- 1School of Life Sciences, Ludong University, Yantai, China
- 2Shandong Marine Resource and Environment Research Institute, Yantai, China
- 3Key Laboratory of Marine Biotechnology in Universities of Shandong (Ludong University), Yantai, China
In recent years, massive Sargassum drifting on the sea surface, known as “golden tides,” negatively impacted on the local marine ecology. However, the physiological mechanisms of its formation remain unclear. To investigate the photosynthetic responses of golden tide algae to UVR, one key factor for drifting Sargassum population, we cultivated thalli of S. horneri, a golden tide alga, under three light treatments: P (photosynthetically active radiation, PAR), PA (PAR+UVA) and PAB (PAR+UVA+UVB) for 120 mins, followed by low light recovery for 240 mins. The photosynthetic characteristics of alga were determined. The results showed that UVR exposure decreased photosynthetic activity, reflected by depressed maximum photochemical quantum yield (Fv/Fm) and contents of Chla and Chlc in the PA and PAB treatments. Higher content of malondialdehyde (MDA) was found in thalli exposed to UVR, which verified the damage of UVR. Electron transfer rate (ETR) was slowed down by UVR, accompanied by the increments of net closing rate of the reaction center and the energy absorbed and dissipated by unit reaction center in PSII. In these effects on photosynthesis of UVR, the PAB treatment expressed more significant inhibition, indicating a remarkable role of UVB. However, based on our results, S. horneri also took some strategies to protect itself from photodamage of UVR. UVR exposure enhanced the contents of UV-absorbing compounds (UVACs) and carotenoid, and simultaneously expedited heat consumption of excess light energy, indicated by the increased non-photochemical quenching coefficient (NPQ) in the PA and PAB treatments. Increased activities of superoxide dismutase (SOD) and peroxidase (POD), and higher content of PsbA (D1) protein were found in the treatments with UVR, which suggested that antioxidant system and the turnover of D1 protein played important roles in protection from UV-induced damages. Due to the above protection pathways, Fv/Fm and ETR gradually recovered when thalli were transferred to low light recovery. Therefore, we suggest that various protection and restoration pathways in S. horneri work together to effectively protect against UVR damage, which may be the reason why drifting populations can adapt to UVR on the seawater surface and form golden tide in case of suitable temperature and nutrients.
Introduction
The golden tide is formed by the rapid growth of certain species of Sargassum drifting on the sea surface, which are naturally born in the subtidal zone (Smetacek and Zingone, 2013). In recent years, Sargassum golden tides broke out in many places in the world, for example, S. fluitans and S. natans golden tides broke out continuously in the Caribbean, West Africa, and the Gulf of Mexico from 2011 to 2016 (Schell et al., 2015; Wang et al., 2019; Qi et al., 2020), and S. horneri golden tides broke out in the East China Sea and the Yellow Sea from 2015 to 2017 (Xing et al., 2017; Qi et al., 2017; Liu et al., 2018). When the golden tide blooms as an invasive genus, mats of drifting Sargassum can change the ecosystem structure of the original sea area (van Tussenbroek et al., 2017; Caselle et al., 2018), negatively impact offshore tourism and maritime transport (Williams and Feagin, 2010; Milledge and Harvey, 2016), and lead to the deterioration of seawater quality after it sinks and decays (Cruz-Rivera et al., 2015). If an episode were to occur in aquaculture areas, golden tides could cause enormous losses to the local mariculture industry (Xing et al., 2017). When the golden tide breaks out, the ultraviolet radiation (UVR) received by Sargassum from the stationary water to the drifting state will be significantly enhanced due to the lack of the subtracting effect of the seawater layer (Tedetti et al., 2010). Excessive UVR often causes the decrease of photosynthetic capacity of algae, resulting in photoinhibition (Gao ang Xu, 2010). The main manifestations are the decrease of antenna pigment content (Xu and Gao, 2012), the degradation of D1 protein in the reaction center (Wu et al., 2011), the decrease of effective photochemical efficiency and electron transfer rate (Yakovleva and Titlyanov, 2001; Erin Cox and Smith, 2015), and the imbalance of reactive oxygen species, ROS) metabolism (Lesser, 1996), which ultimately affects the survival and growth of algae. However, how Sargassum spp. responds to such UV stress to maintain its rapid growth remains still unclear.
Sargassum horneri belongs to Phaeophyta, Fucales, Sargassaceae and Sargassum. It is an important part of coastal algal field. Because of its rapid growth, it can create a huge algal field to provide habitat, enemy avoidance and breeding place for many organisms (Terawaki et al., 2003). Additionally, the algae can absorb a lot of N, P and other nutrients from the surrounding seawater while growing rapidly, which has a good effect on the purification of water quality (Davis et al., 2000). On the other hand, because of the high adaptability and rapid growth of S. horneri, it often becomes an invasive species (Sfriso and Facca, 2013), which affects the local marine ecosystem (van Tussenbroek et al., 2017; Byeon et al., 2019). Especially in recent years, the S. horneri golden tide which appeared in drifting population has been outbreak in the North Pacific Ocean many times, which has attracted more and more attention from all walks of life in the world (Ma et al., 2020).
Considering that S. horneri drifting on the sea surface received more light (including UVR) during the golden tide, we speculate that it may have an efficient or special photosynthetic mechanism to adapt to UVR. Therefore, in this study, we focused on UVR, the key environmental factor for drifting population and investigated the photosynthetic responses, to reveal the mechanisms of photodamage and photoprotection in this alga exposed to UVR. Our results may provide data reference for the future study in the physiological and ecological mechanism of the formation of Sargassum golden tide.
Materials and methods
Materials
Samples of Sargassum horneri were collected from a drifting population in Lidao Bay, Rongcheng City, Shandong Province, China (37°15′ N, 122°35′ E) on May 30, 2021. The collected samples were brought to the laboratory in an insulated cooler of 4°C in 2 hours. Healthy and unified individuals were selected and cleaned with autoclaved natural seawater (salinity, 30 PSU; , 25.0 mM; , 0.8 mM and DIP, 1.6 mM), and temporarily cultured for 24 hours. The temperature was set at 18°C, and the light level was 100 μmol m-2 s-1 with light and dark periods of 12 h: 12 h. The media were continuously aerated.
UVR treatments and low light recovery
The thallus branches of S. horneri with a total fresh weight (FW) of 1.0 g were cultured in a quartz tube (which can pass through UVR) filled with 500 mL of natural seawater. The quartz tube was placed in a water bath, and temperature was controlled at 18°C by a low-temperature thermostatic bath (YRDC-0506, China). Cultivation light radiations were provided by a solar simulator (Sol 1200, Hönle GmbH, Germany) and the PAR (photosynthetically active radiation, 400~700 nm), UVA (320~400 nm) and UVB (280~320 nm) irradiance were set at 1000 μmol m-2 s-1, 47.1 W m-2 and 1.5 W m-2, respectively, referring to the irradiance level on the sea surface when the golden tide of S.horneri broke out. The irradiance level was measured by a radiometer (Solar Light, PMA2100, USA). Quartz tubes were covered by different types of optical filters (Nantong Yinxing Optical Co., Ltd., China) to obtain different radiation treatments.
P treatment: The quartz tube was covered with ZJB-400 filter, which can only transmit light with wavelength above 400 nm, so that the algae in the quartz tube can only receive PAR (400~700 nm).
PA treatment: The quartz tube was covered with ZJB-320 filter. The ZJB-320 filter can only transmit light with wavelength above 320 nm, and the algae can receive PAR+UVA radiation (320~700 nm).
PAB treatment: The quartz tube was covered with ZJB-280 filter, and the algae received the light radiation of PAR+UVA+UVB (280~700 nm).
According to the duration of high light at noon and low light in afternoon during the golden tide outbreak season under natural conditions, samples with three repetitions were cultured under different radiation treatments for 120 mins, and then allowed to recovery with lower visible light of 10 μmol m-2 s-1 for 240 mins in a light incubator (GXZ-380D, Ningbo Jiangnan Instrument, China), the temperature was set at 18°C.
Fluorescence measurement and parameterization
Chlorophyll fluorescence was measured using a dual-modulation kinetic fluorometer (FL6000, Photon Systems Instruments, Czech Republic). A multiple turnover saturating flash was applied to measure the maximum quantum yield of photochemistry of photosystem II (Fv/Fm) according to (Fm−F0)/Fm. Where the difference between the maximum (Fm) and minimal fluorescence (F0) is used to calculate the variable fluorescence (Fv) (van Kooten and Snel, 1990). The non-photochemical quenching coefficient (NPQ) was calculated as (Fm−Fm′)/Fm′, where Fm′ is the maximum fluorescence measured in the presence of actinic light.
Polyphasic rise of Chl a fluorescence transients and JIP-test
Subsamples were dark adapted for 5 min, and the polyphasic rise of chlorophyll a fluorescence transients was measured by using a dual-modulation kinetic fluorometer (FL6000, Photon Systems Instruments, Czech Republic). The fluorescence signals were recorded within a time scale of 10 μs to 2 s. The JIP test was developed to characterize the fluorescence rise kinetics based on the theory of energy fluxes in the membrane in the photosynthetic apparatus (Strasser et al., 2000). According to this theory, the light system captures and absorbs light energy (ABS) through the light-trapping complex on the thylakoid membrane, a small part of which is used for heat dissipation (D) and fluorescence dissipation (F), and the rest is used for photochemical reaction (P). Most of the light energy (TR) captured by the reaction center (RCs) is used to reduce QA. After that, transfers electrons to QB for re-oxidation. In this process, the energy (ET) generated by electron transfer is used for subsequent reactions such as Calvin cycle. According to Strasser et al. (2000), the energy fluxes for absorption per reaction center (ABS/RC), the energy fluxes for dissipation per reaction center (DI0/RC), the energy fluxes for trapping per reaction center (TR0/RC), the energy flux for electron transfer from QA to per reaction center (ET0/RC), the relatively variable fluorescence at J point (VJ), the net closing rate of PSII reaction center (M0), and the probability of electron transport beyond QA (Ψ0) were calculated by the following equations:
Measurement of rapid light response curve
At room temperature, the rapid light response curves (LC) were obtained by using a palm top chlorophyll fluorescence instrument (AquaPen-C 100, Photon Systems Instruments, Czech Republic). Seven light intensity gradients (10, 20, 50, 100, 300, 500, 1000 μmol m-2 s-1) were set, and the interval light adaptation time was 10 s. The algae samples were put into AquaPen-C100 for measurement after dark adaptation for 5 min. The PSII relative electron transfer rate (rETR) was calculated from the measured φPSII, and the formula was as follows (Jassby and Platt, 1976):
In the formula, rETR is the relative electron transfer rate, PFD is the actinic light intensity, φPSII is the actual light quantum yield of PSII, and the coefficient of 0.5 indicates that about 50% of all absorbed light quantum is allocated to PSII.
The maximum relative electron transfer rate (rETRmax) and the initial slope (α) of the rapid light response curve were obtained by fitting formula, and the light saturation point Ek was calculated by rETRmax and α, and the formula were as follows:
Analysis of oxidative stress response
At the end of 120 mins UVR exposure and 240 mins low light recovery, approximately 0.2 g FW samples were collected and frozen in liquid nitrogen (-80°C) for subsequent analysis of oxidative stress response. Before determination, 1.8 mL volume of homogenate medium (physiological saline) were added. The mixture was ground into 10% tissue homogenate in ice-water bath (0°C). After the homogenizedextract was centrifuged at 3500 rpm (4°C) for 10 mins, MDA contents, SOD and POD activities were tested through assay kits (Nanjing Jiancheng Biological Engineering Company, China), respectively. The content of MDA was measured by condensation of MDA in lipid peroxide degradation products with thiobarbituric acid (TBA) to form a red product with the maximum absorption peak at 532 nm. One unit of SOD activity was defined as the amount of enzyme which resulted in a 50% inhibition of the rate of nitro-blue tetrazolium reduction at 560 nm. Based on the principle of catalysing hydrogen peroxide reaction by peroxidase, the POD activity was obtained by measuring the change of absorbance at 420 nm.
Determination of photosynthetic pigment
Approximately 0.1 g FW of thalli were ground with 90% acetone, and then adjusted constant volume to 8 mL. After the dark extraction for12 hours at 4°C, extracting solution was centrifuged for 15 mins at 4000 rpm at 4°C, and the absorbance of supernatant was determined with ultraviolet spectrophotometer (UV-2600, Shimadzu, Japan). The contents (mg g-1 FW) of chlorophyll (Chla, Chlc) and carotenoids (Car) were calculated as described by Jeffrey and Humphrey (1975) and Parsons et al. (1984), respectively.
Where A664, A630, A480, A510 indicated the absorption values at wavelengths of 664, 630, 480 and 510 nm, respectively. V represented the volume of 90% acetone, and M represented FW of algae.
Determination of UV-absorbing compounds content
Approximately 0.1 g FW of algae were ground and then extracted in 10 mL methanol for 24 hours at 4°C, and the solution was centrifuged for 15 mins at 4000 rpm. The absorbance of supernatant was scanned from 250 nm to 750 nm with ultraviolet spectrophotometer (UV-2600, Shimadzu, Japan). The ratio of peak height at 335 nm to FW of algae was used to express the relative content of UV-absorbing compounds (UVACs).
Relative quantitation of D1 proteins by immunoblotting
Algal thylakoid membrane proteins were extracted in cool extracting medium (50 mM Tris–HCl, pH 7.6, 5.0 mM MgCl2, 10 mM NaCl, 0.4 M sucrose, and 0.1% BSA) according to Ma et al. (2016). Protein concentration determined by the Bradford method. And 10 μg of extracted proteins were used for 12% separating gel (pH 8.9) in Tris-glycine buffer (pH 8.3). For immunoblotting, the SDS-PAGE gel was transferred to a PVDF membrane with a DYY-6C Transblot semi-dry transfer apparatus, according to the manufacturer’s instructions. Membranes were blocked for 60 min with 5% skimmed milk in TBS (20 mM Tris, 150 mM NaCl, pH 7.4) at 37°C. Rabbit anti-the C-terminal part of PsbA (AgriSera; 1:50000) and goat anti-rabbit IgG-HRP (1:1000) antibodies used as primary and second antibodies, respectively. All incubations were performed at room temperature. The membranes were developed with 3′3-diminobenzidine (DAB) substrate. After scanning the membranes, scan strips are analyzed by Image J image processing software, and the average optical density value of each strip was used as the relative amount of D1 protein.
Data analysis
Results were expressed as mean ± standard deviation (n = 3). SPSS 17.0 was used for statistical analysis. One-way ANOVA (Duncan) was used for difference analysis, and the difference significance level was set as P<0.05.
Results
Maximum photochemical quantum yield (Fv/Fm)
The maximum photochemical quantum yields (Fv/Fm) of S. horneri during UVR exposure and low light recovery were shown in Figure 1. Fv/Fm with the initial value of 0.750 ± 0.007 at time 0 decreased sharply by 49.47%, 57.07% and 72.40% after exposed to P, PA and PAB treatments for 120 mins, respectively. There were significant differences among the three treatments (P<0.001). In the following process of low light recovery, Fv/Fm rose to 84.93%, 78.80% and 72.00% for P, PA and PAB treatments respectively as compared with the initial value after 240 mins, there were significant difference between these treatments (P<0.001).
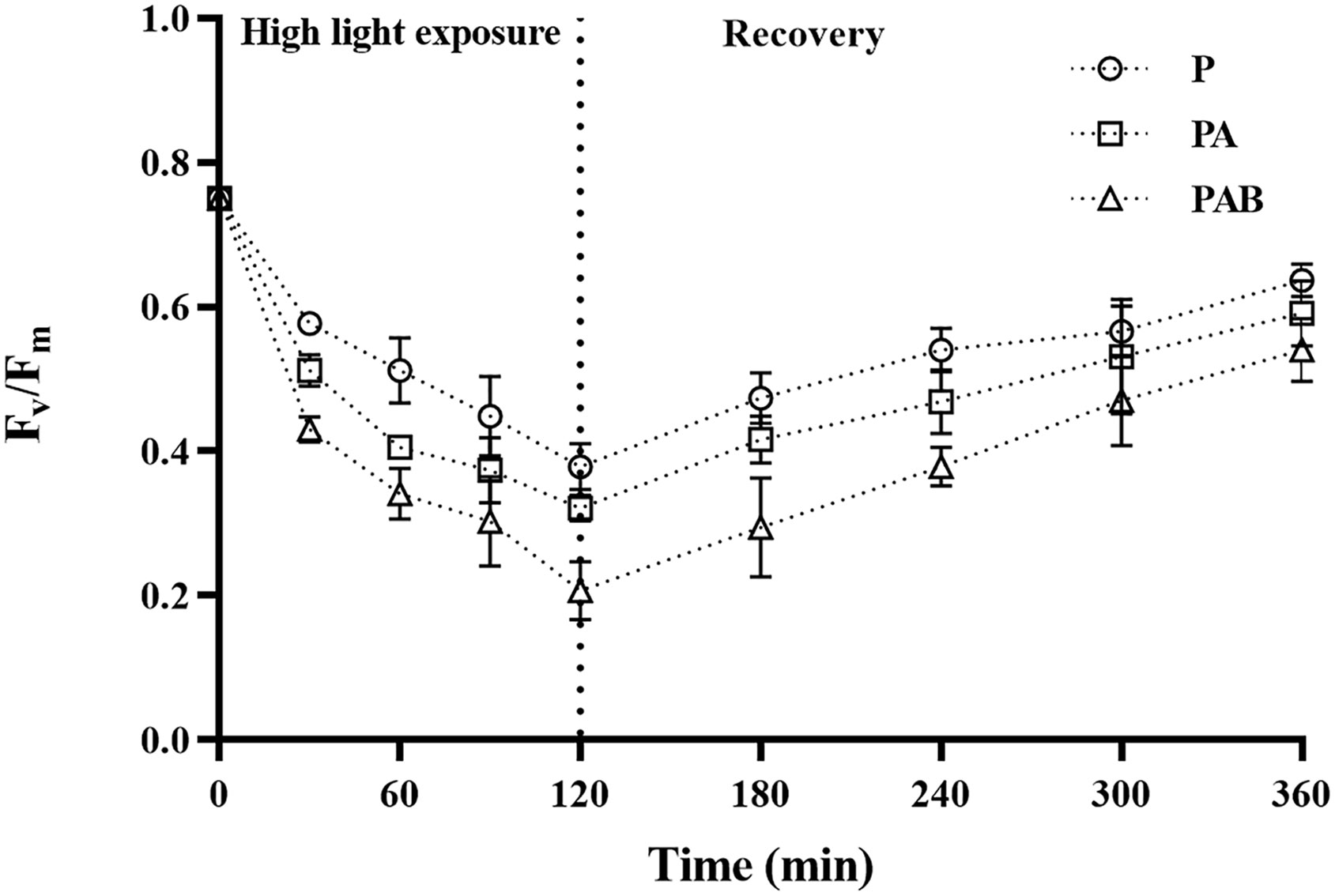
Figure 1 Changes of the maximum photochemical quantum yield (Fv/Fm) in Sargassum horneri during P, PA and PAB exposure and low light recovery. Data are means ± SD (n = 3).
Electron transfer and energy flow distribution
In order to study the effect of UV radiation on PSII electron transfer reaction, we determined the fast chlorophyll fluorescence induction of S. horneri during the P, PA and PAB exposure and the subsequent low light recovery. The results of ABS/RC, DI0/RC, TR0/RC and ET0/RC were shown in Figure 2. ABS/RC, DI0/RC and TR0/RC values gradually increased during high light exposure, PAB treatment led to the highest value of 4.74 for ABS/RC, 6.00 for DI0/RC and 1.65 for TR0/RC after 120 mins exposure, which is 2.96 times, 15.11 times and 1.54 times of P treatment, respectively. When shifted to low light, ABS/RC, DI0/RC and TR0/RC decreased, but still higher than the initial value measured before the high light treatment, the highest value was still kept under PAB treatment. However, ET0/RC declined gradually 37.99%, 43.99% and 51.46% under P, PA and PAB treatments respectively as compared with the original value, and recovered to 93.67%, 88.96% and 85.23% after 240 min recovery. In contrast to the increase of ABS/RC, DI0/RC and TR0/RC by PAB treatment, ET0/RC showed the opposite changes.
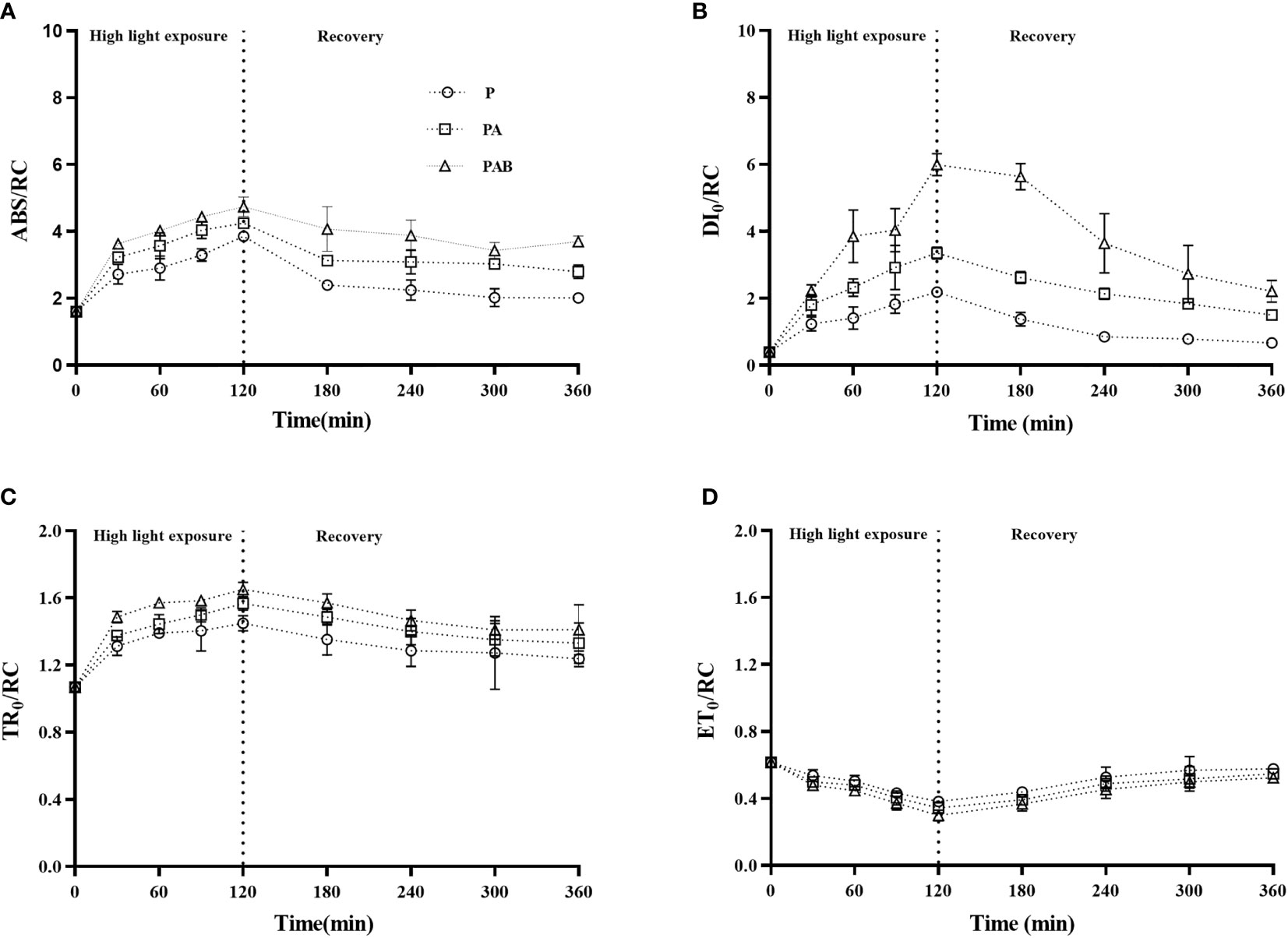
Figure 2 The energy fluxes for absorption per reaction center, ABS/RC (A), the energy fluxes for dissipation per reaction center, DI0/RC (B), the energy fluxes for trapping per reaction center, TR0/RC (C), and the energy fluxes for electron transfer from QA to QA- per reaction center, ET0/RC (D) versus time in S. horneri during P, PA and PAB exposure and low light recovery. Data are means ± SD (n = 3).
Figure 3 exhibited the changes in the relative variable fluorescence (VJ), variation of net closure rate (M0) and the probability of electron transport beyond QA (Ψ0) during UVR exposure and the subsequent low light recovery. The value of VJ increased by 53.59%, 61.75% and 69.12% under exposure to P, PA and PAB for 120 mins respectively, and then exhibit a trend of decrease during the recovery period. (Figure 3A). Through JIP-test, we also found Ψ0 was gradually reduced with the prolonged UVR exposure and showed recovery when S. horneri was shifted to low light, which further indicated high light exposure, particularly for UV radiation, could inhibit electron transfer from to QB. M0 expressed the net closing rate of reaction centers during the high light exposure, it increased as the exposure prolonged, but decreased when S. horneri was transferred to low light. The presence of UVR resulted in much higher M0 value than P treatment (P<0.001).
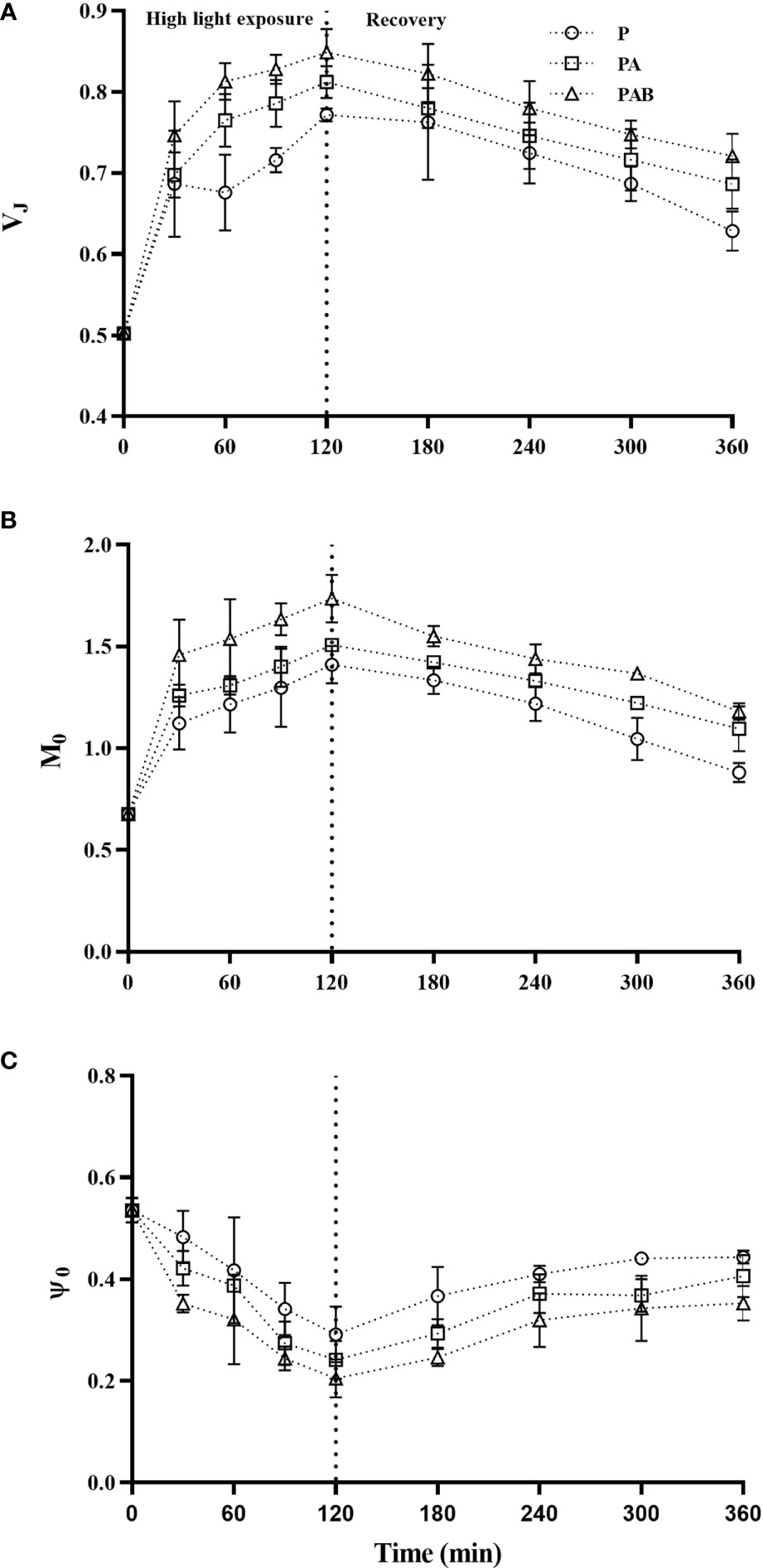
Figure 3 The relative variable fluorescence (VJ, A), approximated initial slope of the fluorescence transients (M0, B) and the probability of electron transport beyond QA (Ψ0, C) in S. horneri during P, PA and PAB exposure and low light recovery. Data are means ± SD (n = 3).
Rapid light response curve
Figure 4 showed initial slope (α), maximum relative electron transfer rate (rETRmax) and light saturation point (Ek) obtained from rapid light response curve (LC) of alga. High light exposure remarkably decreased α and rETRmax (P<0.001). Compared with PAR, the presence of UVA led to a further decrease of 55.04% and 63.11% for α and rETRmax respectively, while the presence of UVB 61.75% and 74.80%. During the following low light recovery, both α and rETRmax gradually increased, and at the end of 240 min recovery, α recovered to 82.84%, 75.75% and 65.86%, while rETRmax to 90.92%, 82.88% and 77.71% of the initial value under P, PA and PAB treatments, respectively. In contrast, the light saturation point (Ek) expressed unobvious changes with insignificant difference between three radiation treatments.
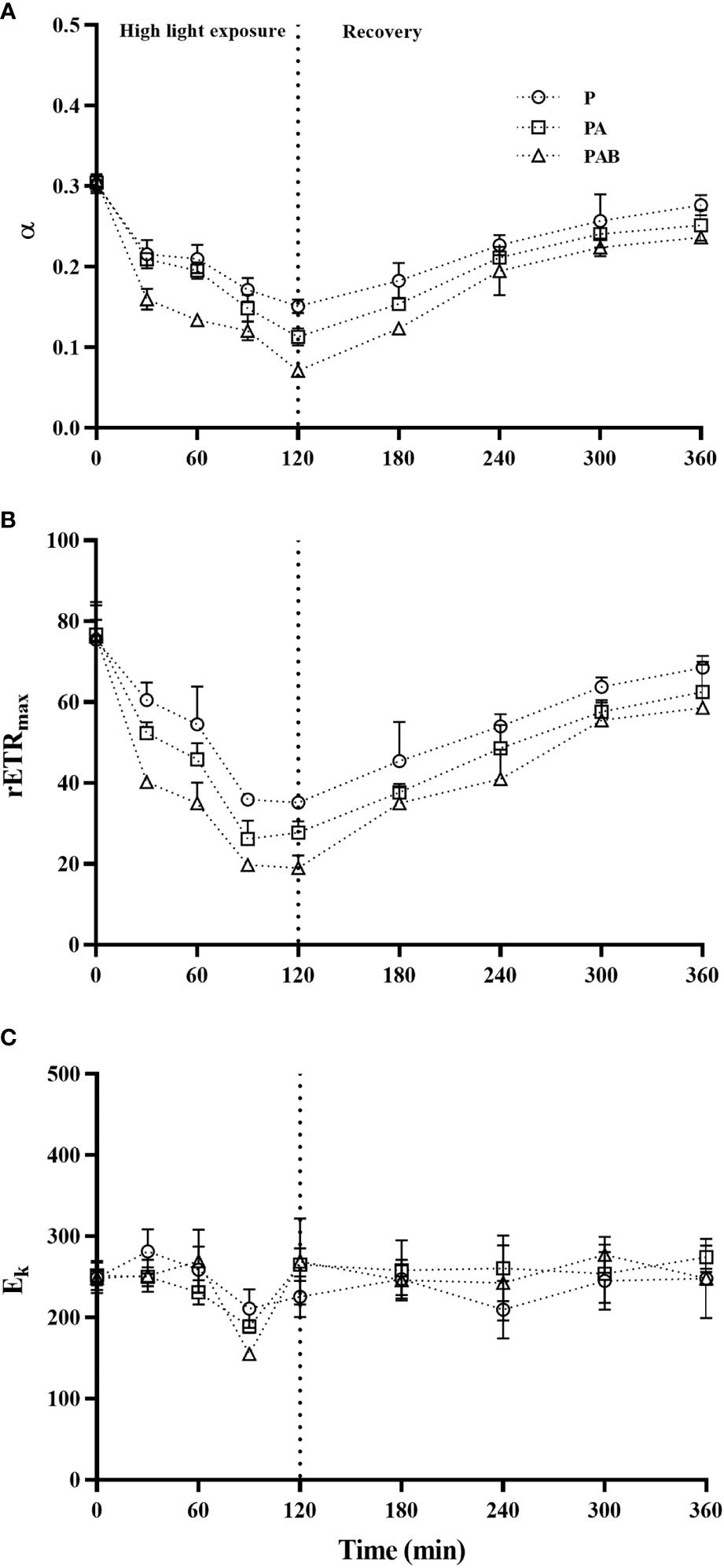
Figure 4 The photosynthetic efficiency parameter (α, A), maximum relative electron transfer rate (rETRmax, B) and light saturation point (Ek, C) versus time in S. horneri during P, PA and PAB exposure and low light recovery. Data are means ± SD (n = 3).
Non-photochemical quenching (NPQ)
NPQ of thalli gradually increased during high light exposure (Figure 5). After 120 min exposure in P, PA and PAB treatments, NPQ increased 2.91, 3.32 and 3.62 times over that of the initial value. And then after 240 mins of low light recovery, NPQ of alga exposed to these radiation treatments relaxed.
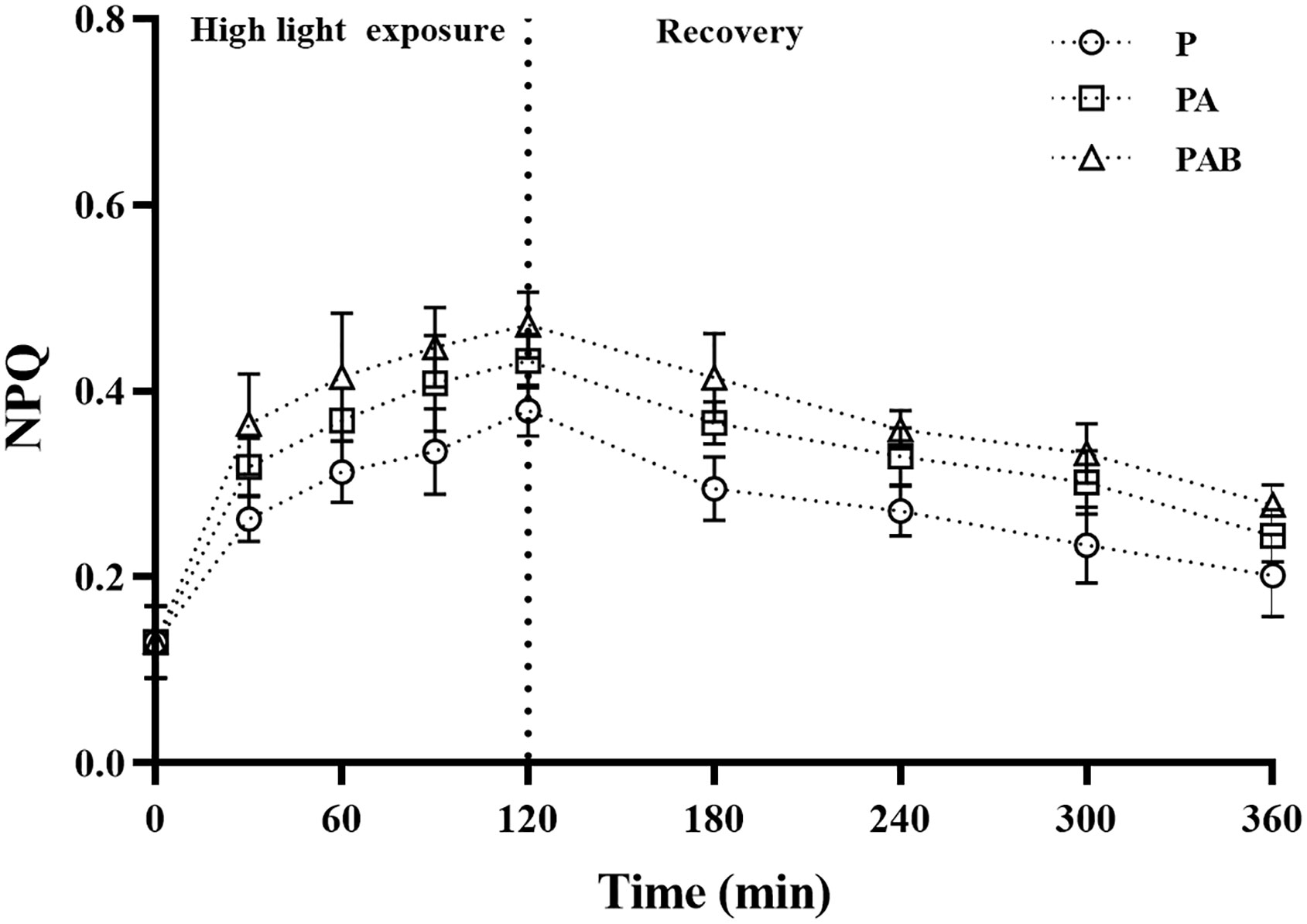
Figure 5 The non-photochemical quenching (NPQ) versus time in S. horneri during P, PA and PAB exposure and low light recovery. Data are means ± SD (n = 3).
Oxidative stress response
Figure 6 showed the content of MDA and the activities of SOD and POD during UVR exposure and following low light recovery. Significant accumulation of MDA was induced after 120-min exposure for all three radiation treatments when compared with the initial level at time 0, and further accumulation occurred during the subsequent recovery. Obviously, the presence of UVR provoked significant cumulative ROS toxicity.
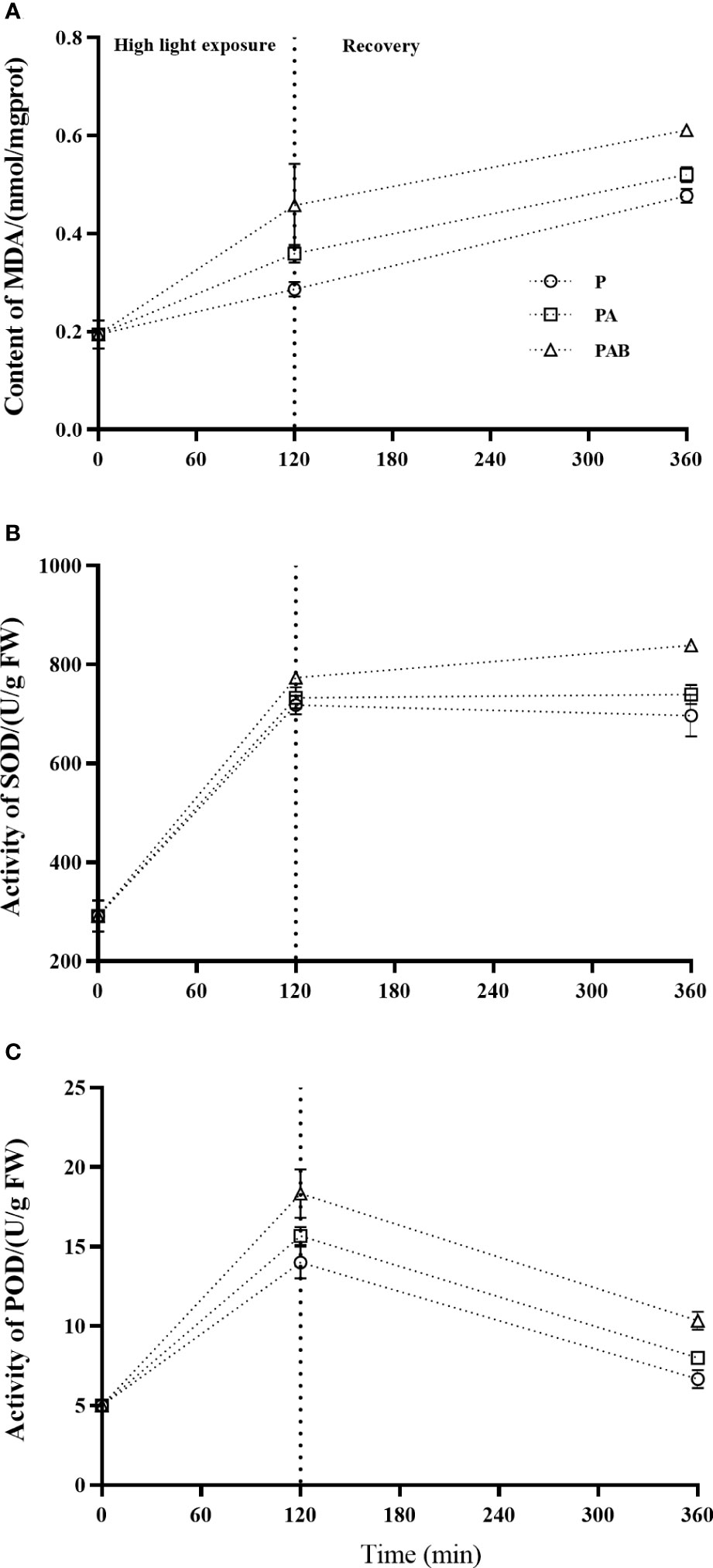
Figure 6 The MDA content (A), SOD (B) and POD (C) activities versus time in S. horneri during P, PA and PAB exposure and low light recovery. Data are means ± SD (n = 3).
The activity of SOD increased 2.47, 2.52 and 2.66 times over that of the initial value at time 0 by P, PA and PAB treatments respectively, with significant difference between P and PAB treatments (P<0.001), and showed stabilization during the 240-min recovery. The activity of POD showed a similar trend of increase during the P, PA and PAB exposure, but decreased when shifted to low light.
Pigments and UV-absorbing compounds (UVACs)
Contents of photosynthetic pigments and UVACs in thalli were exhibited in Figure 7. Compared with the initial value, both contents of Chla and Chlc reduced during UVR exposure and following low light recovery, with no significant difference between P and PA treatments. However, contents of these two pigments expressed lower value in PAB treatment than in P and PA treatments, whether after UVR exposure or low light recovery (Figures 7A, B). Additionally, after 240 min low light recovery, Chla content of alga cultured in P, PA and PAB treatments returned to 90.91%, 87.25% and 72.86% of the initial value, respectively (P=0.002).
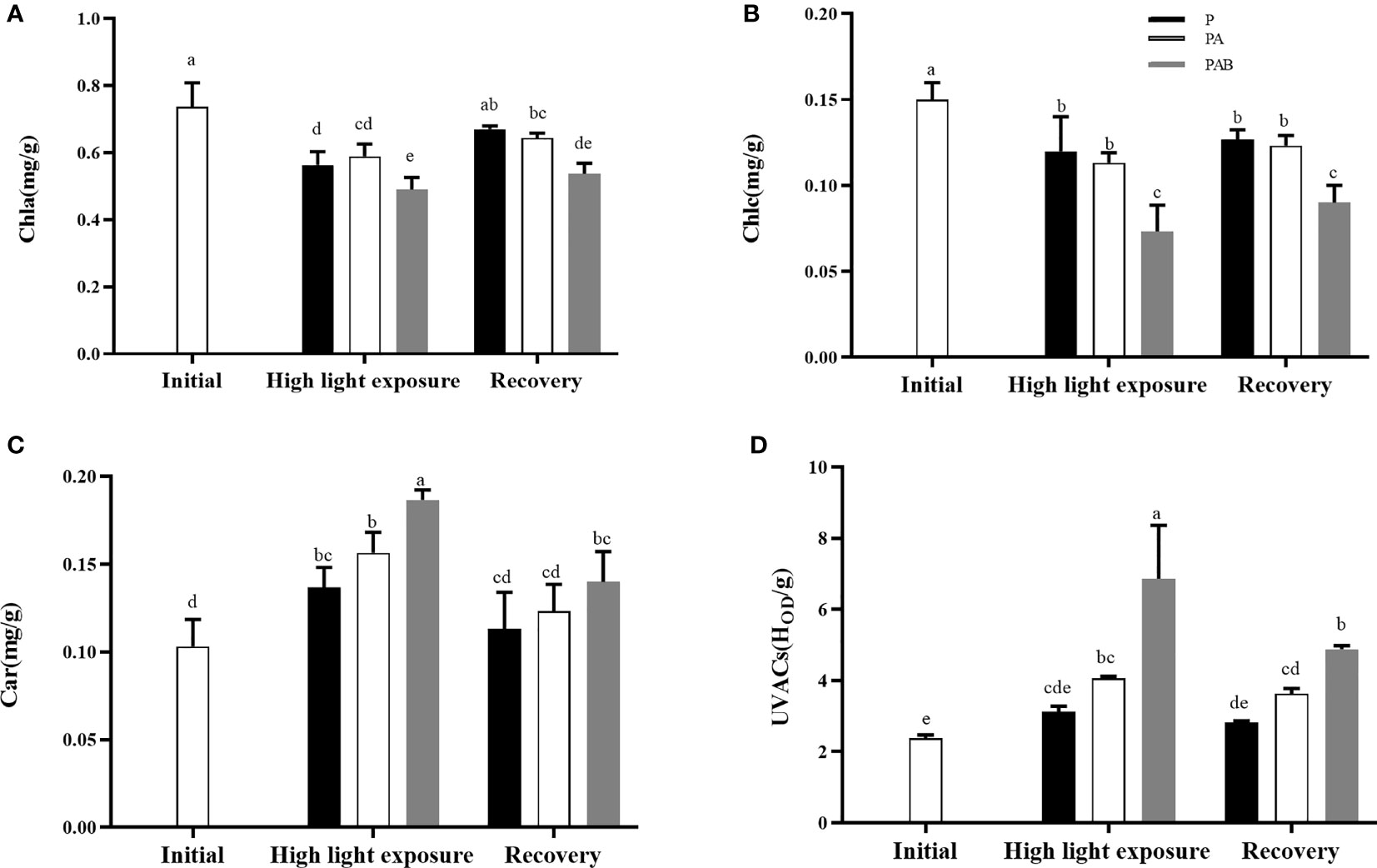
Figure 7 The contents of Chla (A), Chlc (B), Car (C) and UV-absorbing compounds (UVACs, (D) versus time in S. horneri during P, PA and PAB exposure and low light recovery. Data are means ± SD (n=3). Significant (P<0.05) differences among the treatments are indicated by different lowercase letters.
Contrarily, content of Car dramatically increased after P, PA and PAB exposure (P<0.05). Furthermore, PA and PAB treatments respectively enhanced it by 14.60% and 36.50%, compared with the P treatment. After 240 min low light recovery, the content of Car decreased to the initial value level in P and PA treatments (P=0.139), while still maintained a relatively high value in PAB treatment.
UVACs was not induced by P treatment. However, PA and PAB treatments enhanced it by 1.71 and 2.89 times after UVR exposure, respectively, indicating that both UVA and UVB promoted the synthesis of UVACs in alga. After 240 min low light recovery, UVACs content in PAB treatment still remained higher than the other radiation treatments.
Relative quantitation of D1 proteins
Figure 8 showed the relative D1 protein content in alga during UVR exposure and low light recovery. The D1 protein content was enhanced by UVR exposure, especially UVB. After low light recovery for 240 mins, D1 protein content increased in P and PA treatment, but decreased in PAB treatment, compared with that under high-light exposure.
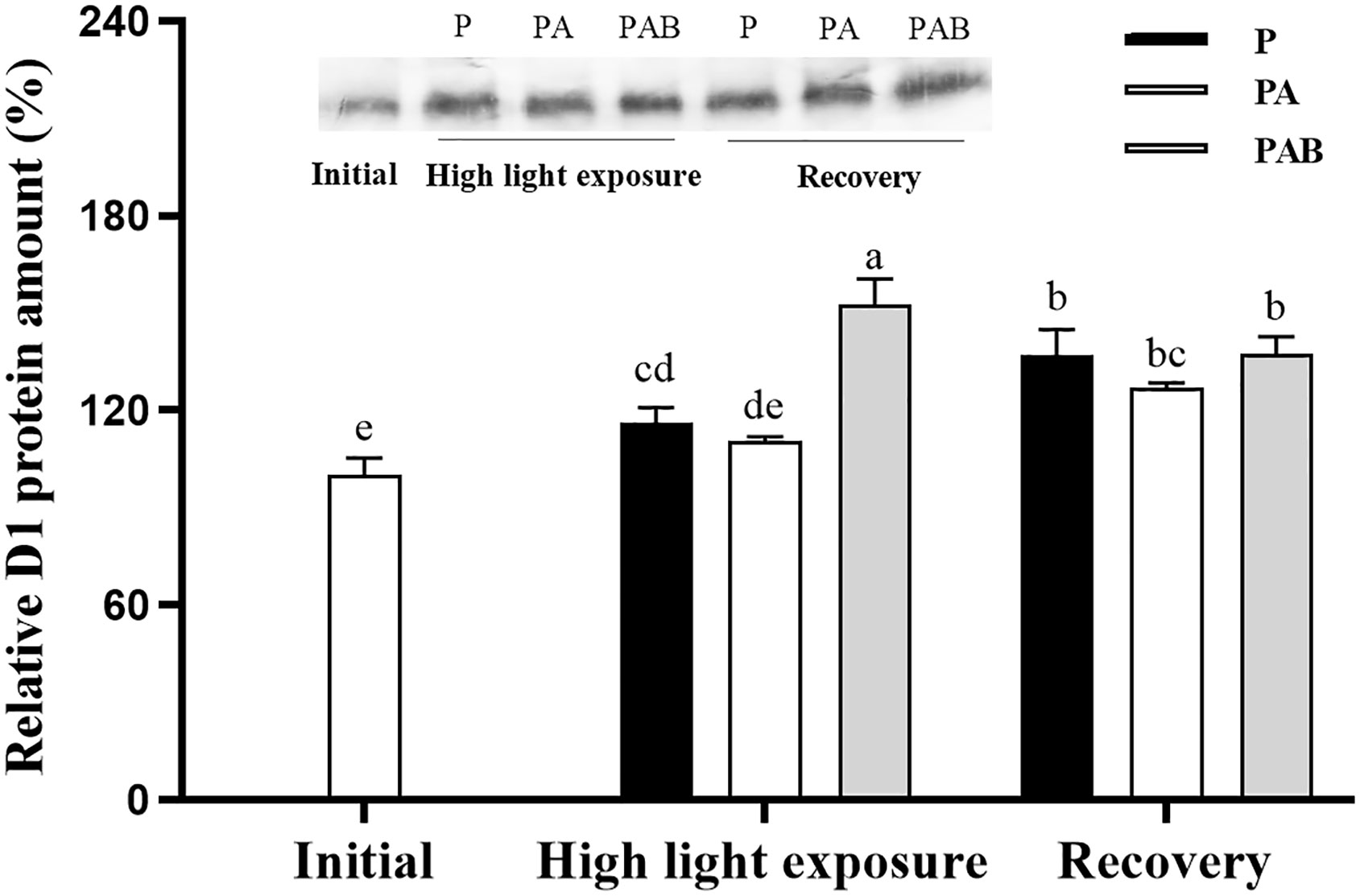
Figure 8 Changes of the relative D1 protein content in S. horneri after exposed to P, PA and PAB treatments and low light recovery. The data are shown after normalization to the value at the initial. Data are means ± SD (n = 3). Significant (P < 0.05) differences among the treatments are indicated by different lowercase letters.
Discussion
Photosynthesis is the most basic and important physiological activity of algae, which can quickly respond to UV radiation. Generally, UV radiation directly acts on photosynthetic organs, and PSII is the most sensitive to UV radiation, especially UV-B, and suffers the most serious light suppression (Spetea et al., 1996; Vass et al., 2002). The maximum photochemical quantum yield of PSII (Fv/Fm) reflects the quantum yield when all PSII reaction centers are open, and it will decrease significantly when algae are under environmental stress. Therefore, Fv/Fm is an important index to study the effects of photoinhibition or various environmental stresses on photosynthesis (Kolber and Falkowski, 1988). In this study, Fv/Fm of S. horneri decreased under strong light treatment, and this stress was aggravated by UVA and UVB. Meanwhile, the stress degree of algae was related to the duration UVR exposure, and the longer the time, the higher the stress degree. This might be due to damaged reaction center pigments and reduced electron transfer rate by UVR. In fact, it was found in the present study that UVR exposure decreased the contents of Chla and Chlc, and inhibited the electron transfer from to QB in S. horneri, reflecting by increased VJ and decreased Ψ0 (Strasser et al., 2000). Therefore, α and rETRmax also decreased with UVR exposure, indicating that the number of active PSII reaction centers of S. horneri decreased by UVR, which was consistent with the results in Porphyra leucosticte, P. umbilicalis and Grateloupia lanceola (Korbee et al., 2005; Huovinen et al., 2006).
Furthermore, under UVR stress, electrons are hard to transfer from Pheo on the receptor side to QA on the secondary electron acceptor in time, resulting in increased QA and P680+. Eventually, PSII will be damaged by 1O2 and P680+, which have strong oxidizability (reviewed by Nishiyama and Murata, 2014). In this study, the MDA contents of S. horneri cultured in PA and PAB treatments were significantly higher than that in the P treatment, reflecting the increased productions of reactive oxygen species (ROS) during UVR exposure, which led to membrane lipid peroxidation (Saber et al., 2020). Similar result was found in Chlorococcum sp. (Abo-Shady et al., 2008).
Macroalgae have evolved in a series of protective mechanisms under UVR stress (Gao and Xu, 2020). Photosynthetic pigments, located on the surface of thylakoid membrane, firstly capture excess energy under UVR (Conde et al., 2004), leading to its structural disintegration, but also protecting the reaction center of PSII (Franklin et al., 2003). Therefore, in this study, the decrease of Chla and Chlc in S. horneri might play an important role in UVR protection of photoreaction centers. Significantly, the content of carotenoids in S. horneri increased with UVR exposure in this study. Carotenoids are important antenna pigments, which can not only capture light energy, but also protect cells from damage through antioxidation (Conde et al., 2004). UVR can induce cells to produce ROS, which will cause structural and compositional damage to photosynthetic organs, for example, resulting in elevated MDA as shown in this study. Carotenoids have antioxidant effects in two ways. One is to quench the excited chlorophyll by self-epoxidation, so as to prevent the production of active oxygen; the other is to directly quench active oxygen. Therefore, the increase of carotenoid content is a protection strategy for algae (Lvarez-Gómez et al., 2019). Additionally, the content of UVACs in S. horneri also increased in PA and PAB treatments, with an absorption peak in UV band, which could shield UVR and protect important intracellular organelles from UV stress (Peinado et al., 2010; Lvarez-Gómez et al., 2019; Gao and Xu, 2020). Actually, it has been reported in many species of alga, including micro- and macroalgae, that UVA and/or UVB can induce the synthesis of UVACs (Yakovleva and Titlyanov, 2001; Han and Han, 2005; Xu and Gao, 2012; Lvarez-Gómez et al., 2019; Gao and Xu, 2020).
In the present study, UVR forced algae to improve the efficiency of the remaining active reaction center, that was, under UV stress, the energy absorbed (ABS/RC) and dissipated (DIo/RC) by the unit active reaction center of S. horneri increased, thus dissipating the excess energy in the electron transfer chain (Gert et al., 2010). Additionally, UVR, especially UVB, increased the NPQ of S. horneri in this study. Xanthophyll cycle can partially resist or accelerate the recovery of algae damaged by UVR through dissipating energy (Zudaire and Roy, 2001). The higher NPQ is related to the accumulation of zeaxanthin in algae (Garcia-Mendoza et al., 2011). Therefore, S. horneri might improve the photo-protective ability to UVR through NPQ related to xanthophyll cycle.
Antioxidant system is another effective way for algae to protect themselves from UVR damage (Foyer et al., 1994), for example, superoxide dismutase (SOD) can catalyze the decomposition of superoxide anion radical ( ) in algae into hydrogen peroxide and molecular oxygen (Grene et al., 2002); and peroxidase (POD) can remove hydrogen peroxide and effectively avoid the accumulation of hydrogen peroxide and organic peroxide in algae (Zhang and Kirkham, 1993). The enzyme activities of SOD and POD in brown algae are generally at a lower level, compared with those in green and red algae. However, Aguilera et al. (2002) found that the activities of these two enzymes in Monostroma affarcticum significantly increased after UVR exposure when the ice broke. Similarly, S. horneri exposed to UVR had higher activities of SOD and POD in this study, which may play an important role in damage repair for drifting thalli to adapt to UVR stress on the sea surface.
It has been reported in some micro- and macroalgae that UV-induced ROS damaged D1 protein in reaction center proteins of PSII, resulting in decreased content of it (Nishiyama and Murata, 2014). Correspondingly, algae under environmental stress could alleviate damage to the photosystem by accelerating the synthesis of D1 protein, whose starting time and speed varied with the species of algae, reflecting the role of D1 protein synthesis in repairing damage (Zhang and Aro, 2002; Wu et al., 2011; Ma et al., 2020). In the present study, the content of D1 protein in S. horneri increased significantly after 120min PAB exposure, with the increased SOD and POD activities, indicating that the accelerated synthesis of D1 protein had started rapidly due to the removal of ROS by SOD and POD. This might suggest that the rapid turnover of D1 protein play an important role in the repair of photodamage of S. horneri. Actually, we have subsequently confirmed this conclusion by adding streptomycin to inhibit D1 protein synthesis, and found that the Fv/Fm of S. horneri exposed to UVR decreased sharply in a short time (Our unpublished data). Moreover, during the 240 min low light recovery period, D1 protein maintained high content in this study, accompanied by the recovery of Fv/Fm and electron transfer rate, which also verified the key role of D1 protein synthesis in UVR protection of S. horneri.
Conclusion
Drifting S. horneri is exposed to increased UVR, resulting that the membrane system is damaged, the maximum photochemical quantum yield decreases, electron transfer rate reduced, and the photosynthetic pigment content decreases. However, this alga can protect and repair its photosynthetic system through a series of efficient pathways, such as synthesizing UVACs, enhancing NPQ, improving SOD and POD activities, and accelerating D1 protein turnover. Various protection and restoration pathways in S. horneri work together to effectively protect against UVR damage, which enable the S. horneri to adapt to the UVR on the sea surface, and thus may form a golden tide when temperature and nutrition conditions are appropriate.
Data availability statement
The original contributions presented in the study are included in the article/supplementary material. Further inquiries can be directed to the corresponding authors.
Author contributions
ZX and LXL conceived of and designed the experiment with help from YM and HW. ZX and LXL carried out the experiment, with help from HJ and FY. The data analyses were performed by ZX and LXL, with help from HJ, FY, LJL, and SZ. ZX and LXL wrote the manuscript with contributions from HJ, FY, LJL, SZ, YM, and HW. All authors reviewed and gave their approval for the final manuscript.
Funding
This work was supported by Shandong Provincial Natural Science Foundation of China to Xu Z (ZR2020MD092) and Zang S (ZR2020QC025).
Conflict of interest
The authors declare that the research was conducted in the absence of any commercial or financial relationships that could be construed as a potential conflict of interest.
Publisher’s note
All claims expressed in this article are solely those of the authors and do not necessarily represent those of their affiliated organizations, or those of the publisher, the editors and the reviewers. Any product that may be evaluated in this article, or claim that may be made by its manufacturer, is not guaranteed or endorsed by the publisher.
References
Abo-Shady A. M., El-Sheekh M. M., El-Naggar A. H. (2008). Effect of UV-b radiation on growth, photosynthetic activity and metabolic activities of Chlorococcum sp. Ann. Microbiol. 58 (1), 21–27. doi: 10.1007/BF03179440
Aguilera J., Bischof K., Karsten U. (2002). Seasonal variation in ecophysiological patterns in macroalgae from an Arctic fjord. II. Pigment accumulation and biochemical defence systems against high light stress. Mar. Biol. 140 (6), 1087–1095. doi: 10.1007/s00227-002-0792-y
Byeon S. Y., Oh H. J., Kim S., Yun S. H., Kang J. H., Park S. R., et al. (2019). The origin and population genetic structure of the 'golden tide' seaweeds, Sargassum horneri, in Korean waters. Sci. Rep-UK. 9, 7757. doi: 10.1038/s41598-019-44170-x
Caselle J. E., Davis K., Marks L. M. (2018). Marine management affects the invasion success of a nonnative species in a temperate reef system in California, USA. Ecol. Lett. 21, 43–53. doi: 10.1111/ele.12869
Conde F. R., Churio M. S., Previtali C. M. (2004). The deactivation pathways of the excited-states of the mycosporine-like amino acids shinorine and porphyra-334 in aqueous solution. Photochem. Photobiol. Sci. 3 (10), 960–967. doi: 10.1039/b405782a
Cruz-Rivera E., Flores-Díaz M. C., Hawkins A. (2015). A fish kill coincident with dense Sargassum accumulation in a tropical bay. B. Mar. Sci. 91, 455–456. doi: 10.5343/bms.2015.1048
Davis T. A., Volesky B., Vieira R. (2000). Sargassum seaweed as biosorbent for heavy metals. Water Res. 34 (17), 4270–4278. doi: 10.1016/S0043-1354(00)00177-9
Erin Cox T., Smith C. M. (2015). Photosynthetic rapid light curves for Padina sanctaecrucis vary with irradiance, aerial exposure, and tides in hawaii’s micro−intertidal zones. Mar. Biol. 162, 1061–1076. doi: 10.1007/s00227-015-2649-1
Foyer C. H., Descourvoeres P., Kunert K. J. (1994). Protection against oxygen radicals: An important defense mechanism studied in transgenic plants. Plant Cell Environ. 17 (5), 507–523. doi: 10.1111/j.1365-3040.1994.tb00146.x
Franklin L. A., Osmond C. B., Larkum A., Larkum A. W. D., Douglas S. E., Raven J. A. (2003). “Photoinhibition, UV-b and algal photosynthesis,” in Photosynthesis in algae: Advances in photosynthesis and respiration (Dordrecht: Springer), 364–375. doi: 10.1007/978-94-007-1038-2_16
Gao K. S, Xu J. T (2008). Ecological and physiological responses of macroalgae to solar and UV radiation. In: Seckbach J, Einav R, Israel A (eds.), Seaweeds and their role in globally changing environments, cellular origin, life in extreme habitats and astrobiology. Dordrecht: Springer. 15, 183–198. doi: 10.1007/978-90-481-8569-6_11
Gao K. S., Xu J. T. (2020). “Ecological and physiological responses of macroalgae to solar and UV radiation,” in Seaweeds and their role in globally changing environments, cellular origin, life in extreme habitats and astrobiology. Eds. Seckbach J., Einav R., Israel A. (Dordrecht, South Holland: Springer Netherlands), 183–198. doi: 10.1007/978-90-481-8569-6_11
Garcia-Mendoza E., Ocampo-Alvarez H., Govindjee (2011). Photoprotection in the brown alga Macrocystis pyrifera: Evolutionary implications. J. Photoch. Photobiol. B. 104 (1-2), 377–385. doi: 10.1016/j.jphotobiol.2011.04.004
Gert H. J., Krüger, Tsimilli-Michael M., Strasser R. J. (2010). Light stress provokes plastic and elastic modifications in structure and function of photosystem II in camellia leaves. Physiol. Plantarum 101 (2), 265–277. doi: 10.1111/j.1399-3054.1997.tb00996.x
Grene A. R., Neval E., Heath L. S. (2002). Role of superoxide dismutases (SODs) in controlling oxidative stress in plants. J. Exp. Bot. 372), 1331–1341. doi: 10.1093/jexbot/53.372.1331
Han Y. S., Han T. (2005). UV-B induction of UV-b protection in Ulva pertusa (Chlorophyta). J. Phycol. 41 (3), 523–530. doi: 10.1111/j.1529-8817.2005.00072.x
Huovinen P., Matos J., Pinto I. S., Figueroa F. (2006). The role of ammonium in photoprotection against high irradiance in the red alga Grateloupia lanceola. Aquat. Bot. 84 (4), 308–316. doi: 10.1016/j.aquabot.2005.12.002
Jassby A. D., Platt T (1976). Mathematical formulation of the relationship between photosynthesis and light for phytoplankton. Limnol. Oceanogr. 21 (4), 540–547. doi: 10.4319/lo.1976.21.4.0540
Jeffrey S. W., Humphrey G. F. (1975). New spectrophotometric equations for determining chlorophylls a, b, c1 and c2 in higher plants, algae and natural phytoplankton. Biochem. Physiol. Pflanzen. 167 (2), 191–194. doi: 10.1016/S0015-3796(17)30778-3
Kolber Z., Falkowski Z. P. (1988). Effects of growth irradiance and nitrogen limitation on photosynthetic energy conversion in photosystem II. Plant Physiol. 88 (3), 923–929. doi: 10.1104/pp.88.3.923
Korbee N., Huovinen P., Figueroa F. L., Aguilera J., Karsten U. (2005). Availability of ammonium influences photosynthesis and the accumulation of mycosporine-like amino acids in two Porphyra species (Bangiales, rhodophyta). Mar. Biol. 146 (4), 645–654. doi: 10.1007/s00227-004-1484-6
Lesser M. P. (1996). Acclimation of phytoplankton to UV-b radiation: oxidative stress and photoinhibition of photosynthesis are not prevented by UV-absorbing compounds in the dinoflagellate Prorocentrum micans. Mar. Ecol. Prog. Ser. 132 (1-3), 287–297. doi: 10.3354/meps132287
Liu F., Liu X. F., Wang Y., Jin Z., Moejes F. W., Sun S. (2018). Insights on the Sargassum horneri golden tides in the yellow Sea inferred from morphological and molecular data. Limnol. Oceanogr. 63, 1762–1773. doi: 10.1002/lno.10806
Lvarez-Gómez F., Korbee N., Figueroa F. L. (2019). Effects of UV radiation on photosynthesis, antioxidant capacity and the accumulation of bioactive compounds in Gracilariopsis longissima, Hydropuntia cornea and Halopithys incurva (Rhodophyta). J. Phycol. 55 (6), 1258–1273. doi: 10.1111/jpy.12899
Ma J., Lv C. F., Xu M. L., Chen G. X., Lv C. G., Gao Z. P. (2016). Photosynthesis performance, antioxidant enzymes, and ultrastructural analyses of rice seedlings under chromium stress. Environmen. Sci. pollut. R. 23, 1768–1778. doi: 10.1007/s11356-015-5439-x
Ma J., Wang W., Liu X. Y., Wang Z. Q., Gao G., Wu H. L. (2020). Zinc toxicity alters the photosynthetic response of red alga Pyropia yezoensis to ocean acidification. Environmen. Sci. Pollut. R. 27, 3202–3212. doi: 10.1007/s11356-019-06872-7
Milledge J. J., Harvey P. J. (2016). Golden tides: problem or golden opportunity? The valorization of Sargassum from beach inundations. J. Mar. Sci. Eng. 4, 1–19. doi: 10.3390/jmse4030060
Nishiyama Y., Murata N. (2014). Revised scheme for the mechanism of photoinhibition and its application to enhance the abiotic stress tolerance of the photosynthetic machinery. Appl. Microbiol. Biotechnol. 98, 8777–8796. doi: 10.1007/s00253-014-6020-0
Parsons T. R., Maita Y., Lalli C. M. (1984). A manual of chemical and biological methods for seawater analysis (New York, NY: Pergamon Press), 173. doi: 10.1016/B978-0-08-030287-4.50034-7
Peinado N. K., Díaz R. T. A., Figueroa F. L. (2010). Ammonium and UV radiation stimulate the accumulation of mycosporine-like amino acids in Porphyra columbina (Rhodophyta) from Patagonia, Argentina. J. Phycol. 40 (2), 248–259. doi: 10.1046/j.1529-8817.2004.03013.x
Qi L., Hu C. M., Mikelsons K., Wang M. H., Lance V., Sun S. J., et al. (2020). In search of floating algae and other organisms in global oceans and lakes. Remote Sen. Environ. 239, 111659. doi: 10.1016/j.rse.2020.111659
Qi L., Hu C. M., Wang M. Q., Shang S. L., Wilson C. (2017). Floating algae blooms in the East China Sea. Geophys. Res. Lett. 44, 11501–11509. doi: 10.1002/2017GL075525
Saber H., El-Sheekh M. M., Ibrahim A. (2020). Effect of UV-b radiation on amino acids profile, antioxidant enzymes and lipid peroxidation of some cyanobacteria and green algae. Int. J. Radiat. Biol. 96 (9), 1–30. doi: 10.1080/09553002.2020.1793025
Schell J. M., Goodwin D. S., Siuda A. N. S. (2015). Recent Sargassum inundation events in the Caribbean: Shipboard observations reveal dominance of a previously rare form. Oceanography 28, 8–10. doi: 10.5670/oceanog.2015.70
Sfriso A., Facca C. (2013). Annual growth and environmental relationships of the invasive species Sargassum muticum and Undaria pinnatifida in the lagoon of Venice. Estuar. Coast. Shelf S. 129 (1), 162–172. doi: 10.1016/j.ecss.2013.05.031
Smetacek V., Zingone A. (2013). Green and golden seaweed tides on the rise. Nature 504, 84–88. doi: 10.1038/nature12860
Spetea C., Hideg É., Vass I. (1996). The quinone electron acceptors are not the main sensitizers of UV-b induced protein damage in isolated photosystem II reaction centre and core complexes. Plant Sci. 115 (2), 207–215. doi: 10.1016/0168-9452(96)04347-6
Strasser R. J., Srivastava A., Tsimilli-Michael M., Yunus M., Pathre U., Mohantz P. (2000). “The fluorescence transient as a tool to characterize and screen photosynthetic samples,” in Probing photosynthesis: mechanisms regulation and adaptation, vol. 25. (London: Taylor and Francis), 445–483.
Tedetti M., Guigue C., Goutx M. (2010). Utilization of a submersible UV fluorometer for monitoring anthropogenic inputs in the Mediterranean coastal waters. Mar. Pollut. Bull. 60 (3), 350–362. doi: 10.1016/j.marpolbul.2009.10.018
Terawaki T., Yoshikawa K., Yoshida G., Uchimura M., Iseki K. (2003). Ecology and restoration techniques for sargassum beds in the seto inland Sea, Japan. Mar. Pollut. Bull. 47 (1), 198–201. doi: 10.1016/S0025-326X(03)00054-7
van Kooten O., Snel J. (1990). The use of chlorophyll fluorescence nomenclature in plant stress physiology. Photosynth. Res. 25, 147–150. doi: 10.1007/BF00033156
van Tussenbroek B. I., Arana H. A. H., Rodríguez-Martínez R. E., Espinoza-Avalos J., Canizales-Flores H. M., González-Godoy C. E., et al. (2017). Severe impacts of brown tides caused by Sargassum spp. on near-shore Caribbean seagrass communities. Mar. Pollut. Bull. 122, 272–281. doi: 10.1016/j.marpolbul.2017.06.057
Vass I., Turcsányi E., Touloupakis E., Ghanotakis D., Petrouleas V. (2002). The mechanism of UV-a radiation-induced inhibition of photosystem II electron transport studied by EPR and chlorophyll fluorescence. Biochemistry 41 (32), 10200–10208. doi: 10.1021/bi020272+
Wang M. Q., Hu C. M., Barnes B. B., Mitchum G., Lapointe B., Montoya J. P. (2019). The great Atlantic Sargassum belt. Science 365, 83–87. doi: 10.1126/science.aaw7912
Williams A., Feagin R. (2010). Sargassum as a natural solution to enhance dune plant growth. Environ. Manage. 46, 738–747. doi: 10.1007/s00267-010-9558-3
Wu H. Y., Cockshutt A. M., McCarthy A., Campbell D. A. (2011). Distinctive photosystem II photoinactivation and protein dynamics in marine diatoms. Plant Physiol. 156, 2184–2195. doi: 10.1104/pp.111.178772
Xing Q. G., Guo R. H., Wu L. L., An D. Y., Cong M., Qin S. (2017). High-resolution satellite observations of a new hazard of golden tides caused by floating Sargassum in winter in the yellow Sea. IEEE Geosci. Remote. Sens. Lett. 14, 1815–1819. doi: 10.1109/LGRS.2017.2737079
Xu Z. G., Gao K. S. (2012). enrichment and UV radiation interact to affect the photosynthesis and nitrogen uptake of Gracilaria lemaneiformis (Rhodophyta). Mar. Pollut. Bull. 64, 99–105. doi: 10.1016/j.marpolbul.2011.10.016
Yakovleva I. M., Titlyanov E. A. (2001). Effect of high visible and UV irradiance on subtidal Chondrus crispus: Stress, photoinhibition and protective mechanisms. Aquat. Bot. 71, 47–61. doi: 10.1016/S0304-3770(01)00167-X
Zhang L., Aro E. M. (2002). Synthesis, membrane insertion and assembly of the chloroplast-encoded D1 protein into photosystem II. FEBS Letters. 512 (1-3), 13–18. doi: 10.1016/S0014-5793(02)02218-4
Zhang J., Kirkham M. B. (1993). Drought-stress-induced changes in activities of superoxide dismutase, catalase, and peroxidase in wheat species. Plant Cell Physiol. 35 (5), 785–791. doi: 10.1093/oxfordjournals.pcp.a078658
Keywords: Sargassum horneri, UVR, photosynthesis, photodamage, photoprotection
Citation: Xu Z, Li L, Jiang H, Yan F, Liu L, Zang S, Ma Y and Wu H (2022) Photosynthetic responses of a golden tide alga (Sargassum horneri) to ultraviolet radiation. Front. Mar. Sci. 9:978376. doi: 10.3389/fmars.2022.978376
Received: 26 June 2022; Accepted: 11 July 2022;
Published: 04 August 2022.
Edited by:
Guang Gao, Xiamen University, ChinaCopyright © 2022 Xu, Li, Jiang, Yan, Liu, Zang, Ma and Wu. This is an open-access article distributed under the terms of the Creative Commons Attribution License (CC BY). The use, distribution or reproduction in other forums is permitted, provided the original author(s) and the copyright owner(s) are credited and that the original publication in this journal is cited, in accordance with accepted academic practice. No use, distribution or reproduction is permitted which does not comply with these terms.
*Correspondence: Yuanqing Ma, c2RtYXl1YW5xaW5nQDE2My5jb20=; Hongyan Wu, c2R3dWhvbmd5YW5AMTI2LmNvbQ==
†These authors have contributed equally to this work