- 1Ocean school, Yantai University, Yantai, China
- 2The Key Laboratory of Mariculture, Ministry of Education, Ocean University of China, Qingdao, China
Estivation is a widespread survival strategy for dealing with adverse environmental conditions such as high temperature, low oxygen and lack of water or food, which has been reported across multiple vertebrate and invertebrate species. The sea cucumber Apostichopus japonicus is an excellent model organism to investigate the adaptive mechanism of estivation in marine invertebrates. In this study, a metabolomics approach based on ultraperformance liquid chromatography coupled with electrospray ionization time-of-flight mass spectrometry (UPLC-ESI-Q-TOF/MS) was performed to reveal the metabolic response of intestines from adult A. japonicus over the annual estivation-arousal cycle: nonestivation (NA), deep-estivation (DA) and arousal from estivation (AA). A total of 424 metabolites were identified, and among them, 243, 238 and 37 significant differentially metabolites (DMs) were further screened in the comparisons of DA vs. NA, AA vs. DA, and AA vs. NA. Specifically, the levels of metabolites involved in glycolysis and the tricarboxylic acid cycle were significantly decreased, while higher amounts of long-chain fatty acids, phospholipids and free amino acids were found in A. japonicus during estivation, implying that sea cucumbers might reorganize metabolic priorities for ATP production by depressing carbohydrate metabolism and promoting lipid and amino acid catabolism. Interestingly, elevated relative carbon flow entry into the pentose phosphate pathway and accumulation of various nonenzymatic antioxidant molecules (e.g., tocotrienols, folic acid, catechin, genistein and resveratrol) were observed in estivating sea cucumbers, which suggested that enhancement of the reactive oxygen species defense system might promote long-term viability in the hypometabolic state in an energy-efficient manner. Thus, this research provides new insights into the adaptation mechanisms of marine invertebrates to estivation at the metabolic level.
1 Introduction
Changing environmental conditions are always a major challenge to living creatures, yet global environmental change in recent years has made it even more intractable. Entering a hypometabolic state (e.g., hibernation, estivation, diapause and anaerobiosis) is an important survival strategy for many species when challenged by environmental stress (Storey and Storey, 2007). Interestingly, a number of previous studies have revealed that these environment-induced hypometabolism phenomena coincidentally shared several common metabolic regulation strategies highly conserved across phylogenic lines, including global metabolic rate depression, altered energy use strategies of fuel sources and metabolic priorities, transcriptional changes, enhancement of defense systems and cellular homeostasis (Heldmaier et al., 2004; Storey and Storey, 2012).
Estivation, as a state of aerobic hypometabolism, is a widespread survival strategy dealing with adverse environmental conditions such as high temperature, low oxygen and lack of water or food, which has been reported across multiple terrestrial and freshwater vertebrate and invertebrate species (Storey and Storey, 2012). However, estivation also occurs in marine organisms, which have not been well studied thus far. The sea cucumber Apostichopus japonicus, as an excellent model organism of marine invertebrate estivator, retreats into dormancy in summer annually when the ambient water temperature is above approximately 25 °C without feeding or locomotion in a period that lasts up to 100 days (Li et al., 1996). During this torpor period, sea cucumbers endure profound metabolic rate suppression, immune system modification and severe atrophy of the digestive tract, along with a loss of over one-third of their initial body mass. Since A. japonicus is one of the most important economic species that is widely cultured in Asia, mainly due to its high nutritional and pharmaceutical value, estivation obviously shortens the growth cycle and drives down the production efficiency in the sea cucumber aquaculture industry (Yuan et al., 2007a). Recently, accumulating studies have focused on the physiological and biochemical strategies of sea cucumber in dealing with estivation, including metabolic rate depression (Yang et al., 2006; Xiang et al., 2016), energetic reorganization (Yuan et al., 2007b), and degeneration of intestinal structure and function (Gao et al., 2008). With the rapid development of omics technology, the molecular regulation mechanisms underpinning estivation have been further elucidated, mainly focusing on global transcriptional and translational regulation (Zhao et al., 2014; Chen et al., 2016a; Li et al., 2018), miRNA-targeted posttranscriptional modifications (Chen et al., 2013; Chen et al., 2018), reversible protein phosphorylation (Chen et al., 2016b) and epigenetic modifications (Zhao et al., 2015; Yang et al., 2020).
Metabolites drive essential cellular functions in interactions between organisms and the environment. Metabolomics, as an important omics technique in systems biology, permits the identification and quantification of multiple metabolic compounds, which could provide deep insight into the adaptive regulatory mechanisms of biochemical pathways in response to environmental changes and autophysiological state shifts (Weckwerth, 2003; Johnson et al., 2016). Currently, the main methodologies that are widely applied in metabolomics analysis include high-performance liquid chromatography (HPLC), nuclear magnetic resonance (NMR), gas chromatography–mass spectrometry (GC–MS) and liquid chromatography–mass spectrometry (LC–MS). Among these analytical techniques, ultraperformance liquid chromatography coupled with electrospray ionization time-of-flight mass spectrometry (UPLC-ESI-Q-TOF/MS) has become an important means for metabolomics studies in multiple areas of sea cucumber research because of its high sensitivity, high separation efficiency, good repeatability and high universality (Li et al., 2019; Zhao et al., 2020; Xing et al., 2021; Guo et al., 2022). However, little is known about the metabolic response in adult A. japonicus across complete natural torpor-arousal cycles. In the present study, UPLC-ESI-Q-TOF/MS was performed to reveal the metabolic profile of the sea cucumber A. japonicus across the estivation period. This work aimed to (1) investigate the physiological metabolic transition during the estivation cycle, (2) identify the key differential metabolites and pathways during estivation, and (3) provide new insight into the potential molecular mechanisms of estivation and assist in guiding the aquaculture management of sea cucumbers.
2 Materials and methods
2.1 Animals
Adult A. japonicus (80-120 g body wet weight) were obtained from the coast of Yantai (Yantai, China) in May, August and October 2021. These three phases of sea cucumbers were sampled across the active-estivation-arousal cycle: (1) nonestivating sea cucumbers (NA) as the control group from seawater temperature approximately 17°C with normal feeding and activity; (2) deep-estivation sea cucumbers (DA) from seawater at a temperature of approximately 26°C, as indicated by no signs of feeding or locomotion and the degeneration and atrophy of the intestine into a very tiny string; and (3) arousal from estivation sea cucumbers (AA) from seawater at a temperature of approximately 20°C with obvious feeding and movement and recovery of the intestinal structure and contents, the pictures of the intestinal tissues in three stages of the active-estivation-arousal cycle were shown in Figure S1. A total of 17 individuals were sacrificed and the intestinal tissue and contents were rapidly dissected out and frozen in liquid nitrogen for later analysis.
2.2 Metabolite extraction
Six individuals for NA group, six individuals for AA group and five individuals for DA group were used for metabolomics analysis. Each sample (~100 mg) was accurately weighed, and 0.6 mL precooled 2-chlorophenylalanine (4 ppm) methanol ( ± 1%) was added to a 2 mL EP tube. After vortexing for 30 seconds, 100 mg glass beads were added to the samples and ground for 90 s at 55 Hz by a tissue grinder. Then, the samples were ultrasonicated at room temperature for 10 minutes and centrifuged at 12000 rpm at 4 °C for 10 min to obtain 200 µL supernatant, which was then filtered through a 0.22 µm membrane for LC–MS analysis. Of note, 20 µL filtrates from each sample were pooled as the quality control (QC) to monitor deviations of the analytical results.
2.3 LC–MS analysis
The metabolic profiling analysis was conducted on a Thermo Vanquish system equipped with an ACQUITY UPLC® HSS T3 (150×2.1 mm, 1.8 µm, Waters) column maintained at 40°C. The temperature of the auto sampler was 8 °C. Gradient elution of analytes was carried out with 0.1% formic acid in water (A2) and 0.1% formic acid in acetonitrile (B2) in positive mode or 5 mM ammonium formate in water (A3) and acetonitrile (B3) in negative mode at a flow rate of 0.25 mL/min. Injection of 2 μL of each sample was performed after equilibration. An increasing linear gradient of solvent B2/B3 (v/v) was used as follows: 0~1 min, 2% B2/B3; 1~9 min, 2%~50% B2/B3; 9~12 min, 50%~98% B2/B3; 12~13.5 min, 98% B2/B3; 13.5~14 min, 98%~2% B2/B3; 14~20 min, 2% B2-positive model (or 14~17 min, 2% B3-negative model).
The ESI-MSn experiments were executed on a Thermo Q Exactive HF-X mass spectrometer with spray voltages of 3.5 kV and -2.5 kV in positive and negative modes, respectively. The sheath gas and auxiliary gas were set at 30 and 10 arbitrary units, respectively. The capillary temperature was 325 °C. The analyzer scanned over a mass range of m/z 81-1 000 for full scan at a mass resolution of 60000. Data-dependent acquisition (DDA) MS/MS experiments were performed with an HCD scan. The normalized collision energy was 30 eV. Dynamic exclusion was implemented to remove some unnecessary information from the MS/MS spectra.
2.4 Data processing
The raw MS data were converted into extensible markup language (mzXML) format by using the software ProteoWizard, and then the cryptographic message syntax (XCMS) program of the R language package was used for peak alignment, retention time correction, and peak area extraction to obtain the data matrix, including mass to charge ratio (m/z), retention time and intensity. The structure of metabolites was further identified by accurate mass matching (molecular weight error ≤30 ppm) and MS/MS data matching based on the online Human Metabolome Database (HMDB) (http://www.hmdb.ca), METLIN (http://metlin.scripps.edu), Massbank (http://www.massbank.jp/), LipidMaps (http://www.lipidmaps.org) and mzClound (https://www.mzcloud.org).
To clarify the relationships of the identified metabolites of sea cucumbers among different groups, principal component analysis (PCA) was applied using the R language package Phyloseq to reduce the data dimensionality. Supervised orthogonal projections for latent structures-discriminant analysis (OPLS-DA) were further performed by the R language package MetaboAnalystR (v1.0.1), and 200 permutation tests were performed to verify the reliability of the model.
2.5 Statistical analysis
For the metabolomics analysis, differential metabolites (DMs) between groups were determined by Student’s t test based on p value ≤0.05, combined with absolute Log2FC (fold change) ≥1 and variable importance in projection (VIP) values ≥1 from the OPLS-DA model. Venn plot was further constructed by jvenn online tool (http://jvenn.toulouse.inra.fr/app/example.html). The DMs were further annotated and mapped to the KEGG compound and pathway databases (http://www.kegg.jp/kegg/compound/ and http://www.kegg.jp/kegg/pathway.html) using a hypergeometric distribution test using the R language package MetaboAnalystR to identify the significantly enriched pathways, where p < 0.05 was considered to be significant.
3 Results and discussion
3.1 LC–MS metabolomic profiles
In this study, we employed UPLC-ESI-Q-TOF/MS-based metabolomics analysis to explore the potential biochemical regulatory mechanism of estivation. Quality control samples were used to evaluate the signal drift of the entire mass spectrometry data during the acquisition process. In the present study, five QC samples showed dense distribution, suggesting the good correction effectiveness of the PCA model, and no obvious outliers were found in the two-dimensional PCA score plot (Figure S2). A total of 424 metabolites were identified in the intestines of A. japonicus. Figure 1A represents the relative abundances of the top 20 metabolites in each sample between the different groups. Since some metabolites play specific roles in an organism, the identified metabolites were further classified into eight categories based on KEGG analysis, including peptides, lipids, organic acids, carbohydrates, nucleic acids, vitamins and cofactors, steroids, and hormones and transmitters (Figure 1B). The results indicated that higher amounts of peptides, steroids, vitamins and cofactors were identified at the DA stage in comparison with the NA and AA stages, while lipids, carbohydrates and organic acids all showed smaller percentages at the DA stage than at the other stages. The increases in vitamins and cofactors might be helpful for the regulation of substance and energy metabolism since many vitamins act as critical cofactors for enzymatic reactions in metabolic processes (Huskisson et al., 2007). As reported previously, strong depression of the metabolic rate is a vital adaptive strategy targeting the energy budget during hypometabolism; hence, a decline in catabolism usually occurs during estivation (Storey and Storey, 1990; Storey and Storey, 2010). In this study, obvious decreases in the contents of carbohydrates and lipids were found in the DA group, consistent with previous results in A. japonicus during estivation (Xiang et al., 2016; Yang et al., 2021), suggesting that some necessary controls might be implemented for energy reorganization during dormancy.
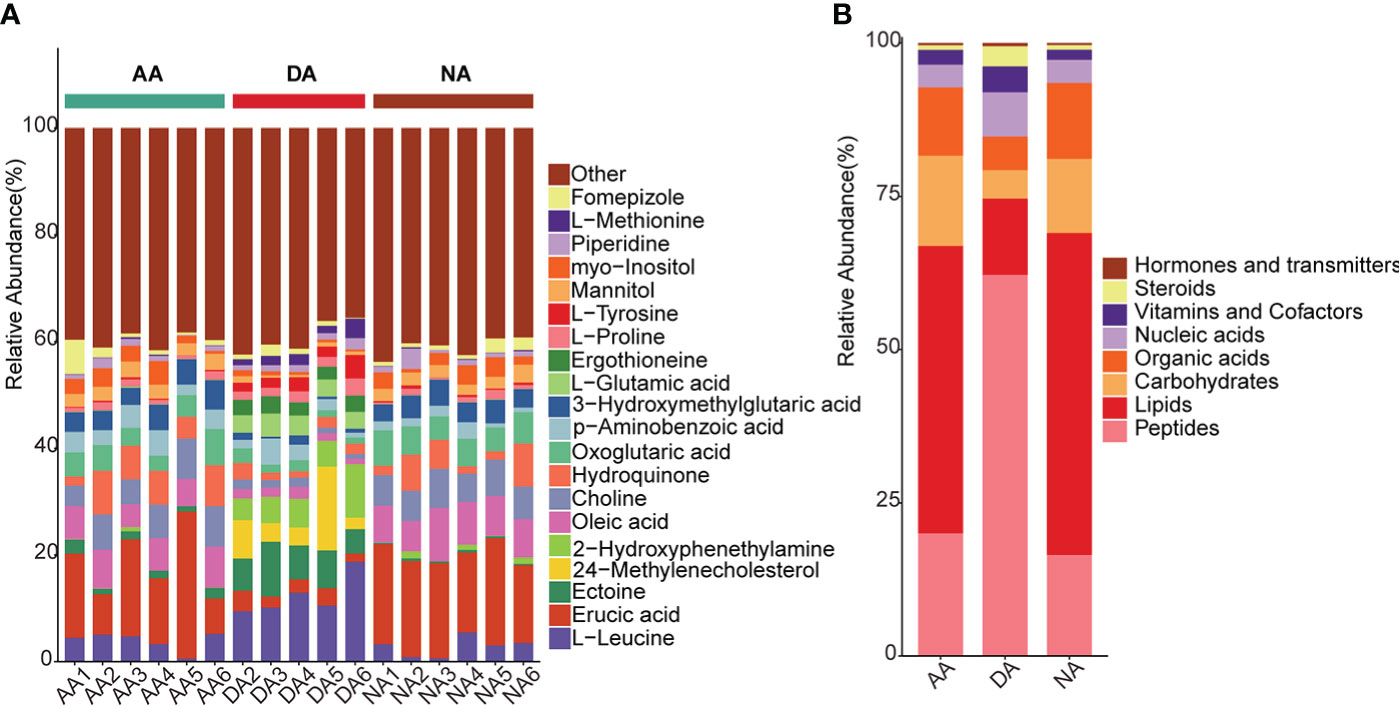
Figure 1 The annotated metabolites in A. japonicus during estivation cycles. (A) the relative abundance of top 20 metabolites in each group, (B) the relative abundance of the annotated metabolites in KEGG database in distribution of eight categories.
PCA was applied to determine the metabolic variations in all groups (NA, DA, and AA), and the score plot is shown in Figure 2A. As indicated in the figure, the metabolites in each group had relatively good aggregation. Furthermore, the first principal axis (PC1) showed 53.7% variability, and the metabolites of A. japonicus in the dormant physiological state (DA) showed clear separation from those in the normal physiological state (NA and AA), suggesting that hypometabolism, such as estivation, could cause obvious metabolic phenotype alterations in the intestine of A. japonicus. Since PCA is an unsupervised algorithm without eliminating random and intragroup errors (Saccenti et al., 2014), a supervised model of OPLS-DA was further conducted to analyze the differences in metabolites between the three groups. As shown in Figure 2B, the OPLS-DA score plot revealed that the three groups (NA, DA, and AA) each clustered according to their physiological state with no overlap, indicating that sea cucumbers showed diverse metabolomic profiles under their various physiological conditions. The permutation tests have provided good model validation and accuracy of OPLS-DA analysis (Figure S3).
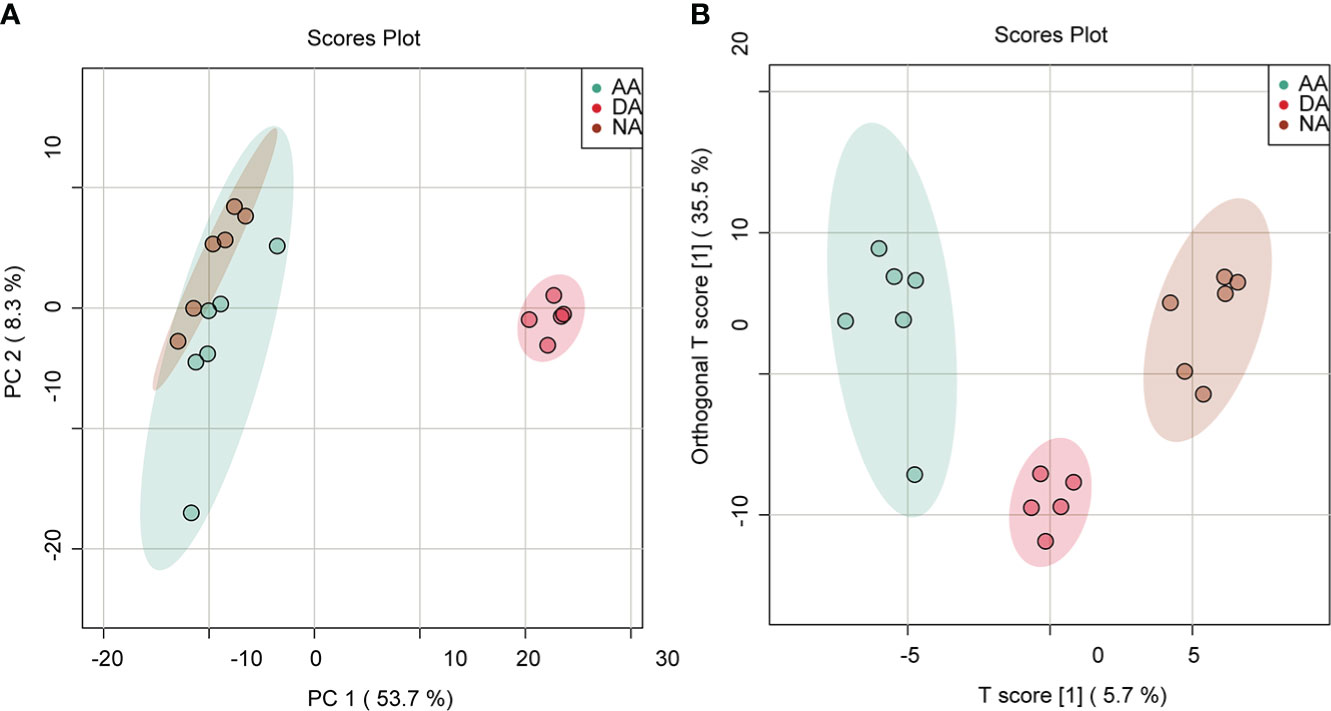
Figure 2 PCA and OPLS-DA score plot from the LC–MS intestinal metabolite profiles in A. japonicus during estivation cycles. (A) PCA score plot, (B) OPLS-DA score plot.
3.2 Differential metabolite analysis
In this study, significant differential metabolites (DMs) were screened by thresholds with VIP ≥1 (from the OPLS-DA model), Log2FC ≥1 (fold change) and p value ≤0.05 (based on Student’s t test). As shown in Figure 3A, 243 DMs (129 upregulated DMs and 114 downregulated DMs) were identified in DA vs. NA, 238 DMs (100 upregulated DMs and 138 downregulated DMs) were identified in AA vs. DA, and 37 DMs (21 upregulated DMs and 16 downregulated DMs) were identified in AA vs. NA. These differential metabolites are listed in Supplementary Tables S1-S3. Among these differentially abundant metabolites, there were 13 overlapping DMs in three pairwise comparisons, while 63 (25.93%), 54 (22.69%), and 10 (27.03%) DMs were exclusively screened in DA vs. NA, AA vs. DA, and AA vs. NA, respectively (Figure 3B). To reveal the physiological response and metabolomic adaptation mechanisms during estivation-arousal cycles of A. japonicus, we listed the important differential metabolites in DA vs. NA and AA vs. DA in Table 1, including a wide range of carbohydrates, lipids, amino acids, nucleic acids and vitamins. To illustrate the possible metabolomic adaptation mechanism of A. japonicus during estivation, the relationships among the important DMs are summarized in Figure 4.
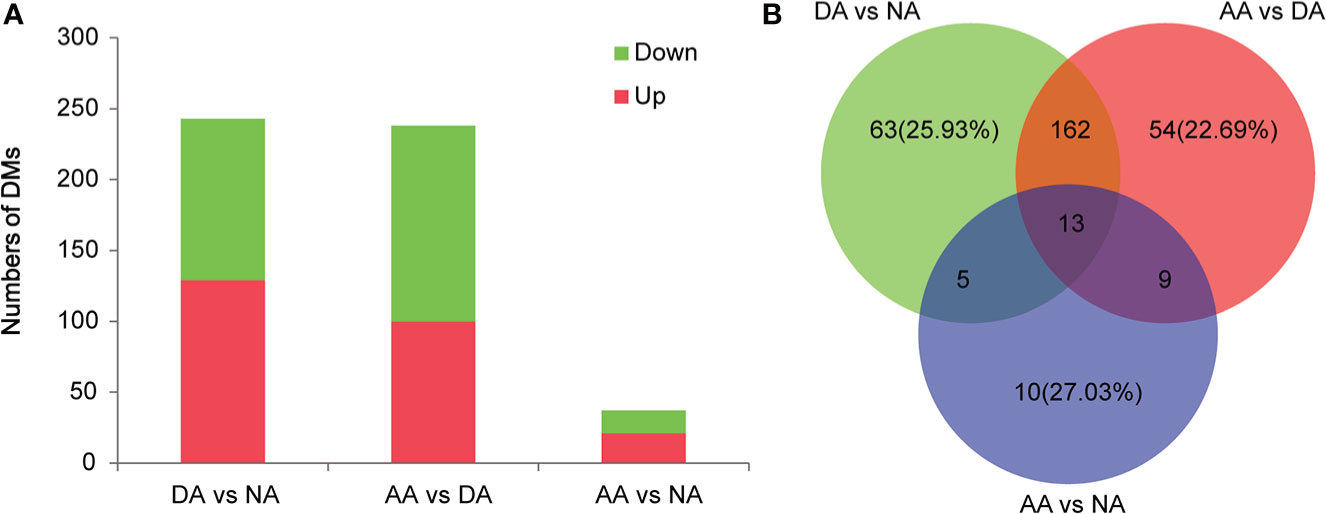
Figure 3 Differential metabolites identified in A. japonicus during estivation cycles. (A) The numbers of differential metabolites in the comparisons of DA vs. NA, AA vs. DA, and AA vs. NA, (B) Venn diagram of differential metabolites pairwise comparison.
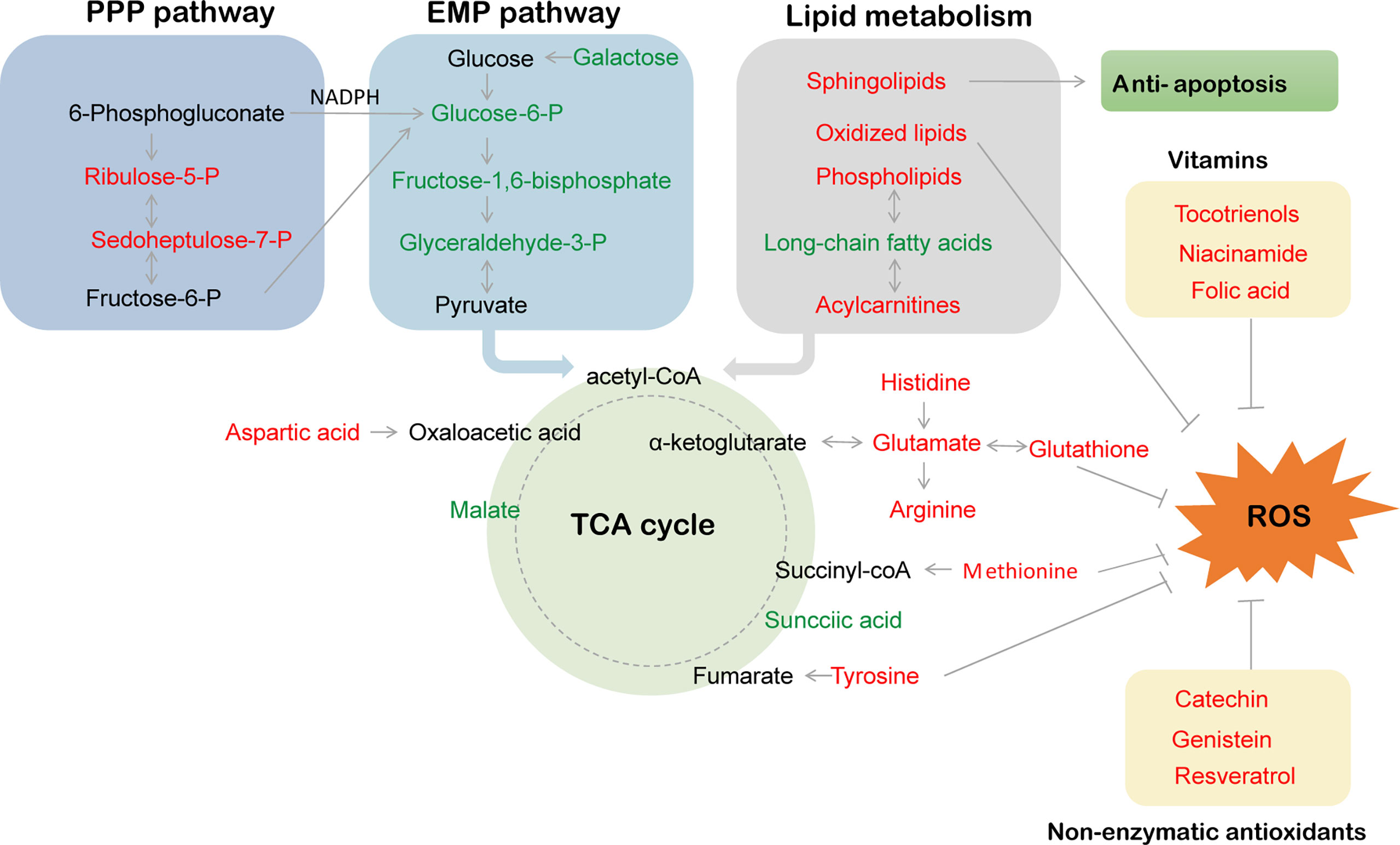
Figure 4 The hypothetical metabolic response map during estivation cycles. PPP pathway, EMP pathway and ROS respectively represent pentose-phosphate pathway, embden meyerhof pathway and reactive oxide species.
3.2.1 Glycolysis, TCA cycle and pentose phosphate pathway
For estivators, dormancy is characterized by metabolic rates lowered to at least 30% compared with those in active animals (Pedler et al., 1996), which requires a complex metabolic ordination of reactions involving both ATP-utilizing and ATP-generating pathways. Since glycolysis is an essential energy-yielding pathway by all cells in animals, regulation of glycolysis is an important part of metabolic depression during estivation (Brooks and Storey, 1997). A previous study of the estivating land snail Otala lactea showed the reduced activity levels of key enzymes in glycolysis pathways, including hexokinase, phosphofructokinase, phosphoglycerate kinase and lactate dehydrogenase (Brooks and Storey, 1990). For A. japonicus, similar depression of glycolytic rates also occurs in the hypometabolic state. Xiang et al. (2016) found significant decreases in glucose and pyruvic acid concentrations in the intestines of estivating A. japonicus, along with reduced enzymatic activity and transcription levels of the rate-limiting enzyme hexokinase. In our study, the levels of the glycolysis precursor or the intermediate metabolites D-galactose, fructose 1,6-bisphosphate, and D-glyceraldehyde 3-phosphate were all significantly decreased in the DA group in comparison with the NA and AA groups, verifying that profound glycolytic suppression occurred in estivating A. japonicus. In addition, the current study showed that the levels of characteristic intermediate metabolites in the citric acid cycle (TCA cycle), such as succinic acid and malate, were also decreased in the DA group in comparison with the other groups, which was consistent with previous results of Chen et al. (2016a) and Yang et al. (2021), indicating decelerations in the rates of TCA cycle turnover and oxidative phosphorylation in the hypometabolic state.
Interestingly, the content levels of several metabolites involved in the pentose phosphate pathway (including ribulose-5-phosphate, ribose 1-phosphate, sedoheptulose 7-phosphate and meso-2,6-diaminoheptanedioate) were significantly increased in the intestines of the DA group in comparison with the NA and AA groups, indicating an elevated relative carbon flow entry into the pentose phosphate pathway in estivating A. japonicus. Likewise, Ramnanan and Storey (2006) reported that estivation could induce a highly phosphorylated glucose-6-phosphate dehydrogenase with greater structural enzymatic stability in O. lactea, which further enhanced carbohydrate flow through the pentose phosphate cycle. Indeed, the pentose phosphate pathway has an essential role in the production of reducing power in the form of NADPH for various antioxidant defense and detoxification reactions (DiGiulio et al., 1989; Das and White, 2002). Hence, preferential carbohydrate flow through the pentose phosphate cycle might favor NADPH production, supplying reduction equivalents for the antioxidant system in response to high oxidative stress induced by estivation (Ramnanan and Storey, 2006).
3.2.2 Lipid metabolism
Many mammals typically rely on the oxidation of prestored lipids for energy production during hibernation (Storey, 2002), and there is accumulating evidence indicating that many estivators also utilize lipids as an important fuel source during dormancy Jones, 1980; Frick et al., 2008). For the sea cucumber A. japonicus, a wide array of long-chain fatty acids (e.g., arachidic acid, eicosadienoic acid, oleic acid and linoleic acid) showed decreased amounts, yet significant increases in some acylcarnitines (e.g., palmitoyl-L-carnitine, propionylcarnitine and butyryl-L-carnitine) during estivation, suggesting an increased reliance on fatty acid oxidation to support the energy need during hypometabolism, which is consistent with the results of the estivating African lungfish Protopterus dolloi, where both 3-hydroxyacyl CoA dehydrogenase (HOAD) and carnitine palmitoyl CoA transferase (CPT) activities were still sustained in the liver despite the 42% reduction in CCO activity during estivation (Frick et al., 2008).
Phospholipids are an essential component of the cell membrane and function in membrane dynamics, protein regulation, signal transduction and secretion. Research shows that phospholipids predominate in the composition of lipids in sea cucumber, accounting for 90% percent of the total fat content (Fan, 2001). In our present study, some kinds of phospholipids and their derivatives (e.g., LysoSM(d18:1), phosphorylcholine and beta-glycerophosphoric acid) were significantly elevated in sea cucumber intestine during deep estivation, which further confirms previous findings reported by Chen et al. (2016a) and Zhao et al. (2014) that upregulations in gene transcription and protein translation were involved in phospholipid catabolic processes in A. japonicus intestines during estivation (e.g., phospholipase, proactivator polypeptide, glycerol kinase, and group XV phospholipase A2), implying that membrane lipid catabolism may also be a major fuel source of energy. Moreover, the increases in phospholipids and cholesterols might enhance the stability of the basic structure of the cell membrane during the dormant period, which is an important adaptive strategy for marine invertebrates in resilience to environmental stress, such as high temperature and hypoxia (Li et al., 2019; Hu et al., 2022).
Remarkably, increased levels of several sphingolipid derivatives (e.g., sphingosine 1-phosphate, N,N-dimethylsphingosine, sphingosine, 3-ketosphingosine and phytosphingosine) were found in estivating sea cucumbers. Sphingolipids play integral roles in membrane domains and signaling, cell proliferation and apoptosis, inflammation and central nervous system development (Quinville et al., 2021). For instance, sphingosine 1-phosphate is a well-known anti-apoptotic regulator that can suppress caspase-3 activity and prevent apoptosis (Rutherford et al., 2013), which suggests that the increased level of sphingosine 1-phosphate during estivation in our present study may perform a protective function by reducing excessive tissue apoptosis. Indeed, inhibition of apoptosis is a key strategy for maintaining long-term viability in a low metabolic state, where the energy expenditure of cell replacement processes must be minimized to prolong survival (Biggar and Storey, 2010). Similar interactions have been described by Chen et al. (2016a) at the protein level, in which mediators of apoptosis were downregulated during deep estivation in sea cucumber. There is growing evidence that sphingolipids also play a role in the cellular response to oxidative stress (Van Brocklyn and Williams, 2012). Other lipids, such as 12-keto-tetrahydro-leukotriene B4 and docosahexaenoic acid, also showed elevated levels in sea cucumbers during estivation. Since leukotriene is an endogenous lipid mediator of inflammation (Le Bel et al., 2014) and docosahexaenoic acid is an antioxidant that can reinforce the antioxidative defense system (Hossain et al., 1999), higher amounts of these metabolites indicate that sea cucumbers were subjected to oxidative and inflammatory stress during estivation. However, the precise roles of these estivation-specific metabolites need to be explored further.
3.2.3 Amino acid and nucleic acid metabolism
Free amino acids are not only directly utilized as fuels by the immune system and energy metabolism but can also be converted to other amino acids or molecules that exert protective effects in organisms under various stresses (Calder, 2006; Chen et al., 2016a; Xu et al., 2017; Huo et al., 2019). In our study, higher amounts of amino acids, namely, L-glutamic acid, beta-tyrosine, L-glycine, L-histidine, L-arginine, L-methionine, L-cystine, L-lysine and L-aspartic acid, were observed at the DA stage than at the NA stage. Among these amino acids, some can also be converted into intermediate metabolites in the TCA cycle and used by cells to produce energy (e.g., tyrosine into fumarate, histidine, arginine and glutamic acid into alpha-ketoglutarate, aspartic acid into oxaloacetic acid, and methionine into succinyl-CoA). In addition, several amino acids are involved in the antioxidant defense system to prevent and repair the deleterious effects from free radicals; for example, tyrosine is an excellent antioxidant inside lipid bilayers and protects cells from oxidative destruction (Moosmann and Behl, 2000); glycine and glutamic acid are integral components of glutathione, which is the major antioxidant molecule of the cell and can effectively scavenge free radicals and other reactive oxygen species and maintain the cellular redox balance (Hensley et al., 2000; Fang et al., 2002); and methionine is easily oxidized to methionine sulfoxide by many reactive species, thus serving to protect other functionally essential residues from oxidative damage (Levine et al., 2010). Furthermore, another investigation of estivating Chilo partellus larvae reported increased amounts of glycine, histidine, arginine, proline, tyrosine, and methionine, which may be constituents of heat-shock proteins to enhance stress tolerance and maintain development (Tanwar et al., 2021). Hence, the elevated levels of the above amino acids in estivating A. japonicus intestines could meet the greater energy requirements for dealing with oxidative stress and heat-shock proteins during torpor.
Additionally, various metabolites associated with nucleotide metabolism, such as pyrimidine ectoine, hypoxanthine, thioguanine and uridine, were significantly changed at the DA stage, as well as a series of mononucleotides (e.g., adenosine monophosphate (AMP), guanosine monophosphate (GMP), uridine monophosphate (UMP), cytidine monophosphate (CMP) and deoxythymoadenylate (dTMP)), reflecting that A. japonicus was facing a real energy crisis during estivation.
3.2.4 Vitamins and other physiological responses
Vitamin E is an essential nutrient for organisms that exerts an antioxidant response to reactive oxygen species (ROS) -induced oxidative stress by inhibiting membrane lipid peroxidation and damage to proteins and DNA (Miyazawa et al., 2019). Folic acid is an important, potent antioxidant that is involved in the trans-sulfuration pathway as a coenzyme, increasing glutathione synthesis and acetaldehyde oxidation and preventing glutathione inactivation (Ebaid et al., 2013). Nicotinamide (NAM) is a form of vitamin B3 that can suppress oxidative stress (Wei et al., 2018). In our study, the significantly increased levels of three isomers of the vitamin E family (alpha-tocotrienol, delta-tocotrienol and epsilon-tocopherol), nicotinamide and folic acid in estivating sea cucumbers implied their enhanced antioxidant effect to protect the body from oxidative damage. In addition, several flavanol and isoflavone antioxidants also increased during estivation, such as catechin, genistein and resveratrol (Simioni et al., 2018). In general, aerobic organisms develop antioxidant systems consisting of antioxidant enzymes (e.g., SOD, CAT and glutathione peroxidase) and low-molecular weight compounds (e.g., glutathione, α-tocopherol, and ascorbic acid) to maintain redox balance from ROS-induced oxidative stress. Our unpublished data and previous studies revealed the reduced activities of several antioxidant enzymes, such as catalase, in estivating A. japonicus, the freshwater snail Biomphalaria tenagophila and Helix pomatia (Ferreira et al., 2003; Nowakowska et al., 2009; Liu et al., 2016; Chen et al., 2016a), while the present study indicated that significantly elevated levels of nonenzymatic antioxidant molecules were observed at the DA stage compared to other stages, suggesting that an adjustment strategy of antioxidant defense might be adapted during hypometabolism in an energy-efficient manner.
Spermidine has a variety of effects, including anti-inflammatory, antioxidant, enhanced mitochondrial metabolic and respiration functions, and improved protein stability and chaperone activity (Madeo et al., 2018). Resolvins D1 and D2 are docosahexaenoic acid (DHA)-derived lipoxygenase metabolites that can exhibit anti-inflammatory effects by modulating the activation of T lymphocytes (Chiurchiu et al., 2016). Hence, changes in the contents of the above metabolites during estivation indicated that anti-inflammatory biological protective effects might be exerted in estivating sea cucumbers.
3.3 KEGG pathway enrichment analysis
To further assess the metabolic profiles of A. japonicus intestines during estivation cycles, pathway enrichment analysis was performed based on the identified metabolites that were significantly differentially expressed. In our present study, 58 and 61 pathways were enriched in the DA vs. NA and AA vs. DA groups, respectively. The top 25 pathways are shown in Figure 5. Furthermore, 6 (sphingolipid metabolism, linoleic acid metabolism, pyrimidine metabolism, D-glutamine and D-glutamate metabolism, lysine biosynthesis and pentose phosphate pathway) and 4 (sphingolipid metabolism, phenylalanine metabolism, pyrimidine metabolism, and lysine biosynthesis) pathways were significantly enriched in the DA vs. NA and AA vs. DA comparisons, respectively (p < 0.05). There were 17 key common increased metabolites involved in these significantly enriched pathways, including sphingosine-1-P, sphingosine, phyto-sphingosine, psychosine, 3-dehydro-sphingosine, L-aspartate, L-lysine, L-saccharopine, UMP, CMP, dTMP, uridine, and deoxyuridine. As previously mentioned, sphingolipid metabolism, D-glutamine and D-glutamate metabolism and pentose phosphate pathway were involved with anti-apoptosis and antioxidant defense, while linoleic acid metabolism and pyrimidine metabolism were involved with energy metabolism, suggesting that sea cucumbers might reorganize metabolic priorities for ATP production and enhance defense mechanisms by these key pathways to promote long-term viability in a hypometabolic state. However, further studies are required to elucidate the specific functions of these pathways in echinoderms during estivation.
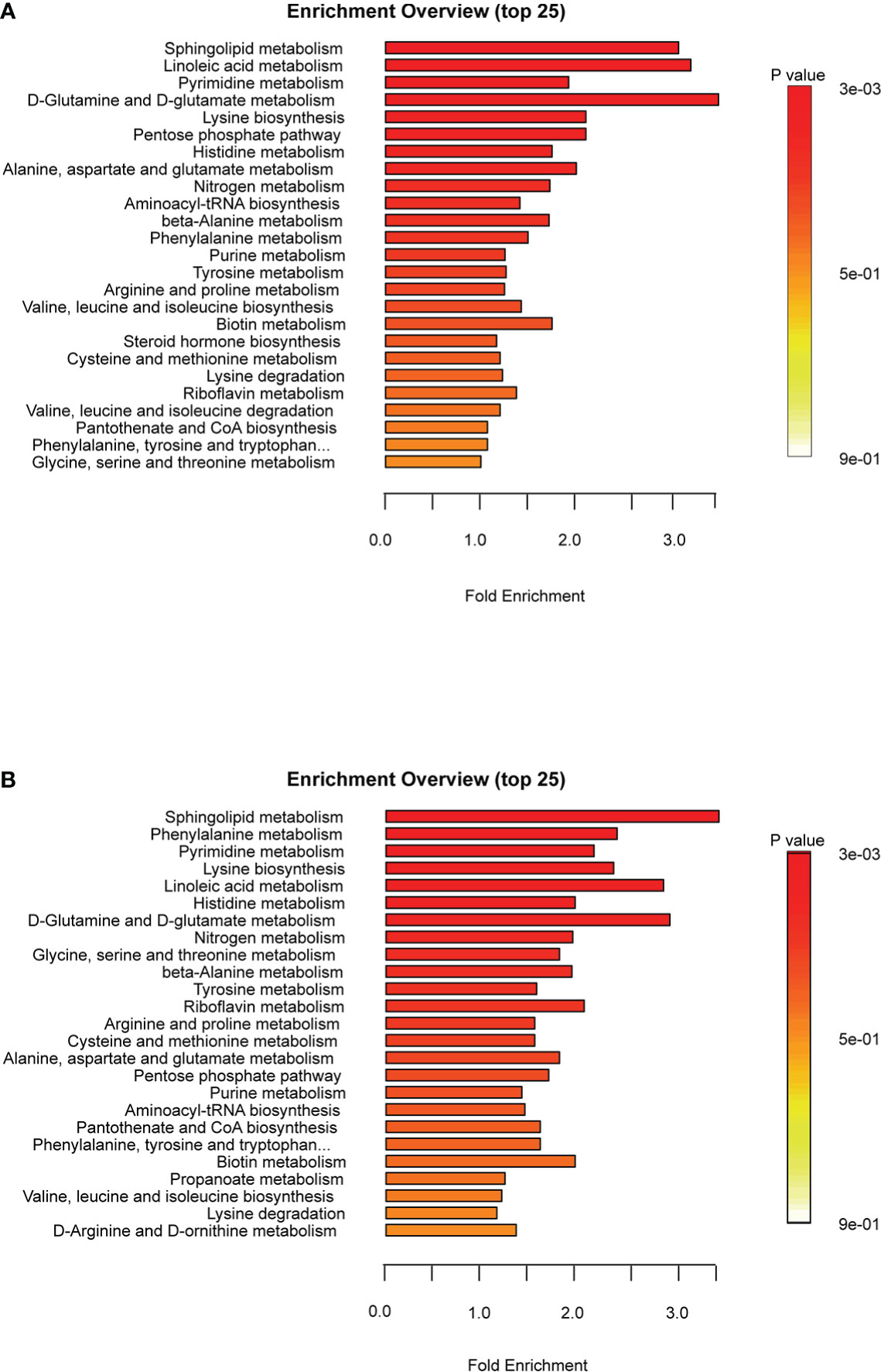
Figure 5 Metabolic pathways analysis of A. japonicus intestines in the comparisons of DA vs. NA (A) and AA vs. DA (B).
In conclusion, our present metabolomics analysis demonstrated that sea cucumbers may meet the challenge of summer estivation by reorganizing metabolic priorities for ATP production (e.g., depressing carbohydrate metabolism and promoting lipid and amino acid catabolism) and enhancing antioxidant defenses in an energy-efficient manner to promote long-term viability. The present study identified a series of key differential metabolites and pathways and explored the potential metabolic strategies of A. japonicus during estivation, which will provide new insights into the adaptation mechanisms of estivation in marine invertebrates.
Data availability statement
The original contributions presented in the study are included in the article/Supplementary Material. Further inquiries can be directed to the corresponding author.
Author contributions
YZ: Conceptualization, Methodology, Writing- Original draft preparation. HaoW: Investigation, Data Curation. HanW: Visualization, Formal analysis. YP: Writing- Reviewing and Editing. MC: Writing- Reviewing and Editing. All authors contributed to the article and approved the submitted version.
Funding
This work was funded by the National Natural Science Foundation of China (No. 41906098 and 31972767).
Acknowledgments
We are grateful to Wekemo Tech Group Co., Ltd. for assisting in bioinformatic analysis.
Conflict of interest
The authors declare that the research was conducted in the absence of any commercial or financial relationships that could be construed as a potential conflict of interest.
Publisher’s note
All claims expressed in this article are solely those of the authors and do not necessarily represent those of their affiliated organizations, or those of the publisher, the editors and the reviewers. Any product that may be evaluated in this article, or claim that may be made by its manufacturer, is not guaranteed or endorsed by the publisher.
Supplementary material
The Supplementary Material for this article can be found online at: https://www.frontiersin.org/articles/10.3389/fmars.2022.980221/full#supplementary-material
Supplementary Figure 1 | The intestine tissue of A. japonicus during estivation cycles. (A) NA stage, (B) DA stage, (C) AA stage.
Supplementary Figure 2 | PCA diagram of quality control analysis.
Supplementary Figure 3 | The permutation tests of OPLS-DA analysis.
Abbreviations
NA, nonestivation; DA, deep-estivation; AA, arousal from estivation; DMs, differentially metabolites.
References
Biggar K. K., Storey K. B. (2010). The emerging roles of microRNAs in the molecular responses of metabolic rate depression. J. Mol. Cell. Biol 3(3)167–75. doi: 10.1093/jmcb/mjq045
Brooks S., Storey K. B. (1990). Glycolytic enzyme binding and metabolic control in estivation and anoxia in the land snail Otala lactea. J. Exp. Biol. 151, 193–204. doi: 10.1242/jeb.151.1.193
Brooks S., Storey K. B. (1997). Glycolytic controls in estivation and anoxia: a comparison of metabolic arrest in land and marine molluscs. Comp. Biochem. Phys. A. 118, 1103–1114. doi: 10.1016/S0300-9629(97)00237-5
Calder P. C. (2006). Branched chain amino acids and immunity. J. Nutr. 136, 288S–293S. doi: 10.1093/jn/136.1.288S
Chen M., Li X., Zhu A., Storey K. B., Sun L., Gao T., et al. (2016a). Understanding mechanism of sea cucumber Apostichopus japonicus aestivation: insights from TMT-based proteomic study. Comp. Biochem. Physiol. D. Genom. Proteom. 19, 78–89. doi: 10.1016/j.cbd.2016.06.005
Chen M., Wang S., Li X., Storey K. B., Zhang X. (2018). The potential contribution of miRNA-200-3p to the fatty acid metabolism by regulating AjEHHADH during aestivation in sea cucumber. PeerJ 6, e5703. doi: 10.7717/peerj.5703
Chen M., Zhang X., Liu J., Storey K. B. (2013). High-throughput sequencing reveals differential expression of miRNAs in intestine from sea cucumber during aestivation. PloS One 8, e76120. doi: 10.1371/journal.pone.0076120
Chen M., Zhu A., Storey K. B. (2016b). Comparative phosphoproteomic analysis of intestinal phosphorylated proteins in active versus aestivating sea cucumbers. J. Proteom. 135, 141–150. doi: 10.1016/j.jprot.2015.09.016
Chiurchiu V., Leuti A., Dalli J., Jacobsson A., Battistini L., Maccarrone M., et al. (2016). Proresolving lipid mediators resolvin D1, resolvin D2, and maresin 1 are critical in modulating T cell responses. Sci. Transl. Med. 8 (353), 353ra111. doi: 10.1126/scitranslmed.aaf7483
Das K. C., White C. W. (2002). Redox systems of the cell: possible links and implications. Proc. Natl. Acad. Sci. U.S.A. 99 (15), 9617–9618. doi: 10.1073/pnas.162369199
DiGiulio R. T., Washburn P. C., Wenning R. J., Winston G. W., Jewell C. S. (1989). Biochemical responses in aquatic animals: a review of determinants of oxidative stress. Environ. Toxicol. Chem. 8, 1103–1123. doi: 10.1002/etc.5620081203
Ebaid H., Bashandy S. A., Alhazza I. M., Rady A., El-Shehry S. (2013). Folic acid and melatonin ameliorate carbon tetrachloride-induced hepatic injury, oxidative stress and inflammation in rats. Nutr. Metab. (Lond) 10, 20. doi: 10.1186/1743-7075-10-20
Fan H. (2001). Sea Cucumber: ginseng in the sea - a review about the research and exploration of the medical care function of sea cucumber and its ingredient. Chin. J. Mar. Drugs 82, 37–44.
Fang Y. Z., Yang S., Wu G. (2002). Free radicals, antioxidants, and nutrition. Nutrition 18, 872–879. doi: 10.1016/S0899-9007(02)00916-4
Ferreira M. V. R., Alencastro A. C. R., Hermes-Lima M. (2003). Role of antioxidant defenses during estivation and anoxia exposure in the freshwater snail Biomphalaria tenagophila (Orbigny 1835). Can. J. Zool. 81, 1239–1248. doi: 10.1139/z03-104
Frick N. T., Bystriansky J. S., Ip Y. K., Chew S. F., Ballantyne J. S. (2008). Lipid, ketone body and oxidative metabolism in the African lungfish, Protopterus dolloi following 60 days of fasting and aestivation. Comp. Biochem. Physiol. A Mol. Integr. Physiol. 151, 93–101. doi: 10.1016/j.cbpa.2008.06.004
Gao F., Yang H. S., Xu Q., Wang F. Y., Liu G. B., German D. P. (2008). Phenotypic plasticity of gut structure and function during periods of inactivity in Apostichopus japonicus. Comp. Biochem. Physiol. B 150, 255–262. doi: 10.1016/j.cbpb.2008.03.011
Guo C., Wang Y., Wu Y., Han L., Gao C., Chang Y., et al. (2022). UPLC-Q-TOF/MS-based metabonomics study on Apostichopus japonicus in various aquaculture models. Aquac. Res. 53 (5), 2004–2014. doi: 10.1111/are.15729
Heldmaier G., Ortmann S., Elvert R. (2004). Natural hypometabolism during hibernation and daily torpor in mammals. Respir. Physiol. Neurobiol. 141, 317–329. doi: 10.1016/j.resp.2004.03.014
Hensley K., Robinson K. A., Gabbita S. P., Salsman S., Floyd R. A. (2000). Reactive oxygen species, cell signaling, and cell injury. Free. Radic. Biol. Med. 28 (10), 1456–1462. doi: 10.1016/S0891-5849(00)00252-5
Hossain M. S., Hashimoto M., Gamoh S., Masumura S. (1999). Antioxidative effects of docosahexaenoic acid in the cerebrum versus cerebellum and brainstem of aged hypercholesterolemic rats. J. Neurochem. 72, 1133–1138. doi: 10.1046/j.1471-4159.1999.0721133.x
Hu Z., Feng J., Song H., Zhou C., Yang M. J., Shi P., et al. (2022). Metabolic response of Mercenaria mercenaria under heat and hypoxia stress by widely targeted metabolomic approach. Sci. Total. Environ. 809, 151172. doi: 10.1016/j.scitotenv.2021.151172
Huo D., Sun L., Zhang L., Ru X., Liu S., Yang H. (2019). Metabolome responses of the sea cucumber Apostichopus japonicus to multiple environmental stresses: heat and hypoxia. Mar. pollut. Bull. 138, 407–420. doi: 10.1016/j.marpolbul.2018.11.063
Huskisson E., Maggini S., Ruf M. (2007). The role of vitamins and minerals in energy metabolism and well-being. J. Int. Med. Res. 35, 277–289. doi: 10.1177/147323000703500301
Johnson C., Ivanisevic J., Siuzdak G. (2016). Metabolomics: beyond biomarkers and towards mechanisms. Nat. Rev. Mol. Cell. Biol. 17, 451–459. doi: 10.1038/nrm.2016.25
Jones R. M. (1980). Metabolic consequences of accelerated urea synthesis during seasonal dormancy of spadefoot toads, Scaphiopus couchii and Scaphiopus multiplicatus. J. Exp. Zool. 212, 255–267. doi: 10.1002/jez.1402120212
Le Bel M., Brunet A., Gosselin J. (2014). Leukotriene B4, an endogenous stimulator of the innate immune response against pathogens. J. Innate. Immun. 6 (2), 159–168. doi: 10.1159/000353694
Levine R. L., Moskovitz J., Stadtman E. R. (2010). Oxidation of methionine in proteins: roles in antioxidant defense and cellular regulation. Iubmb. Life. 50, 301–307. doi: 10.1080/713803735
Li L., Chen M., Storey K. B. (2019). Metabolic response of longitudinal muscles to acute hypoxia in sea cucumber Apostichopus japonicus (Selenka): a metabolome integrated analysis. Comp. Biochem. Physiol. D. 29, 235–244. doi: 10.1016/j.cbd.2018.12.007
Li F., Liu Y., Song B., Sun H., Gu B., Zhang X. (1996). Study on aestivating habit of sea cucumber (Apostichopus japonicus selenka): 2. the factors relating to aestivation. J. Fish. China. 3, 49–57.
Liu S., Zhou Y., Ru X., Zhang M., Cao X., Yang H. (2016). Differences in immune function and metabolites between aestivating and non-aestivating Apostichopus japonicus. Aquaculture 459, 36–42. doi: 10.1016/j.aquaculture.2016.03.029
Li Y., Wang R., Xun X., Wang J., Bao L., Thimmappa R., et al. (2018). Sea Cucumber genome provides insights into saponin biosynthesis and aestivation regulation. Cell. Discovery 4, 1–17. doi: 10.1038/s41421-018-0030-5
Madeo F., Eisenberg T., Pietrocola F., Kroemer G. (2018). Spermidine in health and disease. Science 359 (6374), eaan2788. doi: 10.1126/science.aan2788
Miyazawa T., Burdeos G. C., Itaya M., Nakagawa K., Miyazawa T. (2019). Vitamin e: Regulatory redox interactions. IUBMB Life 71 (4), 430–441. doi: 10.1002/iub.2008
Moosmann B., Behl C. (2020). Cytoprotective antioxidant function of tyrosine and tryptophan residues in transmembrane proteins. Eur. J. Biochem. 267 (18), 5687–92. doi: 10.1046/j.1432-1327.2000.01658.x
Nowakowska A., Świderska-Kołacz G., Rogalska J., Caputa M. (2009). Antioxidants and oxidative stress in Helix pomatia snails during estivation. Comp. Biochem. Phys. C. 150 (4), 481–486. doi: 10.1016/j.cbpc.2009.07.005
Pedler S., Fuery C. J., Withers P. C., Flanigan J., Guppy M. (1996). Effectors of metabolic depression in an estivating pulmonate snail (Helix aspersa): whole animal and in vitro tissue studies. J. Comp. Physiol. B. 166, 375–381. doi: 10.1007/BF02336920
Quinville B. M., Deschenes N. M., Ryckman A. E., Walia J. S. (2021). A comprehensive review: sphingolipid metabolism and implications of disruption in sphingolipid homeostasis. Int. J. Mol. Sci. 22 (11), 5793. doi: 10.3390/ijms22115793
Ramnanan C. J., Storey K. B. (2006). Glucose-6-phosphate dehydrogenase regulation during hypometabolism. Biochem. Biophys. Res. Commun. 339, 7–16. doi: 10.1016/j.bbrc.2005.10.036
Rutherford C., Childs S., Ohotski J., McGlynn L., Riddick M., MacFarlane S., et al. (2013). Regulation of cell survival by sphingosine-1-phosphate receptor S1P1via reciprocal ERK-dependent suppression of bim and PI-3-kinase/protein kinase c-mediated upregulation of mcl-1. Cell. Death. Dis. 4, e927. doi: 10.1038/cddis.2013.455
Saccenti E., Hoefsloot H. C. J., Smilde A. K., Westerhuis J. A., Hendriks M. M. (2014). Reflections on univariate and multivariate analysis of metabolomics data. Metabolomics 10 (3), 361–374. doi: 10.1007/s11306-013-0598-6
Simioni C., Zauli G., Martelli A. M., Vitale M., Sacchetti G., Gonelli A., et al. (2018). Oxidative stress: role of physical exercise and antioxidant nutraceuticals in adulthood and aging. Oncotarget 9, 17181–17198. doi: 10.18632/oncotarget.24729
Storey K. B. (2002). Life in the slow lane: molecular mechanisms of estivation. Comp. Biochem. Physiol. A. 133, 733–754. doi: 10.1016/S1095-6433(02)00206-4
Storey K. B., Storey J. M. (1990). Facultative metabolic rate depression: molecular regulation and biochemical adaptation in anaerobiosis, hibernation, and estivation. Q. Rev. Biol. 65, 145–174. doi: 10.1086/416717
Storey K. B., Storey J. M. (2007). Putting life on ‘pause’ - molecular regulation of hypometabolism. J. Exp. Biol. 210, 1700–1714. doi: 10.1242/jeb.02716
Storey K. B., Storey J. M. (2010). Metabolic regulation and gene expression during aestivation. Prog. Mol. Subcell. Biol. 49, 25–45. doi: 10.1007/978-3-642-02421-4_2
Storey K. B., Storey J. M. (2012). Aestivation: Signaling and hypometabolism. J. Exp. Biol. 215, 1425–1433. doi: 10.1242/jeb.054403
Tanwar A. K., Kirti J. S., Kumar S., Dhillon M. K. (2021). The amino acid and lipophilic profiles of Chilo partellus (swinhoe) larvae fluctuate with diapause. J. Exp. Zool. A. Ecol. Genet. Physiol. 335 (7), 595–601. doi: 10.1002/jez.2502
Van Brocklyn J. R., Williams J. B. (2012). The control of the balance between ceramide and sphingosine-1-phosphate by sphingosine kinase: oxidative stress and the seesaw of cell survival and death. comp. biochem. Physiol. B. Biochem. Mol. Biol. 163 (1), 26–36. doi: 10.1016/j.cbpb.2012.05.006
Weckwerth W. (2003). Metaboomics in systems biology. Annu. Rev. Plant Biol. 54, 669–689. doi: 10.1146/annurev.arplant.54.031902.135014
Wei X., Yin Q., Zhao H., Cao Y., Cai C., Yao J. (2018). Metabolomics for the effect of biotin and nicotinamide on transition dairy cows. J. Agric. Food. Chem. 66 (22), 5723–5732. doi: 10.1021/acs.jafc.8b00421
Xiang X., Chen M., Wu C., Zhu A., Yang J., Lv Z., et al. (2016). Glycolytic regulation in aestivation of the sea cucumber Apostichopus japonicus: evidence from metabolite quantification and rate-limiting enzyme analyses. Mar. Biol. 163 (8), 167. doi: 10.1007/s00227-016-2936-5
Xing L., Sun L., Liu S., Zhang L., Yang H. (2021). Comparative metabolomic analysis of the body wall from four varieties of the sea cucumber Apostichopus japonicus. Food. Chem. 352, 129339. doi: 10.1016/j.foodchem.2021.129339
Xu D., Zhou S., Yang H. (2017). Carbohydrate and amino acids metabolic response to heat stress in the intestine of the sea cucumber Apostichopus japonicus. Aquac. Res., 00:1–9. doi: 10.1111/are.13411
Yang Q., Zhang X., Zhen L. U., Huang R., Tuan T. N., Wu J. S., et al. (2021). Transcriptome and metabolome analyses of sea cucumbers Apostichopus japonicus in southern china during the summer aestivation period. J. Ocean Univ. China. 20 (1), 198–212. doi: 10.1007/s11802-021-4482-0
Yang Y., Zheng Y., Sun L., Chen M. (2020). Genome-wide DNA methylation signatures of sea cucumber Apostichopus japonicus during environmental induced aestivation. Genes 11 (9), 1020. doi: 10.3390/genes11091020
Yang H., Zhou Y., Zhang T., Yuan X., Li X., Liu Y., et al. (2006). Metabolic characteristics of sea cucumber Apostichopus japonicus (Selenka) during aestivation. J. Exp. Mar. Biol. Ecol. 330, 505–510. doi: 10.1016/j.jembe.2005.09.010
Yuan X., Yang H., Chen M., Gao F. (2007a). Research advances in aestivation of sea cucumber Apostichopus japonicus (Selenka): A review. Mar. Sci. 31, 88–90.
Yuan X., Yang H., Wang L., Zhou Y., Zhang T., Liu Y. (2007b). Effects of aestivation on the energy budget of sea cucumber Apostichopus japonicus (Selenka) (Echinodermata: Holothuroidea). Acta Ecol. Sin. 27, 3155–3161. doi: 10.1016/s1872-2032(07)60070-5
Zhao Y., Chen M., Storey K. B., Sun L., Yang H. (2015). DNA Methylation levels analysis in four tissues of sea cucumber Apostichopus japonicus based on fluorescence-labeled methylation-sensitive amplified polymorphism (F-MSAP) during aestivation. Comp. Biochem. Phys. B. 181, 26–32. doi: 10.1016/j.cbpb.2014.11.001
Zhao Y., Yang H. S., Storey K. B., Chen M. Y. (2014). RNA-Seq dependent transcriptional analysis unveils gene expression profile in the intestine of sea cucumber Apostichopus japonicus during aestivation. Comp. Biochem. Phys. D. 10, 30–43. doi: 10.1016/j.cbd.2014.02.002
Keywords: Apostichopus japonicus, estivation, metabolomics, differential metabolites, oxidative stress, echinoderm
Citation: Zhao Y, Wang H, Wang H, Pi Y and Chen M (2022) Metabolic response of the sea cucumber Apostichopus japonicus during the estivation-arousal cycles. Front. Mar. Sci. 9:980221. doi: 10.3389/fmars.2022.980221
Received: 28 June 2022; Accepted: 19 July 2022;
Published: 08 August 2022.
Edited by:
Jun Ding, Dalian Ocean University, ChinaCopyright © 2022 Zhao, Wang, Wang, Pi and Chen. This is an open-access article distributed under the terms of the Creative Commons Attribution License (CC BY). The use, distribution or reproduction in other forums is permitted, provided the original author(s) and the copyright owner(s) are credited and that the original publication in this journal is cited, in accordance with accepted academic practice. No use, distribution or reproduction is permitted which does not comply with these terms.
*Correspondence: Ye Zhao, enl6eTE5ODcyMDA2QDE2My5jb20=; Muyan Chen, Y2hlbm11eWFuQG91Yy5lZHUuY24=