- Marine Biology Department, Coral Ecophysiology team, Centre Scientifique de Monaco, Monaco, Principality of Monaco
Coral bleaching, the breakdown of the coral-Symbiodiniaceae association has been identified as a major cause of coral reef decline worldwide. When symbiont functions are compromised, corals receive fewer photosynthetic products from their symbionts and suffer significant starvation along with changes in nutrient cycling. Not all coral species are equally susceptible to bleaching, but despite intensive research, our understanding of the causes for coral bleaching remains incomplete. Here, we investigated nutrient exchange between host and symbionts of two coral- Symbiodiniaceae associations that are differentially susceptible to bleaching when maintained under heterotrophy in the dark. We followed the fate of heterotrophic nutrients using bulk isotope and compound-specific (amino acid) isotope analyses. We showed that symbiont starvation is a major cause of symbiotic breakdown in the dark. While Oculina patagonica transferred almost all heterotrophically-acquired amino acids within two weeks in the dark to its symbionts and did not bleach, Turbinaria reniformis, transferred only 2 amino acids to its symbionts after 4 weeks in the dark, and experienced significant bleaching. These results pave the way for future studies on the role of nutrition in coral stress response and the importance of maintaining a healthy symbiont population to avoid coral bleaching.
Introduction
Symbioses based on nutritional interactions between two or more partners are widespread in nature and range from mutualism to parasitism (Paracer and Ahmadjian, 2000). The symbiosis between corals and Symbiodiniaceae, which is the basis for coral reef growth and formation, is considered mutualistic because both partners derive nutritional benefits from the association (Muscatine, 1980). While the symbionts fix dissolved inorganic compounds through photosynthesis and transfer photosynthates to the host (autotrophy), the coral host takes up dissolved and particulate organic matter from the environment (heterotrophy) and shares it with the symbionts, along with its metabolic waste products (Tremblay et al., 2016). Except for a few exceptions [i.e. Oulastrea crispata (Denis et al., 2012)], most scleractinian corals are highly dependent on Symbiodiniaceae to meet their nutritional requirements. In well-lit shallow waters, algal symbionts can translocate up to 90% of their photosynthates to the coral host, providing most of the daily needs of the association (Muscatine et al., 1984; Davies, 1991).
The coral-Symbiodiniaceae association is fragile, and numerous stressors such as high seawater temperatures, seawater pollution, or excessive sunlight can cause coral bleaching, the loss of symbionts, and/or photosynthetic pigments (van Oppen and Lough, 2018; Suggett and Smith, 2020). Many bleached coral species will starve and die if they do not quickly reestablish a functioning symbiont population. As the frequency of bleaching events has increased over the past century (Hughes et al., 2018), it is crucial to understand why and how corals bleach and to unlock the biological mechanisms at play. On the one hand, corals are associated with various Symbiodiniaceae genera with different heat resistance and abilities to support coral host nutrition (Stat et al., 2008; Baker et al., 2013; Pernice et al., 2015; Ezzat et al., 2017). On the other hand, several core processes, independent of symbiont genotype, have been identified during heat or light stress-induced bleaching, such as the accumulation of reactive oxygen species (ROS) in coral tissue (Krueger et al., 2015). Nevertheless, the trigger of coral bleaching is still unknown (Suggett and Smith, 2020). Another approach to identifying key bleaching mechanisms is to perform bleaching experiments using stressors other than high irradiance and temperature, and which do not trigger ROS increases (Tolleter et al., 2013). For example, darkness is a stressor that disrupts the fine-tuned nutritional and metabolic interactions between the coral partners (Matthews et al., 2017; Cui et al., 2019), which are both extremely important to consider for coral bleaching (Cziesielski et al., 2019). In the dark, but also under other types of stress, symbionts lose their photosynthetic competence. As a result, they tend to become more self-sustaining by reducing the transfer of photosynthates to their host and by retaining more nutrients for their own use (Tremblay et al., 2012; Baker et al., 2018). Therefore, the coral-Symbiodiniaceae association may transition from mutualism to commensalism or even parasitism (Baker et al., 2018). This transition corresponds to a loss of benefit parity between host and symbionts and increased costs that exceed benefits to the host (Douglas, 2008), a situation that promotes coral bleaching (Tolleter et al., 2013; Tremblay et al., 2016; Baker et al., 2018; Morris et al., 2019).
Although many coral-Symbiodiniaceae associations are disrupted when symbionts are maintained in dark or dim light conditions and are photosynthetically inefficient (Tolleter et al., 2013; Baker et al., 2018; Morris et al., 2019; Rädecker et al., 2021), some corals can maintain a large population of symbionts within their tissues (Wagner et al., 2011; Polinski and Voss, 2018; Padilla-Gamiño et al., 2019). This is true for mesophotic tropical corals but also for many temperate coral species. For example, the temperate symbiotic coral Oculina patagonica can maintain symbionts from bright shallow reefs to completely dark caves (Fine et al., 2001; Zaquin et al., 2019; Martinez et al., 2021). Similarly, when maintained for several weeks in the dark, the Mediterranean scleractinian coral Cladocora caespitosa exhibit much higher symbiont densities than tropical corals (Hoogenboom et al., 2010). Therefore, the process by which the above coral species avoid bleaching deserves to be considered, especially when coral bleaching becomes more frequent worldwide due to environmental stress.
To this end, we worked with a tropical symbiotic association (Turbinaria reniformis- Cladocopium sp.) that bleaches in dim light or dark and a Mediterranean association (Oculina patagonica- Brevolium psygmophilum) that can live in an entirely symbiotic state in the dark. We maintained these corals for eight weeks in the dark with zooplankton supply. Both coral species are known to have high grazing rates on plankton (Tremblay et al., 2011) and to often thrive in turbid and particle-rich environments (Anthony, 2006; Rubio-Portillo et al., 2014), suggesting that they can both rely on heterotrophic feeding in the dark. To follow the exchange of nutrients between the symbiotic partners, we used the technique of compound-specific isotope analysis (CSIA) to measure the δ15N signal of individual amino acid (AA). Amino acids have two different δ15N isotopic enrichment behaviors during trophic transfer. While “trophic” amino acids are enriched in 15N with each trophic transfer, the “source” amino acids undergo little change (McClelland and Montoya, 2002). These measurements provide an elegant way to assess the fate of external food within the symbiotic association (Fujii et al., 2020; Wall et al., 2020; Martinez et al., 2020a; Ferrier-Pagès et al., 2021). Our study addresses two fundamental questions. First, what are the changes in physiology and symbiotic state of the temperate and tropical symbiotic associations when maintained on a heterotrophic diet and in the dark for eight weeks? Second, how do the fluxes of heterotrophically-acquired amino acids change in these two coral-Symbiodiniaceae associations during light to dark transition? We hypothesize that the bleaching intensity and nutrient exchange between host and symbionts will be different between the tropical and temperate coral-Symbiodiniaceae associations. As a result, we predict that the temperate association will maintain its symbiont population by transferring large quantities of heterotrophic nutrients to the symbionts, while the tropical association will not.
Material and methods
Experimental setup
The scleractinian tropical species Turbinaria reniformis [associated with Cladocopium sp. (Tremblay et al., 2015)] and the Mediterranean species Oculina patagonica [associated with Brevolium psygmophilum (Martinez et al., 2021)], were used in this experiment. Colonies of O. patagonica were sampled at a depth of 5 meters in Albissola (Gulf of Genoa, Italy, western Mediterranean Sea), while colonies of T. reniformis were collected at 5 meters depth in the Gulf of Aqaba (Jordan, Red Sea). Both species were grown under controlled conditions in 6 open-water flow-through aquaria (3 per species) at the Centre Scientifique de Monaco. Aquaria were supplied with oligotrophic seawater pumped from 40 meters depth in front of the laboratory, filtered through sand filters, and renewed at a rate of 12 L h-1. Temperature was kept constant at 25°C for T. reniformis and 16°C for O. patagonica using heaters connected to Elli-Well PC 902/T controllers. Light (200 µmoles photons m-2 s-1) was provided by several HQI lamps, in a 12:12 light:dark cycle. Light intensity was controlled by a LI-COR data logger (LI- 1000) connected to a spherical quantum sensor (LI-193).
Five mother colonies of each species were used. Each colony was divided into three large nubbins (n=15, one nubbin per colony and aquaria). Nubbins were allowed to heal for 4 weeks on nylon mesh at the bottom of the aquaria under the above light, temperature, and seawater conditions. During this healing period, they were fed Artemia salina nauplii at repletion twice a week. After the 4 weeks, three nubbins per species (one per colony and aquaria) were sampled, designated as time 0 (T0), while the remaining nubbins were kept in the dark at 25°C or 16°C, in 3 aquaria per species. Under these conditions, corals received no light but were fed Artemia salina nauplii at repletion 3 times a week (every second day). Three nubbins of each species and aquarium were sampled after 2, 4, 6, and 8 weeks (T2, T4, T6, and T8, respectively) and immediately frozen before further analyses were carried out.
Sample preparation
For all nubbins, the tissue was removed from the skeleton using an air pick with ultra-pure water, and the slurry was homogenized with a potter tissue grinder. Subsequently, 2 ml of the slurry was removed to determine the symbiont density and total protein content. The remainder was centrifuged three times at 500 g for 10 min at 4°C to separate host tissue from symbionts. Finally, the symbionts were washed twice with ultra-pure water. The two fractions were freeze-dried before subsequent analysis of their CSIA-AAs and bulk stable isotope. Symbiont density was quantified using three 100 µl sub-samples and a Z1 Coulter Particle Counter (Beckman Coulter), and the Bradford assay was used for the total protein concentration. Sample absorbance was read using triplicate technical replicates, and protein concentration was determined using BSA standards. Data were normalized to the surface area (cm2) using the wax-dipping technique (Veal et al., 2010). Due to technical issues, the week 6 physiological samples could not be processed.
Amino acid stable isotope analysis
The nitrogen and carbon isotopic compositions of amino acids were determined by gas chromatography/combustion/isotope ratio mass spectrometry (GC/C/IRMS). The acid-hydrolyzed host and symbiont samples were first derivatized using the Ezfaast kit before isotopic analysis was performed, according to Martinez et al. (2020b). Briefly, 3.5 mg of the hydrolyzed samples were derivatized with the Ezfaast kit with a slight modification of replacing reagent 6 with dichloromethane as solvent. Amino acids were separated on a Zebron ZB-50 column (30 m, 0.25 mm, and 0.25 µm) on a Thermo Scientific Trace 1300 Gas Chromatograph using helium as the carrier gas at a constant flow of 1.5 ml/min. For carbon analysis, 1.5 µl was injected in split mode (1:15) at 250°C, while 2 µl was injected in split mode (1:5) at 250°C for nitrogen analysis. The separated amino acids were split on the MicroChannel device into two direction flows: Thermo Scientific ISQ quadrupole for amino acid identification and Thermo Scientific Delta-V advantage for N isotope analysis. In order to determine the isotopic ratio of nitrogen, the separated amino acids were combusted in a Thermo scientific GC isolink II at 1000°C for nitrogen analysis. Before the sample was run into the Delta-V for N2 analysis, it passed through a cold trap with liquid nitrogen to freeze all other gases. Triplicates were injected from each sample. Stable isotope ratios were expressed in standard δ notation, being atmospheric N2 (air). The trophic position (TPglu-phe) was calculated using glutamic acid and phenylalanine with the predefined equation of Chikaraishi et al. (2009) with the constants from Martinez et al. (2020b).
Bulk isotope analysis
Approximately 600 µg of lyophilized host and symbiont material were transferred to tin caps to analyze the nitrogen bulk isotopic content using an Integra II isotope ratio mass spectrometer (Sercon, United Kingdom).
Statistics
Statistical analysis of CSIA-AA was performed using PRIMER-e 7 with PERMANOVA+ add-on. Similarity matrices were created for the data using Euclidian distance followed by permutational multivariate analysis of variance (PERMANOVA) to test for statistical significance. Because there were fewer than 5 replicates, we used PERMANOVA with Monte-Carlo. We also used the pair-wise PERMANOVA test to analyze the significant differences between groups. Only values with p < 0.05 were considered significant. For all the rest, Statistica 10 (Statsoft) was used to perform an analysis of variance (ANOVA). Data were checked using Levene’s test to ensure that they met the assumptions for parametric tests. Tukey HSD was used as a post hoc analysis for multiple comparisons between treatments with a significance level of p < 0.05.
Results
Symbiont and protein concentrations per skeletal surface area were significantly higher in O. patagonica than in T. reniformis (t-test p<0.001 and p<0.001, respectively). In O. patagonica (Figures 1A, C) there was no significant effect of the incubation time on symbiont density (Tukey HSD p>0.15) or protein concentration (Tukey HSD p>0.069), normalized to the skeletal surface area. However, symbiont density normalized to protein content (Figures 1E) decreased by 50% between T0 and T2 (Tukey HSD p=0.009) before steadily increasing again, reaching a significantly higher density at T8 than at other incubation times (Tukey HSD p<0.007). These results suggest that symbiont concentration decreased faster than protein concentration during the first two weeks, while the opposite was observed thereafter.
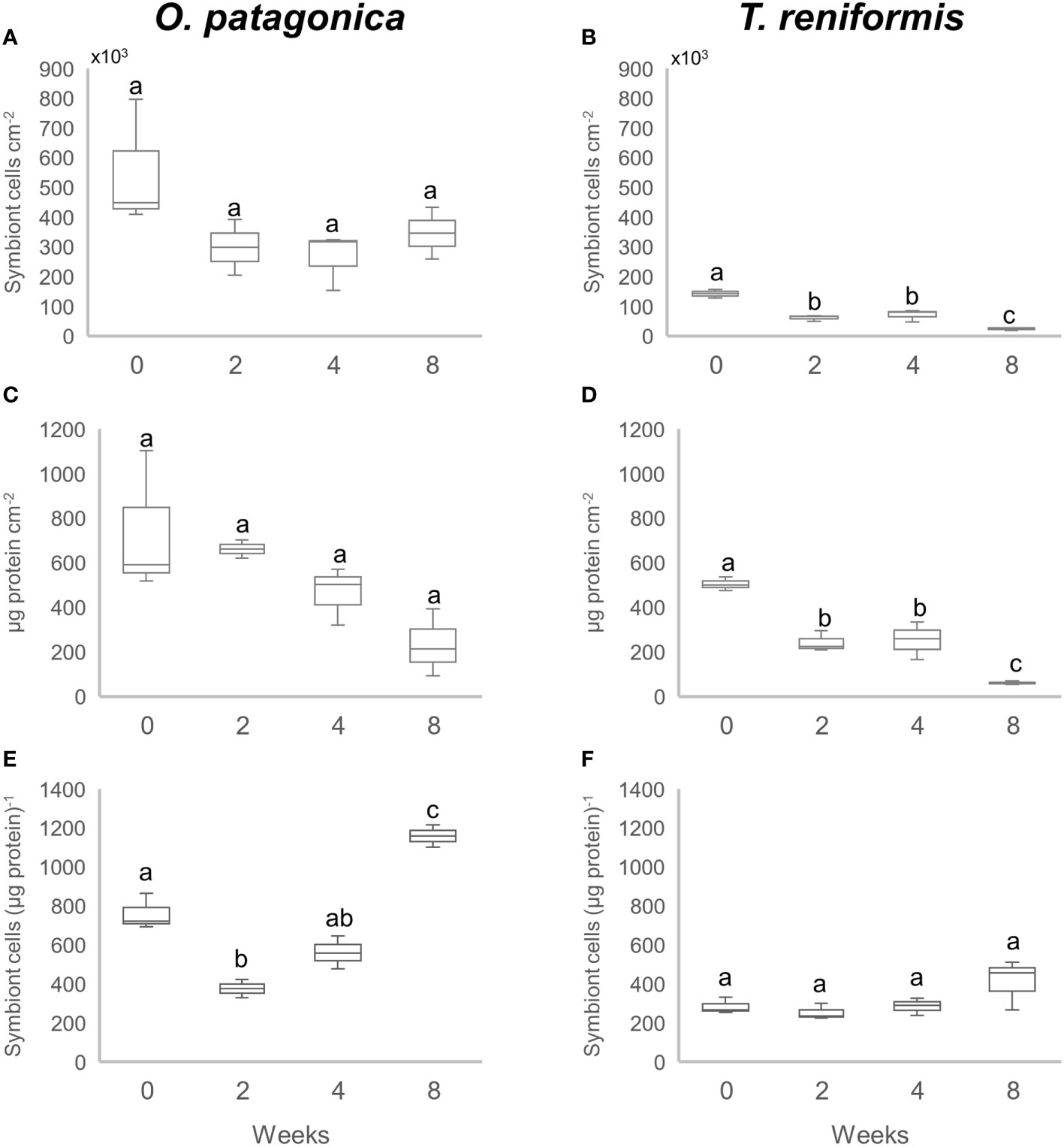
Figure 1 Symbiont density and protein concentration in the corals after a 0, 2, 4 and 8 week incubation in the dark. (A) and (B) Number of symbiont cells per skeletal surface area (cm2) in O. patagonica and T. reniformis, respectively. (C) and (D) Total protein content per skeletal surface area (cm2) in O. patagonica and T. reniformis, respectively. (E) and (F) Number of symbiont cells per protein in O. patagonica and T. reniformis, respectively. Significant differences between weeks are represented by different letters.
T. reniformis showed a completely different pattern in symbiont and protein changes compared to O. patagonica (Figures 1B, D). Symbiont density normalized to skeletal surface area decreased continuously and significantly during the dark incubation. Thus, the density was two to three times higher at T0 compared to all other time points (Tukey HSD p<0.002), and twice higher at T4 compared to T8 (Tukey HSD p=0.04). The protein concentration showed the same decrease pattern during the dark incubation. It decreased between T0 and T2 (Tukey HSD p=0.05) and again between T4 and T8 (Tukey HSD p=0.013). The symbiont density normalized to protein content (Figure 1F) did not change during the first 4 weeks of incubation (Tukey HSD p>0.45), but significantly increased between T4 and T8 (Tukey HSD p<0.005). The protein concentration thus showed a higher decrease than the symbiont concentration at T8.
There was a significant increase in the δ15N-AAs of the O. patagonica host over the incubation (Figure 2, PERMANOVA p=0.001), with a significant difference at T6 and T8 compared to the previous sampling times (Figure 3, PERMANOVA pair-wise test p<0.05). The δ15N-AAs of O. patagonica symbionts also increased already after 2 weeks (T2, Figure 2, PERMANOVA p=0.01), with higher values at T4 and T8 compared to T0 and T2 (Figure 3, PERMANOVA pair-wise test p<0.05). There was a significant increase in the δ15N-AAs of T. reniformis host over the incubation (Figure 2, PERMANOVA p=0.034), with a significant difference between T0 and T4–T6 (Figure 3, PERMANOVA pair-wise test p=0.044 and p=0.024, respectively). However, the overall δ15N signature of the AAs of T. reniformis symbionts did not change over time (Figure 3, PERMANOVA p=0.29). Only two AAs, namely alanine and leucine presented a 4‰ increase inδ15N after 8 weeks compared to T0.
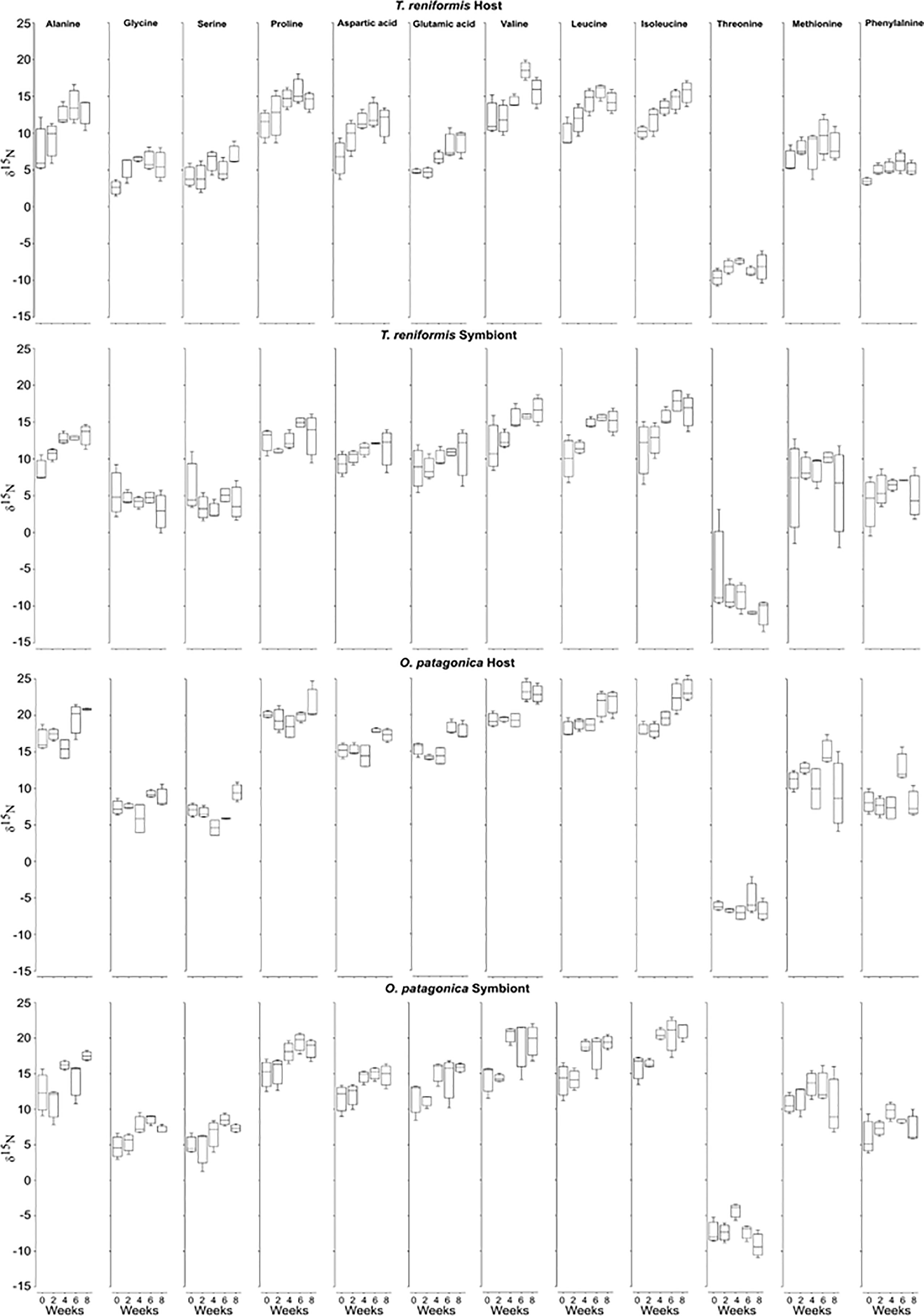
Figure 2 δ15N-AAs values of the host and symbionts of O. patagonica and T. reniformis over the 8 weeks of incubation in the dark.
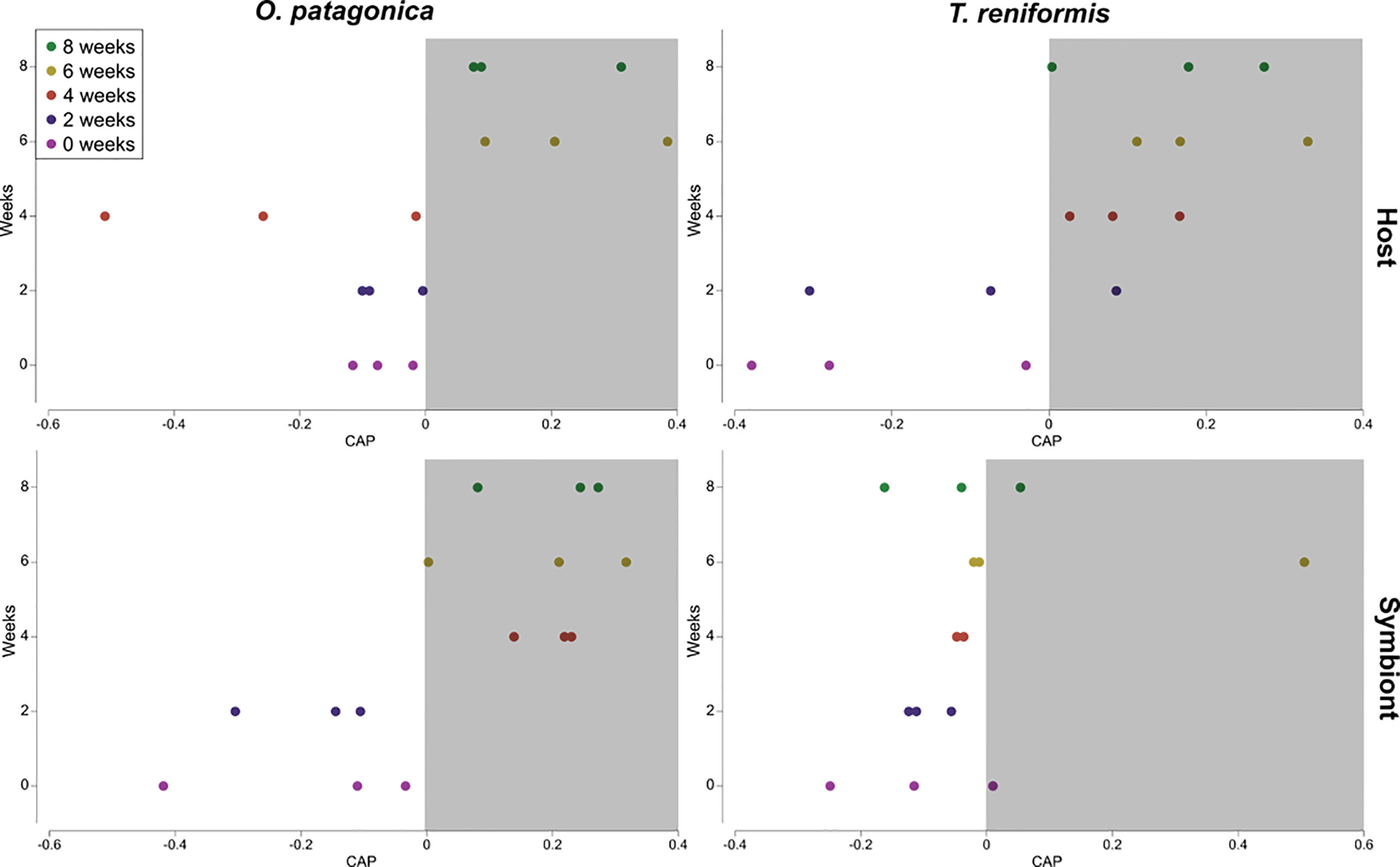
Figure 3 Canonical analysis of principal coordinates (CAP) plot performed using Euclidean distance matrix of the δ15N values of the amino acids (alanine, glycine, serine, proline, aspartic-acid, glutamic-acid, valine, leucine, isoleucine, threonine, methionine, and phenylalanine) of the host and symbiont compartments of the corals O. patagonica and T. reniformis over 8 weeks incubation in the dark. Weeks in the white area significantly differ from weeks in the grey area. However, if weeks are overlapping between white and grey areas, they are not different.
There was no significant change in the TP(glu-phe) of O. patagonica host and symbionts over the eight weeks of incubation in the dark (Figure 4, Tukey HSD p>0.24). There was also no significant change in the TP(glu-phe) of T. reniformis symbionts over the incubation (Figure 4, Tukey HSD p>0.13). In contrast, the TP(glu-phe) of T. reniformis host increased during the incubation, and a significant difference was observed between T2 and T6–T8 (Figure 4, Tukey HSD p<0.03).
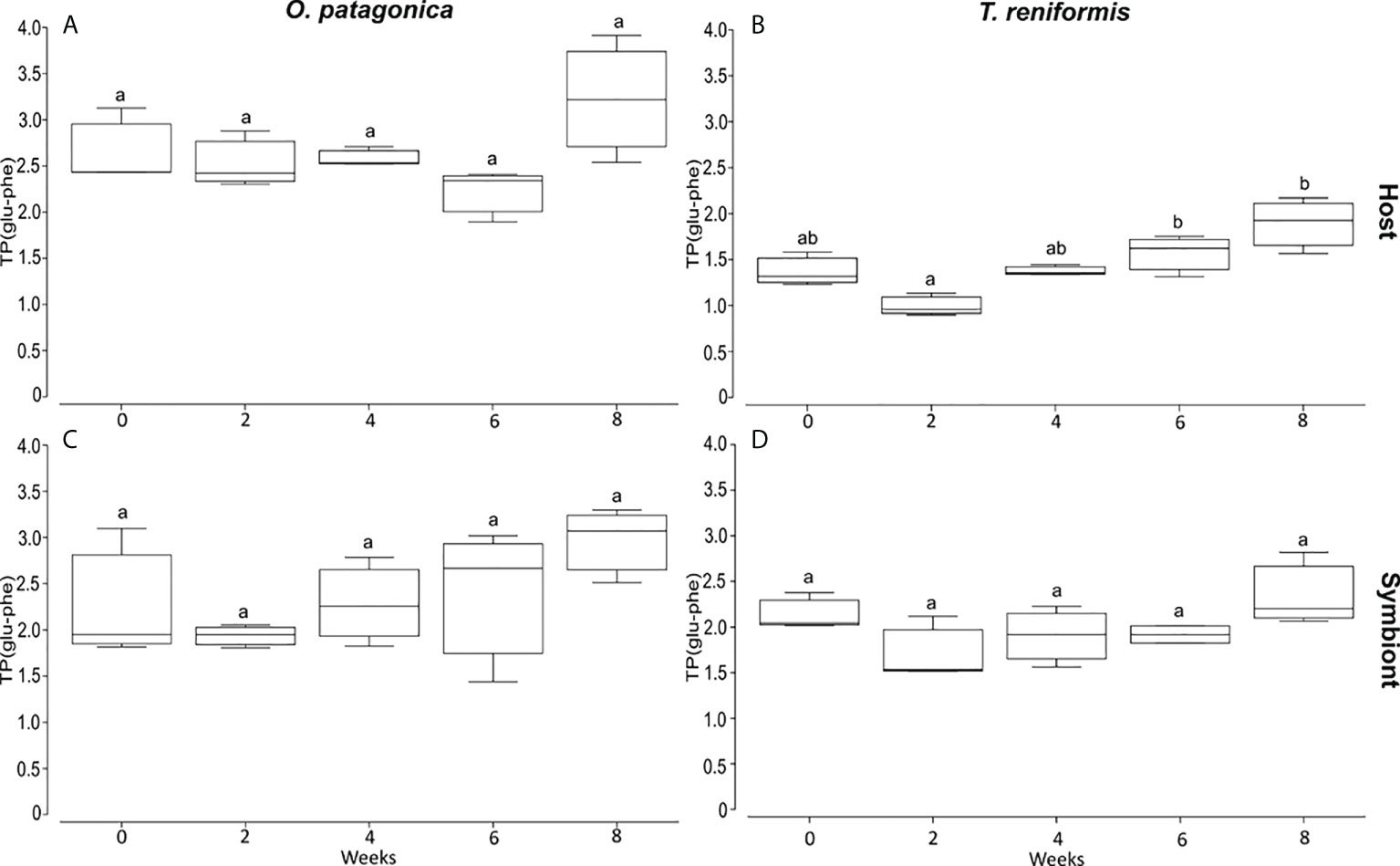
Figure 4 Trophic position based on the isotopic value of glutamic acid and phenylalanine (TPglu-phe) over 8 weeks of incubation in the dark. (A) and (B) are O. patagonica and T. reniformis host tissue. (C) and (D) are O. patagonica and T. reniformis symbionts. Significant differences between weeks are represented by different letters.
The bulk nitrogen values of the host tissue and symbionts of both coral species did not change over time, except for the host of O. patagonica, for which the δ15N values significantly decreased between T4 and T8 (Figure 5, Tukey HSD p=0.011).
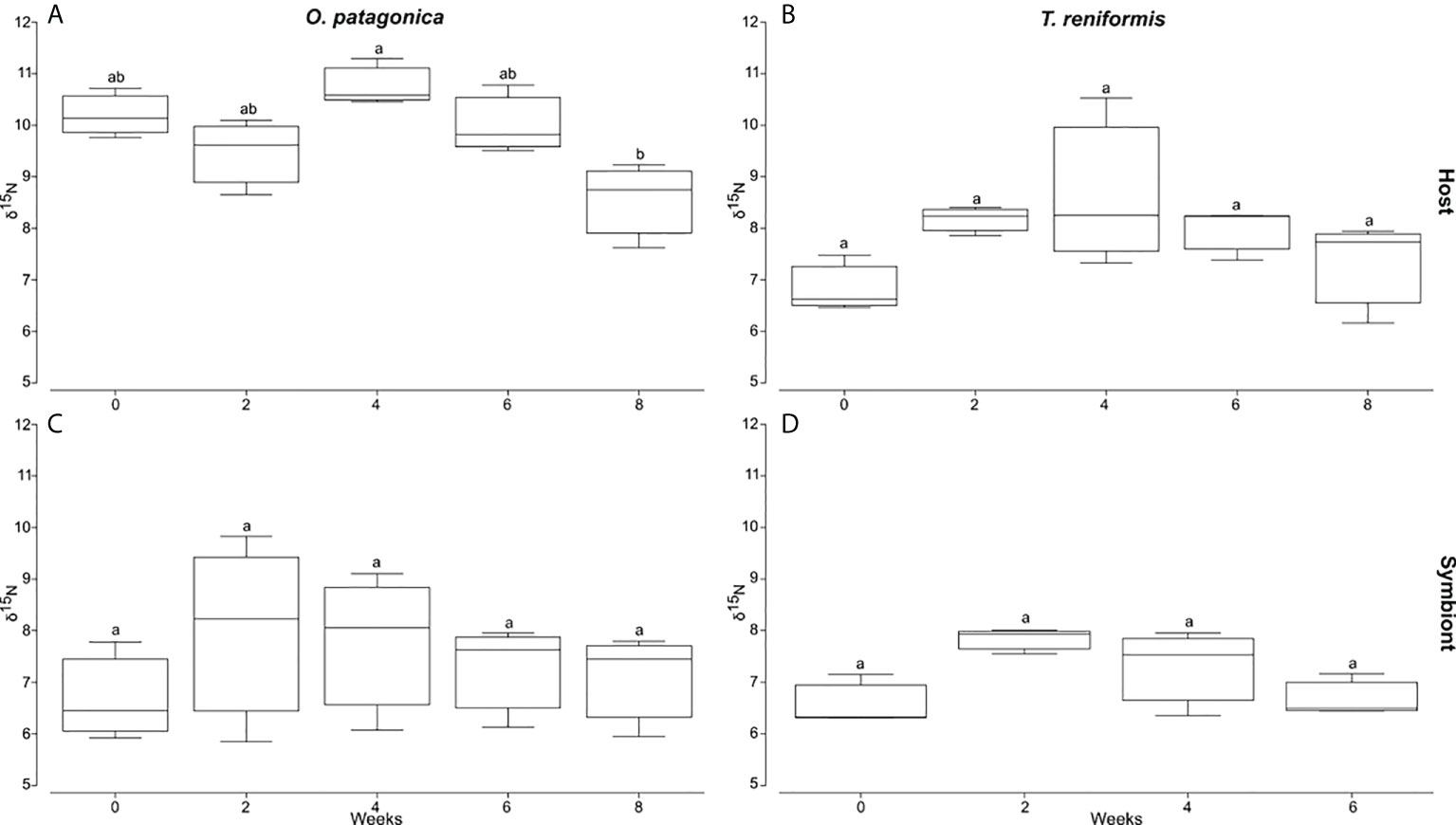
Figure 5 Bulk δ15N values of the host tissue and symbionts of O. patagonica and T. reniformis over 8 week incubation in the dark. (A) and (B) are O. patagonica and T. reniformis host tissue. (C) and (D) are O. patagonica and T. reniformis symbionts. Significant differences between weeks are represented by different let.
Discussion
This study reveals different symbiotic interactions in tropical and temperate Mediterranean corals both under light and dark conditions, which could explain differences in their response to changing environmental conditions. While O. patagonica continuously delivered in the dark a large panel of heterotrophic amino acids to its symbionts to maintain a large symbiont population, this was not the case in T. reniformis which underwent bleaching. These results highlight the role of the coral host and symbiont nutrition in the bleaching process.
The trophic position measures the position of a species in a food web, starting with primary producers (e.g., phytoplankton) with a TP(glu-phe) of 1 and moving through primary consumers (TP(glu-phe)= 2) to secondary consumers (TP(glu-phe) > 2). Mixotrophs such as corals can occupy a trophic position between one and two since they acquire autotrophic food from the symbionts and heterotrophic food from the host. At the start of the experiment under light conditions, symbionts of both T. reniformis and O. patagonica showed a high TP(glu-phe) index (>2), suggesting that they acquired a large fraction of heterotrophic nutrients delivered in the form of Artemia salina nauplii. This transfer of nutrients from the host to the symbionts under normal light conditions has already been observed in previous studies (Piniak et al., 2003; Tremblay et al., 2015; Ferrier-Pagès et al., 2021). The TP (glu-phe) index of the coral hosts however showed a greater dependence on heterotrophy for the temperate compared to the tropical host. Although the two coral species were grown under similar feeding and light conditions, and the symbiont density was significantly higher in O. patagonica, the initial TP(glu-phe) of O. patagonica host was twice as high (TP(glu-phe)=2.7 ± 0.4) as of T. reniformis (host TP(glu-phe)=1.4 ± 0.2). This result suggests that O. patagonica derives most of its energy from predation, whereas T. reniformis relies more on symbiont photosynthesis. This difference is due to higher capture rates of zooplankton (Tremblay et al., 2011) and better assimilation rates of nutrients by O. patagonica than T. reniformis (Tremblay et al., 2015). Therefore, according to the TP(glu-phe) index measured under light conditions, O. patagonica acquired enough food to share it between the coral host and symbionts, while heterotrophic nutrients were mostly used by symbionts of T. reniformis. It is generally believed that temperate corals meet a more significant proportion of their daily nutrient requirements through heterotrophy (Tremblay et al., 2015). Such dependence on heterotrophy is confirmed by a similarly high TP(glu-phe) (2.8) measured in situ for shallow and mesophotic O. patagonica (Martinez et al., 2021). In addition, O. patagonica exhibited much higher protein concentration and symbiont density than T. reniformis. Higher heterotrophic feeding in O. patagonica may have provided additional nutrients such as nitrogen and phosphorus, leading to increased growth of algal symbionts and a lack of symbiont regulation (Dean et al., 2016). In contrast, tropical corals are generally more limited by nutrient availability (Muscatine et al., 1989) or have lower heterotrophic capacities (Tremblay et al., 2015), which in turn reduces the maximum size of the symbiont population. In addition, models of nutrient trading between host and symbionts have suggested that coral holobionts adapted to high light conditions, such as the tropical coral T. reniformis, exhibit a higher degree of host control over the symbionts (Lowe et al., 2016). This control allows the coral host to prevent excessive symbiont growth and stops the ultimate exploitation of the host by the symbionts. Such control can be achieved by limiting inorganic nitrogen availability to the symbionts (Muscatine et al., 1989; Morris et al., 2019).
Incubation in the dark shows that both tropical and temperate coral hosts are capable of trophic plasticity, relying more heavily on heterotrophy when photosynthesis is impaired. This is evidenced in T. reniformis by the significant increase in host TP(glu-phe) and δ15N-AAs values toward zooplankton values. O. patagonica already exhibited very high host TP(glu-phe) in the light, suggesting that the coral continuously depends on heterotrophy. Nevertheless, δ15N-AAs values of O. patagonica increased after four weeks of incubation in the dark, indicating higher assimilation of heterotrophic food. It should be noted that the bulk δ15N values did not change in either species during incubation in the dark, despite changes in TP(glu-phe) and δ15N-AAs. These methodological differences suggest that bulk stable isotope analysis is less sensitive to nutritional changes than the other two proxies. Furthermore, the significant decrease in protein concentration at the end of the experiment in T. reniformis and O. patagonica indicates that heterotrophy alone is insufficient to maintain tissue biomass at the highest level and that symbiont photosynthesis is essential for host growth and well-being.
A minimum of four weeks of feeding in the dark was necessary to observe a significant increase in δ15N-AAs values in the host tissue of T. reniformis and O. patagonica. The rate of 15N incorporation into AAs can be influenced by several factors, such as biochemical reactions that control metabolic breakdown. The molecular weight and structure of AAs can also affect the kinetic isotope effects associated with AA transport (Bradley et al., 2014). In T. reniformis, glycine and phenylalanine were the first amino acids presenting an increase in δ15N signature with heterotrophy. This is expected for glycine as it is the smallest and structurally least complex amino acid and should have the fastest turnover rate. On the other hand, phenylalanine is an essential amino acid that is being transferred from primary producers to consumers without change. Therefore, the increased δ15N-phenylalanine value was likely due to the change in the nitrogen source of the coral when it switched from symbiont autotrophy to host heterotrophy, as previously observed (Ferrier-Pagès et al., 2021). At the end of the dark incubation, all amino acids in T. reniformis host tissue showed increased δ15N values, except threonine and methionine, probably due to different assimilation processes than other amino acids. For example, threonine is one of the three amino acids, along with lysine and proline, that is not always transaminated. When transaminated, its δ15N value decreases rather than increases with each trophic level (Fuller and Petzke, 2017). In animals, there is also a small trophic discrimination factor for methionine since it does not have the C–N bond cleaved in its first metabolic step (Ishikawa et al., 2018).
The dark incubation also revealed a different host-symbiont relationship and pattern of heterotrophic food allocation in the temperate and tropical species studied. Although symbiont density was very high in O. patagonica at the beginning of the experiment, which could be a stress in the dark, the loss of symbionts was low and insignificant. High symbiont density was previously observed in another Mediterranean scleractinian species, Cladocora caespitosa, growing under low winter light (Hoogenboom et al., 2010), in mesophotic colonies of O. patagonica (Martinez et al., 2021), as well as in O. patagonica grown in the dark for two years (Zaquin et al., 2019). Such maintenance of a stable symbiotic association is likely enabled by a strong reliance on heterotrophy and sharing of heterotrophic amino acids with symbionts. This is highlighted in this study by very high symbiont TP(glu-phe) (equal to 2.4 ± 0.6 throughout the experiment) and a rapid increase, after only 2 weeks, of the δ15N-AAs values towards zooplankton signature in the dark. Such an increase in symbiont δ15N-AAs values occurred faster (after 2 weeks) than in the host (after 4 weeks), suggesting that symbiont nutrition was favored compared to the host nutrition. Maintaining high symbiont density in O. patagonica tissues suggests either a poor host control over symbiont growth (Dean et al., 2016), or an essential role for symbionts other than photosynthesis. This could be similar to the black corals from Hawaii that grow below the photic zone and yet harbour symbiotic algae (Wagner et al., 2011). Black coral symbionts are thought to be critical to the holobiont’s nitrogen supply because they can take up and assimilate nitrate, which is present in high concentrations in deep waters. Because temperate corals generally have access to large amounts of particulate and dissolved organic matter, it is not very costly for the host to transfer amino acids from its heterotrophic diet to the symbionts. This transfer, in turn, allows the maintenance of a large density of symbionts that can become immediately active when the coral returns to normal light conditions. The availability of heterotrophic nutrients also prevents the host from starving and using amino acids as alternative energy substrates (Rädecker et al., 2021). In this case, the switch from amino acid anabolism to catabolism increases nitrogen (ammonium) availability to algal symbionts, which then may grow faster and may exacerbate host starvation by reducing carbon translocation.
On the contrary to O. patagonica, T. reniformis bleached significantly and continuously in the dark, reaching a very low symbiont concentration. Such dark-induced bleaching has been previously observed in other tropical coral species (DeSalvo et al., 2012; Tolleter et al., 2013; Rouan et al., 2021). It has been associated with various dysfunctions such as damage to the photosynthetic apparatus of the symbionts (Tolleter et al., 2013), shortening of telomere length (Rouan et al., 2021), growth arrest, and metabolic changes (DeSalvo et al., 2012). In this study, isotopic measurements of T. reniformis and the comparison with O. patagonica, demonstrate that bleaching in the dark occurred in parallel to a poor translocation of heterotrophic food from the coral host to its symbionts. Indeed, the δ15N of only 2 amino acids increased in the symbionts (against 9-10 in O. patagonica symbionts), and after 4 weeks of feeding only. The acquisition of heterotrophic nutrients was therefore delayed compared to O. patagonica symbionts, for which the δ15N-AAs increased after only 2 weeks of feeding. The highest loss of symbionts in T. reniformis occurred in the first two weeks when they did not receive enough heterotrophic nutrients. Such lack of nutrient provisioning to the symbionts is also suggested by the decrease in their TP glu-phe index, from 2 to 1.5 after two weeks in the dark. In a previous experiment in which T. reniformis was kept in the light and fed abundantly (5 times a week) 15N-labelled artemia (Tremblay et al., 2015), a significant 15N enrichment of the symbionts was observed, suggesting that symbionts can have access to heterotrophic nutrients. However, the feeding level was maybe not sufficient in our experiment (3 times a week) to observe a significant effect of heterotrophy on T. reniformis symbionts. Alternatively, incubation in the dark did not allow symbionts to take advantage of the heterotrophic nutrients acquired by the host. A similar link between bleaching and symbiont starvation in trace elements and other essential nutrients was highlighted in previous studies (Ferrier-Pagès et al., 2018; Blanckaert et al., 2022). In these studies, providing the symbionts with essential metals (e.g. iron, zinc, and manganese), either through heterotrophic feeding or dust leaching, mitigated the corals’ susceptibility to bleaching. This is consistent with previous studies showing that heterotrophy maintained symbiont density and photosynthesis during heat stress and promoted photosynthate translocation (Ferrier-Pagès et al., 2010; Tremblay et al., 2016). Overall, this study, along with the earlier study by Rädecker et al. (2021), clearly demonstrates that bleaching is partly due to the disruption of the nutrient trafficking between the coral host and symbionts. Any situation that leads to starvation of the animal or the symbionts will promote coral bleaching. The question remains as to why O. patagonica transferred heterotrophic food to the symbionts to maintain maximum symbiont density while T. reniformis didn’t do so, despite being fed a similar diet. The TP glu-phe indicates that for the same diet, O. patagonica is more heterotroph and likely assimilates more food than T. reniformis. It also hosts a different symbiont genotype, which might have a faster growth or is better adapted to high food levels. For example, different Symbiodiniaceae strains may translocate different types and quantities of photosynthate to the host (Davy et al., 2012), affecting the host fitness. Several studies have shown that corals dominated by Durusdinium grow more slowly (Little et al., 2004) than conspecifics dominated by Cladocopium, likely because Cladocopium translocates more carbon to its host compared to Durusdinium under ambient conditions (Cantin et al., 2009). Future studies should aim at investigating the metabolic differences in the two coral-dinoflagellates associations studied here to further understand which partner is driving the exchange of nutrients.
Conclusion
Efforts to prevent or reduce the occurrence of mass coral bleaching due to ocean warming, acidification, or pollution are hampered by an incomplete understanding of the processes underlying the breakdown of the coral-algae symbiosis. Our study shows that bleaching triggered by dark incubation is associated with symbiont starvation. While the Mediterranean coral O. patagonica was able to maintain a stable symbiont population in the dark by transferring heterotrophic amino acids from the host to the symbionts, this was not observed in the tropical coral T. reniformis which experienced significant bleaching. Together with previous observations, the results of this study suggest that the tolerance of symbiotic corals to environmental stress is related to the nutritional status of both the animal host and the symbionts. In the future, not only macronutrients but also micronutrients need to be considered to obtain a holistic view of the effects of nutrients on the stability of the symbiosis. In the nutrient-poor waters of coral reefs, heterotrophy appears to be the main source of macro and micronutrients for corals, and it has been shown to be an essential factor explaining coral resistance and resilience to environmental stress. Therefore, future experiments should investigate whether the intensity of bleaching in tropical coral Symbiodiniaceae symbioses is related to the intensity of starvation of the host and the symbiont.
Data availability statement
The original contributions presented in the study are included in the article/supplementary material. Further inquiries can be directed to the corresponding author.
Author contribution
SM, CF-P, and RG designed and ran the experiments. SM performed the CSIA-AA and the statistics while RG performed the bulk isotope analysis. all authors interpreted the data and wrote the manuscript. All authors contributed to the article and approved the submitted version.
Acknowledgments
We would like to thank Prof. Tali Mass and Prof. Dan Tchernov from the University of Haifa for allowing us to use their GC-IRMS to run our samples.
Conflict of interest
The authors declare that the research was conducted in the absence of any commercial or financial relationships that could be construed as a potential conflict of interest.
Publisher’s note
All claims expressed in this article are solely those of the authors and do not necessarily represent those of their affiliated organizations, or those of the publisher, the editors and the reviewers. Any product that may be evaluated in this article, or claim that may be made by its manufacturer, is not guaranteed or endorsed by the publisher.
References
Anthony K. R. N. (2006). Enhanced energy status of corals on coastal, high-turbidity reefs. Mar. Ecol. Prog. Ser. 319, 111–116. doi: 10.3354/meps319111
Baker D. M., Andras J. P., Jordán-Garza A. G., Fogel M. L. (2013). Nitrate competition in a coral symbiosis varies with temperature among symbiodinium clades. ISME J. 7, 1248–1251. doi: 10.1038/ismej.2013.12
Baker D. M., Freeman C. J., Wong J. C. Y., Fogel M. L., Knowlton N. (2018). Climate change promotes parasitism in a coral symbiosis. ISME J. 12, 921–930. doi: 10.1038/s41396-018-0046-8
Blanckaert A. C. A., Omanović D., Fine M., Grover R., Ferrier-Pagès C. (2022). Desert dust deposition supplies essential bioelements to red Sea corals. Glob. Change Biol. 28, 2341–2359. doi: 10.1111/gcb.16074
Bradley C. J., Madigan D. J., Block B. A., Popp B. N. (2014). Amino acid isotope incorporation and enrichment factors in pacific bluefin tuna, thunnus orientalis. PloS One 9, 1–15. doi: 10.1371/journal.pone.0085818
Cantin N. E., Van Oppen M. J. H., Willis B. L., Mieog J. C., Negri A. P. (2009). Juvenile corals can acquire more carbon from high-performance algal symbionts. Coral Reefs 28, 405–414. doi: 10.1007/s00338-009-0478-8
Chikaraishi Y., Ogawa N. O., Kashiyama Y., Takano Y., Suga H., Tomitani A., et al. (2009)Determination of aquatic food-web structure based on compound-specific nitrogen isotopic composition of amino acids (Accessed June 11, 2014).
Cui G., Liew Y. J., Li Y., Kharbatia N., Zahran N. I., Emwas A. H., et al. (2019). Host-dependent nitrogen recycling as a mechanism of symbiont control in aiptasia. PloS Genet. 15, 1–19. doi: 10.1371/journal.pgen.1008189
Cziesielski M. J., Schmidt-Roach S., Aranda M. (2019). The past, present, and future of coral heat stress studies. Ecol. Evol. 9, 10055–10066. doi: 10.1002/ece3.5576
Davies P. S. (1991). Effect of daylight variations on the energy budgets of shallow-water corals. Mar. Biol. 108, 137–144. doi: 10.1007/BF01313481
Davy S. K., Allemand D., Weis V. M. (2012). Cell biology of cnidarian-dinoflagellate symbiosis. Microbiol. Mol. Biol. Rev. 76, 229–261. doi: 10.1128/mmbr.05014-11
Dean A. D., Minter E. J. A., Sørensen M. E. S., Lowe C. D., Cameron D. D., Brockhurst M. A., et al. (2016). Host control and nutrient trading in a photosynthetic symbiosis. J. Theor. Biol. 405, 82–93. doi: 10.1016/j.jtbi.2016.02.021
Denis V., Leung J. K. L., Hsu C.-M., Hsieh H. J., Tsai W.-S., Chen C. A. (2012). Dark survival of oulastrea crispata. Galaxea J. Coral Reef Stud. 14, 117–118. doi: 10.3755/galaxea.14.117
DeSalvo M. K., Estrada A., Sunagawa S., Medina M. (2012). Transcriptomic responses to darkness stress point to common coral bleaching mechanisms. Coral Reefs 31, 215–228. doi: 10.1007/s00338-011-0833-4
Douglas A. E. (2008). Conflict, cheats and the persistence of symbioses. New Phytol. 177, 849–858. doi: 10.1111/j.1469-8137.2007.02326.x
Ezzat L., Fine M., Maguer J.-F., Grover R., Ferrier-Pagès C. (2017). Carbon and nitrogen acquisition in shallow and deep holobionts of the scleractinian coral s. pistillata. Front. Mar. Sci. 4. doi: 10.3389/fmars.2017.00102
Ferrier-Pagès C., Martinez S., Grover R., Cybulski J., Shemesh E., Tchernov D. (2021). Tracing the trophic plasticity of the coral–dinoflagellate symbiosis using amino acid compound-specific stable isotope analysis. Microorganisms 9, 182. doi: 10.3390/microorganisms9010182
Ferrier-Pagès C., Rottier C., Beraud E., Levy O. (2010). Experimental assessment of the feeding effort of three scleractinian coral species during a thermal stress: Effect on the rates of photosynthesis. J. Exp. Mar. Bio. Ecol. 390, 118–124. doi: 10.1016/j.jembe.2010.05.007
Ferrier-Pagès C., Sauzéat L., Balter V. (2018). Coral bleaching is linked to the capacity of the animal host to supply essential metals to the symbionts. Glob. Change Biol. 24, 3145–3157. doi: 10.1111/gcb.14141
Fine M., Zibrowius H., Loya Y. (2001). Oculina patagonica: A non-lessepsian scleractinian coral invading the Mediterranean sea. Mar. Biol. 138, 1195–1203. doi: 10.1007/s002270100539
Fujii T., Tanaka Y., Maki K., Saotome N., Morimoto N., Watanabe A., et al. (2020). Organic carbon and nitrogen isoscapes of reef corals and algal symbionts: Relative influences of environmental gradients and heterotrophy. Microorganisms 8, 1–26. doi: 10.3390/microorganisms8081221
Fuller B. T., Petzke K. J. (2017). The dietary protein paradox and threonine 15 n-depletion: Pyridoxal-5’-phosphate enzyme activity as a mechanism for the δ 15 n trophic level effect. Rapid Commun. Mass Spectrom. 31, 705–718. doi: 10.1002/rcm.7835
Hoogenboom M., Beraud E., Ferrier-Pagès C. (2010). Relationship between symbiont density and photosynthetic carbon acquisition in the temperate coral cladocora caespitosa. Coral Reefs 29, 21–29. doi: 10.1007/s00338-009-0558-9
Hughes T. P., Anderson K. D., Connolly S. R., Heron S. F., Kerry J. T., Lough J. M., et al. (2018). Spatial and temporal patterns of mass bleaching of corals in the anthropocene. Sci. (80-.) 359, 80–83. doi: 10.1126/science.aan8048
Ishikawa N. F., Chikaraishi Y., Takano Y., Sasaki Y., Takizawa Y., Tsuchiya M., et al. (2018). A new analytical method for determination of the nitrogen isotopic composition of methionine: Its application to aquatic ecosystems with mixed resources. Limnol. Oceanogr. Methods 16, 607–620. doi: 10.1002/lom3.10272
Krueger T., Hawkins T. D., Becker S., Pontasch S., Dove S., Hoegh-Guldberg O., et al. (2015). Differential coral bleaching-contrasting the activity and response of enzymatic antioxidants in symbiotic partners under thermal stress. Comp. Biochem. Physiol. -Part A Mol. Integr. Physiol. 190, 15–25. doi: 10.1016/j.cbpa.2015.08.012
Little A. F., van Oppen M. J. H., Willis B. L., Angela F., Madeleine J. H., van Oppen B. L. W. (2004). Flexibility in algal endosymbioses. Science 304, 1492–1495. doi: 10.1126/science.1095733
Lowe C. D., Minter E. J., Cameron D. D., Brockhurst M. A. (2016). Shining a light on exploitative host control in a photosynthetic endosymbiosis. Curr. Biol. 26, 207–211. doi: 10.1016/j.cub.2015.11.052
Martinez S., Bellworthy J., Ferrier-Pagès C., Mass T. (2021). Selection of mesophotic habitats by oculina patagonica in the Eastern Mediterranean Sea following global warming. Sci. Rep. 11, 1–15. doi: 10.1038/s41598-021-97447-5
Martinez S., Kolodny Y., Shemesh E., Scucchia F., Nevo R., Levin-Zaidman S., et al. (2020a). Energy sources of the depth-generalist mixotrophic coral stylophora pistillata. Front. Mar. Sci. 7. doi: 10.3389/fmars.2020.566663
Martinez S., Lalzar M., Shemesh E., Einbinder S., Goodman B., Tchernov D. (2020b). Effect of different derivatization protocols on the calculation of trophic position using amino acids compound-specific stable isotopes. Front. Mar. Sci. 7. doi: 10.3389/fmars.2020.561568
Matthews J. L., Crowder C. M., Oakley C. A., Lutz A., Roessner U., Meyer E., et al. (2017). Optimal nutrient exchange and immune responses operate in partner specificity in the cnidarian-dinoflagellate symbiosis. Proc. Natl. Acad. Sci. U. S. A. 114, 13194–13199. doi: 10.1073/pnas.1710733114
McClelland J., Montoya J. (2002). Trophic relationships and the nitrogen isotopic composition of amino acids in plankton. Ecology 83, 2173–2180. doi: 10.1890/0012-9658(2002)083%5B2173:TRATNI%5D2.0.CO%3B2[AccessedJuly17
Morris L. A., Voolstra C. R., Quigley K. M., Bourne D. G., Bay L. K. (2019). Nutrient availability and metabolism affect the stability of coral–symbiodiniaceae symbioses.Trends Microbiol. 27, 678–689. doi: 10.1016/j.tim.2019.03.004
Muscatine L. (1980). Productivity of zooxanthellae. Primary Productivity Sea (Boston MA: Springer US), 19, 381–402. doi: 10.1007/978-1-4684-3890-1_21
Muscatine L., Falkowski P. G., Dubinsky Z., Cook P. A., McCloskey L. R. (1989). The effect of external nutrient resources on the population dynamics of zooxanthellae in a reef coral. Proc. - R. Soc London B 236, 311–324. doi: 10.1098/rspb.1989.0025
Muscatine L., Falkowski P. G., Porter J. W., Dubinsky Z. (1984). Fate of photosynthetic fixed carbon in light- and shade-adapted colonies of the symbiotic coral stylophora pistillata. Proc. R. Soc London Ser. B. Biol. Sci. 222, 181–202. doi: 10.1098/rspb.1984.0058
Padilla-Gamiño J. L., Roth M. S., Rodrigues L. J., Bradley C. J., Bidigare R. R., Gates R. D., et al. (2019). Ecophysiology of mesophotic reef-building corals in hawai’i is influenced by symbiont–host associations, photoacclimatization, trophic plasticity, and adaptation. Limnol. Oceanogr. 64, 1980–1995. doi: 10.1002/lno.11164
Paracer S., Ahmadjian V. (2000). Symbiosis: an introduction to biological associations. 2nd ed (198 Madison avenue, New-York, New-York 10016: New: Oxford University Press (OUP).
Pernice M., Dunn S. R., Tonk L., Dove S., Domart-Coulon I., Hoppe P., et al. (2015). A nanoscale secondary ion mass spectrometry study of dinoflagellate functional diversity in reef-building corals. Environ. Microbiol. 17, 3570–3580. doi: 10.1111/1462-2920.12518
Piniak G., Lipschultz F., McClelland J. (2003). Assimilation and partitioning of prey nitrogen within two anthozoans and their endosymbiotic zooxanthellae. Mar. Ecol. Prog. Ser. 262, 125–136. doi: 10.3354/meps262125
Polinski J. M., Voss J. D. (2018). Evidence of photoacclimatization at mesophotic depths in the coral-symbiodinium symbiosis at flower garden banks national marine sanctuary and McGrail bank. Coral Reefs 37, 779–789. doi: 10.1007/s00338-018-1701-2
Rädecker N., Pogoreutz C., Gegner H. M., Cárdenas A., Roth F., Bougoure J., et al. (2021). Heat stress destabilizes symbiotic nutrient cycling in corals. Proc. Natl. Acad. Sci. U. S. A. 118, 1–11. doi: 10.1073/pnas.2022653118
Rouan A., Pousse M., Tambutté E., Djerbi N., Zozaya W., Capasso L., et al. (2021). Telomere dysfunction is associated with dark-induced bleaching in the reef coral stylophora pistillata. Mol. Ecol. 00, 1–13 doi: 10.1111/mec.16199
Rubio-Portillo E., Vázquez-Luis M., Valle C., Izquierdo-Muñoz A., Ramos-Esplá A. A. (2014). Growth and bleaching of the coral oculina patagonica under different environmental conditions in the western Mediterranean Sea. Mar. Biol. 161, 2333–2343. doi: 10.1007/s00227-014-2509-4
Stat M., Morris E., Gates R. D. (2008). Functional diversity in coral-dinoflagellate symbiosis. Proc. Natl. Acad. Sci. U. S. A. 105, 9256–9261. doi: 10.1073/pnas.0801328105
Suggett D. J., Smith D. J. (2020). Coral bleaching patterns are the outcome of complex biological and environmental networking. Glob. Change Biol. 26, 68–79. doi: 10.1111/gcb.14871
Tolleter D., Seneca F. O., Denofrio J. C., Krediet C. J., Palumbi S. R., Pringle J. R., et al. (2013). Coral bleaching independent of photosynthetic activity. Curr. Biol. 23, 1782–1786. doi: 10.1016/j.cub.2013.07.041
Tremblay P., Ferrier-Pagès C., Maguer J. F., Rottier C., Legendre L., Grover R. (2012). Controlling effects of irradiance and heterotrophy on carbon translocation in the temperate coral cladocora caespitosa. PloS One 7, 1–13. doi: 10.1371/journal.pone.0044672
Tremblay P., Gori A., Maguer J. F., Hoogenboom M., Ferrier-Pagès C. (2016). Heterotrophy promotes the re-establishment of photosynthate translocation in a symbiotic coral after heat stress. Sci. Rep. 6, 1–14. doi: 10.1038/srep38112
Tremblay P., Maguer J. F., Grover R., Ferrier-Pagès C. (2015). Trophic dynamics of scleractinian corals: A stable isotope evidence. J. Exp. Biol. 218, 1223–1234. doi: 10.1242/jeb.115303
Tremblay P., Peirano A., Ferrier-Pagés C. (2011). Heterotrophy in the Mediterranean symbiotic coral cladocora caespitosa: Comparison with two other scleractinian species. Mar. Ecol. Prog. Ser. 422, 165–177. doi: 10.3354/meps08902
van Oppen M. J. H., Lough J. M. (2018). Synthesis: Coral bleaching: Patterns, processes, causes and consequences Coral Bleaching 233, 343–348. doi: 10.1007/978-3-319-75393-5_14
Veal C. J., Carmi M., Fine M., Hoegh-Guldberg O. (2010). Increasing the accuracy of surface area estimation using single wax dipping of coral fragments. Coral Reefs 29, 893–897. doi: 10.1007/s00338-010-0647-9
Wagner D., Pochon X., Irwin L., Toonen R. J., Gates R. D. (2011). Azooxanthellate? most Hawaiian black corals contain symbiodinium. Proc. R. Soc B Biol. Sci. 278, 1323–1328. doi: 10.1098/rspb.2010.1681
Wall C. B., Kaluhiokalani M., Popp B. N., Donahue M. J., Gates R. D. (2020). Divergent symbiont communities determine the physiology and nutrition of a reef coral across a light-availability gradient. ISME J. 2, 1–14. doi: 10.1038/s41396-019-0570-1
Keywords: CSIA-AA, isotope, bleaching, dinoflagellate, compound-specific isotope analysis of amino acids, heterotrophy, coral reefs, holobiont
Citation: Martinez S, Grover R and Ferrier-Pagès C (2022) Symbiont starvation affects the stability of the coral–Symbiodiniaceae symbiosis. Front. Mar. Sci. 9:979563. doi: 10.3389/fmars.2022.979563
Received: 27 June 2022; Accepted: 24 August 2022;
Published: 13 September 2022.
Edited by:
Zhiyong Li, Shanghai Jiao Tong University, ChinaReviewed by:
Shashank Keshavmurthy, Academia Sinica, TaiwanJean-Baptiste Raina, University of Technology Sydney, Australia
Copyright © 2022 Martinez, Grover and Ferrier-Pagès. This is an open-access article distributed under the terms of the Creative Commons Attribution License (CC BY). The use, distribution or reproduction in other forums is permitted, provided the original author(s) and the copyright owner(s) are credited and that the original publication in this journal is cited, in accordance with accepted academic practice. No use, distribution or reproduction is permitted which does not comply with these terms.
*Correspondence: Stephane Martinez, U3RlcGhhbmUubWFydGluZXpAZ21haWwuY29t