our experimental animals were not meeting the costs of maturation from internal reserves, and were instead acquiring them via ingestion. There are multiple observations of recently moulted females needing to feed prior to completing maturation and commencing egg production. For example, in the lower St. Lawrence Estuary, the final stages of oocyte maturation in C. finmarchicus females does not begin until feeding conditions become favourable in June (Plourde and Runge, 1993), and in the Norwegian Sea, <50% of female C. finmarchicus are mature during the pre-bloom period (March through April), after which the population undergoes rapid maturation as the bloom develops through May (Niehoff et al., 1999). Indeed, recent work suggests that the final step in terminating diapause in C. finmarchicus may also be dependent upon the presence of food (Hatlebakk et al., 2022).
The average C:N ratio of our experimental females was 6.8 (ranging between 5.6 – 8.7) by atoms, which is consistent with the understanding that many of them were likely still in the process of reaching maturation (Supplementary Figure 1). Indeed, only 66% of the females incubated in parallel egg production experiments produced eggs (Cook et al., unpublished), suggesting that the remaining third of the population were still undergoing gonad maturation. In addition to explaining the fate of the excess C, the process of gonad maturation occurring in some, but not all of our experimental females would also explain why the observed egg production rates were not correlated with ingestion, and why the proportion of females that were spawning was positively correlated with egg production rate (Supplementary Figure 4; Cook et al., unpublished). In turn, this suggests that our ability to estimate egg production rates in C. finmarchicus and many other high-latitude copepods may be improved by considering their level of maturity, whether by means of morphological investigation or by the development of a metabolic proxy for the level of gonad maturation.
Our budgets focused on C, but that is not to say that the animals were necessarily requiring this element only. Indeed, the experimental animals contained visible quantities of lipid (Supplementary Figure 5), confirmed by their average biomass C:N (6.8 by atoms), which was well above that of an actively spawning female and suggests that they still had C available. Producing mature ovaries and the resulting eggs from stored lipids only, which are largely devoid of N, seems unlikely, particularly as the C:N ratio of C. finmarchicus eggs ranges between 4-7 by atoms (Ohman and Runge, 1994; Runge and Plourde, 1996; Mayor et al., 2009b; Swalethorp et al., 2011) and hence contain a substantial amount of N. We therefore suggest that, in addition to helping meet the energetic costs of maturation, the apparently excessive rates of ingestion prior to reproduction were also required to provide the animals with the amino acids and proteins required to finish producing and maturing their ovaries. We still know relatively little about N-based physiology in C. finmarchicus and if, how, or where they are able to store compounds that bear this element (Mayor et al., 2022). This lack of fundamental understanding hinders our ability to mechanistically represent important aspects of their life histories in ecosystem- and biogeochemical models and predict how they will change in the future (Anderson et al., 2022).
Conclusion
We have shown that female C. finmarchicus are able to take advantage of the abundant feeding conditions encountered during May in the Fram Strait, in part due to their flexible and diverse diet. Egg production did not correlate with food availability or ingestion. Metabolic budgets for our experimental females showed that the ingested food was typically more than that required to produce the observed numbers of eggs and estimated rates of respiration and faecal pellet production. The generally low egg production rates and the relatively high biomass C:N values suggest that a sizeable fraction of the incubated females were reproductively immature, and were using the excess food to meet the energetically-expensive process of gonad maturation and as a source of N-bearing compounds that are required to produce ovary tissues and eggs. This suggestion is supported by the observed gonad maturation status of the sampled female populations. Our study highlights the need to consider ontogenetic development when examining the relationship between ingestion and production in copepods. Developing mechanistic models to reliably predict how the ecological and biogeochemical roles of C. finmarchicus and other high-latitude copepods will respond to climate-driven changes in their food environment requires an improved understanding of both the C- and N-based physiologies of these important animals, particularly during the gonad maturation phase.
Data availability statement
The original contributions presented in the study are included in the article/Supplementary Material. Further inquiries can be directed to the corresponding author.
Author contributions
HJ, KC, TA and DM contributed to the conception and design of the study. HJ conducted experiments, microscopy, and statistical analysis. BT performed the elemental analyses. EM determined plankton cell sizes. EJ analysed gonad maturity. HJ wrote the first draft of the manuscript. All authors contributed to the manuscript and read and approved the submitted version.
Funding
We are grateful to the Natural Environment Research Council (NERC, UK) for funding the Changing Arctic Ocean Research Programme. HJ, FA, KC, EM, TA and DM all received funding from NERC (DIAPOD; NE/P006353/1).
Acknowledgments
We gratefully acknowledge assistance from the Master and crew of the RRS James Clark Ross (JR17005), and the insightful comments from two anonymous reviewers which helped improve this manuscript.
Conflict of interest
The authors declare that the research was conducted in the absence of any commercial or financial relationships that could be construed as a potential conflict of interest.
Publisher’s note
All claims expressed in this article are solely those of the authors and do not necessarily represent those of their affiliated organizations, or those of the publisher, the editors and the reviewers. Any product that may be evaluated in this article, or claim that may be made by its manufacturer, is not guaranteed or endorsed by the publisher.
Supplementary material
The Supplementary Material for this article can be found online at: https://www.frontiersin.org/articles/10.3389/fmars.2022.981461/full#supplementary-material
References
AMAP (2021) Arctic Climate change update 2021: Key trends and impacts. summary for policy-makers. Available at: https://www.amap.no/documents/download/6759/inline.
Anderson T. R., Hessen D. O., Boersma M., Urabe J., Mayor D. J. (2017). Will invertebrates require increasingly carbon-rich food in a warming world? Am. Nat. 190 (6), 000–000. doi: 10.1086/694122
Anderson T. R., Hessen D. O., Gentleman W. C., Yool A., Mayor D. J. (2022). Quantifying the roles of food intake and stored lipid for growth and development throughout the life cycle of a high-latitude copepod, and consequences for ocean carbon sequestration. Front. Mar. Sci. doi: 10.3389/fmars.2022.928209
Anderson T. R., Hessen D. O., Mayor D. J. (2021). Is the growth of marine copepods limited by food quantity or quality? Limnol. Oceanogr. Lett. 6 (3), 127–133. doi: 10.1002/lol2.10184
Anderson T. R., Raubenheimer D., Hessen D. O., Jensen K., Gentleman W. C., Mayor D. J. (2020). Geometric stoichiometry: Unifying concepts of animal nutrition to understand how protein-rich diets can be “Too much of a good thing”. Front. Ecol. Evol. 8. doi: 10.3389/fevo.2020.00196
Bachy C., Sudek L., Choi C. J., Eckmann C. A., Nöthig E.-M., Metfies K., et al. (2022). Phytoplankton surveys in the Arctic Fram Strait demonstrate the tiny eukaryotic alga Micromonas and other picoprasinophytes contribute to deep Sea export. Microorganisms 10, (5). doi: 10.3390/microorganisms10050961
Båmstedt U., Gifford D. J., Irigoien X., Atkinson A., Roman M. R. (2000). “Chapter 8 - feeding,” in ICES zooplankton methodology manual, 297–399. doi: 10.1016/B978-012327645-2/50009-8
Båmstedt U., Nejstgaard J. C., Solberg P. T. (1999). Utilisation of small-sized food algae by Calanus finmarchicus (Copepoda, calanoida) and the significance of feeding history. Sarsia 84 (1), 19–38. doi: 10.1080/00364827.1999.10420449
Basedow S. L., Sundfjord A., von Appen W.-J., Halvorsen E., Kwasniewski S., Reigstad M. (2018). Seasonal variation in transport of zooplankton into the Arctic basin through the Atlantic gateway, Fram Strait. Front. Mar. Sci. 5. doi: 10.3389/fmars.2018.00194
Blachowiak-Samolyk K., Kwasniewski S., Dmoch K., Hop H., Falk-Petersen S. (2007). Trophic structure of zooplankton in the Fram Strait in spring and autumn 2003. Deep-Sea. Res. Part II.: Topical. Stud. Oceanogr. 54 (23–26), 2716–2728. doi: 10.1016/j.dsr2.2007.08.004
Bonnet D., Titelman J., Harris R. P. (2004). Calanus the cannibal. J. Plankton. Res. 26 (8), 937–948. doi: 10.1093/plankt/fbh087
Carroll E. L., Gallego R., Sewell M. A., Zeldis J., Ranjard L., Ross H. A., et al. (2019). Multi-locus DNA metabarcoding of zooplankton communities and scat reveal trophic interactions of a generalist predator. Sci. Rep. 9 (1), 1–14. doi: 10.1038/s41598-018-36478-x
Castellani C., Irigoien X., Mayor D. J., Harris R. P., Wilson D. (2008). Feeding of Calanus finmarchicus and Oithona similis on the microplankton assemblage in the Irminger Sea, North Atlantic. J. Plankton. Res. 30 (10), 1095–1116. doi: 10.1093/plankt/fbn074
Cleary A. C., Søreide J. E., Freese D., Niehoff B., Gabrielsen T. M. (2017). Feeding by Calanus glacialis in a high arctic fjord: Potential seasonal importance of alternative prey. ICES. J. Mar. Sci. 74 (7), 1937–1946. doi: 10.1093/icesjms/fsx106
Corkett C. J., McLaren I. A., Sevigny J.-M. (1986). The rearing of the marine calanoid copepods Calanus finmarchicus (Gunnerus), C. glacialis jaschnov and C. hyperboreus Kroyer with comment on the equiproportional rule. Syllogeus 58, 539–546.
Cowles T. J., Olson R. J., Chisholm S. W. (1988). Food selection by copepods: discrimination on the basis of food quality. Mar. Biol. 100 (1), 41–49. doi: 10.1007/BF00392953
Dai A., Luo D., Song M., Liu J. (2019). Arctic Amplification is caused by sea-ice loss under increasing CO2. Nat. Commun. 10 (1), 1–13. doi: 10.1038/s41467-018-07954-9
Djeghri N., Atkinson A., Fileman E. S., Harmer R. A., Widdicombe C. E., McEvoy A. J., et al. (2018). High prey-predator size ratios and unselective feeding in copepods: A seasonal comparison of five species with contrasting feeding modes. Prog. Oceanogr. 165, 63–74. doi: 10.1016/j.pocean.2018.04.013
Falk-Petersen S., Mayzaud P., Kattner G., Sargent J. R. (2009). Lipids and life strategy of Arctic Calanus. Mar. Biol. Res. 5 (1), 18–39. doi: 10.1080/17451000802512267
Frost B. W. (1972). Effect of size and concentration of food particles on the feeding behaviour of the marine planktonic copepod Calanus pacificus. Limnol. Oceanogr. 1972 (17), 805–815. doi: 10.4319/lo.1972.17.6.0805
Gamble J. C. (1978). Copepod grazing during a declining spring phytoplankton bloom in the northern North Sea. Mar. Biol. 49 (4), 303–315. doi: 10.1007/BF00455025
Garnier S. (2018) Viridis: Default color maps from ‘matplotlib’. Available at: https://cran.r-project.org/package=viridis.
Gatten R. R., Sargent J. R. (1973). Wax ester biosynthesis in calanoid copepods in relation to vertical migration. Netherlands J. Sea. Res. 7 (7), 150–158. doi: 10.2307/2389678
Gluchowska M., Dalpadado P., Beszczynska-Möller A., Olszewska A., Ingvaldsen R. B., Kwaśniewski S. (2017). Interannual zooplankton variability in the main pathways of the Atlantic water flow into the Arctic Ocean (Fram Strait and Barents Sea branches). ICES. J. Mar. Sci. 74 (7), 1921–1936. doi: 10.1093/icesjms/fsx033
Gradinger R. R., Baumann M. E. M. (1991). Distribution of phytoplankton communities in relation to the large-scale hydrographical regime in the Fram Strait. Mar. Biol. 111 (2), 311–321. doi: 10.1007/BF01319714
Hansen B. W., Tande K. S., Berggreen U. C. (1990). On the trophic fate of phaeocystis pouchetii (Harlot). III. functional responses in grazing demonstrated on juvenile stages of Calanus finmarchicus (Copepoda) fed diatoms and Phaeocystis. J. Plankton. Res. 12 (6), 1173–1187. doi: 10.1093/plankt/12.6.1173
Harris R. P., Irigoien X., Head R. N., Rey C., Hygum B. H., Hansen B. W., et al. (2000). Feeding, growth, and reproduction in the genus Calanus. ICES. J. Mar. Sci. 57 (6), 1708–1726. doi: 10.1006/jmsc.2000.0959
Hatlebakk M., Niehoff B., Choquet M., Hop H., Wold A., Hoarau G., et al. (2022). Seasonal enzyme activities of sympatric Calanus glacialis and C . finmarchicus in the high-Arctic. Front. Mar. Sci. 9. doi: 10.3389/fmars.2022.877904
Hillebrand H., Dürselen C. D., Kirschtel D., Pollingher U., Zohary T. (1999). Biovolume calculation for pelagic and benthic microalgae. J. Phycol. 35 (2), 403–424. doi: 10.1046/j.1529-8817.1999.3520403.x
Hirche H. J. (1990). Egg production of Calanus finmarchicus at low temperature. Mar. Biol. 106 (1), 53–58. doi: 10.1007/BF02114674
Hirche H. J. (1996). The reproductive biology of the marine copepod, Calanus finmarchicus — a review. Ophelia 44 (1–3), 111–128. doi: 10.1080/00785326.1995.10429842
Hirche H. J., Bohrer R. N. (1987). Reproduction of the Arctic copepod Calanus glacialis in Fram Strait. Mar. Biol. 94 (1), 11–17. doi: 10.1007/BF00392894
Hirche H. J., Kosobokova K. (2007). Distribution of Calanus finmarchicus in the northern North Atlantic and Arctic Ocean-expatriation and potential colonization. Deep-Sea. Res. Part II.: Topical. Stud. Oceanogr. 54 (23–26), 2729–2747. doi: 10.1016/j.dsr2.2007.08.006
Hirche H. J., Meyer U., Niehoff B. (1997). Egg production of Calanus finmarchicus: Effect of temperature, food and season. Mar. Biol. 127 (4), 609–620. doi: 10.1007/s002270050051
Hirst A. G., Bunker A. J. (2003). Growth of marine planktonic copepods: Global rates and patterns in relation to chlorophyll a, temperature, and body weight. Limnol. Oceanogr. 48 (5), 1988–2010. doi: 10.4238/2015.June.12.13
Holland M. M., Bitz C. M. (2003). Polar amplification of climate change in coupled models. Climate Dyn. 21 (3–4), 221–232. doi: 10.1007/s00382-003-0332-6
Hop H., Falk-Petersen S., Svendsen H., Kwasniewski S., Pavlov V., Pavlova O., et al. (2006). Physical and biological characteristics of the pelagic system across Fram Strait to Kongsfjorden. Prog. Oceanogr. 71 (2–4), 182–231. doi: 10.1016/j.pocean.2006.09.007
Ikeda T., Torres J. J., Hernández-León S., Geiger S. P. (2000). "Chapter 10 - Metabolism" in ICES zooplankton methodology manual, 455–532. doi: 10.1016/B978-012327645-2/50011-6
Ikeda T., Kanno Y., Ozaki K., Shinada A. (2001). Metabolic rates of epipelagic marine copepods as a function of body mass and temperature. Mar. Biol. 139 (3), 587–596. doi: 10.1007/s002270100608
Irigoien X., Harris R. P., Verheye H. M., Joly P., Runge J. A., Starr M., et al. (2002). Copepod hatching success in marine ecosystems with high diatom concentrations. Nature 419, 387–389. doi: 10.1038/nature01072.1
Irigoien X., Head R., Klenke U., Meyer-Harms B., Harbour D., Niehoff B., et al. (1998). A high frequency time series at weathership M, Norwegian Sea, during the 1997 spring bloom: Feeding of adult female Calanus finmarchicus. Mar. Ecol. Prog. Ser. doi: 10.3354/meps172127
Jónasdóttir S. H. (1999). Lipid content of Calanus finmarchicus during overwintering in the Faroe-Shetland Channel. Fish. Oceanogr. 8 (SUPPL. 1), 61–72. doi: 10.1046/j.1365-2419.1999.00003.x
Jónasdóttir S. H., Gudfinnsson H. G., Gislason A., Astthorsson O. S. (2002). Diet composition and quality for Calanus finmarchicus egg production and hatching success off south-west Iceland. Mar. Biol. 140 (6), 1195–1206. doi: 10.1007/s00227-002-0782-0
Jónasdóttir S. H., Naustvoll L., Teglhus F. W., Agersted M. D., Grenwald J. C., Melle W., et al. (2022). Calanus finmarchicus basin scale life history traits and role in community carbon turnover during spring. ICES. J. Mar. Sci., 785–802. doi: 10.1093/icesjms/fsac013
Jónasdóttir S. H., Richardson K., Heath M. R., Ingvarsdóttir A., Christoffersen A. (2008). Spring production of Calanus finmarchicus at the Iceland-Scotland ridge. Deep-Sea. Res. Part I.: Oceanogr. Res. Papers. 55 (4), 471–489. doi: 10.1016/j.dsr.2007.12.009
Jónasdóttir S. H., Visser A. W., Richardson K., Heath M. R. (2015). Seasonal copepod lipid pump promotes carbon sequestration in the deep North Atlantic. Proc. Natl. Acad. Sci. 112 (39), 12122–12126. doi: 10.1073/pnas.1512110112
Kahru M., Brotas V., Manzano-Sarabia M., Mitchell B. G. (2011). Are phytoplankton blooms occurring earlier in the Arctic? Global Change Biol. 17 (4), 1733–1739. doi: 10.1111/j.1365-2486.2010.02312.x
Karpouzoglou T., Steur L., Smedsrud L. H., Sumata H. (2022). Observed changes in the Arctic freshwater outflow in Fram Strait. J. Geophys. Res.: Oceans. 127 (3), 1–17. doi: 10.1029/2021jc018122
Kiørboe T. (2011). How zooplankton feed: Mechanisms, traits and trade-offs. Biol. Rev. 86 (2), 311–339. doi: 10.1111/j.1469-185X.2010.00148.x
Kiørboe T., Saiz E., Viitasalo M. (1996). Prey switching behaviour in the planktonic copepod Acartia tonsa. Mar. Ecol. Prog. Series. 143, 65–75. doi: 10.3354/meps143065
Kleppel G. S. (1993). On the diets of calanoid copepods. Mar. Ecol. Prog. Ser 99, 183–195. doi: 10.3354/meps099183
Kohlbach D., Schmidt K., Hop H., Wold A., Al-Habahbeh A. K., Belt S. T., et al. (2021). Winter carnivory and diapause counteract the reliance on ice algae by Barents Sea zooplankton. Front. Mar. Sci. 8. doi: 10.3389/fmars.2021.640050
Koski M., Wexels Riser C. (2006). Post-bloom feeding of Calanus finmarchicus copepodites: Selection for autotrophic versus heterotrophic prey. Mar. Biol. Res. 2 (2), 109–119. doi: 10.1080/17451000600684367
Lee R. F., Hagen W., Kattner G. (2006). Lipid storage in marine zooplankton. Mar. Ecol. Prog. Series. 307 (1863), 273–306. doi: 10.3354/Meps307273
Leiknes Ø., Striberny A., Tokle N. E., Olsen Y., Vadstein O., Sommer U., et al. (2014). Feeding selectivity of Calanus finmarchicus in the Trondheimsfjord. J. Sea. Res. 85, 292–299. doi: 10.1016/j.seares.2013.05.012
Lewis K. M., Van Dijken G. L., Arrigo K. R. (2020). Changes in phytoplankton concentration now drive increased Arctic Ocean primary production. Science 369 (6500), 198–202. doi: 10.1126/science.aay8380
Li W. K. W., McLaughlin F. A., Lovejoy C., Carmack E. C. (2009). Smallest algae thrive as the Arctic Ocean freshens. Science 326 (5952), 539–539. doi: 10.1126/science.1179798
Lindeque P. K., Hann I., Parry H. E., Cook K. B., Lindley J. A., Mayor D. J. (2022). Red pigmentation can be used to reliably distinguish between live Calanus finmarchicus and Calanus glacialis females in the Fram Strait. Front. Mar. Sci. (in review) 9, 906465. doi: 10.3389/fmars.2022.906465
Lund J. W. G., Kipling C., Le Cren E. D. (1958). The inverted microscope method of estimating algal numbers and the statistical basis of estimations by counting. Hydrobiologia 11 (2), 143–170. doi: 10.1007/BF00007865
Møller E. F., Bohr M., Kjellerup S., Maar M., Møhl M., Swalethorp R., et al. (2016). Calanus finmarchicus egg production at its northern border. J. Plankton. Res. 38 (5), 1206–1214. doi: 10.1093/plankt/fbw048
Malzahn A. M., Boersma M. (2012). Effects of poor food quality on copepod growth are dose dependent and non-reversible. Oikos 121 (9), 1408–1416. doi: 10.1111/j.1600-0706.2011.20186.x
Marshall S. M., Orr A. P. (1958). On the biology of Calanus finmarchicus. x. seasonal changes in oxygen consumption. J. Mar. Biol. Assoc. United. Kingdom. 37 (2), 459–472. doi: 10.1017/S002531540002381X
Mauchline J., Blaxter J. H. S., Southward A. J., Tyler P. A. (1998). The biology of calanoid copepods. Academic Press, London. 170 pp
Mayor D. J., Anderson T. R., Irigoien X., Harris R. (2006). Feeding and reproduction of Calanus finmarchicus during non-bloom conditions in the Irminger Sea. J. Plankton. Res. 28 (12), 1167–1179. doi: 10.1093/plankt/fbl047
Mayor D. J., Anderson T. R., Pond D. W., Irigoien X. (2009a). Egg production and associated losses of carbon, nitrogen, and fatty acids from maternal biomass in Calanus finmarchicus before the spring bloom. J. Mar. Syst. 78 (4), 505–510. doi: 10.1016/j.jmarsys.2008.12.019
Mayor D. J., Anderson T. R., Pond D. W., Irigoien X. (2009b). Limitation of egg production in Calanus finmarchicus in the field: A stoichiometric analysis. J. Mar. Syst. 78 (4), 511–517. doi: 10.1016/j.jmarsys.2008.12.020
Mayor D. J., Cook K. B., Thornton B., Atherden F., Tarling G. A., Anderson T. R. (2022). Biomass turnover rates in metabolically active and inactive marine calanoid copepods. Front. Mar. Sci. 9. doi: 10.3389/fmars.2022.907290
Mayor D. J., Cook K. B., Thornton B., Walsham P., Witte U. F. M., Zuur A. F., et al. (2011). Absorption efficiencies and basal turnover of C, N and fatty acids in a marine calanoid copepod. Funct. Ecol. 25 (3), 509–518. doi: 10.1111/j.1365-2435.2010.01791.x
Mayzaud P. (1976). Respiration and nitrogen excretion of zooplankton. IV. the influence of starvation on the metabolism and the biochemical composition of some species. Mar. Biol. 37 (1), 47–58. doi: 10.1007/BF00386778
Melle W., Skjoldal H. R. (1998). Reproduction and development of Calanus finmarchicus, C. glacialis and C. hyperboreus in the Barents Sea. Mar. Ecol. Prog. Series. 169, 211–228. doi: 10.3354/meps169211
Menden-Deuer S., Lessard E. J. (2000). Carbon to volume relatinship for dinoflagellates, diatoms and other protist plankton. Limnol. Oceanogr. 45 (3), 569–579. doi: 10.4319/lo.2000.45.3.0569
Meyer B., Irigoien X., Graeve M., Head R., Harris R. P. (2002). Feeding rates and selectivity among nauplii, copepodites and adult females of Calanus finmarchicus and Calanus helgolandicus. Helgoland. Mar. Res. 56 (3), 169–176. doi: 10.1007/s10152-002-0105-3
Nejstgaard J. C., Frischer M. E., Simonelli P., Troedsson C., Brakel M., Adiyaman F., et al. (2008). Quantitative PCR to estimate copepod feeding. Mar. Biol. 153 (4), 565–577. doi: 10.1007/s00227-007-0830-x
Nejstgaard J. C., Gismervik I., Solberg P. T. (1997). Feeding and reproduction by Calanus finmarchicus , and microzooplankton grazing during mesocosm blooms of diatoms and the coccolithophore Emiliania huxleyi. Mar. Ecol. Prog. Series. 147, 197–217. doi: 10.3354/meps147197
Neukermans G., Oziel L., Babin M. (2018). Increased intrusion of warming Atlantic water leads to rapid expansion of temperate phytoplankton in the Arctic. Global Change Biol. 24 (6), 2545–2553. doi: 10.1111/gcb.14075
Niehoff B. (2004). The effect of food limitation on gonad development and egg production of the planktonic copepod Calanus finmarchicus. J. Exp. Mar. Biol. Ecol. 307 (2), 237–259. doi: 10.1016/j.jembe.2004.02.006
Niehoff B. (2007). Life history strategies in zooplankton communities: The significance of female gonad morphology and maturation types for the reproductive biology of marine calanoid copepods. Prog. Oceanogr. 74 (1), 1–47. doi: 10.1016/j.pocean.2006.05.005
Niehoff B., Klenke U., Hirche H. J., Irigoien X., Head R., Harris R. P. (1999). A high frequency time series at weathership M, Norwegian Sea, during the 1997 spring bloom: The reproductive biology of Calanus finmarchicus. Mar. Ecol. Prog. Series. 176 (1953), 81–92. doi: 10.3354/meps176081
Niehoff B., Madsen S. D., Hansen B. W., Nielsen T. G. (2002). Reproductive cycles of three dominant Calanus species in Disko Bay, West Greenland. Mar. Biol. 140 (3), 567–576. doi: 10.1007/s00227-001-0731-3
Niehoff B., Runge J. A. (2003). A revised methodology for prediction of egg production Calanus finmarchicus from preserved samples. J. Plankton. Res. 25 (12), 1581–1587. doi: 10.1093/plankt/fbg104
Nöthig E.-M., Bracher A., Engel A., Metfies K., Niehoff B., Peeken I., et al. (2015). Summertime plankton ecology in Fram Strait–a compilation of long- and short-term observations. Polar. Res. 34 (1), 23349. doi: 10.3402/polar.v34.23349
Ohman M. D., Runge J. A. (1994). Sustained fecundity when phytoplankton resources are in short supply: Omnivory by Calanus finmarchicus in the Gulf of St. Lawrence. Limnol. Oceanogr. 39 (1), 21–36. doi: 10.4319/lo.1994.39.1.0021
Pasternak A. F., Arashkevich E. G., Grothe U., Nikishina A. B., Solovyev K. A. (2013). Different effects of increased water temperature on egg production of Calanus finmarchicus and C. glacialis. Mar. Biol. 53 (5), 547–553. doi: 10.1134/S0001437013040085
Peter K. H., Sommer U. (2012). Phytoplankton cell size: Intra- and interspecific effects of warming and grazing. PloS One 7, (11). doi: 10.1371/journal.pone.0049632
Plourde S., Runge J. A. (1993). Reproduction of the planktonic copepod Calanus finmarchicus in the lower St Lawrence estuary: Relation to the cycle of phytoplankton production and evidence for a Calanus pump. Mar. Ecol. Prog. Series. 102 (3), 217–228. doi: 10.3354/meps102217
Poulet S. A., Ianora A., Laabir M., Klein Breteler W. C. M. (1995). Towards the measurement of secondary production and recruitment in copepods. ICES. J. Mar. Sci. 52 (3–4), 359–368. doi: 10.1016/1054-3139(95)80051-4
Ray J. L., Althammer J., Skaar K. S., Simonelli P., Larsen A., Stoecker D., et al. (2016a). Metabarcoding and metabolome analyses of copepod grazing reveal feeding preference and linkage to metabolite classes in dynamic microbial plankton communities. Mol. Ecol. 25 (21), 5585–5602. doi: 10.1111/mec.13844
Ray J. L., Skaar K. S., Simonelli P., Larsen A., Sazhin A., Jakobsen H. H., et al. (2016b). Molecular gut content analysis demonstrates that Calanus grazing on Phaeocystis pouchetii and Skeletonema marinoi is sensitive to bloom phase but not prey density. Mar. Ecol. 542, 63–77. doi: 10.3354/meps11560
R Core Team (2021). R core team, (2021). r: A language and environment for statistical computing (Vienna, Austria: R Foundation for Statistical Computing). Available at: http://www.R-project.org.
Redfield A. C. (1958). The biological control of chemical factors in the environment. Am. Sci. 46 (3), 205–221. doi: 10.5194/bg-11-1599-2014
Rey C., Carlotti F., Tande K. S., Hygum B. H. (1999). Egg and faecal pellet production of Calanus finmarchicus females from controlled mesocosms and in situ populations: influence of age and feeding history. Mar. Ecol. Prog. Series. 188, 133–148. doi: 10.3354/meps188133
Rey-Rassat C., Irigoien X., Harris R., Carlotti F. (2002). Energetic cost of gonad development in Calanus finmarchicus and C. helgolandicus. Mar. Ecol. Prog. Series. 238, 301–306. doi: 10.3354/meps238301
Richardson K., Jónasdóttir S. H., Hay S. J., Christoffersen A. (1999). Calanus finmarchicus egg production and food availability in the Faroe-Shetland Channel. and northern North Sea: October-march. Fish. Oceanogr. 8 (1), 153–162. doi: 10.1046/j.1365-2419.1999.00007.x
Roncalli V., Turner J. T., Kulis D., Anderson D. M., Lenz P. H. (2016). The effect of the toxic dinoflagellate Alexandrium fundyense on the fitness of the calanoid copepod Calanus finmarchicus. Harmful. Algae. 51, 56–66. doi: 10.1016/j.pediatrneurol.2015.01.016.Pathophysiology
Runge J. A. (1984). Egg production of the marine, planktonic copepod, Calanus Pacificus Irodsky: Laboratory observations. J. Exp. Mar. Biol. Ecol. 74 (1), 53–66. doi: 10.1016/0022-0981(84)90037-6
Runge J. A., Plourde S. (1996). Fecundity characteristics of Calanus finmarchicus in coastal waters of eastern Canada. Ophelia 44 (1–3), 171–187. doi: 10.1080/00785326.1995.10429846
Søreide J. E., Falk-Petersen S., Hegseth E. N., Hop H., Carroll M. L., Hobson K. A., et al. (2008). Seasonal feeding strategies of Calanus in the high-Arctic Svalbard region. Deep-Sea. Res. Part II.: Topical. Stud. Oceanogr. 55 (20–21), 2225–2244. doi: 10.1016/j.dsr2.2008.05.024
Sakshaug E. (2004). “Primary and secondary production in the Arctic seas,” in The organic carbon cycle in the Arctic Ocean, Springer, Berlin, Heidelberg, 57–81. doi: 10.1007/978-3-642-18912-8_3
Sargent J. R., Falk-Petersen S. (1988). The lipid biochemistry of calanoid copepods. Hydrobiologia 167 (1), 101–114. doi: 10.1007/BF00026297
Smith S. L. (1990). Egg production and feeding by copepods prior to the spring bloom of phytoplankton in Fram Strait, Greenland Sea. Mar. Biol. 106, 59–69. doi: 10.1007/BF02114675
Smith S. L., Lane P. V. Z. (1988). Grazing of the spring diatom bloom in the new York bight by the calanoid copepods Calanus finmarchicus, Metridia lucens and Centropages typicus. Continental. Shelf. Res. 8 (5–7), 485–509. doi: 10.1016/0278-4343(88)90065-9
Straile D. (1997). Gross growth efficiencies of protozoan and metazoan zooplankton and their dependence on food concentration, predator-prey weight ratio, and taxonomic group. Limnol. Oceanogr. 42 (6), 1375–1385. doi: 10.4319/lo.1997.42.6.1375
Swalethorp R., Kjellerup S., Dünweber M., Nielsen T. G., Møller E. F., Rysgaard S., et al. (2011). Grazing, egg production, and biochemical evidence of differences in the life strategies of Calanus finmarchicus, C. glacialis and C. hyperboreus in Disko Bay, Western Greenland. Mar. Ecol. Prog. Series. 429, 125–144. doi: 10.3354/meps09065
Tande K. S. (1982). Ecological investigations on the zooplankton community of Balsfjorden, northern Norway: generation cycles, and variations in body weight and body content of carbon and nitrogen related to overwintering and reproduction in the copepod Calanus finmarchicus. J. Exp. Mar. Biol. Ecol. 62, 129–142. doi: 10.1016/0022-0981(82)90087-9
Tarling G. A., Freer J. J., Banas N. S., Belcher A., Blackwell M., Castellani C., et al. (2022). Can a key boreal Calanus copepod species now complete its life-cycle in the Arctic? evidence and implications for Arctic food-webs. Ambio 51 (2), 333–344. doi: 10.1007/s13280-021-01667-y
Teegarden G. J., Campbell R. G., Anson D. T., Ouellett A., Westman B. A., Durbin E. G. (2008). Copepod feeding response to varying alexandrium spp. cellular toxicity and cell concentration among natural plankton samples. Harmful. Algae. 7 (1), 33–44. doi: 10.1016/j.hal.2007.05.010
Thomas D. N., Arévalo-Martínez D. L., Crocket K. C., Große F., Grosse J., Schulz K., et al. (2022). A changing Arctic Ocean. Ambio 51 (2), 293–297. doi: 10.1007/s13280-021-01677-w
Wickham H. (2016). ggplot2: Elegant graphics for data analysis (New York: Springer-Verlag). Available at: https://ggplot2.tidyverse.org.
Keywords: arctic, copepods, reproduction, climate change, life history, zooplankton physiology
Citation: Jenkins HE, Atherden F, Cook KB, Anderson TR, Thornton B, Mitchell E, Jacob E and Mayor DJ (2022) Grazing, egg production and carbon budgets for Calanus finmarchicus across the Fram Strait. Front. Mar. Sci. 9:981461. doi: 10.3389/fmars.2022.981461
Received: 29 June 2022; Accepted: 25 August 2022;
Published: 20 September 2022.
Edited by:
Hiroaki Saito, The University of Tokyo, JapanCopyright © 2022 Jenkins, Atherden, Cook, Anderson, Thornton, Mitchell, Jacob and Mayor. This is an open-access article distributed under the terms of the Creative Commons Attribution License (CC BY). The use, distribution or reproduction in other forums is permitted, provided the original author(s) and the copyright owner(s) are credited and that the original publication in this journal is cited, in accordance with accepted academic practice. No use, distribution or reproduction is permitted which does not comply with these terms.
*Correspondence: Holly E. Jenkins, aG9sbHlqZW5raW5zQHNvdG9uLmFjLnVr