- 1School of Aquatic and Fishery Sciences, College of the Environment, University of Washington, Seattle, WA, United States
- 2Bodega Marine Laboratory, Coastal and Marine Sciences Institute, College of Biological Sciences, University of California, Davis, Bodega Bay, CA, United States
- 3Alaska Fisheries Science Center, National Marine Fisheries Service, National Oceanic and Atmospheric Administration, Seattle, WA, United States
- 4Department of Biology, Woods Hole Oceanographic Institution, Woods Hole, MA, United States
- 5Land and Food Systems, University of British Columbia, Vancouver, BC, Canada
Sexual reproduction is a fundamental process essential for species persistence, evolution, and diversity. However, unprecedented oceanographic shifts due to climate change can impact physiological processes, with important implications for sexual reproduction. Identifying bottlenecks and vulnerable stages in reproductive cycles will enable better prediction of the organism, population, community, and global-level consequences of ocean change. This article reviews how ocean acidification impacts sexual reproductive processes in marine invertebrates and highlights current research gaps. We focus on five economically and ecologically important taxonomic groups: cnidarians, crustaceans, echinoderms, molluscs and ascidians. We discuss the spatial and temporal variability of experimental designs, identify trends of performance in acidified conditions in the context of early reproductive traits (gametogenesis, fertilization, and reproductive resource allocation), and provide a quantitative meta-analysis of the published literature to assess the effects of low pH on fertilization rates across taxa. A total of 129 published studies investigated the effects of ocean acidification on 122 species in selected taxa. The impact of ocean acidification is dependent on taxa, the specific reproductive process examined, and study location. Our meta-analysis reveals that fertilization rate decreases as pH decreases, but effects are taxa-specific. Echinoderm fertilization appears more sensitive than molluscs to pH changes, and while data are limited, fertilization in cnidarians may be the most sensitive. Studies with echinoderms and bivalve molluscs are prevalent, while crustaceans and cephalopods are among the least studied species even though they constitute some of the largest fisheries worldwide. This lack of information has important implications for commercial aquaculture, wild fisheries, and conservation and restoration of wild populations. We recommend that studies expose organisms to different ocean acidification levels during the entire gametogenic cycle, and not only during the final stages before gametes or larvae are released. We argue for increased focus on fundamental reproductive processes and associated molecular mechanisms that may be vulnerable to shifts in ocean chemistry. Our recommendations for future research will allow for a better understanding of how reproduction in invertebrates will be affected in the context of a rapidly changing environment.
Introduction
Global climate change is impacting physical, biological, and chemical processes in the marine environment (Hoegh-Guldberg and Bruno, 2010; Howes et al., 2015; Shukla et al., 2019). Absorption of excess carbon dioxide by seawater lowers pH and reduces carbonate ion concentration and aragonite saturation state (Doney et al., 2009). These alterations in water chemistry are referred to as ocean acidification (OA) and can impact marine organisms at multiple levels of biological organization (Melzner et al., 2019). At the organismal level, changing chemistry can influence physiological processes (e.g., calcification, internal pH control, respiration and nutrient uptake), affecting performance and survival (Kroeker et al., 2010; Howes et al., 2015; Melzner et al., 2019). Although there is extensive research on the effects of OA in marine invertebrates, gaps in our understanding of how OA affects sexual reproductive processes (Figure 1) constrain predictions of species persistence, evolutionary adaptation, and biodiversity. For example, acidification could result in decoupling of biological and environmental cues, leading to reproductive failure with significant consequences for population dynamics in marine ecosystems (Shlesinger and Loya, 2019; Olischläger and Wild, 2020) (Figure 2).
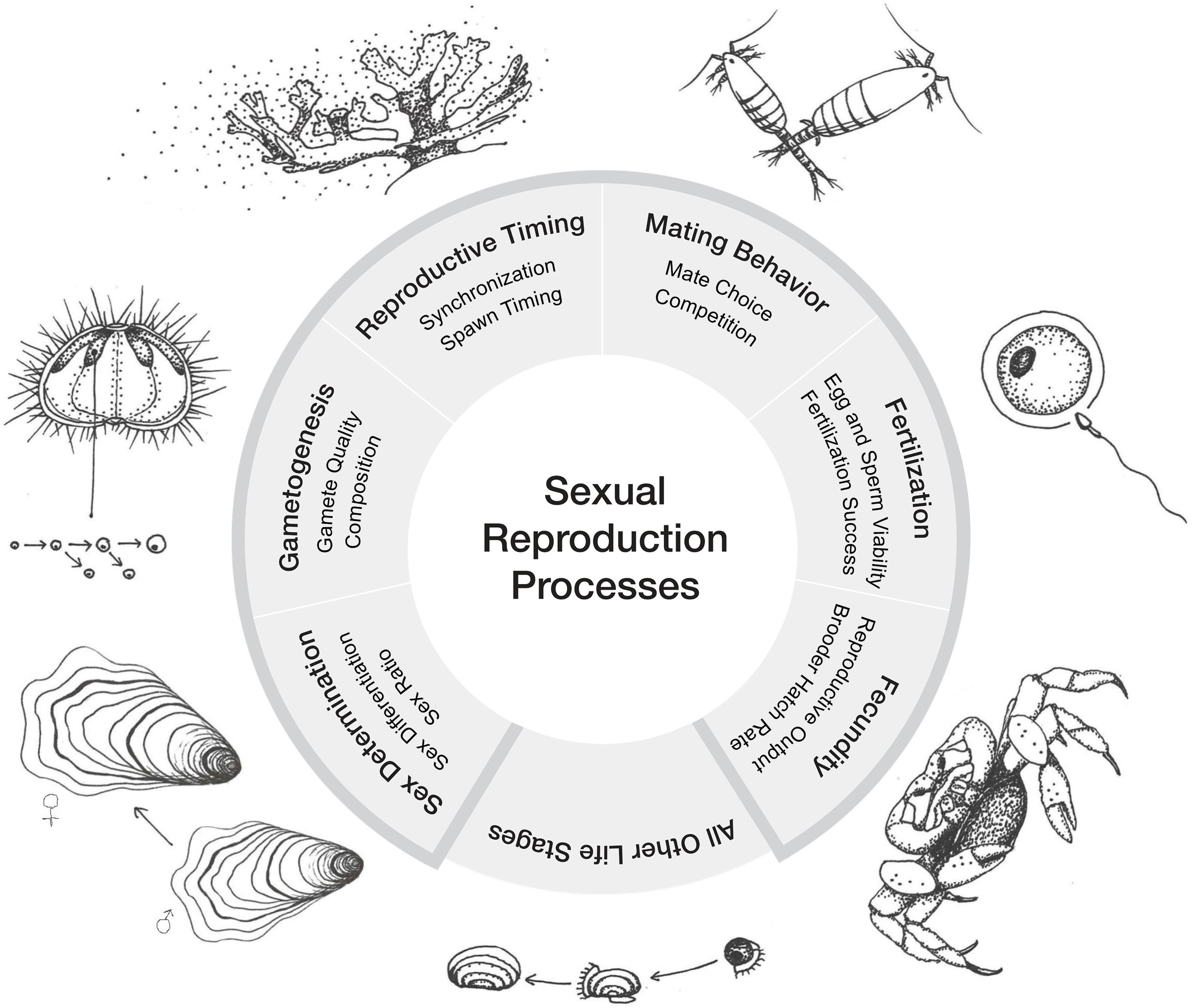
Figure 1 Sexual reproduction processes in marine invertebrates. The six processes discussed in this review are presented clockwise beginning with Sex Determination. Sex determination is the initial event that determines whether the gonads will develop as testes or ovaries and has great importance to the consideration of sex ratios, particularly for non-hermaphroditic species. Mechanisms for sex determination can be genetic or environmental. Sex differentiation is the development of sex differences after determination. Gametogenesis is the process of gamete formation which can lead to gametes with different characteristics (i.e., size, reserves, viability). Reproductive timing includes synchronization of gamete development, spawning, and any other reproductive process. Mate selection, courtship display, and mate guarding are common mating behaviors. Fertilization success depends on gamete quality, reproductive timing, mating behavior and synchronization. Fecundity is a measure of reproductive potential of an organism.
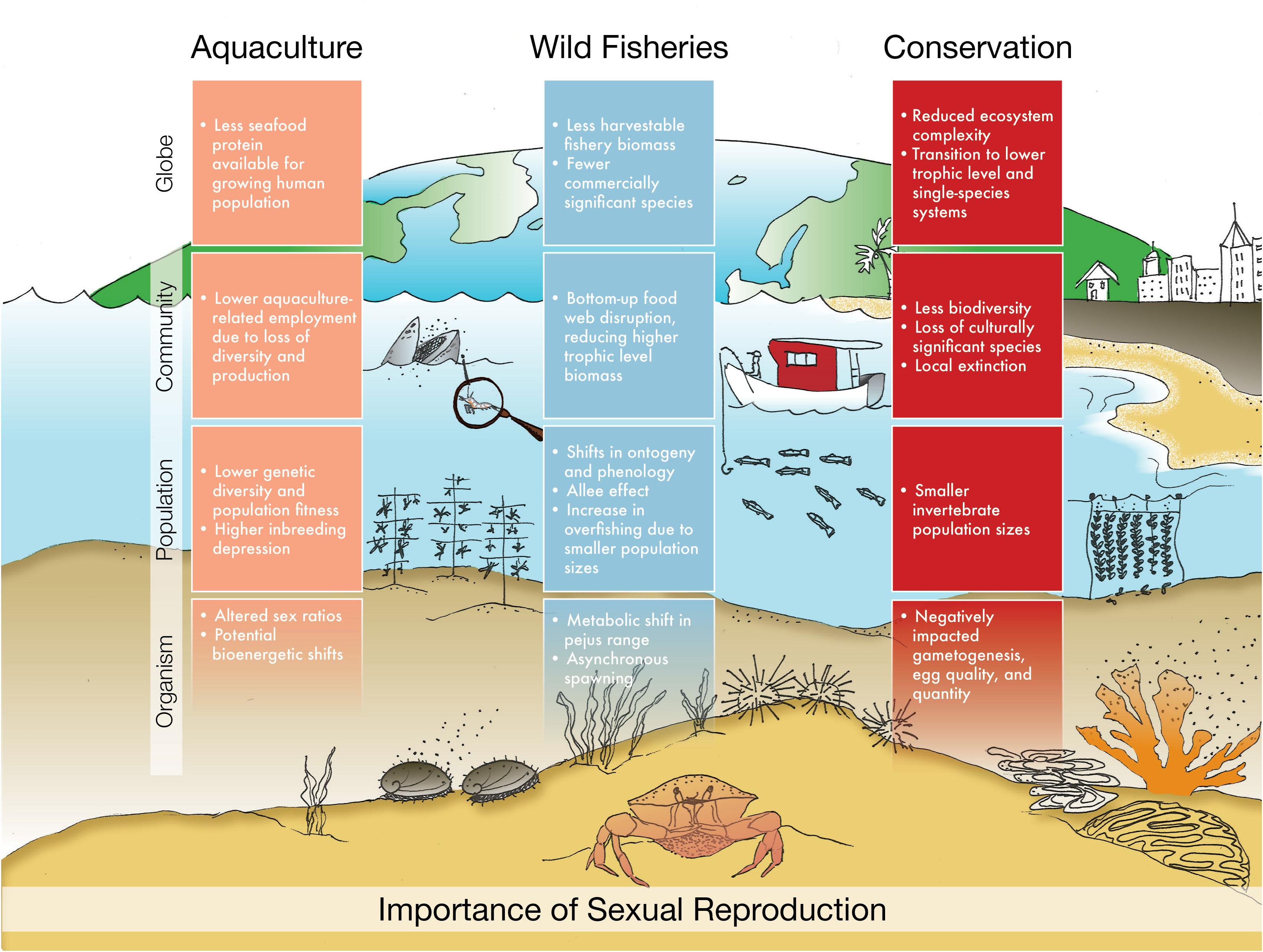
Figure 2 Potential effects of OA-caused changes in reproductive processes at the organism, population, community, and global level for commercial and restoration aquaculture, wild fisheries, and conservation and restoration of wild populations. Implications mentioned are not necessarily exclusive to one category.
Reproduction is a complex process that relies on numerous mechanisms (Figure 1, Box 1); using multiple parameters to evaluate reproductive performance is fundamental to predict how fitness may be impacted by future environmental conditions. OA research has centered around processes during early-life history stages (embryos, larvae, and juveniles), and previous reviews have synthesized trends in organismal responses to OA (Kurihara et al., 2008; Dupont et al., 2010; Albright, 2011b; Ross et al., 2011; Byrne and Przeslawski, 2013; Przeslawski et al., 2015; Ross et al., 2016; Foo and Byrne, 2017). While larval or juvenile performance can be indicative of successful reproduction, an appropriate chemical and physical environment for gamete development, spawning, mating behavior, and fertilization success is also crucial.
Box 1. Glossary of key reproduction terms.
Egg fertilization mechanisms: molecular processes in eggs involved in successful fertilization, including release of egg-derived chemicals, egg-sperm fusion, egg activation and polyspermy prevention
Egg receptivity: the length of time an egg can be fertilized by a sperm cell
Exogenous vs. endogenous reproductive cues: external environmental cues vs. cues originating within the organism
F1: first filial generation comprised of offspring resulting from a cross between two individuals from parental generation
Fecundity: number of eggs (or embryos) per individual. For colonial organisms, the number or eggs or sperm per module (i.e., polyp) or number of fecund modules per weight unit, body length or projected area
Fertilization: union of male and female gametes during sexual reproduction to form a zygote
Gamete quality: size, composition, and developmental stage of gametes
Gametogenesis: process by which gametes are produced through meiosis and cell differentiation. Formation of ova occurs through oogenesis and spermatozoa through spermatogenesis
Gonad condition: Assessment of gonad development via histological examination
Gonad Index (GI) or Gonadosomatic Index (GSI): gonad mass as a proportion of the total body mass [(gonad weight / total tissue weight) X 100]
Gravid: carrying eggs or young offspring
Hatching success: percentage of eggs which produce viable offspring
Indeterminate vs. determinate sex: ability to change sex during an organism’s lifetime vs having a defined sex at birth
Mating behavior: social interaction that prepares for, or increases the success of, copulation and fertilization
Mass spawning: synchronous release of gametes by many species or the majority of a mating aggregation
Oocyte: cell in an ovary which may undergo meiotic division to form an ovum
Oosorption (atresia): process of resorbing vitellogenic eggs under stress to reuse lipids for other physiological processes
Phenology: study of periodic events in biological life cycles and how they are influenced by local, seasonal, and interannual environmental variation
Polyspermy: occurs when an egg is fertilized by more than one sperm
Reproductively inactive vs. sexually immature: sexually mature individual not presently breeding vs. one not old or big enough to undergo gametogenesis
Reproductive output: number of reproductive elements (spermary or egg) per unit body volume. Can also be measured by counting embryos per unit body volume. In colonial organisms, reproductive output is the total amount of gametes released by the colony. In some cases, fecundity and reproductive output are used interchangeably
Resource allocation: proportion of an organism’s energy budget allocated to reproduction
Sex determination: initial event before sex differentiation that determines whether gonads will develop as male or female
Sex differentiation: events after sex determination that ultimately produce either the male or female sexual phenotype
Sire and dam: father and mother of a genetic line
Spawned bundle: sperm and/or egg clusters released to the water column for external fertilization
Sperm activity: sperm swimming behavior, including motility, velocity or speed, and path linearity
Sperm linearity: how straight the sperm is swimming, as calculated by the ratio of average velocity on a straight line from start to endpoint of the sperm’s path to the curvilinear velocity along the sperm’s path
Sperm velocity: defined by (1) curvilinear velocity along the sperm’s path, (2) smoothed average sperm path velocity, (3) average velocity on a straight line from start to endpoint of the sperm’s path
Spermatocyte: male gametocyte from which spermatozoa develop
Standardized Gonad Index (SGI): reproductive cycle indicator based on the differences between the observed and expected weights of the gonads for an individual of a given size. Takes into account allometric gonadal growth
Synchronization: coordination of reproductive events to increase mating potential, fertilization, and offspring success
Timing of reproduction: milestones that rely on environmental and chronological cues
Vitellogenins: principal precursors to the yolk proteins (the vitellins) of egg‐laying animals, but not present in all marine invertebrates
Volitional vs. strip or induced spawning: release of gametes via natural or hormonal means vs artificially releasing gametes through anthropogenic means
In 2016, nearly 26 million tons of invertebrates were produced for consumption via commercial aquaculture, comprising 32% of global aquaculture and 77% of marine and coastal aquaculture that year (FAO, 2018). Invertebrate aquaculture is increasingly leveraged by restoration groups to enhance wild populations that are struggling to recover naturally (Froehlich et al., 2017; Wasson et al., 2020). Both commercial and restoration aquaculture depend upon reliable sources of viable gametes or larvae, which are either produced in controlled hatchery conditions or collected from the wild (Helm et al., 2004; Washington Sea Grant, 2015). Aquaculture production could be hampered if acidification decreases reproductive capacity (Figure 2). In contrast to aquaculture, wild fisheries depend upon an organism’s ability to reproduce in the natural environment; any impact of acidification on reproduction will directly influence wild stock available for fisheries. Threatened and endangered marine invertebrates increasingly require human interventions to conserve and restore populations (Elliott et al., 2007). Success of these programs is contingent upon a population’s ability to persist naturally and successfully reproduce once interventions cease. Therefore, it is critical that aquaculture, fisheries, and conservation groups identify whether reproductive processes will be impacted by low pH, and how that will impact their focal species’ abundance and distribution (Figure 2).
In this review we synthesize the literature to date that has explored how OA impacts reproductive processes of five ecologically, economically, and culturally important marine invertebrate taxa: cnidarians, crustaceans, echinoderms, molluscs, and ascidians. These groups play important roles as keystone species, ecological engineers, aquaculture and fishery products, and model organisms in developmental biology. Our synthesis identifies important gaps in knowledge of the physiological mechanisms involved in reproduction in response to OA in invertebrate taxa important to aquaculture, fisheries, and conservation efforts. In addition to fertilization and brooding, we focus on pre-fertilization processes (Figure 1) that are poorly understood both mechanistically and in the context of climate change. Finally, we provide recommendations for future research to better understand how invertebrate sexual reproduction will be affected in the context of a rapidly changing environment.
Methods
To identify empirical studies for this review, we searched the literature databases ProQuest, Web of Science, Google Scholar, and European Project on Ocean Acidification (EPOCA) for articles published in English-language journals through the end of 2021 that examined the effects of OA on reproduction in our focal taxa (Table 1). Keywords included British and American spelling for each taxonomic group. We used Boolean search operators to specify or expand our search results. ProQuest, Web of Science, and Google Scholar queries were sorted by relevance and at minimum 350 results were manually reviewed. We searched all available papers on EPOCA. Google Scholar limits word count for each search, so we broke searches down into several iterations, using the list of common terms in each search and alternating through the taxa-specific terms. We included studies featuring experiments in laboratory and field conditions that included several spatial (m to km) and temporal (minutes to years) scales. We did not include gray literature or observational studies. Throughout the manuscript we will refer to ocean acidification, OA, low pH, or acidification interchangeably.
For each study, the effect of OA on reproduction was determined for the following processes: 1) sex determination, differentiation, and ratio, 2) gametogenesis and gamete quality, 3) fecundity and reproductive output, 4) timing of reproduction and synchronization, 5) mating behavior, and 6) fertilization (Figure 1). While we acknowledge that reproductive processes are linked, we define specific metrics associated with individual processes used to categorize all findings (Box 1). The number of independent findings per reproductive process was summarized for each taxonomic group. If a study examined multiple reproductive processes, they were categorized independently. Studies that investigated multiple response variables within a single reproductive process (e.g., fertilization rate and sperm motility) and reported an effect of OA on at least one response variable were classified as showing an effect on reproduction.
To characterize geographic location of research, study site and collection site were assigned to one of the 12 Marine Realms of the World (Spalding et al., 2007). If organisms were collected from a different marine realm than where the experiment took place, only the collection location was considered.
Given the extensive literature that quantified and reported fertilization rate in varying pH conditions, we conducted a meta-analysis to explore the response of fertilization to reduced pH in cnidarians, echinoderms, and molluscs. Crustaceans and ascidians were excluded as no studies have directly measured fertilization rate in varying pH treatments. A generalized linear mixed model was developed to estimate fertilization rate by pH using a logit-linked beta distribution. When reported, the following metrics were extracted from publications, then tested as candidate predictor variables for fertilization success with Analysis of Deviance and chi-squared statistics: 1) difference between experimental and control pH (ΔpH), 2) phylum, 3) lower taxonomic group (e.g. urchin, oyster, coral), 4) incubation time, 5) sperm concentration, 6) sperm:egg ratio, 7) number of sires, 8) number of dams, and 9) pH of the control treatment (Supplemental Table 3). Analysis of covariance (ANCOVA) and the Akaike Information Criterion (AIC) were used to identify the maximal predictive model using variables that significantly affected fertilization rate as sole predictors or as covariates with ΔpH. Significance of intercepts and slope estimates were determined using z-tests. Fertilization data from multiple stressor studies were included in the analysis for all treatments (i.e., all temperature, dissolved oxygen, and salinity treatments) to capture the effect of pH on fertilization rate across a range of environmental conditions. Data from treatments that combined OA and secondary metal contaminants and cocaine byproduct stressors were excluded. When necessary, fertilization rate estimates were extracted from published figures using WebPlotDigitizer v4.2. In addition to exploring fertilization rates across all experimental pH levels (6.0 - 8.5), we also examined the response of fertilization to pH using conditions more relevant to OA (pH >7.6) (IPCC, 2022). For all models tested, study was included as a random effect. Data were weighted by the inverse of one plus the sampling variance squared (1/(1+σ2)2), which was determined from the fertilization mean, error rate (standard deviation), and number of trials conducted at each pH level. The value 1 was added to every sampling variance to prevent overinflation of low variances (between 0-1) in models (Fiorenza et al., 2020). Resultant p-values were considered significant if they were less than α = 0.05.
Of the 70 studies that experimentally tested fertilization rates in multiple pH conditions, six were omitted (one, four, and one in echinoderms, molluscs, and cnidarians, respectively) due to missing error rates (corresponding authors were contacted). The resulting meta-analysis included data from 35, 23, and 6 studies in echinoderms, molluscs, and cnidarians, respectively. We recognize that the fertilization rate reported by some studies may represent both fertilization rate and early embryonic development success. Other reproductive processes included in this review (Figure 1) were not considered for meta-analyses given the inconsistent metrics reported and/or the limited number of studies (Figure 3C).
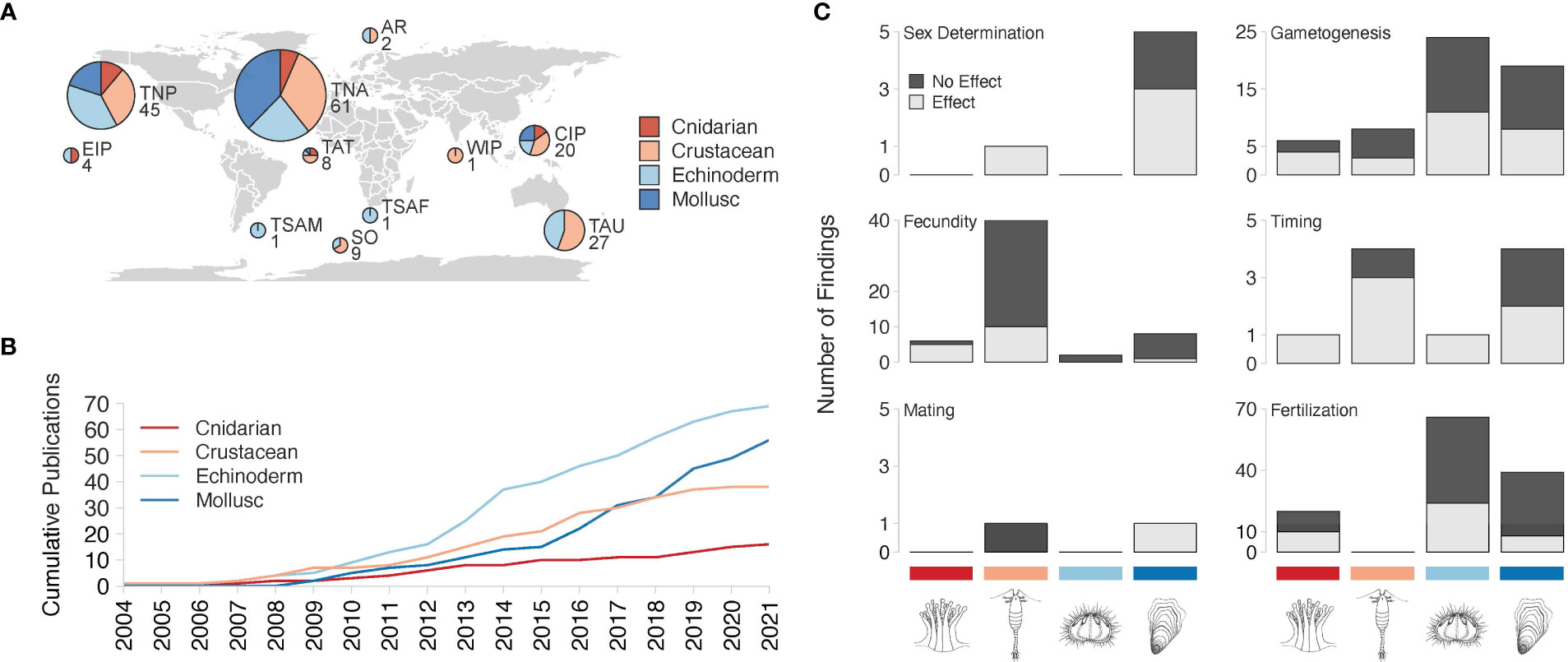
Figure 3 Summary of study locations, number of publications, and effects of Ocean Acidification on Reproductive Processes across taxa. (A) Graphical description of published studies used in this review. Pie charts for each geographic study locations are labeled with initials and the number of studies from that realm: Eastern Indo-Pacific (4), Temperate Northern Pacific (45), Temperate South America (1), Temperate Northern Atlantic (61), Tropical Atlantic (8), Southern Ocean (9), Temperate Southern Africa (1), Arctic (2), Western Indo-Pacific (1), Central Indo-Pacific (20), and Temperate Australasia (27). There were no studies from Tropical Eastern Pacific. Pie charts represent the proportion of taxa-specific studies in which study organisms were collected in each region, and pie size and numbers underneath the pies represent the total number of studies in that region. Studies may be represented more than once in a pie chart if the study examined multiple taxa. (B) Cumulative number of papers published for each taxon from 2004 to 2021. One echinoderm study was published in 1924 but was not included in this figure. Studies may be represented more than once per year if the study examined multiple taxa. (C) Number of findings that reported an OA effect (either positive or negative, light gray) or no effect (black) for various reproductive processes by taxa (from left to right: cnidarians, crustaceans, echinoderms, molluscs).
Results and discussion
We identified a total of 129 studies (16 cnidarian studies, 25 crustacean studies, 69 echinoderm studies, and 56 molluscan studies) that investigated the effects of OA on reproduction in 122 species total (19 cnidarian species, 38 crustacean species, 30 echinoderm species, and 35 mollusc species; Supplemental Table 1). Only two studies examining OA impacts on ascidian reproduction were identified. In ascidian Ciona robusta, OA significantly decreased sperm motility, mitochondrial membrane potential (MMP), and intracellular pH (pHi) (Gallo et al., 2019) but did not affect sperm intracellular reactive oxygen species (ROS), lipid peroxidation, nor viability (Gallo et al., 2019; Esposito et al., 2020). In situ and microcosm experiments showed that in C. robusta, OA initially decreased sperm motility, viability, MMP, pHi, and morphology, but sperm underwent rapid recovery after within one week of exposure, suggesting that ascidian spermatozoa is resilient to OA (Gallo et al., 2019). Given the lack of ascidian studies, the remainder of this review will focus on the remaining four taxa.
Echinoderm research comprises the majority (53.5%) of OA and reproduction studies, followed by research in molluscs (43.4%) (Figures 3A, B). The same echinoderm and mollusc species are examined in multiple studies, while cnidarian and crustacean studies tend to study unique species (Supplementary Table 1). Except for one echinoderm study published in 1924, all OA and reproduction research highlighted in this review was conducted between 2004 and 2021 (Figure 3B). The majority of the studies were performed under laboratory conditions (Supplementary Table 2) highlighting the need for more research in mesocosms and the natural environment. Life stages exposed to OA and duration of experiments were variable among taxa (Supplementary Table 2). Most studies collected organisms from the Temperate Northern Atlantic Marine Realms (Spalding et al., 2007), followed by Temperate Northern Pacific and Temperate Australasia (Figure 3A). Collection sites are reported in Figure 3A, and study sites (which in some instances differ from collection location) are included in Supplemental Table 1.
Published research suggests that the effects of OA depend on the taxa and the specific process studied (Figure 3C). Within each taxon, OA studies focus on a handful of species. Copepods dominated crustacean literature, while urchin species were prominent in echinoderm studies. All cnidarian studies examined corals. There was only one cephalopod study within molluscan research.
Impacts of ocean acidification on sexual reproduction processes
In this section, we summarize ocean acidification studies for cnidarians, crustaceans, echinoderms, and molluscs by reproductive process (Supplemental Table 1). See the Supplementary Materials for a more comprehensive synthesis of results.
Sex determination, differentiation, and ratio
The sex of many invertebrate species is sensitive to environmental conditions, which can skew populations’ sex ratios (Korpelainen, 1990; Yusa, 2007). Changes to the number and proportion of mature females and males at a given time can alter a population’s reproductive capacity, its effective population size, and its genetic diversity. There are no studies that have directly examined effects of acidification on sex determination or differentiation in individuals of any taxa covered in this review. However, we identified several studies that examined effects of acidification on sex ratios. The sole crustacean study did not find differences in sex ratios in copepods when reared in OA conditions (Kita et al., 2013). Two studies that exposed oysters to low pH prior to and during gametogenesis also reported no impact on gonad sex ratios (Venkataraman et al., 2019; Clements et al., 2020). In contrast, three oyster studies indicated that acidification exposure during gametogenesis may alter populations’ sex ratios by impacting egg and sperm development unequally. Sex-specific impacts of OA on gametogenesis therefore reduce the proportion of females (Boulais et al., 2017) or males (Parker et al., 2018; Spencer et al., 2020) capable of breeding in a population, thereby reducing the effective population size. These studies are challenging due to the difficulty of identifying sex without harming or destroying the organisms being investigated (Ellis et al., 2017), the lack of sex biomarkers, and the limited understanding of hormonal control on reproductive processes in marine invertebrates.
Gametogenesis and gamete quality
Environmental conditions during gametogenesis can influence population dynamics through changes to gamete development and quality. Several metrics are associated with gametogenesis, including gamete size, stage, biocomposition, integrity, viability, developmental rate, maturation, and spawning capability. In cnidarians, OA negatively affected octocoral egg size (Rossin et al., 2019), while hexacoral studies showed no effect of OA on gamete size (Fine and Tchernov, 2007; Gizzi et al., 2017; Caroselli et al., 2019; Marchini et al., 2021) or maturation stage (Fine and Tchernov, 2007; Gizzi et al., 2017; Caroselli et al., 2019). However, Marchini et al. (2021) showed a delay in spermary development due to OA. In crustaceans, OA resulted in delayed gametogenesis and smaller spermatophores (Fitzer et al., 2012a; Cripps et al., 2014; Meseck et al., 2016; Conradi et al., 2019). OA did not affect copepod egg viability, oogenesis (Vehmaa et al., 2013; Thor et al., 2018), or barnacle and copepod gametogenesis (Smith et al., 2017; Pansch et al., 2018).
OA had negative effects on gonad growth, maturation, egg size, and gamete development in some echinoderm species (Siikavuopio et al., 2007; Kurihara, 2008; Stumpp et al., 2012; Kurihara et al., 2013; Suckling et al., 2014; Suckling et al., 2015; Verkaik et al., 2016; Dworjanyn and Byrne, 2018; Hu et al., 2018; Hue et al., 2020; Marčeta et al., 2020; Anand et al., 2021), while other studies reported no change in gamete development and quality (Wood et al., 2008; Uthicke et al., 2013; Hazan et al., 2014; Uthicke et al., 2014; Dell’Acqua et al., 2019; Karelitz et al., 2019; Wong et al., 2019; Hue et al., 2020; Uthicke et al., 2020). One study reported a positive effect on gonad protein storage after low pH exposure (Challener et al., 2014). Suckling et al. (2015) suggests egg size is plastic in response to OA, and longer exposure to low pH can lead to larger eggs due to increased maternal provisioning.
Most studies in molluscs indicate that low pH exposure affects the rate of gametogenesis, typically by decreasing gamete development or spawning rates (Xu et al., 2016; Boulais et al., 2017; Parker et al., 2018; Zhao et al., 2019; Spencer et al., 2020; Wang et al., 2021), or less commonly by accelerating gamete development (Dell’Acqua et al., 2019; Clements et al., 2020). However, no impact of OA on molluscan gametogenesis has also been reported (Le Moullac et al., 2016; Venkataraman et al., 2019). Molluscan egg quality may be resilient to OA, as most studies report no impact to egg size or lipid content (Parker et al., 2017; Parker et al., 2018; Scanes et al., 2018; Parker et al., 2021; Reed et al., 2021; Gibbs et al., 2021a) (but see Spady et al., 2020, the sole non-bivalve study). Two studies do report increased egg size or lipid content in response to OA (Zhao et al., 2019; Gibbs et al., 2021b), which can be indicative of higher energy content and egg quality (Moran and McAlister, 2009). While other egg quality parameters are possibly vulnerable (e.g., egg rupture rate, Omoregie et al., 2019), most studies indicate that molluscan species prioritize per-egg maternal investment when exposed to low pH, in some cases over gametogenic rate. In one of the few studies utilizing naturally low pH environments, Bathymodiolus septemdierum mussels were collected from hydrothermal vents, which reach conditions as low as pH 5.2. These mussels can maintain similar egg sizes and gametogenic cycles compared to those living in higher pH sites, possibly at the expense of calcification (Rossi and Tunnicliffe, 2017). This study provides evidence that OA-adapted populations are capable of gametogenesis despite extreme pH conditions. Given the variable responses in gametogenesis and gamete quality across phyla, species, and even populations, broader characterizations are needed to better understand how OA will impact physiological performance and allocation to energy expensive processes such as gamete development.
Fecundity and reproductive output
The fecundity and reproductive output of individuals and populations can vary along environmental gradients. In this section we highlight studies that assess fecundity and reproductive output in varying OA conditions. We considered hatch rate as a proxy for fecundity and reproductive success in brooding organisms. Cnidarian studies report no effect of OA on fecundity in hexacorals. The number of eggs, sperm, or gamete bundles were not affected by OA in four hexacoral species (Jokiel et al., 2008; Gizzi et al., 2017; Caroselli et al., 2019; Marchini et al., 2021). Most of these studies report reduced adult growth under OA, suggesting resources may have been allocated towards reproduction over growth. In the sole octocoral study, exposure to OA reduced the number of eggs per polyp (Rossin et al., 2019). In echinoderms, OA negatively affected egg production (Dupont et al., 2013). Three molluscan studies report negative effects of OA across reproductive modes, with decreased pH reducing fecundity and spawn rate in a broadcast spawner (Parker et al., 2018), and reducing clutch size and egg masses in two oviparous species (Dionísio et al., 2017; Spady et al., 2020). Two studies reveal the complexity of responses to OA in molluscs, including reduced fecundity but more frequent spawn events (Manno et al., 2016), and unaffected spawn frequency and number of egg capsules (Kita et al., 2013).
Studies that measure hatch rate in brooders were limited to crustaceans and molluscs, as hatch rate was not reported for brooding species in any other taxa. Several brooding crustacean species held at low pH were found to have differences in fecundity, hatch success, or number of offspring (Kurihara et al., 2004; Mayor et al., 2007; Kurihara et al., 2008; Findlay et al., 2009; Zhang et al., 2011; Vehmaa et al., 2012; Weydmann et al., 2012; Kita et al., 2013; Long et al., 2013; McConville et al., 2013; Zervoudaki et al., 2013; Cripps et al., 2014; Cao et al., 2015; Swiney et al., 2015; Thor and Dupont, 2015; Choi et al., 2016; Miller et al., 2016; Vehmaa et al., 2016; Borges et al., 2018a; Cardoso et al., 2018; Gravinese, 2018; Pansch et al., 2018; Thor et al., 2018; Lee et al., 2020). One study in copepods found that OA reduced the number of brooding females (Lee et al., 2019). Several crustacean studies, however, showed that fecundity or hatch rate were unaffected by OA (Kurihara et al., 2004; Mayor et al., 2007; Kurihara and Ishimatsu, 2008; Egilsdottir et al., 2009; Zhang et al., 2011; Weydmann et al., 2012; McConville et al., 2013; Vehmaa et al., 2013; Cripps et al., 2014; Cao et al., 2015; Isari et al., 2015; Almén et al., 2016; Rains et al., 2016; Zervoudaki et al., 2017; Thor et al., 2018; Langer et al., 2019). One study found that OA can increase fecundity, and another found that low pH increased reproductive output, but at the cost of body size and shell integrity (Fitzer et al., 2012b; Engström-Öst et al., 2014). In molluscs, OA exposure during breeding reduced bivalve brood size (Wippel, 2017; Maboloc and Chan, 2021), while a previous OA exposure resulted in increased brood size but no effect to overall larval production (Spencer et al., 2020). Instances where reproductive output increases may be a short-term stress response or may indicate adaptive potential to pH change (Engström-Öst et al., 2014). Overall, research to date suggests that OA negatively affects fecundity and reproductive output in molluscs and echinoderms, while some cnidarians appear to be less sensitive, and responses in crustaceans are variable.
Timing of reproduction and synchronization
Synchronicity and timing of reproductive maturation, spawning, hatching, and planulation are important to ensure reproductive success. In a brooding coral, OA conditions delayed planulation timing (Putnam et al., 2020). OA may affect the timing at which brooding crustaceans reach milestones associated with hatching. When female copepods and crabs were acclimated to OA conditions, copepod hatching occurred earlier and crabs hatched for a longer duration (Long et al., 2013; Langer et al., 2019). However, there was no effect of OA on egg onset time in the barnacles (McDonald et al., 2009). On the other hand, OA did not affect the number of days that copepods needed to form mating pairs, successfully copulate, and spawn (Kita et al., 2013). A volitional spawning experiment in sea cucumbers reported no effect of OA on spawn timing (Verkaik et al., 2016). In molluscs, studies that induced spawning found reductions in spawning success in acidified conditions (Xu et al., 2016; Parker et al., 2018). Two studies examined impacts of OA on volitional spawning in a brooding molluscan species: one reported no effects to larval release timing (Spencer et al., 2020), and another reported delayed larval release onset in one of three trials (Wippel, 2017). More studies that incorporate natural spawning and planulation conditions are needed. Studies that monitor the number and timing of naturally breeding males and females would improve our understanding of ecologically relevant impacts of acidification on spawn timing and synchronicity.
Mating behavior
Marine species are diverse in their mating behaviors. However, there is a very limited number of OA studies to date examining mating behavior, limiting the number of observable behavioral metrics. We summarize two studies that measure mate selection, courtship display, and mate guarding. Male mate tracking and guarding behavior in amphipods were disrupted under OA conditions, which may have negative consequences for fertilization (Borges et al., 2018b). The ability for amphipods to detect and orient toward female pheromone cues through chemotaxis was greatly reduced under OA, suggesting potential disruptions in chemosensory cues related to food acquisition, defense, and predator avoidance behaviors under low pH. Squid mating pair behavior was unaffected by OA, even though females laid denser egg clutches after the pair were exposed to low pH (Spady et al., 2020). More studies are needed to explore the impacts of OA on invertebrate mating behavior, particularly in cephalopod, gastropod, and crustacean species with sophisticated mating behaviors (e.g., changing body patterns and textures, parallel swimming, male-male fighting, and mate guarding). It is critical that we expand our understanding of mating behavior in invertebrates exposed to OA because disruptions may have rippling effects on population fitness and ultimately, the future of the species.
Fertilization
Many marine invertebrates broadcast spawn and undergo external fertilization, which exposes gametes to the surrounding environment prior to and during fertilization. Acidification may affect fertilization success by altering chemosensory and biochemical egg-sperm interactions, therefore directly influencing gamete activation, sperm activity, egg biochemistry and polyspermy defense (Mortensen and Mortensen, 1921; Nakajima et al., 2005; Miyazaki, 2006; Lymbery et al., 2019). The impact of OA on fertilization and gametes has been reviewed previously (Byrne, 2011; Byrne, 2012; Byrne and Przeslawski, 2013; Foo and Byrne, 2017). Here we provide an updated review of 92 studies that have assayed fertilization, sperm quality, and/or egg biochemical processes under OA conditions, the majority of which were published since prior reviews. Additionally, we conducted a meta-analysis to evaluate general trends across and within echinoderms, molluscs, and cnidarians. Crustaceans were not included in the meta-analysis as no studies have directly measured fertilization rate in varying pH treatment, likely because they undergo internal fertilization.
Fertilization rate
Reviews by Byrne (2011; 2012) found that fertilization rate is robust in pH above ~7.6. However, discrepancies were noted among studies of the same species and conspecifics from different habitats. The authors encouraged researchers to examine other taxa with standardized experimental designs. Since then, an additional 51 studies have assessed fertilization rate in varying pH conditions.
The majority of studies, which predominantly evaluate echinoderms (largely urchins) and bivalve molluscs, conclude that acidification negatively affects fertilization rate when gametes are directly exposed (Smith and Clowes, 1924; Kurihara and Shirayama, 2004; Havenhand et al., 2008; Parker et al., 2009; Ericson, 2010; Parker et al., 2010; Kimura et al., 2011; Moulin et al., 2011; Schlegel et al., 2012; Van Colen et al., 2012; Barros et al., 2013; Gonzales-Bernat et al., 2013; Uthicke et al., 2013; Foo et al., 2014; Frieder, 2014; Scanes et al., 2014; Suckling et al., 2014; Sung et al., 2014; Riba et al., 2016; Boch et al., 2017; Shi et al., 2017a; Shi et al., 2017b; Szalaj et al., 2017; Zhan et al., 2017; Basallote et al., 2018; García et al., 2018; Świeżak et al., 2018; Zhan et al., 2018; Smith et al., 2019; Sui et al., 2019; Wang et al., 2020), or parents are exposed prior to fertilization (Graham et al., 2015). The pH level at which fertilization rate becomes compromised varies considerably across taxa, and also depends on factors such as sperm and egg concentration (Ericson, 2010; Albright, 2011a; Ericson et al., 2012; Albright and Mason, 2013; Gonzales-Bernat et al., 2013; Ho et al., 2013; Frieder, 2014), gamete incubation time (Bechmann et al., 2011; Gianguzza et al., 2014; García et al., 2018; Kong et al., 2019), inter-individual and mating pair variability (Schlegel et al., 2012; Foo et al., 2014; White et al., 2014; Smith et al., 2019), collection location and time of year (Martin et al., 2011; Cohen-Rengifo et al., 2013; Pecorino et al., 2014; Riba et al., 2016; Basallote et al., 2018; García et al., 2018; da Silva Souza et al., 2019; Pereira et al., 2020; Caetano et al., 2021), and species hybridization (Striewski, 2012). Parental acclimatization to low or highly variable conditions (including pH) can either increase (Moulin et al., 2011; Suckling et al., 2014; Kapsenberg et al., 2017) or decrease fertilization rates in low pH (Graham et al., 2015).
Negative effects of OA were not universally reported (Byrne et al., 2009; Byrne et al., 2010a; Byrne et al., 2010b; Schlegel et al., 2012; Byrne et al., 2013; Chua et al., 2013; Kamya et al., 2014; Onitsuka et al., 2014; Stavroff, 2014; Bylenga et al., 2015; Iguchi et al., 2015; Schutter et al., 2015; Foo et al., 2016; Zhan et al., 2016; Boulais et al., 2017; García et al., 2018; Armstrong et al., 2019; Lenz et al., 2019; Smith et al., 2019; Guo et al., 2020; Hue et al., 2020; Pitts et al., 2020), which indicates that fertilization in some species may be more resilient than others to the effects of acidification. Several studies report that Mytilus spp. mussels, for example, are capable of maintaining high fertilization rates across a broad pH range (Bechmann et al., 2011; Eads et al., 2016; Riba et al., 2016; Gallo et al., 2020). Fertilization in some molluscan and echinoderm species can persist at severely low pH (e.g., ~23% of Crassostrea gigas eggs were successfully fertilized at pH 6.0, Riba et al., 2016).
In rare instances, low pH can increase fertilization rate. Higher fertilization rates occur in Mytilus galloprovincialis when sperm are pre-exposed to low pH conditions in the presence of egg-derived chemicals (Lymbery et al., 2019). These results could be explained by a stronger attraction of sperm to egg-derived chemicals under low pH conditions, resulting in increased fertilization rates. As suggested by Lymbery et al. (2019), increased fertilization rates are not necessarily beneficial to population fitness. OA could alter chemical cues that would typically only occur among genetically compatible sperm and eggs, resulting in higher rates of fertilization among incompatible genotypes, and ultimately decreasing the overall population fitness (Lymbery et al., 2019).
Fertilization rate meta-analysis
Studies that reported fertilization rates across multiple pH levels were leveraged to perform a meta-analysis to quantify broad-scale impacts of pH on fertilization rate in cnidarians, echinoderms, and molluscs. Change in pH (ΔpH = pHexperimental - pHcontrol) across all taxa significantly predicts fertilization success as a sole predictor (X2 = 22.0, p = 2.7e-6). Using ANCOVA we modeled fertilization rate by ΔpH and other candidate covariates. The most parsimonious model was selected using AIC criterion, and retained only the interaction between ΔpH and phylum (phylum:ΔpH, X2 = 34.3, p = 1.7e-7), and phylum as a main effect (X2 = 3.4, p = 0.18), indicating that the response of fertilization rate is phylum-specific. The probability of successful fertilization is estimated by:
where the ΔpH coefficient estimates for cnidarians, echinoderms, and molluscs are 2.43 (z-score = 1.24, p = 0.22), 1.92 (z-score = 5.75, p = 9.0e-9), and 0.32 (z-score=0.71, p = 0.48), respectively (Figure 4). The positive ΔpH coefficients indicate that fertilization rate decreases with pH in all phyla; however, ΔpH only significantly predicts fertilization for echinoderms. While pH is not a significant factor in the cnidarian model, likely due to limited data (6 studies), the model does suggest high pH sensitivity (Figure 4) and underlines the need for more cnidarian fertilization studies. The large number of studies in the echinoderm (35) and mollusc (23) models enables more confident predictions in those two taxa. Echinoderm fertilization appears to be more sensitive than molluscs to pH changes, which could reflect habitat differences in most species studied to date (largely subtidal echinoderms and intertidal molluscs).
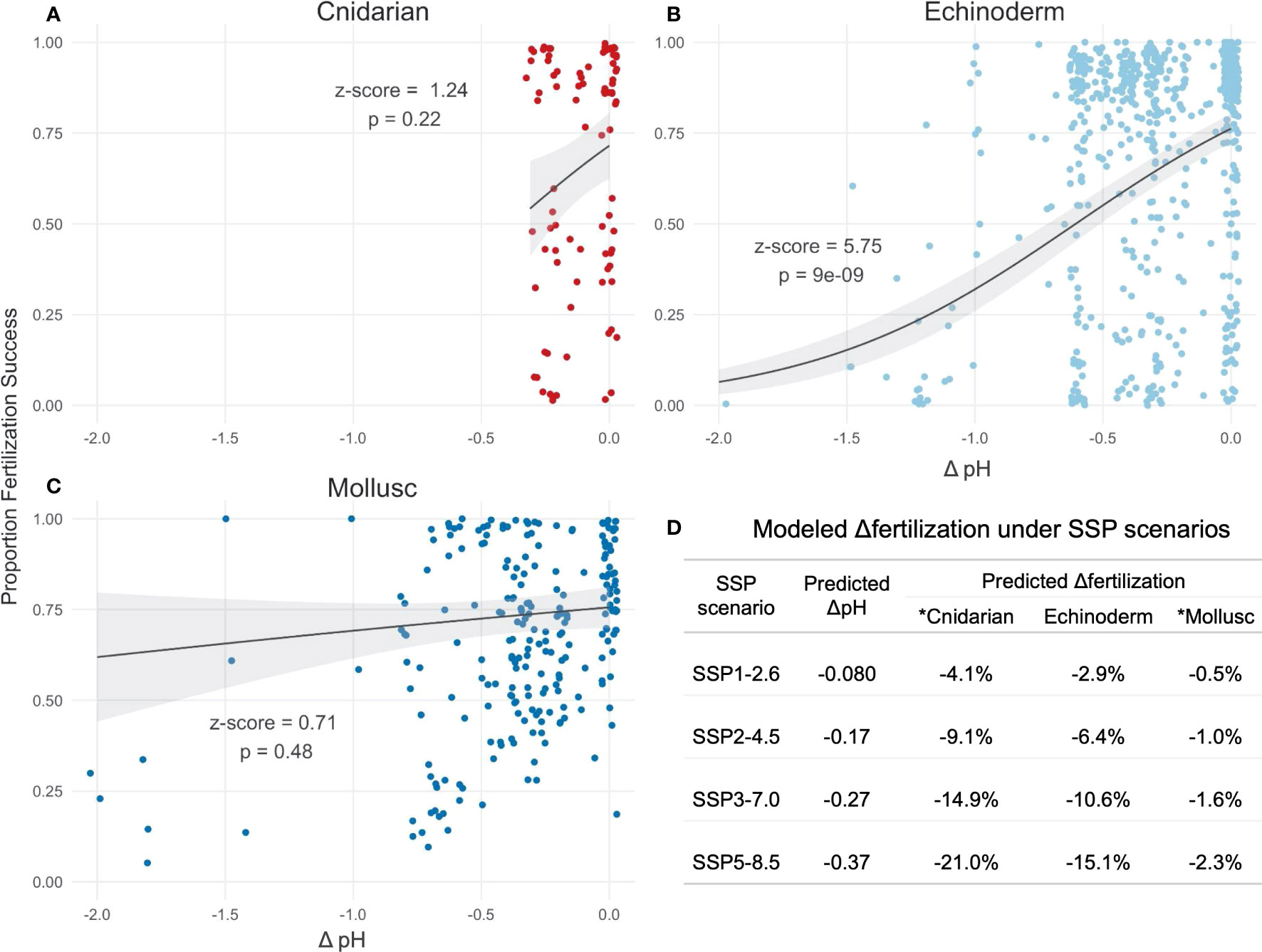
Figure 4 Fertilization success by ΔpH (the difference between experimental pH and control) for (A) cnidarians (6 studies), (B) echinoderms (35 studies), and (C) molluscs (23 studies). Control pH (ΔpH = 0) is defined separately by each experimental study, and typically represents present-day ambient pH at experimental location. The meta-analysis was performed using a beta regression model, and indicates that the effect of pH on fertilization rate is phylum-dependent (phylum:ΔpH interaction, X2 = 34.3, p = 1.7e-7). In all figures, each point reflects the average percent fertilization reported by one study at each ΔpH and secondary variable tested, curves indicate the fitted models, ribbons indicate 95% confidence intervals, and z-scores and p-values indicate the significance of the ΔpH coefficient estimates for each phylum. (D) Models were used to predict the change in fertilization rate under four Shared Socioeconomic Pathways (SSPs) for each phylum, which predict the pH change for the end of the 21st century (IPCC, 2022) relative to 1995–2014. *The pH coefficient was not a significant factor in the mollusc or cnidarian models, therefore these predictions should be considered descriptive, particularly for cnidarians for which there is limited data. No fertilization studies were found for crustaceans or ascidians.
Using the phylum-specific models, we estimate that under the Shared Socioeconomic Pathways (SSPs) SSP1-2.6, SSP2-4.5, SSP3-7.0 and SSP5-8.5 predicted for the end of the 21st century (Cooley et al., 2022; IPCC, 2022), fertilization rate will decrease by 2.9%-15.1% for echinoderms, and by a non-significant amount for molluscs (0.5%-2.3%), relative to 1995–2014 (Figure 4). Our low-confidence predictions for cnidarians estimate a decrease of 4.1%-21.0%.
Since many studies included pH levels beyond those predicted under SSP5-8.5, we reconstructed our meta-analysis using data from experimental pH ≥ 7.6. This reduced the dataset by ~20% for echinoderms and molluscs but did not affect the cnidarian data due to the narrower range of experimental pH levels tested for cnidarians (Figure 4). In the revised analysis, ΔpH remains significant but is less predictive of fertilization rate (X2 = 4.39, p = 0.036). The best fit model includes ΔpH only and predicts a decrease in fertilization rate across all taxa by 2.0%, 4.3%, 7.1%, and 9.9% under SSP1-2.6, SSP2-4.5, SSP3-7.0 and SSP5-8.5, respectively. Predictions from this more conservative approach support previous conclusions that marine invertebrate fertilization is more robust — albeit still negatively impacted — at pH 7.6 and above (Byrne, 2011; Byrne, 2012), which may better reflect relevant open ocean pH projections. However, many species covered in this review are coastal and periodically experience pH levels below 7.6; therefore, the full model should not be disregarded.
As noted by Byrne (2011; 2012), there are many factors found to influence fertilization success that vary across studies. Given the diversity of species and secondary variables tested (e.g. warming), limiting the meta-analysis to only studies that used the same procedures was not possible. Instead, we took a broader approach, including all studies with a variety of secondary variables (Supplemental Table 3). We then tested for effects of six additional experimental variables (when reported, see Methods and Supplemental Table 3). We found that experimental sperm concentration significantly affects fertilization rates as a sole predictor but does not interact with ΔpH alone or as an additional covariate with phylum. Given that only five of the 34 studies that reported sperm concentration included multiple concentrations in the experimental design (Supplemental Table 3), the model was likely unable to fully characterize the interaction between pH and sperm concentration. In the natural environment, sperm levels can be considerably more dilute than the optimal levels used in most studies. Whenever possible, studies should test effects of pH on fertilization across a range of sperm concentrations.
Other experimental variables that are not commonly reported or considered may interact with pH to affect fertilization rate, making it difficult to fully describe the impacts of OA. For instance, given the rapid pace of ocean change, many experimental animals likely already experienced OA, which could have affected fertilization rates through changes to their gametes (e.g. maternal effects, epigenetic changes). This is particularly pertinent because control pH levels applied by researchers often reflected conditions entering their experimental systems or the historical pH for a given region, which could differ dramatically from conditions at the animal collection site. Future studies should strive to characterize their focal species’ current and future environments to determine pH treatment levels, as they will provide the most ecologically relevant results.
In summary, our meta-analysis indicates that OA will reduce fertilization rates in many marine invertebrates, and effects are taxa specific. Fertilization rates are likely to decrease in echinoderms but remain relatively similar in molluscs. More studies are needed for cnidarians, but preliminary work indicates that their fertilization rates may be the most affected.
Gamete mechanisms that influence fertilization rate
There are five major events that typically occur during fertilization: 1) sperm chemoattraction to the egg, 2) sperm binding to the egg envelope, 3) sperm acrosomal reaction, 4) sperm penetration through the egg envelope, and 5) gamete fusion (Vacquier, 1998). Successful fertilization therefore involves a cascade of biochemical processes in both sperm and eggs, many of which include intracellular pH and calcium ion changes (Morisawa and Yoshida, 2005; Nakajima et al., 2005; Byrne, 2011; Sherwood et al., 2012). OA could alter fertilization rates through changes to chemoattractants, surface receptors, jelly coat, polyspermy defense system, and activation processes in eggs, and/or activation, motility, velocity, mitochondrial activity, chemotaxis, and fusion reactions in sperm.
Several studies examined the impact of acidification on egg fertilization mechanisms. In urchins, low pH increased the percentage of abnormally fertilized eggs (Zhan et al., 2018), polyspermy frequency, and time to completely block polyspermy (Reuter et al., 2011; Sewell et al., 2014). In molluscs, polyspermy rates increased as pH decreased when clam Tegillarca granosa eggs were pre-exposed to various pH treatments (Han et al., 2021). The inability to block polyspermy may be related to low pH reducing the protective egg jelly coat area, which can subsequently impair sperm chemotaxis (Foo, 2015; Foo et al., 2018). These impacts are likely species- and population-specific, with some urchins exhibiting no impact of low pH on egg jelly coat area, and populations exhibiting varied impacts depending on prior pH exposure (Foo et al., 2018). Egg intracellular pH adjusts according to the external chemical environment (Ciapa and Philippe, 2013; Bögner et al., 2014), which may also hamper egg activation by interfering with calcium signaling and actin dynamics (Limatola et al., 2020). Indeed, Shi et al. (2017b) observed that low pH disrupts the intracellular calcium ion (Ca2+) activity in T. granosa eggs, which was associated with reduced fertilization rates.
The influence of low pH on sperm activity varies. Three cnidarian (hexacoral) studies report negative effects of pH on sperm activity (Morita et al., 2010; Albright, 2011a; Nakamura and Morita, 2012). Albright’s initial examination of OA impacts on hexacoral sperm velocity showed no effect, but results from a second experiment suggested a negative effect (Albright, 2011a). OA reduced sperm swimming speed, motility, and linearity in some echinoderms (Havenhand et al., 2008; Morita et al., 2010; Uthicke et al., 2013; Campbell et al., 2016; Caballes et al., 2017), but had no effect or positively impacted sperm swimming speed in others (Caldwell et al., 2011; Sung et al., 2014; Graham et al., 2015). Similarly, pH negatively affected sperm swimming speed, velocity, motility, and mitochondrial activity in some molluscs (Vihtakari et al., 2013; Vihtakari et al., 2016; Shi et al., 2017a; Shi et al., 2017b; Omoregie et al., 2019; Esposito et al., 2020), but had no effect on sperm motility in others (Havenhand and Schlegel, 2009; Vihtakari et al., 2016). In one population of C. gigas oysters, low pH increased sperm motility (Falkenberg et al., 2019).
Contrasting impacts on sperm activity could be due to varied sensitivities among genotypes within species (Schlegel et al., 2015; Campbell et al., 2016; Vihtakari et al., 2016; Falkenberg et al., 2019; Smith et al., 2019). Individual sperm characteristics can also respond differently to low pH (Schlegel et al., 2012; Graham et al., 2015). For instance, OA had a negative effect on the proportion of motile sperm, but not sperm swimming speed, in urchin Heliocidaris erythrogramma sperm (Schlegel et al., 2012). Impacts on sperm activity can also be influenced by experimental conditions, such as the exposure duration (e.g. Eads et al., 2016) or the method used to prepare experimental pH levels (pCO2 vs. HCl, Shi et al., 2017b).
Implications
Our synthesis of the OA reproduction literature to date reveals the complexity of responses across and within species. For all reproductive processes there are studies that report effects of OA, while others found no effect (Figure 3C). Predicting future community-level changes at this stage is therefore not possible. There are, however, some observable trends for those well-studied reproductive processes (gametogenesis, fecundity, fertilization): for all phyla, negative effects were observed in at least one species (and typically many more), and reports of positive effects were rare. Echinoderm fertilization is quite sensitive to pH changes while molluscs are not. Gametogenesis is delayed and fecundity is reduced in many echinoderms and molluscs, but those processes are less sensitive in crustaceans. Cnidarian and ascidian studies are limited. Cnidarian studies suggest that gametogenesis and fecundity are relatively robust to OA, and fertilization may be quite sensitive. These observations provide the basis for hypotheses of the potential challenges faced by those in the aquaculture, fisheries, and conservation sectors. Not all reproductive processes will be affected in all species, but for those that are, here are some considerations.
Aquaculture
Impacts of OA on reproduction have the potential to negatively affect our marine food systems. Aquaculture programs may need to adjust their hatchery culturing techniques to accommodate effects of OA. For example, gametogenesis in many species is delayed in OA, so conditioning them in buffered seawater could ensure or expedite gamete development. This may also improve synchronization of mature males and females in some species, as unequal impacts of acidification on oogenesis and spermatogenesis have been observed in oysters (e.g. Boulais et al., 2017). Seawater may also need to be buffered during fertilization to maintain high fertilization rates, particularly for some echinoderms and possibly cnidarian species. Alternatively, fertilization conditions could be re-optimized at a lower pH, such as by adjusting sperm concentrations. Should these measures be needed, larger facilities with more complex seawater treatment systems, more resources, and increased handling will be required, thus increasing the overall cost of production.
Wild fisheries
Wild fisheries do not have the capability of modulating the environment during reproduction, and may therefore be more susceptible to the effects of OA. Acidification may impact invertebrate fishery stocks by slowing gametogenesis, and reducing fecundity and fertilization, which could ultimately reduce reproductive output. While not covered in this review, there are many other possible indirect effects of OA on reproduction that could impact fisheries. Reproduction-related mortality may increase, as acidification can alter immune and stress-response functions, increasing vulnerability to pathogens and secondary stressors during and after reproduction (Mackenzie et al., 2014; Liu et al., 2016; Wang et al., 2016). In many invertebrates, reproductive capacity is closely tied to food availability before or during gametogenesis (Bernard et al., 2011). Changes to prey species dynamics due to acidification, such as altered phytoplankton communities’ composition, abundance, and bloom timing (Cheung et al., 2011; Gao et al., 2012), may result in asynchronous invertebrate reproductive processes such that larval production and quality decreases.
Conservation and restoration
Conservation and restoration programs may need to update their strategic plans to account for impacts of acidification on reproduction. For instance, natural recovery of wild populations following removal of stressors (e.g., creation of a marine protected area following overharvest) could be impeded by decreased larval supply due to reduced reproductive success. Populations may take longer to recover, requiring programs to allocate more time and resources for longer-term interventions. Conservation and restoration approaches may need to change altogether, such as moving from passive strategies (e.g., removing disturbances and allowing succession to occur naturally), to active strategies (e.g., transplanting or augmenting wild populations) (Figure 2). For instance, programs may need to incorporate restoration aquaculture and gamete cryopreservation to rebuild threatened populations. While preliminary studies in coral report a variety of response, some aspects of coral reproduction may be uniquely vulnerable to acidification due to their sexual reproduction strategies (Richmond and Hunter, 1990; Burke et al., 2011). In many coral species, gametes take several months to develop, and spawning occurs only a few nights a year over a few hours (Babcock et al., 1986). If OA interferes with these highly coordinated spawning events (Olischläger and Wild, 2020), sexual reproduction could be severely inhibited in the wild, but this has yet to be tested.
Genetic considerations
Genetic diversity, an important factor for species resilience to environmental stressors (Bernhardt and Leslie, 2013; Timpane-Padgham et al., 2017), could shift as a consequence of acidification-induced changes to reproduction and effective population size. Many invertebrate species are r-strategists with highly fecund females (Ramirez Llodra, 2002). Should acidification reduce the number of individuals that reproduce each season due, for instance, to delayed gametogenesis, populations could still be sustained by a few individuals that “win the reproduction lottery” (Hedgecock and Pudovkin, 2011; Sanford and Kelly, 2011). It may therefore be important for programs to actively facilitate genetic diversity and/or resistance to acidification. For commercial and restoration aquaculture facilities, broodstock may need to be collected later or conditioned longer in the hatchery to increase the number of breeding individuals. Conservation programs may need to design marine protected areas that encompass a variety of current and projected pH conditions, not just buffered areas, to enable both diversity and selection for low pH-resilient individuals. To build resilient populations and lines, restoration and breeding programs could leverage standing genetic variability to target genotypes that are reproductively viable in acidified conditions (Parker et al., 2011; Rossi and Tunnicliffe, 2017). This may include careful consideration of broodstock collection sites, such as areas with naturally low or variable pH conditions. Should the number of individuals contributing gametes become dangerously low, programs may need to begin cryopreserving gametes to preserve allelic diversity in a “seed bank” for future use (Figure 2).
Within- and inter-generational acclimatization have been observed in some species in response to acidification (Ross et al., 2016). Adult exposure to acidification may result in beneficial carryover effects for offspring and future generations (Parker et al., 2012; Wong et al., 2019; Zhao et al., 2019; Spencer et al., 2020), which could mitigate some of the negative effects to reproduction reviewed here. While additional research is needed and there may be limitations to inter-generational acclimatization (Byrne et al., 2019), these beneficial carryover effects may be leveraged to improve offspring fitness for production and breeding purposes (Parker et al., 2011; Durland et al., 2019).
Gaps in understanding for OA impacts on reproduction mechanisms
In addition to the critical need to know more about the basic biology and natural history of reproduction in marine invertebrate communities (Box 2), the most pressing need is for increased mechanistic knowledge of reproductive processes to gauge the impact of OA on marine invertebrates (Figure 5). In this section we outline mechanisms that should be explored with future research.
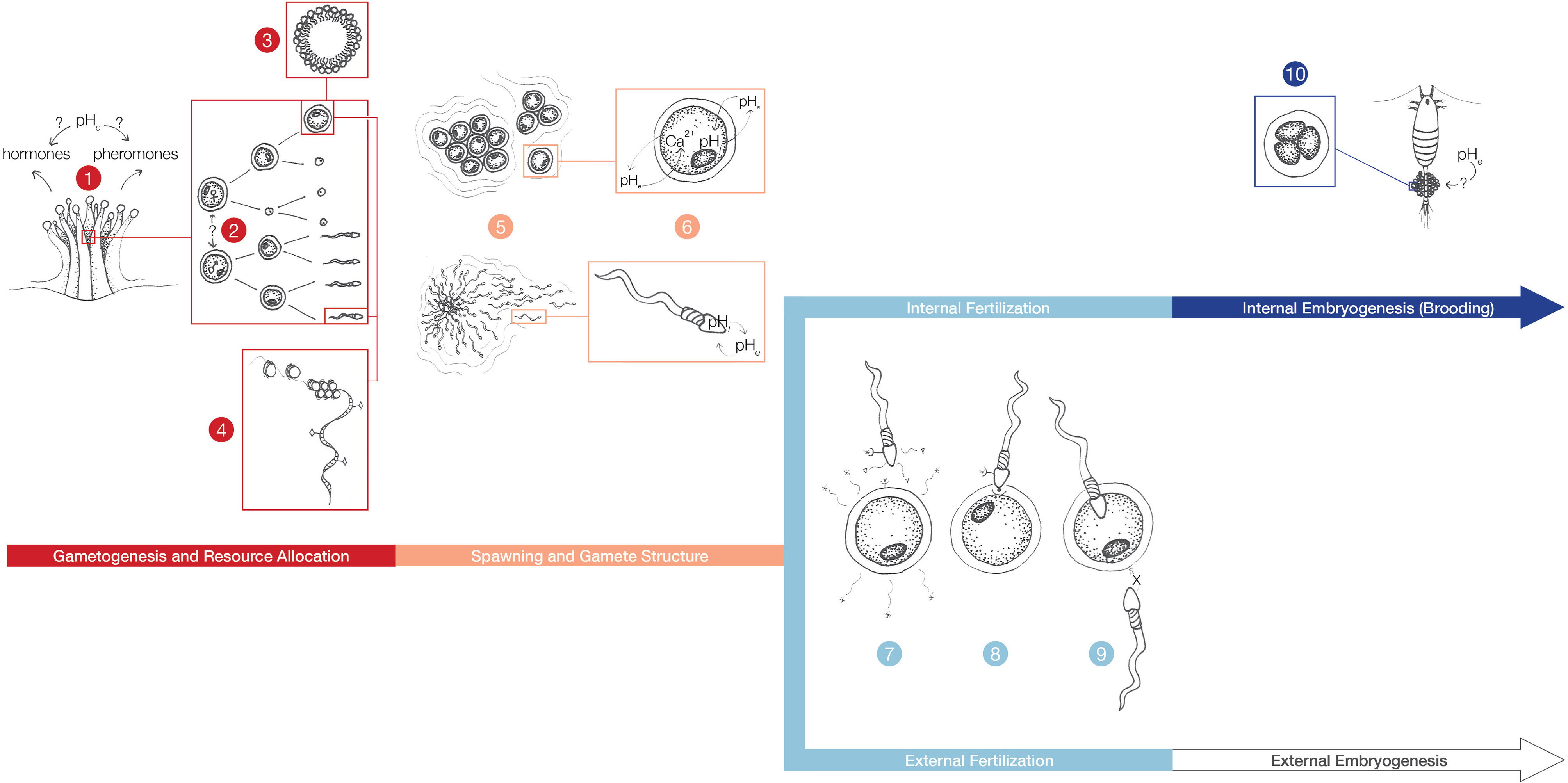
Figure 5 Sexual reproduction mechanisms that may be compromised by OA. Changes in the chemical environment can impact the release of hormones and pheromones that trigger gametogenesis, or gamete release in broadcast spawning species (1). Sex determination and differentiation processes (2), gametogenesis timing, and energy allocation (specifically maternal provisioning) (3) can be impacted by low pH. Gametes undergo substantial chromatin reorganization and DNA methylation (4) that can impact how gametes and subsequent offspring respond to acidified conditions. Spawn timing may be affected by OA (5). Gamete bundles undergo breakage, further exposing gamete intracellular conditions to changes in extracellular pH (pHe) (6). To begin the fertilization process internally or externally, chemoattractants are released from eggs and sperm (7). A single sperm binds to the egg with the aid of gamete recognition proteins (8). Successful fertilization triggers calcium release or an egg jelly coat to prevent polyspermy (9). Internal embryogenesis can be affected by OA (10). For organisms with internal fertilization, brooding may shield embryo development from changes in pHe or expose embryos to more extreme conditions.
Box 2. Experimental considerations for future OA and reproduction studies
See previous reviews for additional challenges in the design and execution of ocean acidification experiments (Albright et al., 2011b; Grazer and Martin, 2012; Prezeslawski et al., 2017).
Improve field research capability
• Spawning timing, duration, and intensity in natural settings are not easily predictable, and we lack baseline reproduction knowledge for most marine species. Additionally, physiological and reproductive responses of laboratory-raised organisms may be compromised by stress associated with captivity.
• Research initiatives should collect baseline knowledge. Technologies should be developed and integrated with biophysical models to track gametes and larvae in the natural environment.
Measure multiple reproductive traits simultaneously
• Reproduction is a complex process. It is important to examine multiple reproductive traits simultaneously to get a better understanding of overall reproductive performance.
• Reporting measures of reproductive success that integrate multiple traits will improve the predictability of demographic models (ex. fecundity and survival of mother, survival of offspring).
Incorporate diversity in test subjects and experimental design
• Studies should maximize genetic variation, as organism origin (i.e., field site, hatchery) and genotype can affect sperm and egg compatibility and confound findings.
• Future work should incorporate taxonomic diversity and multiple stressors to expand the breadth of species and conditions studied.
Account for length of gametogenic cycles
• Gametogenic cycles vary between species. Many studies only expose organisms to OA when gametes are almost fully developed.
• Accounting for gametogenic cycle length improves understanding of OA effects on gamete development and parental effects.
Test multiple sperm concentrations
• The probability of detecting a treatment effect may be dependent upon sperm concentrations. “Optimal” sperm concentration may be higher than ecologically relevant concentrations. Low sperm concentrations can exacerbate OA effects.
• Experiments should use multiple sperm concentrations and gamete incubation times, as these factors may alter the probability of fertilization and/or polyspermy.
Understand limitations of intergenerational studies
• Artificially induced fertilization methods (i.e., strip spawning, chemical/hormonal injection, etc.) used in intergenerational studies may cause organisms to release gametes before complete gametogenesis.
• Many intergenerational OA studies inherently include adult exposure during reproduction, so those studies should formally measure and report reproduction metrics.
• Future research should differentiate between within-generation carryover effects, cross-generational impacts (F0-F1), and multigenerational plasticity (F1-F2+), and determine how reproductive conditioning may impact each facet of plasticity.
Fertilization mechanisms
Fertilization mechanisms are the most studied reproductive processes in marine invertebrates. Even so, there are still considerable knowledge gaps, particularly in how changes to gamete quality and sperm-egg interactions will affect fertilization (Figure 5). As a model organism for developmental biology, urchin fertilization has been well-studied, with cellular processes potentially impacted by OA reviewed in Bögner (2016). Compared to the numerous echinoderm and molluscan bivalve studies, only a handful of studies have explored OA and fertilization in cnidarians (none in non-hexacoral cnidarians) and crustaceans, and there are none in cephalopods. Considerable research on fertilization mechanisms has come from ascidians (Sawada and Saito, 2022); however, only two studies have examined ascidian fertilization under OA conditions. More studies are clearly needed for these ecologically and economically important taxa.
Gamete quality depends on maintaining an optimal intracellular pH (pHi). Reductions in extracellular pH are thought to affect pHi, suppress motility, and impact fertilization success (Christen et al., 1983; Alavi and Cosson, 2005; Morita et al., 2006; Boulais et al., 2018). In certain species, an increase in pH may be required for sperm activation (Nakajima et al., 2005). Explicit measurements of sperm pHi, including those taken in the field, can help uncover the mechanism behind pHi activation of sperm motility and its vulnerability to environmental pH conditions.
The impact of acidification on spawned eggs likely depends on their composition, structure, and intracellular conditions. Egg size is used as a proxy for well-provisioned eggs, but few studies empirically test this assumption with lipid analyses. Intracellular egg calcium content can also impact fertilization. Calcium triggers the second meiotic division in eggs, readying it to unite with sperm to complete the fertilization process (Miyazaki, 2006), and is part of the polyspermy defense (Epel, 1978). Egg calcium content can also be influenced by environmental pH (Ciapa and Philippe, 2013). More research should be conducted on energy allocation, maternal provisioning, and egg lipid and calcium content in low pH conditions.
Other structural components that could be influenced by acidification include the egg jelly coat and gamete recognition proteins. Present in echinoderms and molluscs, the jelly coat protects the egg from intense wave action and prevents polyspermy and increases the likelihood of fertilization by increasing the size of the egg (Farley and Levitan, 2001; Podolsky, 2004). Additionally, the jelly coat is known to contain chemoattractants that activate and guide sperm or prevent polyspermy and harbor protective chaperone and gamete recognition proteins (Evans and Sherman, 2013). The dissolution of the jelly coat by low pH conditions observed in echinoderms can reduce percent fertilization, with jelly coats in some populations and species resilient to low pH. Cnidarian eggs do not have jelly coats, and similar structures have not been described in crustaceans. A general survey of gamete accessory structures in marine invertebrates will be beneficial to determine how low pH will impact eggs.
Mechanisms beyond fertilization
Mechanisms controlling pre-fertilization reproductive processes are understudied generally and in the context of OA. In many marine invertebrates, gametogenesis, spawn timing, and synchronicity is influenced by exogenous signals such as temperature, photoperiod, food, and pheromones from conspecific gametes (Galtsoff, 1938; Lawrence and Soame, 2004), and by endogenous signals such as hormones or neuropeptides (Tanabe et al., 2010; Jouaux et al., 2012). Applying knowledge of how these environmental cues are registered and acted upon by organisms may elucidate how low pH will affect these pathways.
Future studies should examine whether OA impacts biochemical cues involved in gametogenesis, coordinated spawning events, and mating behavior. Since many invertebrates are osmoconformers with open circulatory systems, reproductive hormones that circulate through the hemolymph are likely to be exposed to environmental pH changes (Tarrant, 2007; Treen et al., 2012). Structural changes have been observed in signaling peptides outside of the reproductive context (Tarrant, 2007; Roggatz et al., 2016). Exposure to acidification, for instance, resulted in protonation of three peptide signaling molecules, which was associated with behavioral impairment in shore crabs (Roggatz et al., 2016). Whether acidification alters marine invertebrate reproductive hormones is not yet known. This framework could be extended to pheromones involved in mating. Pheromones come in direct contact with the environment, and may be important for spawning synchrony, long-distance mate searches, mate guarding, and copulation dances. Out of all marine invertebrates, reproductive pheromones are best understood in decapod crustaceans. Pheromones released by female crabs instigate courtship displays and guarding behaviors in males (Kamio et al., 2022). Male crabs can distinguish female molting stages and copulation readiness based on pheromone detection (Kamio et al., 2022). Alteration of pheromone chemical composition, molecule shape, and residence time in low pH conditions could impact necessary mating behaviors and successful reproduction (Roggatz et al., 2016; Kamio et al., 2022). Additionally, the ability of invertebrates to produce and detect these compounds may be impacted by low pH (Roggatz et al., 2016; Kamio et al., 2022). A recent hypothesis developed by Olischläger and Wild (2020) suggests that gamete release timing during periods of low water motion is possibly regulated by the carbon concentrating mechanism in corals and/or their symbionts, which may make the synchronized spawning of organisms with photo-symbionts vulnerable to OA. Future work should examine hormones, pheromones, and other factors governing reproductive cues and behavior to identify molecular signals vulnerable to OA.
For a more comprehensive understanding of changes to reproductive resource allocation in acidified conditions, future studies should examine multiple physiological metrics. OA-induced changes in sex-specific growth, tissue regeneration, and energy allocation could impact reproductive resource allocation. While there are no studies correlating internal pH at the site of gametogenesis and environmental pH, organisms that regulate their internal environment may have increased metabolic demand in low pH conditions, and less energy available for reproductive processes (Sokolova et al., 2012). In corals, tissue damage caused a reduction in coral fecundity (Rinkevich and Loya, 1989; Van Veghel and Bak, 1994; Rinkevich, 1996) and tissue regenerated at a lower rate under OA in various hexacorals (Horwitz and Fine, 2014). When exposed to acidified conditions, the intracellular fluid of the crab Chionoecetes bairdi did not change in low pH. However, there were significantly more dead hemolymph cells and less granular cells within acidified treatments. This suggests that metabolic demands related to increased cell apoptosis, immune response, and phagocytosis may be diverting energy away from reproduction (Meseck et al., 2016). If organisms can buffer extracellular pH to promote somatic growth or maintenance, the diversion of energetic resources to this task may impact reproduction.
There is a lack of research on how OA affects on brooding behavior in cnidarians, echinoderms, and molluscs. Brooding echinoderms may be disproportionately impacted by OA in the Southern Ocean, based on predicted changes to aragonite and calcite saturations at depths where these species are found (Sewell and Hofmann, 2011). Recent studies show that internal brooding itself may impart tolerance in these taxa. For example, pH conditions in the brood chambers of Ostrea spp. oysters are lower than the surrounding water (Gray et al., 2019; Gray et al., 2022). Naturally lower pH in brood chambers could make fertilization more resilient to OA (Lucey et al., 2015; Gray et al., 2019; Gray et al., 2022), thereby providing a potential evolutionary advantage. Potential advantages from brood chambers may not apply to external brooding species like the octocoral Rhytisma fulvum (Liberman et al., 2021). Further research should compare low pH impacts on external and internal brooding to determine if both reproductive strategies provide similar evolutionary advantages.
Genomic regulation of reproduction in marine invertebrates is a crucial, yet understudied, area. Determining how OA affects baseline genetic processes related to reproduction is imperative for understanding if processes can be phenotypically plastic or evolve in response to stressors. Genes specific to sex, maturation stage, early gametogenesis, sex determination, and differentiation have been identified for the oyster C. gigas (Dheilly et al., 2012; Cavelier et al., 2017; Yue et al., 2018). In other economically-important and edible species like the urchin P. lividus and scallop Pecten maximus, transcriptome information has been used to identify markers for sex determination (Machado et al., 2022) and gamete quality (Pauletto et al., 2017), respectively. Expressed transcriptomes of reproductively active individuals at various time points may elucidate genetic regulation of reproduction in low pH conditions. In fish, miRNA molecules regulate oogenesis genes; similar analyses should be conducted in invertebrate species to understand other regulators of gametogenesis (Juanchich et al., 2013). If organisms experience low pH during reproductive conditioning, environmental cues may be integrated in the germline through DNA methylation and other epigenetic modifications (Bell and Hellmann, 2019). Sex-associated methylation differences have been documented in C. gigas, and OA exposure modified the reproductive tissue methylome in C. gigas and C. virginica (Venkataraman et al., 2020; Sun et al., 2022; Venkataraman et al., 2022). Changes to DNA methylation due to low pH may impact successful reproduction or explain intergenerational effects of these conditions on marine invertebrates. Analysis of DNA methylation enzymes during the maternal-to-zygotic transition in coral M. capitata embryos exposed to OA suggests that methylome programming occurs within 4 hours of fertilization (Chille et al., 2021), further emphasizing the vulnerability of molecular machinery to OA and its influence on carryover effects. Beyond DNA methylation, changes to chromatin organization may influence reproduction and carryover effects. Gametes, particularly sperm, are subject to significant chromatin reorganization and packaging that can affect the availability of genes for methylation or expression (Eirin-Lopez and Putnam, 2018). As successful reproduction hinges on the survival of offspring, understanding genetic and epigenetic mechanisms that drive reproduction is important to elucidate changes to reproductive processes and offspring performance.
Conclusion
Despite the large body of research examining the effects of OA on reproduction, there are notable gaps in the literature (Box 2). In addition to the mechanisms described in the previous section, long-term ecological baselines for reproduction are missing for most organisms. This information is essential for understanding the impacts of OA on organismal phenology and the resilience and plasticity of reproductive processes. More ecologically relevant experimental designs are also needed. For instance, knowledge of acidification effects on spawn timing and synchronicity is, to date, largely based on rates of spawning when induced or other metrics that approximate spawn readiness, such as the number of gravid individuals. Moving forward, it will be important to monitor natural spawn rates and timing in variable pH conditions. Studies on how OA affect mating behavior and sex determination and differentiation are very limited, and additional research into the effects of OA and additional environmental stressors on reproduction are also needed (for a summary of multi-stressor studies to date, see Supplemental Materials).
It is crucial that we expand OA studies to include a more diverse set of species with aquaculture, fisheries, and conservation importance. Of the taxa discussed in this review, those that constitute the largest fisheries and cultured species are among the least studied. Crustaceans are notably underrepresented. In wild fisheries, crustacean taxa represent the largest wild-caught group (6.31 billion tons in 2017), with over half of the fishery comprising shrimp and prawn species (3.60 billion tons combined, FAO, 2019), and to date there are no studies that have looked at the effects of OA on the top farmed marine crustaceans worldwide (FAO, 2018). Cephalopods are another overlooked group. Despite 3.77 billion tons of squid, cuttlefish, and octopus harvested globally in 2017 (FAO, 2019), we identified only one cephalopod study in this review that exposed adults to OA and monitored reproductive activity (Spady et al., 2020). Finally, while cnidarians are among the most threatened by changing oceanic conditions (Hoegh-Guldberg et al., 2017), they are also among the least studied. It will be important for future studies to prioritize impacts of acidification on reproduction in species that support aquaculture and fishing communities, restoration, and those species of conservation concern.
Author contributions
JLP-G, LA, LHS, YRV, and LW contributed to conception and design of the study. LA, LHS, YRV, and LW organized the data base. LS performed statistical analyses. LW and YRV produced the illustrations. JLP-G, LA, LHS, YRV, and LW wrote the manuscript. All authors contributed to manuscript revision, read, and approved the submitted version.
Funding
Alfred P. Sloan Research Fellowship, NOAA-Saltonstall Kennedy, NOAA Ocean Acidification Program (NA21OAR4170315), Kenneth K. Chew Endowed Professorship in Aquaculture, Washington Sea Grant, NSF IOS-IEP (1655682) and NSF CAREER BIO-OCE (2044840) awarded to JLP-G. The Joint Institute for the Study of the Atmosphere and Ocean (JISAO), University of Washington Victor and Tamara Loosanoff Endowed Fellowship awarded to LA. National Science Foundation Graduate Research Fellowship, WHOI Postdoctoral Scholar Program awarded to YRV. National Science Foundation Graduate Research Fellowship, NOAA INTERN program, University of Washington Victor and Tamara Loosanoff Endowed Fellowship awarded to LHS. This work was supported by the School of Aquatic and Fishery Sciences at University of Washington.
Acknowledgments
We would like to thank Nùria Viladrich, Paul McElhany and Eileen Bates for their helpful comments with earlier versions of this manuscript and Evan Fiorenza for guidance on the meta-analysis.
Conflict of interest
The authors declare that the research was conducted in the absence of any commercial or financial relationships that could be construed as a potential conflict of interest.
Publisher’s note
All claims expressed in this article are solely those of the authors and do not necessarily represent those of their affiliated organizations, or those of the publisher, the editors and the reviewers. Any product that may be evaluated in this article, or claim that may be made by its manufacturer, is not guaranteed or endorsed by the publisher.
Supplementary material
The Supplementary Material for this article can be found online at: https://www.frontiersin.org/articles/10.3389/fmars.2022.977754/full#supplementary-material
References
Alavi S. M. H., Cosson J. (2005). Sperm motility in fishes. i. effects of temperature and pH: a review. Cell Biol. Int. 29, 101–110. doi: 10.1016/j.cellbi.11.021
Albright R. (2011a). Effects of ocean acidification on early life history stages of Caribbean scleractinian corals. Hindawi Publishing Corporation, Journal of Marine Biology . Available at: https://scholarship.miami.edu/esploro/outputs/991031447465902976. 2011, 14 doi: 10.1155/2011/473615
Albright R. (2011b). Reviewing the effects of ocean acidification on sexual reproduction and early life history stages of reef-building corals. J. Mar. Biol (Hindawi Publishing Corporation) 2011, 14. doi: 10.1155/2011/473615
Albright R., Mason B. (2013). Projected near-future levels of temperature and pCO2 reduce coral fertilization success. PloS One 8, e56468. doi: 10.1371/journal.pone.0056468
Almén A.-K., Vehmaa A., Brutemark A., Bach L., Lischka S., Stuhr A., et al. (2016). Negligible effects of ocean acidification on Eurytemora affinis (Copepoda) offspring production. Biogeosciences 13, 1037–1048. doi: 10.5194/bg-13-1037-2016
Anand M., Rangesh K., Maruthupandy M., Jayanthi G., Rajeswari B., Priya R. J. (2021). Effect of CO2 driven ocean acidification on calcification, physiology and ovarian cells of tropical sea urchin Salmacis virgulata – a microcosm approach. Heliyon 7 (1), e05970. doi: 10.1016/j.heliyon.2021.e05970
Armstrong E. J., Dubousquet V., Mills S. C., Stillman J. H. (2019). Elevated temperature, but not acidification, reduces fertilization success in the small giant clam, Tridacna maxima. Mar. Biol. 167, 8. doi: 10.1007/s00227-019-3615-0
Babcock R. C., Bull G. D., Harrison P. L., Heyward A. J., Oliver J. K., Wallace C. C., et al. (1986). Synchronous spawnings of 105 scleractinian coral species on the great barrier reef. Mar. Biol. 90, 379–394. doi: 10.1007/BF00428562
Barros P., Sobral P., Range P., Chícharo L., Matias D. (2013). Effects of sea-water acidification on fertilization and larval development of the oyster Crassostrea gigas. J. Exp. Mar. Bio. Ecol. 440, 200–206. doi: 10.1016/j.jembe.2012.12.014
Basallote M. D., Rodríguez-Romero A., De Orte M. R., Del Valls T. Á., Riba I. (2018). CO2 leakage simulation: effects of the pH decrease on fertilisation and larval development of Paracentrotus lividus and sediment metals toxicity. Chem. Ecol. 34, 1–21. doi: 10.1080/02757540.2017.1396319
Bechmann R. K., Taban I. C., Westerlund S., Godal B. F., Arnberg M., Vingen S., et al. (2011). Effects of ocean acidification on early life stages of shrimp (Pandalus borealis) and mussel (Mytilus edulis). J. Toxicol. Environ. Health A 74, 424–438. doi: 10.1080/15287394.2011.550460
Bell A. M., Hellmann J. K. (2019). An integrative framework for understanding the mechanisms and multigenerational consequences of transgenerational plasticity. Annu. Rev. Ecology Evolution Systematics 50, 97–118. doi: 10.1146/annurev-ecolsys-110218-024613
Bernard I., de Kermoysan G., Pouvreau S. (2011). Effect of phytoplankton and temperature on the reproduction of the pacific oyster Crassostrea gigas: Investigation through DEB theory. J. Sea Res. 66, 349–360. doi: 10.1016/j.seares.2011.07.009
Bernhardt J. R., Leslie H. M. (2013). Resilience to climate change in coastal marine ecosystems. Ann. Rev. Mar. Sci. 5, 371–392. doi: 10.1146/annurev-marine-121211-172411
Boch C. A., Litvin S. Y., Micheli F., De Leo G., Aalto E. A., Lovera C., et al. (2017). Effects of current and future coastal upwelling conditions on the fertilization success of the red abalone (Haliotis rufescens). ICES J. Mar. Sci. 74, 1125–1134. doi: 10.1093/icesjms/fsx017
Bögner D. (2016). Life under climate change scenarios: Sea urchins’ cellular mechanisms for reproductive success. J. Mar. Sci. Eng. 4, 28. doi: 10.3390/jmse4010028
Bögner D., Bickmeyer U., Köhler A. (2014). CO2-induced fertilization impairment in Strongylocentrotus droebachiensis collected in the Arctic. Helgol. Mar. Res. 68, 341–356. doi: 10.1007/s10152-014-0394-3
Borges F. O., Figueiredo C., Sampaio E., Rosa R., Grilo T. F. (2018a). Transgenerational deleterious effects of ocean acidification on the reproductive success of a keystone crustacean (Gammarus locusta). Mar. Environ. Res. 138, 55–64. doi: 10.1016/j.marenvres.2018.04.006
Borges F. O., Sampaio E., Figueiredo C., Rosa R., Grilo T. F. (2018b). Hypercapnia-induced disruption of long-distance mate-detection and reduction of energy expenditure in a coastal keystone crustacean. Physiol. Behav. 195, 69–75. doi: 10.1016/j.physbeh.2018.07.023
Boulais M., Chenevert K. J., Demey A. T., Darrow E. S., Robison M. R., Roberts J. P., et al. (2017). Oyster reproduction is compromised by acidification experienced seasonally in coastal regions. Sci. Rep. 7, 13276. doi: 10.1038/s41598-017-13480-3
Boulais M., Suquet M., Arsenault-Pernet E. J., Malo F., Queau I., Pignet P., et al. (2018). pH controls spermatozoa motility in the pacific oyster (Crassostrea gigas). Biol. Open 7 (3), bio031427. doi: 10.1242/bio.031427
Burke L., Reytar K., Spalding M., Perry A. (2011). Reefs at risk revisited (Washington DC: World Resources Institute). Available at: https://hdl.handle.net/20.500.12348/1107.
Bylenga C. H., Cummings V. J., Ryan K. G. (2015). Fertilisation and larval development in an Antarctic bivalve, Laternula elliptica, under reduced pH and elevated temperatures. Mar. Ecol. Prog. Ser. 536, 187–201. doi: 10.3354/meps11436
Byrne M. (2011). Impact of ocean warming and ocean acidification on marine invertebrate life history stages: vulnerabilities and potential for persistence in a changing ocean. Oceanogr. Mar. Biol. Annu. Rev. 49, 1–42. doi: 10.1201/b11009-2
Byrne M. (2012). Global change ecotoxicology: Identification of early life history bottlenecks in marine invertebrates, variable species responses and variable experimental approaches. Mar. Environ. Res. 76, 3–15. doi: 10.1016/j.marenvres.2011.10.004
Byrne M., Foo S. A., Ross P. M., Putnam H. M. (2019). Limitations of cross and multigenerational plasticity for marine invertebrates faced with global climate change. Glob. Change Biol. 26, 80–102. doi: 10.1111/gcb.14882
Byrne M., Gonzalez-Bernat M., Doo S., Foo S., Soars N., Lamare M. (2013). Effects of ocean warming and acidification on embryos and non-calcifying larvae of the invasive sea star Patiriella regularis. Mar. Ecol. Prog. Ser. 473, 235–246. doi: 10.3354/meps10058
Byrne M., Ho M., Selvakumaraswamy P., Nguyen H. D., Dworjanyn S. A., Davis A. R. (2009). Temperature, but not pH, compromises sea urchin fertilization and early development under near-future climate change scenarios. Proc. Biol. Sci. 276, 1883–1888. doi: 10.1098/rspb.2008.1935
Byrne M., Przeslawski R. (2013). Multistressor impacts of warming and acidification of the ocean on marine invertebrates’ life histories. Integr. Comp. Biol. 53, 582–596. doi: 10.1093/icb/ict049
Byrne M., Soars N. A., Ho M. A., Wong E., McElroy D., Selvakumaraswamy P., et al. (2010a). Fertilization in a suite of coastal marine invertebrates from SE Australia is robust to near-future ocean warming and acidification. Mar. Biol. 157, 2061–2069. doi: 10.1007/s00227-010-1474-9
Byrne M., Soars N., Selvakumaraswamy P., Dworjanyn S. A., Davis A. R. (2010b). Sea Urchin fertilization in a warm, acidified and high pCO2 ocean across a range of sperm densities. Mar. Environ. Res. 69, 234–239. doi: 10.1016/j.marenvres.2009.10.014
Caballes C. F., Pratchett M. S., Raymundo M. L., Rivera-Posada J. A. (2017). Environmental tipping points for sperm motility, fertilization, and embryonic development in the crown-of-Thorns starfish. Diversity 9, 10. doi: 10.3390/d9010010
Caetano L. S., Pereira T. M., Envangelista J. D., Cabral D. S., Carvalho Coppo G., de Souza L. A., et al. (2021). Impact on fertility rate and embryo-larval development due to the association acidification, ocean warming and lead contamination of a Sea urchin Echinometra lucunter (Echinodermata: Echinoidea). Bull. Environ. Contam. Toxicol. 106, 923–928. doi: 10.1007/s00128-021-03225-4
Caldwell G. S., Fitzer S., Gillespie C. S., Pickavance G., Turnbull E., Bentley M. G. (2011). Ocean acidification takes sperm back in time. Invertebrate Reprod. Dev. 55, 217–221. doi: 10.1080/07924259.2011.574842
Campbell A. L., Levitan D. R., Hosken D. J., Lewis C. (2016). Ocean acidification changes the male fitness landscape. Sci. Rep. 6, 31250. doi: 10.1038/srep31250
Cao Z., Mu F., Wei X., Sun Y. (2015). Influence of CO2-induced seawater acidification on the development and lifetime reproduction of Tigriopus japonicus Mori1931 938. J. Nat. Hist. 49, 2813–2826. doi: 10.1080/00222933.2015.1034213
Cardoso P. G., Loganimoce E. M., Neuparth T., Rocha M. J., Rocha E., Arenas F. (2018). Interactive effects of increased temperature, pCO2 and the synthetic progestin levonorgestrel on the fitness and breeding of the amphipod Gammarus locusta. Environ. pollut. 236, 937–947. doi: 10.1016/j.envpol.2017.10.065
Caroselli E., Gizzi F., Prada F., Marchini C., Airi V., Kaandorp J., et al. (2019). Low and variable pH decreases recruitment efficiency in populations of a temperate coral naturally present at a CO2 vent. Limnology Oceanography 64, 1059–1069. doi: 10.1002/lno.11097
Cavelier P., Cau J., Morin N., Delsert C. (2017). Early gametogenesis in the pacific oyster: new insights using stem cell and mitotic markers. J. Exp. Biol. 220, 3988–3996. doi: 10.1242/jeb.167734
Challener R. C., Watts S. A., McClintock J. B. (2014). Effects of hypercapnia on aspects of feeding, nutrition, and growth in the edible sea urchin Lytechinus variegatus held in culture. Mar. Freshw. Behav. Physiol. 47, 41–62. doi: 10.1080/10236244.2013.875273
Cheung W. W. L., Dunne J., Sarmiento J. L., Pauly D. (2011). Integrating ecophysiology and plankton dynamics into projected maximum fisheries catch potential under climate change in the northeast Atlantic. ICES J. Mar. Sci. 68, 1008–1018. doi: 10.1093/icesjms/fsr012
Chille E., Strand E., Neder M., Schmidt V., Sherman M., Mass T., et al. (2021). Developmental series of gene expression clarifies maternal mRNA provisioning and maternal-to-zygotic transition in the reef-building coral Montipora capitata. BMC Genomics 22, 1–17. doi: 10.1186/s12864-021-08114-y
Choi K.-H., Jang M.-C., Shin H. H., Lee W.-J., Shin K. (2016). In situ hatching success of calanoid copepod eggs in hypoxic sediments of a coastal bay. J. Coast. Res. 32, 333–338. doi: 10.2112/JCOASTRES-D-14-00096.1
Christen R., Schackmann R. W., Shapiro B. M. (1983). Metabolism of sea urchin sperm. interrelationships between intracellular pH, ATPase activity, and mitochondrial respiration. J. Biol. Chem. 258, 5392–5399. doi: 10.1016/S0021-9258(20)81902-4
Chua C., Leggat W., Moya A., Baird A. H. (2013). Temperature affects the early life history stages of corals more than near future ocean acidification. Mar. Ecol. Prog. Ser. 475, 85–92. doi: 10.3354/meps10077
Ciapa B., Philippe L. (2013). Intracellular and extracellular pH and Ca are bound to control mitosis in the early sea urchin embryo via ERK and MPF activities. PloS One 8, e66113. doi: 10.1371/journal.pone.0066113
Clements J. C., Carver C. E., Mallet M. A., Comeau L. A., Mallet A. L. (2020). CO2-induced low pH in an eastern oyster (Crassostrea virginica) hatchery positively affects reproductive development and larval survival but negatively affects larval shape and size, with no intergenerational linkages. ICES J. Mar. Sci. 78, 349–359. doi: 10.1093/icesjms/fsaa089
Cohen-Rengifo M., Garcia E., Hernandez C. A., Hernandez J. C., Clemente S. (2013). Global warming and ocean acidification affect fertilization and early development of the sea urchin Paracentrotus lividus. Cah. Biol. Mar. 54, 667–675.
Conradi M., Sánchez-Moyano J. E., Galotti A., Jiménez-Gómez F., Jiménez-Melero R., Guerrero F., et al. (2019). CO2 leakage simulation: Effects of the decreasing pH to the survival and reproduction of two crustacean species. Mar. pollut. Bull. 143, 33–41. doi: 10.1016/j.marpolbul.2019.04.020
Cooley S., Schoeman D., Bopp L., Boyd P., Donner S., Ghebrehiwet D. Y., et al. (2022). Oceans and Coastal Ecosystems and Their Services. In: Climate Change 2022: Impacts, Adaptation and Vulnerability. Contribution of Working Group II to the Sixth Assessment Report of the Intergovernmental Panel on Climate Change Pörtner H.-O., Roberts D.C., Tignor M., Poloczanska E.S., Mintenbeck K.. (eds.) Cambridge, UK and New York, NY, USA: Cambridge University Press, pp. 379–550. doi: 10.1017/9781009325844.005
Cripps G., Lindeque P., Flynn K. (2014). Parental exposure to elevated pCO2 influences the reproductive success of copepods. J. Plankton Res. 36, 1165–1174. doi: 10.1093/plankt/fbu052
da Silva Souza L., Pusceddu F. H., Cortez F. S., de Orte M. R., Seabra A. A., Cesar A., et al. (2019). Harmful effects of cocaine byproduct in the reproduction of sea urchin in different ocean acidification scenarios. Chemosphere 236, 124284. doi: 10.1016/j.chemosphere.2019.07.015
Dell’Acqua O., Ferrando S., Chiantore M., Asnaghi V. (2019). The impact of ocean acidification on the gonads of three key Antarctic benthic macroinvertebrates. Aquat. Toxicol. 210, 19–29. doi: 10.1016/j.aquatox.2019.02.012
Dheilly N. M., Lelong C., Huvet A., Kellner K., Dubos M.-P., Riviere G., et al. (2012). Gametogenesis in the pacific oyster Crassostrea gigas: a microarrays-based analysis identifies sex and stage specific genes. PloS One 7, e36353. doi: 10.1371/journal.pone.0036353
Dionísio G., Faleiro F., Bilan M., Rosa I. C., Pimentel M., Serôdio J., et al. (2017). Impact of climate change on the ontogenetic development of “solar-powered” sea slugs. Mar. Ecol. Prog. Ser. 578, 87–97. doi: 10.3354/meps12227
Doney S. C., Fabry V. J., Feely R. A., Kleypas J. A. (2009). Ocean acidification: the other CO2 problem. Ann. Rev. Mar. Sci. 1, 169–192. doi: 10.1146/annurev.marine.010908.163834
Dupont S., Dorey N., Stumpp M., Melzner F., Thorndyke M. (2013). Long-term and trans-life-cycle effects of exposure to ocean acidification in the green sea urchin Strongylocentrotus droebachiensis. Mar. Biol. 160, 1835–1843. doi: 10.1007/s00227-012-1921-x
Dupont S., Ortega-Martínez O., Thorndyke M. (2010). Impact of near-future ocean acidification on echinoderms. Ecotoxicology 19, 449–462. doi: 10.1007/s10646-010-0463-6
Durland E., Waldbusser G., Langdon C. (2019). Comparison of larval development in domesticated and naturalized stocks of the pacific oyster Crassostrea gigas exposed to high pCO2 conditions. Mar. Ecol. Prog. Ser. 621, 107–125. doi: 10.3354/meps12983
Dworjanyn S. A., Byrne M. (2018). Impacts of ocean acidification on sea urchin growth across the juvenile to mature adult life-stage transition is mitigated by warming. Proc. Biol. Sci. 285 (1876), 20172684. doi: 10.1098/rspb.2017.2684
Eads A. R., Kennington W. J., Evans J. P. (2016). Interactive effects of ocean warming and acidification on sperm motility and fertilization in the mussel Mytilus galloprovincialis. Mar. Ecol. Prog. Ser. 562, 101–111. doi: 10.3354/meps11944
Egilsdottir H., Spicer J. I., Rundle S. D. (2009). The effect of CO2 acidified sea water and reduced salinity on aspects of the embryonic development of the amphipod Echinogammarus marinus (Leach). Mar. pollut. Bull. 58, 1187–1191. doi: 10.1016/j.marpolbul.2009.03.017
Eirin-Lopez J. M., Putnam H. M. (2019). Marine environmental epigenetics. Ann. Rev. Mar. Sci. 11, 335–368. doi: 10.1146/annurev-marine-010318-095114
Elliott M., Burdon D., Hemingway K. L., Apitz S. E. (2007). Estuarine, coastal and marine ecosystem restoration: confusing management and science–a revision of concepts. Estuar. Coast. Shelf Sci. 74, 349–366. doi: 10.1016/j.ecss.2007.05.034
Ellis R. P., Davison W., Queirós A. M., Kroeker K. J., Calosi P., Dupont S., et al. (2017). Does sex really matter? explaining intraspecies variation in ocean acidification responses. Biol. Lett. 13 (2), 20160761. doi: 10.1098/rsbl.2016.0761
Engström-Öst J., Holmborn T., Brutemark A., Hogfors H., Vehmaa A., Gorokhova E. (2014). The effects of short-term pH decrease on the reproductive output of the copepod Acartia bifilosa – a laboratory study. Mar. Freshw. Behav. Physiol. 47, 173–183. doi: 10.1080/10236244.2014.919096
Epel D. (1978). Chapter 7 mechanisms of activation of sperm and egg during fertilization of Sea urchin gametes. Curr. Topics Dev. Biol., 12, 185–246. doi: 10.1016/s0070-2153(08)60597-9
Ericson J. (2010). Effects of ocean acidification on fertilisation and early development in polar and temperate marine invertebrates ([Dunedin (New Zealand: University of Otago). Available at: http://hdl.handle.net/10523/2945.
Ericson J. A., Ho M. A., Miskelly A., King C. K., Virtue P., Tilbrook B., et al. (2012). Combined effects of two ocean change stressors, warming and acidification, on fertilization and early development of the Antarctic echinoid Sterechinus neumayeri. Polar Biol. 35, 1027–1034. doi: 10.1007/s00300-011-1150-7
Esposito M. C., Boni R., Cuccaro A., Tosti E., Gallo A. (2020). Sperm motility impairment in free spawning invertebrates under near-future level of ocean acidification: uncovering the mechanism. Front. Mar. Science. 6, 794 doi: 10.3389/fmars.2019.00794
Evans J. P., Sherman C. D. H. (2013). Sexual selection and the evolution of egg-sperm interactions in broadcast-spawning invertebrates. Biol. Bull. 224, 166–183. doi: 10.1086/BBLv224n3p166
Falkenberg L. J., Styan C. A., Havenhand J. N. (2019). Sperm motility of oysters from distinct populations differs in response to ocean acidification and freshening. Sci. Rep. 9, 7970. doi: 10.1038/s41598-019-44321-0
FAO (2018). The state of world fisheries and Aquaculture2012, 018 - meeting the sustainable development goals (Rome: License: CC). Available at: https://www.fao.org/3/i9540en/i9540en.pdf.
FAO (2019). FAO yearbook. fishery and aquaculture Statistics2012, 017/FAO annuaire. statistiques des pêches et de l’aquaculture2012, 017/FAO anuario. estadísticas de pesca y acuicultura2012, 017. Food Agric. Org. 82.
Farley G. S., Levitan D. R. (2001). The role of jelly coats in sperm-egg encounters, fertilization success, and selection on egg size in broadcast spawners. Am. Nat. 157, 626–636. doi: 10.1086/320619
Findlay H. S., Kendall M. A., Spicer J. I., Widdicombe S. (2009). Future high CO2 in the intertidal may compromise adult barnacle Semibalanus balanoides survival and embryonic development rate. Mar. Ecol. Prog. Ser. 389, 193–202. doi: 10.3354/meps08141
Fine M., Tchernov D. (2007). Scleractinian coral species survive and recover from decalcification. Science 315, 1811. doi: 10.1126/science.1137094
Fiorenza E. A., Wendt C. A., Dobkowski K. A., King T. L., Pappaionou M., Rabinowitz P., et al. (2020). It’s a wormy world: Meta-analysis reveals several decades of change in the global abundance of the parasitic nematodes anisakis spp. and Pseudoterranova spp. in marine fishes and invertebrates. Glob. Change Biol. 26, 2854–2866. doi: 10.1111/gcb.15048
Fitzer S. C., Bishop J. D. D., Caldwell G. S., Clare A. S., Upstill-Goddard R. C., Bentley M. G. (2012a). Visualisation of the copepod female reproductive system using confocal laser scanning microscopy and two-photon microscopy. J. Crustacean Biol. 32, 685–692. doi: 10.1163/193724012X639788
Fitzer S. C., Caldwell G. S., Close A. J., Clare A. S., Upstill-Goddard R. C., Bentley M. G. (2012b). Ocean acidification induces multi-generational decline in copepod naupliar production with possible conflict for reproductive resource allocation. J. Exp. Mar. Bio. Ecol. 418-419, 30–36. doi: 10.1016/j.jembe.2012.03.009
Foo S. A. (2015). “Acclimatisation and adaptive capacity of sea urchins in a changing ocean: Effects of ocean warming and acidification on early development and the potential to persist,” (Sydney (Australia: University of Sydney). Available at: http://hdl.handle.net/2123/14988.
Foo S. A., Byrne M. (2017). Marine gametes in a changing ocean: Impacts of climate change stressors on fecundity and the egg. Mar. Environ. Res. 128, 12–24. doi: 10.1016/j.marenvres.2017.02.004
Foo S. A., Deaker D., Byrne M. (2018). Cherchez la femme - impact of ocean acidification on the egg jelly coat and attractants for sperm. J. Exp. Biol. 221(13), jeb177188. doi: 10.1242/jeb.177188
Foo S. A., Dworjanyn S. A., Khatkar M. S., Poore A. G. B., Byrne M. (2014). Increased temperature, but not acidification, enhances fertilization and development in a tropical urchin: potential for adaptation to a tropicalized eastern Australia. Evol. Appl. 7, 1226–1237. doi: 10.1111/eva.12218
Foo S. A., Dworjanyn S. A., Poore A. G. B., Harianto J., Byrne M. (2016). Adaptive capacity of the sea urchin Heliocidaris erythrogramma to ocean change stressors: responses from gamete performance to the juvenile. Mar. Ecol. Prog. Ser. 556, 161–172. doi: 10.3354/meps11841
Frieder C. A. (2014). Present-day nearshore pH differentially depresses fertilization in congeneric sea urchins. Biol. Bull. 226, 1–7. doi: 10.1086/BBLv226n1p1
Froehlich H. E., Gentry R. R., Halpern B. S. (2017). Conservation aquaculture: Shifting the narrative and paradigm of aquaculture’s role in resource management. Biol. Conserv. 215, 162–168. doi: 10.1016/j.biocon.2017.09.012
Gallo A., Boni R., Buia M. C., Monfrecola V., Esposito M. C., Tosti E. (2019). Ocean acidification impact on ascidian Ciona robusta spermatozoa: New evidence for stress resilience. Sci. Total Environ. 697, 134100. doi: 10.1016/j.scitotenv.2019.134100
Gallo A., Esposito M. C., Cuccaro A., Buia M. C., Tarallo A., Monfrecola V., et al. (2020). Adult exposure to acidified seawater influences sperm physiology in Mytilus galloprovincialis: Laboratory and in situ transplant experiments. Environ. pollut. 265, 115063. doi: 10.1016/j.envpol.2020.115063
Galtsoff P. S. (1938). Physiology of reproduction of Ostrea virginica. Biol. Bull. 75, 286–307. doi: 10.2307/1537736
Gao K., Xu J., Gao G., Li Y., Hutchins D. A., Huang B., et al. (2012). Rising CO2 and increased light exposure synergistically reduce marine primary productivity. Nat. Climate Change 2, 519–523. doi: 10.1038/nclimate1507
García E., Hernández J. C., Clemente S. (2018). Robustness of larval development of intertidal sea urchin species to simulated ocean warming and acidification. Mar. Environ. Res. 139, 35–45. doi: 10.1016/j.marenvres.2018.04.011
Gianguzza P., Visconti G., Gianguzza F., Vizzini S., Sarà G., Dupont S. (2014). Temperature modulates the response of the thermophilous sea urchin Arbacia lixula early life stages to CO2-driven acidification. Mar. Environ. Res. 93, 70–77. doi: 10.1016/j.marenvres.2013.07.008
Gibbs M. C., Parker L. M., Scanes E., Byrne M., O’Connor W. A., Ross P. M. (2021a). Adult exposure to ocean acidification and warming leads to limited beneficial responses for oyster larvae. ICES J. Mar. Sci. 78, 2017–2030. doi: 10.1093/icesjms/fsab071
Gibbs M. C., Parker L. M., Scanes E., Byrne M., O’Connor W. A., Ross P. M. (2021b). Adult exposure to ocean acidification and warming remains beneficial for oyster larvae following starvation. ICES J. Mar. Sci. 78, 1587–1598. doi: 10.1093/icesjms/fsab066
Gizzi F., de Mas L., Airi V., Caroselli E., Prada F., Falini G., et al. (2017). Reproduction of an azooxanthellate coral is unaffected by ocean acidification. Sci. Rep. 7, 13049. doi: 10.1038/s41598-017-13393-1
Gonzales-Bernat M. J., Lamare M., Uthicke S., Byrne M. (2013). Fertilization, embryogenesis and larval development in the tropical intertidal sand dollar Arachnoides placenta in response to reduced seawater pH. Mar. Biol. 160, 1927–1194. doi: 10.1007/s00227-012-2034-2
Graham H., Rastrick S. P. S., Findlay H. S., Bentley M. G., Widdicombe S., Clare A. S., et al. (2015). Sperm motility and fertilisation success in an acidified and hypoxic environment. ICES J. Mar. Sci. 73, 783–790. doi: 10.1093/icesjms/fsv171
Gravinese P. M. (2018). Ocean acidification impacts the embryonic development and hatching success of the Florida stone crab, Menippe mercenaria. J. Exp. Mar. Bio. Ecol. 500, 140–146. doi: 10.1016/j.jembe.2017.09.001
Gray M. W., Chaparro O., Huebert K. B., O’Neill S. P., Couture T., Moreira A., et al. (2019). Life history traits conferring larval resistance against ocean acidification: The case of brooding oysters of the genus Ostrea. J. Shellfish Res. 38, 751. doi: 10.2983/035.038.0326
Gray M. W., Salas-Yanquin L. P., Bűchner-Miranda J. A., Chaparro O. R. (2022). The Ostrea chilensis pallial cavity: nursery, prison, and time machine. Mar. Biol. 169, 25. doi: 10.1007/s00227-021-04015-6
Grazer V. M., Martin C. (2012). Investigating climate change and reproduction: experimental tools from evolutionary biology. Biology, 1(2), 411–438.
Guo X., Huang M., Shi B., You W., Ke C. (2020). Effects of ocean acidification on toxicity of two trace metals in two marine molluscs in their early life stages. Aquac. Environ. Interact. 12, 281–296. doi: 10.3354/aei00362
Han Y., Shi W., Tang Y., Zhao X., Du X., Sun S., et al. (2021). Ocean acidification increases polyspermy of a broadcast spawning bivalve species by hampering membrane depolarization and cortical granule exocytosis. Aquat. Toxicol. 231, 105740. doi: 10.1016/j.aquatox.2020.105740
Havenhand J. N., Buttler F.-R., Thorndyke M. C., Williamson J. E. (2008). Near-future levels of ocean acidification reduce fertilization success in a sea urchin. Curr. Biol. 18, R651–R652. doi: 10.1016/j.cub.2008.06.015
Havenhand J. N., Schlegel P. (2009). Near-future levels of ocean acidification do not affect sperm motility and fertilization kinetics in the oyster Crassostrea gigas. Biogeosciences 6, 3009–3015. doi: 10.5194/bg-6-3009-2009
Hazan Y., Wangensteen O. S., Fine M. (2014). Tough as a rock-boring urchin: adult Echinometra sp. EE from the red Sea show high resistance to ocean acidification over long-term exposures. Mar. Biol. 161, 2531–2545. doi: 10.1007/s00227-014-2525-4
Hedgecock D., Pudovkin A. I. (2011). Sweepstakes reproductive success in highly fecund marine fish and shellfish: A review and commentary. Bull. Mar. Sci. 87, 971–1002. doi: 10.5343/bms.2010.1051
Helm M. M., Bourne N., Lovatelli A. (2004). Hatchery culture of bivalves: a practical manual (FAO, Rome). Available at: http://www.sidalc.net/cgi-bin/wxis.exe/?IsisScript=UACHBC.xis&method=post&formato=2&cantidad=1&expresion=mfn=102646.
Hoegh-Guldberg O., Bruno J. F. (2010). The impact of climate change on the world’s marine ecosystems. Science 328, 1523–1528. doi: 10.1126/science.1189930
Hoegh-Guldberg O., Poloczanska E. S., Skirving W., Dove S. (2017). Coral reef ecosystems under climate change and ocean acidification. Front. Mar. Sci. 4. doi: 10.3389/fmars.2017.00158
Ho M. A., Price C., King C. K., Virtue P., Byrne M. (2013). Effects of ocean warming and acidification on fertilization in the Antarctic echinoid Sterechinus neumayeri across a range of sperm concentrations. Mar. Environ. Res. 90, 136–141. doi: 10.1016/j.marenvres.2013.07.007
Horwitz R., Fine M. (2014). High CO2 detrimentally affects tissue regeneration of red Sea corals. Coral Reefs 33, 819–829. doi: 10.1007/s00338-014-1150-5
Howes E., Joos F., Eakin M., Gattuso J.-P. (2015). An updated synthesis of the observed and projected impacts of climate change on the chemical, physical and biological processes in the oceans. Front. Mar. Sci. 2. doi: 10.3389/fmars.2015.00036
Hue T., Chateau O., Lecellier G., Kayal M., Lanos N., Gossuin H., et al. (2020). Temperature affects the reproductive outputs of coral-eating starfish acanthaster spp. after adult exposure to near-future ocean warming and acidification. Mar. Environ. Res. 162, 105164. doi: 10.1016/j.marenvres.2020.105164
Hu M. Y., Lein E., Bleich M., Melzner F., Stumpp M. (2018). Trans-life cycle acclimation to experimental ocean acidification affects gastric pH homeostasis and larval recruitment in the sea star Asterias rubens. Acta Physiol. 224, e13075. doi: 10.1111/apha.13075
Iguchi A., Suzuki A., Sakai K., Nojiri Y. (2015). Comparison of the effects of thermal stress and CO₂-driven acidified seawater on fertilization in coral Acropora digitifera. Zygote 23, 631–634. doi: 10.1017/S0967199414000185
IPCC (2022). Climate Change2022, 022: Impacts, adaptation, and vulnerability. contribution of working group II to the sixth assessment report of the intergovernmental panel on climate change. Eds. Pörtner H.-O., Roberts D. C., Tignor M., Poloczanska E. S., Mintenbeck K., Alegría A., Craig M., Langsdorf S., Löschke S., Möller V., Okem A., Rama B. (Cambridge, UK and New York, NY, USA: Cambridge University Press). 3056.
Isari S., Zervoudaki S., Peters J., Papantoniou G., Pelejero C., Saiz E. (2015). Lack of evidence for elevated CO2-induced bottom-up effects on marine copepods: a dinoflagellate–calanoid prey–predator pair. ICES J. Mar. Sci. 73, 650–658. doi: 10.1093/icesjms/fsv078
Jokiel P. L., Rodgers K. S., Kuffner I. B., Andersson A. J., Cox E. F., Mackenzie F. T. (2008). Ocean acidification and calcifying reef organisms: a mesocosm investigation. Coral Reefs 27, 473–483. doi: 10.1007/s00338-008-0380-9
Jouaux A., Franco A., Heude-Berthelin C., Sourdaine P., Blin J. L., Mathieu M., et al. (2012). Identification of ras, pten and p70S6K homologs in the pacific oyster Crassostrea gigas and diet control of insulin pathway. Gen. Comp. Endocrinol. 176, 28–38. doi: 10.1016/j.ygcen.2011.12.008
Juanchich A., Le Cam A., Montfort J., Guiguen Y., Bobe J. (2013). Identification of differentially expressed miRNAs and their potential targets during fish ovarian development. Biol. Reprod. 88, 128. doi: 10.1095/biolreprod.112.105361
Kamio M., Yambe H., Fusetani N. (2022). Chemical cues for intraspecific chemical communication and interspecific interactions in aquatic environments: applications for fisheries and aquaculture. Fish. Sci. 88, 203–239. doi: 10.1007/s12562-021-01563-0
Kamya P. Z., Dworjanyn S. A., Hardy N., Mos B., Uthicke S., Byrne M. (2014). Larvae of the coral eating crown-of-thorns starfish, Acanthaster planci in a warmer-high CO2 ocean. Glob. Change Biol. 20, 3365–3376. doi: 10.1111/gcb.12530
Kapsenberg L., Okamoto D. K., Dutton J. M., Hofmann G. (2017). Sensitivity of sea urchin fertilization to pH varies across a natural pH mosaic. Ecol. Evol. 7, 1737–1750. doi: 10.1002/ece3.2776
Karelitz S., Lamare M. D., Mos B., De Bari H., Dworjanyn S. A., Byrne M. (2019). Impact of growing up in a warmer, lower pH future on offspring performance: transgenerational plasticity in a pan-tropical sea urchin. Coral Reefs 38, 1085–1095. doi: 10.1007/s00338-019-01855-z
Kimura R., Takami H., Ono T., Onitsuka T., Nojiri Y. (2011). Effects of elevated pCO2 on the early development of the commercially important gastropod, ezo abalone Haliotis discus hannai. Fish. Oceanogr. 20, 357–366. doi: 10.1111/j.1365-2419.2011.00589.x
Kita J., Kikkawa T., Asai T., Ishimatsu A. (2013). Effects of elevated pCO2 on reproductive properties of the benthic copepod Tigriopus japonicus and gastropod Babylonia japonica. Mar. pollut. Bull. 73, 402–408. doi: 10.1016/j.marpolbul.2013.06.026
Kong H., Jiang X., Clements J. C., Wang T., Huang X., Shang Y., et al. (2019). Transgenerational effects of short-term exposure to acidification and hypoxia on early developmental traits of the mussel Mytilus edulis. Mar. Environ. Res. 145, 73–80. doi: 10.1016/j.marenvres.2019.02.011
Korpelainen H. (1990). Sex ratios and conditions required for environmental sex determination in animals. Biol. Rev. Camb. Philos. Soc 65, 147–184. doi: 10.1111/j.1469-185x.1990.tb01187.x
Kroeker K. J., Kordas R. L., Crim R. N., Singh G. G. (2010). Meta-analysis reveals negative yet variable effects of ocean acidification on marine organisms. Ecol. Lett. 13, 1419–1434. doi: 10.1111/j.1461-0248.2010.01518.x
Kurihara H. (2008). Effects of CO2-driven ocean acidification on the early developmental stages of invertebrates. Mar. Ecol. Prog. Ser. 373, 275–284. doi: 10.3354/meps07802
Kurihara H., Ishimatsu A. (2008). Effects of high CO2 seawater on the copepod (Acartia tsuensis) through all life stages and subsequent generations. Mar. pollut. Bull. 56, 1086–1090. doi: 10.1016/j.marpolbul.2008.03.023
Kurihara H., Matsui M., Furukawa H., Hayashi M., Ishimatsu A. (2008). Long-term effects of predicted future seawater CO2 conditions on the survival and growth of the marine shrimp Palaemon pacificus. J. Exp. Mar. Bio. Ecol. 367, 41–46. doi: 10.1016/j.jembe.2008.08.016
Kurihara H., Shimode S., Shirayama Y. (2004). Effects of raised CO2 concentration on the egg production rate and early development of two marine copepods (Acartia steueri and Acartia erythraea). Mar. pollut. Bull. 49, 721–727. doi: 10.1016/j.marpolbul.2004.05.005
Kurihara H., Shirayama Y. (2004). Effects of increased atmospheric CO2 on sea urchin early development. Mar. Ecol. Prog. Ser. 274, 161–169. doi: 10.3354/meps274161
Kurihara H., Yin R., Nishihara G. N., Soyano K., Ishimatsu A. (2013). Effect of ocean acidification on growth, gonad development and physiology of the sea urchin Hemicentrotus pulcherrimus. Aquat. Biol. 18, 281–292. doi: 10.3354/ab00510
Langer J. A. F., Meunier C. L., Ecker U., Horn H. G., Schwenk K., Boersma M. (2019). Acclimation and adaptation of the coastal calanoid copepod Acartia tonsa to ocean acidification: a long-term laboratory investigation. Mar. Ecol. Prog. Ser. 619, 35–51. doi: 10.3354/meps12950
Lawrence A. J., Soame J. M. (2004). The effects of climate change on the reproduction of coastal invertebrates. Ibis 146, 29–39. doi: 10.1111/j.1474-919X.2004.00325.x
Lee E. H., Choi S. Y., Seo M. H., Lee S. J., Soh H. Y. (2020). Effects of temperature and pH on the egg production and hatching success of a common Korean copepod. Diversity 12, 372. doi: 10.3390/d12100372
Lee Y. H., Kang H.-M., Kim M.-S., Wang M., Kim J. H., Jeong C.-B., et al. (2019). Effects of ocean acidification on life parameters and antioxidant system in the marine copepod Tigriopus japonicus. Aquat. Toxicol. 212, 186–193. doi: 10.1016/j.aquatox.2019.05.007
Le Moullac G., Soyez C., Vidal-Dupiol J., Belliard C., Fievet J., Sham-Koua M., et al. (2016). Impact of pCO2 on the energy, reproduction and growth of the shell of the pearl oyster Pinctada margaritifera. Estuar. Coast. Shelf Sci. 182, 274–282. doi: 10.1016/j.ecss.2016.03.011
Lenz B., Fogarty N. D., Figueiredo J. (2019). Effects of ocean warming and acidification on fertilization success and early larval development in the green sea urchin Lytechinus variegatus. Mar. pollut. Bull. 141, 70–78. doi: 10.1016/j.marpolbul.2019.02.018
Liberman R., Fine M., Benayahu Y. (2021). Simulated climate change scenarios impact the reproduction and early life stages of a soft coral. Mar. Environ. Res. 163, 105215. doi: 10.1016/j.marenvres.2020.105215
Limatola N., Chun J. T., Santella L. (2020). Effects of salinity and pH of seawater on the reproduction of the Sea urchin Paracentrotus lividus. Biol. Bull. 239, 13–23. doi: 10.1086/710126
Liu S., Shi W., Guo C., Zhao X., Han Y., Peng C., et al. (2016). Ocean acidification weakens the immune response of blood clam through hampering the NF-kappa β and toll-like receptor pathways. Fish Shellfish Immunol. 54, 322–327. doi: 10.1016/j.fsi.2016.04.030
Long C. W., Swiney K. M., Foy R. J. (2013). Effects of ocean acidification on the embryos and larvae of red king crab, Paralithodes camtschaticus. Mar. pollut. Bull. 69, 38–47. doi: 10.1016/j.marpolbul.2013.01.011
Lucey N. M., Lombardi C., DeMarchi L., Schulze A., Gambi M. C., Calosi P. (2015). To brood or not to brood: Are marine invertebrates that protect their offspring more resilient to ocean acidification? Sci. Rep. 5, 12009. doi: 10.1038/srep12009
Lymbery R. A., Kennington W. J., Cornwall C. E., Evans J. P. (2019). Ocean acidification during prefertilization chemical communication affects sperm success. Ecol. Evol. 49, 1. doi: 10.1002/ece3.5720
Maboloc E. A., Chan K. Y. K. (2021). Parental whole life cycle exposure modulates progeny responses to ocean acidification in slipper limpets. Glob. Change Biol. 27, 3272–3281. doi: 10.1111/gcb.15647
Machado A. M., Fernández-Boo S., Nande M., Pinto R., Costas B., Castro L. F. C. (2022). The male and female gonad transcriptome of the edible sea urchin, Paracentrotus lividus: Identification of sex-related and lipid biosynthesis genes. Aquaculture Rep. 22, 100936. doi: 10.1016/j.aqrep.2021.100936
Mackenzie C. L., Lynch S. A., Culloty S. C., Malham S. K. (2014). Future oceanic warming and acidification alter immune response and disease status in a commercial shellfish species, Mytilus edulis l. PloS One 9, e99712. doi: 10.1371/journal.pone.0099712
Manno C., Peck V. L., Tarling G. A. (2016). Pteropod eggs released at high pCO2 lack resilience to ocean acidification. Sci. Rep. 6, 25752. doi: 10.1038/srep25752
Marčeta T., Matozzo V., Alban S., Badocco D., Pastore P., Marin M. G. (2020). Do males and females respond differently to ocean acidification? an experimental study with the sea urchin Paracentrotus lividus. Environ. Sci. Pollut. Res. Int. 27 (31), 39516–39530. doi: 10.1007/s11356-020-10040-7
Marchini C., Gizzi F., Pondrelli T., Moreddu L., Marisaldi L., Montori F., et al. (2021). Decreasing pH impairs sexual reproduction in a Mediterranean coral transplanted at a CO2 vent. Limnology Oceanography 66, 3990–4000. doi: 10.1002/lno.11937
Martin S., Richier S., Pedrotti M.-L., Dupont S., Castejon C., Gerakis Y., et al. (2011). Early development and molecular plasticity in the Mediterranean sea urchin Paracentrotus lividus exposed to CO2-driven acidification. J. Exp. Biol. 214, 1357–1368. doi: 10.1242/jeb.051169
Mayor D. J., Matthews C., Cook K., Zuur A. F., Hay S. (2007). CO2-induced acidification affects hatching success in Calanus finmarchicus. Mar. Ecol. Prog. Ser. 350, 91–97. doi: 10.3354/meps07142
McConville K., Halsband C., Fileman E. S., Somerfield P. J., Findlay H. S., Spicer J. I. (2013). Effects of elevated CO2 on the reproduction of two calanoid copepods. Mar. pollut. Bull. 73, 428–434. doi: 10.1016/j.marpolbul.2013.02.010
McDonald M. R., McClintock J. B., Amsler C. D., Rittschof D., Angus R. A., Orihuela B., et al. (2009). Effects of ocean acidification over the life history of the barnacle Amphibalanus amphitrite. Mar. Ecol. Prog. Ser. 385, 179–187. doi: 10.3354/meps08099
Melzner F., Mark F. C., Seibel B. A., Tomanek L. (2020). Ocean acidification and coastal marine invertebrates: Tracking CO2 effects from seawater to the cell. Ann. Rev. Mar. Sci. 12, 499–523 doi: 10.1146/annurev-marine-010419-010658
Meseck S. L., Alix J. H., Swiney K. M., Long W. C., Wikfors G. H., Foy R. J. (2016). Ocean acidification affects hemocyte physiology in the tanner crab (Chionoecetes bairdi). PloS One 11, e0148477. doi: 10.1371/journal.pone.0148477
Miller J. J., Maher M., Bohaboy E., Friedman C. S., McElhany P. (2016). Exposure to low pH reduces survival and delays development in early life stages of dungeness crab (Cancer magister). Mar. Biol. 163 (5), 1–11. doi: 10.1007/s00227-016-2883-1
Miyazaki S. (2006). Thirty years of calcium signals at fertilization. Semin. Cell Dev. Biol. 17, 233–243. doi: 10.1016/j.semcdb.2006.02.007
Moran A. L., McAlister J. S. (2009). Egg size as a life history character of marine invertebrates: Is it all it’s cracked up to be? Biol. Bull. 216 (3), 226–242. doi: 10.1086/BBLv216n3p226
Morisawa M., Yoshida M. (2005). Activation of motility and chemotaxis in the spermatozoa: From invertebrates to humans. Reprod. Med. Biol. 4, 101–114. doi: 10.1111/j.1447-0578.2005.00099.x
Morita M., Nishikawa A., Nakajima A., Iguchi A., Sakai K., Takemura A., et al. (2006). Eggs regulate sperm flagellar motility initiation, chemotaxis and inhibition in the coral Acropora digitifera, A. gemmifera and A. tenuis. J. Exp. Biol. 209, 4574–4579. doi: 10.1242/jeb.02500
Morita M., Suwa R., Iguchi A., Nakamura M., Shimada K., Sakai K., et al. (2010). Ocean acidification reduces sperm flagellar motility in broadcast spawning reef invertebrates. Zygote 18, 103–107. doi: 10.1017/S0967199409990177
Mortensen T., Mortensen T. (1921). Studies of the development and larval forms of echinoderms. GEC Gad. doi: 10.5962/bhl.title.11376
Moulin L., Catarino A. I., Claessens T., Dubois P. (2011). Effects of seawater acidification on early development of the intertidal sea urchin Paracentrotus lividus (Lamarck1811, 816). Mar. pollut. Bull. 62, 48–54. doi: 10.1016/j.marpolbul.2010.09.012
Nakajima A., Morita M., Takemura A., Kamimura S., Okuno M. (2005). Increase in intracellular pH induces phosphorylation of axonemal proteins for activation of flagellar motility in starfish sperm. J. Exp. Biol. 208, 4411–4418. doi: 10.1242/jeb.01906
Nakamura M., Morita M. (2012). Sperm motility of the scleractinian coral Acropora digitifera under preindustrial, current, and predicted ocean acidification regimes. Aquat. Biol. 15, 299–302. doi: 10.3354/ab00436
Olischläger M., Wild C. (2020). How does the sexual reproduction of marine life respond to ocean acidification? Diversity 12, 241. doi: 10.3390/d12060241
Omoregie E., Mwatilifange N. S. I., Liswaniso G. (2019). Futuristic ocean acidification levels reduce growth and reproductive viability in the pacific oyster (Crassostrea gigas). J. Appl. Sci. Environ. Manage. 23, 1747–1754. doi: 10.4314/jasem.v23i9.21
Onitsuka T., Kimura R., Ono T., Takami H., Nojiri Y. (2014). Effects of ocean acidification on the early developmental stages of the horned turban, Turbo cornutus. Mar. Biol. 161, 1127–1138. doi: 10.1007/s00227-014-2405-y
Pansch C., Hattich G. S. I., Heinrichs M. E., Pansch A., Zagrodzka Z., Havenhand J. N. (2018). Long-term exposure to acidification disrupts reproduction in a marine invertebrate. PloS One 13, e0192036. doi: 10.1371/journal.pone.0192036
Parker L. M., O’Connor W. A., Byrne M., Coleman R. A., Virtue P., Dove M., et al. (2017). Adult exposure to ocean acidification is maladaptive for larvae of the Sydney rock oyster Saccostrea glomerata in the presence of multiple stressors. Biol. Lett. 13 (2), 20160798. doi: 10.1098/rsbl.2016.0798
Parker L. M., O’Connor W. A., Byrne M., Dove M., Coleman R. A., Pörtner H.-O., et al. (2018). Ocean acidification but not warming alters sex determination in the Sydney rock oyster, Saccostrea glomerata. Proc. R. Soc B 285, 20172869. doi: 10.1098/rspb.2017.2869
Parker L. M., Ross P. M., O’connor W. A. (2009). The effect of ocean acidification and temperature on the fertilization and embryonic development of the Sydney rock oyster Saccostrea glomerata (Gould1851, 850). Glob. Change Biol. 15, 2123–2136. doi: 10.1111/j.1365-2486.2009.01895.x
Parker L. M., Ross P. M., O’Connor W. A. (2010). Comparing the effect of elevated pCO2 and temperature on the fertilization and early development of two species of oysters. Mar. Biol. 157, 2435–2452. doi: 10.1007/s00227-010-1508-3
Parker L. M., Ross P. M., O’Connor W. A. (2011). Populations of the Sydney rock oyster, Saccostrea glomerata, vary in response to ocean acidification. Mar. Biol. 158, 689–697. doi: 10.1007/s00227-010-1592-4
Parker L. M., Ross P. M., O’Connor W. A., Borysko L., Raftos D. A., Pörtner H.-O. (2012). Adult exposure influences offspring response to ocean acidification in oysters. Glob. Change Biol. 18, 82–92. doi: 10.1111/j.1365-2486.2011.02520.x
Parker L. M., Scanes E., O’Connor W. A., Ross P. M. (2021). Transgenerational plasticity responses of oysters to ocean acidification differ with habitat. J. Exp. Biol. 224 (12), 12. doi: 10.1242/jeb.239269
Pauletto M., Milan M., Huvet A., Corporeau C., Suquet M., Planas J. V., et al. (2017). Transcriptomic features of Pecten maximus oocyte quality and maturation. PloS One 12, e0172805. doi: 10.1371/journal.pone.0172805
Pecorino D., Barker M. F., Dworjanyn S. A., Byrne M., Lamare M. D. (2014). Impacts of near future sea surface pH and temperature conditions on fertilisation and embryonic development in Centrostephanus rodgersii from northern new Zealand and northern new south Wales, Australia. Mar. Biol. 161, 101–110. doi: 10.1007/s00227-013-2318-1
Pereira T. M., Gnocchi K. G., Merçon J., Mendes B., Lopes B. M., Passos L. S., et al. (2020). The success of the fertilization and early larval development of the tropical sea urchin Echinometra lucunter (Echinodermata: Echinoidea) is affected by the pH decrease and temperature increase. Mar. Environ. Res. 161, 105106. doi: 10.1016/j.marenvres.2020.105106
Pitts K. A., Campbell J. E., Figueiredo J., Fogarty N. D. (2020). Ocean acidification partially mitigates the negative effects of warming on the recruitment of the coral, Orbicella faveolata. Coral Reefs 39, 281–292. doi: 10.1007/s00338-019-01888-4
Podolsky R. D. (2004). Life-history consequences of investment in free-spawned eggs and their accessory coats. Am. Nat. 163, 735–753. doi: 10.1086/382791
Prezeslawski R., Byrne M., Mellin C. (2017). A review and meta-analysis of the effects of multiple abiotic stressors on marine embryos and larvae. Glob. Change Biol. 21, 2122–2140. doi: 10.1111/gcb.12833
Putnam H. M., Ritson-Williams R., Cruz J. A., Davidson J. M., Gates R. D. (2020). Environmentally-induced parental or developmental conditioning influences coral offspring ecological performance. Sci. Rep. 10, 13664. doi: 10.1038/s41598-020-70605-x
Rains S. A. M., Wilberg M. J., Miller T. J. (2016). Sex ratios and average sperm per female blue crab Callinectes sapidus in six tributaries of Chesapeake bay. Mar. Coast. Fish. 8, 492–501. doi: 10.1080/19425120.2016.1208126
Ramirez Llodra E. (2002). Fecundity and life-history strategies in marine invertebrates. Adv. Mar. Biol. 43, 87–170. doi: 10.1016/s0065-2881(02)43004-0
Reed A. J., Godbold J. A., Solan M., Grange L. J. (2021). Invariant gametogenic response of dominant infaunal bivalves from the Arctic under ambient and near-future climate change conditions. Front. Mar. Sci. 8. doi: 10.3389/fmars.2021.576746
Reuter K. E., Lotterhos K. E., Crim R. N., Thompson C. A., Harley C. D. G. (2011). Elevated pCO2 increases sperm limitation and risk of polyspermy in the red sea urchin Strongylocentrotus franciscanus. Glob. Change Biol. 17, 163–171. doi: 10.1111/j.1365-2486.2010.02216.x
Riba I., Gabrielyan B., Khosrovyan A., Luque A., Del Valls T. A. (2016). The influence of pH and waterborne metals on egg fertilization of the blue mussel (Mytilus edulis), the oyster (Crassostrea gigas) and the sea urchin (Paracentrotus lividus). Environ. Sci. pollut. Res. Int. 23, 14580–14588. doi: 10.1007/s11356-016-6611-7
Richmond R. H., Hunter C. L. (1990). Reproduction and recruitment of corals: comparisons among the Caribbean, the tropical pacific, and the red Sea. Mar. Ecol. Prog. Ser. 60, 185–203. doi: 10.3354/meps060185
Rinkevich B. (1996). Do reproduction and regeneration in damaged corals compete for energy allocation? Mar. Ecol. Prog. Ser. 143, 297–302. doi: 10.3354/meps143297
Rinkevich B., Loya Y. (1989). Reproduction in regenerating colonies of the coral Stylophora pistillata. Environ. Qual. ecosystem stability 4, 257–265.
Roggatz C. C., Lorch M., Hardege J. D., Benoit D. M. (2016). Ocean acidification affects marine chemical communication by changing structure and function of peptide signaling molecules. Glob. Change Biol. 22, 3914–3926. doi: 10.1111/gcb.13354
Rossin A. M., Waller R. G., Stone R. P. (2019). The effects of in-vitro pH decrease on the gametogenesis of the red tree coral, Primnoa pacifica. PloS One 14, e0203976. doi: 10.1371/journal.pone.0203976
Rossi G. S., Tunnicliffe V. (2017). Trade-offs in a high CO2 habitat on a subsea volcano: condition and reproductive features of a bathymodioline mussel. Mar. Ecol. Prog. Ser. 574, 49–64. doi: 10.3354/meps12196
Ross P. M., Parker L., Byrne M. (2016). Transgenerational responses of molluscs and echinoderms to changing ocean conditions. ICES J. Mar. Sci. 73, 537–549. doi: 10.1093/icesjms/fsv254
Ross P. M., Parker L., O’Connor W. A., Bailey E. A. (2011). The impact of ocean acidification on reproduction, early development and settlement of marine organisms. Water 3, 1005–1030. doi: 10.3390/w3041005
Świeżak J., Borrero-Santiago A. R., Sokołowski A., Olsen A. J. (2018). Impact of environmental hypercapnia on fertilization success rate and the early embryonic development of the clam Limecola balthica (Bivalvia, tellinidae) from the southern Baltic Sea - a potential CO2 leakage case study. Mar. pollut. Bull. 136, 201–211. doi: 10.1016/j.marpolbul.2018.09.007
Sanford E., Kelly M. W. (2011). Local adaptation in marine invertebrates. Ann. Rev. Mar. Sci. 3, 509–535. doi: 10.1146/annurev-marine-120709-142756
Sawada H., Saito T. (2022). Mechanisms of sperm-egg interactions: What ascidian fertilization research has taught us. CElls. 11, 2096. doi: 10.3390/cells11132096
Scanes E., Parker L. M., O’Connor W. A., Gibbs M. C., Ross P. M. (2018). Copper and ocean acidification interact to lower maternal investment, but have little effect on adult physiology of the Sydney rock oyster Saccostrea glomerata. Aquat. Toxicol. 203, 51–60. doi: 10.1016/j.aquatox.2018.07.020
Scanes E., Parker L. M., O’Connor W. A., Ross P. M. (2014). Mixed effects of elevated pCO2 on fertilisation, larval and juvenile development and adult responses in the mobile subtidal scallop Mimachlamys asperrima (Lamarck1811 819). PloS One 9, e93649. doi: 10.1371/journal.pone.0093649
Schlegel P., Binet M. T., Havenhand J. N., Doyle C. J., Williamson J. E. (2015). Ocean acidification impacts on sperm mitochondrial membrane potential bring sperm swimming behaviour near its tipping point. J. Exp. Biol. 218, 1084–1090. doi: 10.1242/jeb.114900
Schlegel P., Havenhand J. N., Gillings M. R., Williamson J. E. (2012). Individual variability in reproductive success determines winners and losers under ocean acidification: a case study with sea urchins. PloS One 7, e53118. doi: 10.1371/journal.pone.0053118
Schutter M., Nozawa Y., Kurihara H. (2015). The effect of elevated CO2 and increased temperature on in vitro fertilization success and initial embryonic development of single male: female crosses of broad-cast spawning corals at mid-and high-latitude locations. J. Mar. Sci. Eng. 3, 216–239. doi: 10.3390/jmse3020216
Sewell M. A., Hofmann G. E. (2011). Antarctic Echinoids and climate change: a major impact on the brooding forms. Glob. Change Biol. 17, 734–744. doi: 10.1111/j.1365-2486.2010.02288.x
Sewell M. A., Millar R. B., Yu P. C., Kapsenberg L., Hofmann G. E. (2014). Ocean acidification and fertilization in the Antarctic sea urchin Sterechinus neumayeri: the importance of polyspermy. Environ. Sci. Technol. 48, 713–722. doi: 10.1021/es402815s
Sherwood L., Klandorf H., Yancey P. (2012). Animal physiology: From genes to organisms (Brooks/Cole, Belmont, California USA: Cengage Learning).
Shi W., Han Y., Guo C., Zhao X., Liu S., Su W., et al. (2017a). Ocean acidification hampers sperm-egg collisions, gamete fusion, and generation of Ca2+ oscillations of a broadcast spawning bivalve, Tegillarca granosa. Mar. Environ. Res. 130, 106–112. doi: 10.1016/j.marenvres.2017.07.016
Shi W., Zhao X., Han Y., Guo C., Liu S., Su W., et al. (2017b). Effects of reduced pH and elevated pCO2 on sperm motility and fertilisation success in blood clam, Tegillarca granosa. N. Z. J. Mar. Freshw. Res. 51, 543–554. doi: 10.1080/00288330.2017.1296006
Shlesinger T., Loya Y. (2019). Breakdown in spawning synchrony: A silent threat to coral persistence. Science 365, 1002–1007. doi: 10.1126/science.aax0110
Shukla P. R., Skea J., Calvo Buendia E., Masson-Delmotte V., Pörtner H. O., Roberts D. C., et al. (2019). IPCC2012 019: Climate change and land: an IPCC special report on climate change, desertification, land degradation, sustainable land management, food security, and greenhouse gas fluxes in terrestrial ecosystems (Geneva, Switzerland: Intergovernmental Panel on Climate Change (IPCC). Available at: https://spiral.imperial.ac.uk/bitstream/10044/1/76618/2/SRCCL-Full-Report-Compiled-191128.pdf.
Siikavuopio S. I., Mortensen A., Dale T., Foss A. (2007). Effects of carbon dioxide exposure on feed intake and gonad growth in green sea urchin, Strongylocentrotus droebachiensis. Aquaculture 266, 97–101. doi: 10.1016/j.aquaculture.2007.02.044
Smith K. E., Byrne M., Deaker D., Hird C. M., Nielson C., Wilson-McNeal A., et al. (2019). Sea Urchin reproductive performance in a changing ocean: poor males improve while good males worsen in response to ocean acidification. Proc. Biol. Sci. 286, 20190785. doi: 10.1098/rspb.2019.0785
Smith H. W., Clowes G. H. A. (1924). The influence of hydrogen ion concentration on the fertilization process in Arbacia, asterias and Chaetopterus eggs. Biol. Bull. 47, 333–344. doi: 10.2307/1536693
Smith J. N., Richter C., Fabricius K. E., Cornils A. (2017). Pontellid copepods, Labidocera spp., affected by ocean acidification: A field study at natural CO2 seeps. PloS One 12, e0175663. doi: 10.1371/journal.pone.0175663
Sokolova I. M., Frederich M., Bagwe R., Lannig G., Sukhotin A. A. (2012). Energy homeostasis as an integrative tool for assessing limits of environmental stress tolerance in aquatic invertebrates. Mar. Environ. Res. 79, 1–15. doi: 10.1016/j.marenvres.2012.04.003
Spady B. L., Munday P. L., Watson S.-A. (2020). Elevated seawater pCO2 affects reproduction and embryonic development in the pygmy squid, Idiosepius pygmaeus. Mar. Environ. Res. 153, 104812. doi: 10.1016/j.marenvres.2019.104812
Spalding M. D., Fox H. E., Allen G. R., Davidson N., Ferdaña Z. A., Finlayson M., et al. (2007). Marine ecoregions of the world: A bioregionalization of coastal and shelf areas. Bioscience 57, 573–583. doi: 10.1641/B570707
Spencer L. H., Venkataraman Y. R., Crim R., Ryan S., Horwith M. J., Roberts S. B. (2020). Carryover effects of temperature and pCO2 across multiple Olympia oyster populations. Ecol. Appl. 30, e02060. doi: 10.1002/eap.2060
Stavroff L.-A. (2014). Effects of ocean acidification combined with multiple stressors on early life stages of the pacific purple sea urchin (Colwood (BC: Royal Roads University). Available at: http://hdl.handle.net/10170/702.
Striewski S. (2012). Impact of ocean acidification on the reproduction, recruitment and growth of scleractinian corals (Bochum (Germany: Ruhr-University Bochum). Available at: https://nbn-resolving.org/urn:nbn:de:hbz:294-46934.
Stumpp M., Trübenbach K., Brennecke D., Hu M. Y., Melzner F. (2012). Resource allocation and extracellular acid–base status in the sea urchin Strongylocentrotus droebachiensis in response to CO2 induced seawater acidification. Aquat. Toxicol. 110-111, 194–207. doi: 10.1016/j.aquatox.2011.12.020
Suckling C. C., Clark M. S., Beveridge C., Brunner L., Hughes A. D., Harper E. M., et al. (2014). Experimental influence of pH on the early life-stages of sea urchins II: increasing parental exposure times gives rise to different responses. Invertebr. Reprod. Dev. 58, 161–175. doi: 10.1080/07924259.2013.875951
Suckling C. C., Clark M. S., Richard J., Morley S. A., Thorne M. A. S., Harper E. M., et al. (2015). Adult acclimation to combined temperature and pH stressors significantly enhances reproductive outcomes compared to short-term exposures. J. Anim. Ecol. 84, 773–784. doi: 10.1111/1365-2656.12316
Sui Y., Zhou K., Lai Q., Yao Z., Gao P. (2019). Effects of seawater acidification on early development of clam Cyclina sinensis. J. Ocean Univ. China 18, 913–918. doi: 10.1007/s11802-019-3942-2
Sung C.-G., Kim T. W., Park Y.-G., Kang S.-G., Inaba K., Shiba K., et al. (2014). Species and gamete-specific fertilization success of two sea urchins under near future levels of pCO2. J. Mar. Syst. 137, 67–73. doi: 10.1016/j.jmarsys.2014.04.013
Sun D., Li Q., Yu H. (2022). DNA Methylation differences between male and female gonads of the oyster reveal the role of epigenetics in sex determination. Gene 820,146260. doi: 10.1016/j.gene.2022.146260
Swiney K. M., Long W. C., Foy R. J. (2015). Effects of high pCO2 on tanner crab reproduction and early life history–part I: long-term exposure reduces hatching success and female calcification, and alters embryonic development. ICES J. Mar. Sci. 73, 825–835. doi: 10.1093/icesjms/fsv201
Szalaj D., De Orte M. R., Goulding T. A., Medeiros I. D., DelValls T. A., Cesar A. (2017). The effects of ocean acidification and a carbon dioxide capture and storage leak on the early life stages of the marine mussel Perna perna (Linneaus1751 758) and metal bioavailability. Environ. Sci. pollut. Res. Int. 24, 765–781. doi: 10.1007/s11356-016-7863-y
Tanabe T., Yuan Y., Nakamura S., Itoh N., Takahashi K. G., Osada M. (2010). The role in spawning of a putative serotonin receptor isolated from the germ and ciliary cells of the gonoduct in the gonad of the Japanese scallop, Patinopecten yessoensis. Gen. Comp. Endocrinol. 166, 620–627. doi: 10.1016/j.ygcen.2010.01.014
Tarrant A. M. (2007). Hormonal signaling in cnidarians: do we understand the pathways well enough to know whether they are being disrupted? Ecotoxicology 16, 5–13. doi: 10.1007/s10646-006-0121-1
Thor P., Dupont S. (2015). Transgenerational effects alleviate severe fecundity loss during ocean acidification in a ubiquitous planktonic copepod. Glob. Change Biol. 21, 2261–2271. doi: 10.1111/gcb.12815
Thor P., Vermandele F., Carignan M.-H., Jacque S., Calosi P. (2018). No maternal or direct effects of ocean acidification on egg hatching in the Arctic copepod Calanus glacialis. PloS One 13, e0192496. doi: 10.1371/journal.pone.0192496
Timpane-Padgham B. L., Beechie T., Klinger T. (2017). A systematic review of ecological attributes that confer resilience to climate change in environmental restoration. PloS One 12, e0173812. doi: 10.1371/journal.pone.0173812
Treen N., Itoh N., Miura H., Kikuchi I., Ueda T., Takahashi K. G., et al. (2012). Mollusc gonadotropin-releasing hormone directly regulates gonadal functions: a primitive endocrine system controlling reproduction. Gen. Comp. Endocrinol. 176, 167–172. doi: 10.1016/j.ygcen.2012.01.008
Uthicke S., Liddy M., Nguyen H. D., Byrne M. (2014). Interactive effects of near-future temperature increase and ocean acidification on physiology and gonad development in adult pacific sea urchin, Echinometra sp. a. Coral Reefs 33, 831–845. doi: 10.1007/s00338-014-1165-y
Uthicke S., Patel F., Karelitz S., Luter H. M., Webster N. S., Lamare M. (2020). Key biological responses over two generations of the sea urchin Echinometra sp. a under future ocean conditions. Mar. Ecol. Prog. Ser. 637, 87–101. doi: 10.3354/meps13236
Uthicke S., Pecorino D., Albright R., Negri A. P., Cantin N., Liddy M., et al. (2013). Impacts of ocean acidification on early life-history stages and settlement of the coral-eating sea star Acanthaster planci. PloS One 8, e82938. doi: 10.1371/journal.pone.0082938
Vacquier V. D. (1998). Evolution of gamete recognition proteins. Science 281 (5385), 1995–1998. doi: 10.1126/science.281.5385.1995
Van Colen C., Debusschere E., Braeckman U., Van Gansbeke D., Vincx M. (2012). The early life history of the clam Macoma balthica in a high CO2 world. PloS One 7, e44655. doi: 10.1371/journal.pone.0044655
Van Veghel M. L. J., Bak R. P. M. (1994). Reproductive characteristics of the polymorphic Caribbean reef building coral Montastrea annularis. III. reproduction in damaged and regenerating colonies. Mar. Ecol. Prog. Ser. 109, 229–233. doi: 10.3354/meps109229
Vehmaa A., Almén A.-K., Brutemark A., Paul A., Riebesell U., Furuhagen S., et al. (2016). Ocean acidification challenges copepod reproductive plasticity. Biogeosciences. 13, 6171–6182. doi: 10.5194/bgd-12-18541-2015
Vehmaa A., Brutemark A., Engström-Öst J. (2012). Maternal effects may act as an adaptation mechanism for copepods facing pH and temperature changes. PloS One 7, e48538. doi: 10.1371/journal.pone.0048538
Vehmaa A., Hogfors H., Gorokhova E., Brutemark A., Holmborn T., Engström-Öst J. (2013). Projected marine climate change: effects on copepod oxidative status and reproduction. Ecol. Evol. 3, 4548–4557. doi: 10.1002/ece3.839
Venkataraman Y. R., Downey-Wall A. M., Ries J., Westfield I., White S. J., Roberts S. B., et al. (2020). General DNA methylation patterns and environmentally-induced differential methylation in the eastern oyster (Crassostrea virginica). Front. Mar. Sci. 225 doi: 10.3389/fmars.2020.00225
Venkataraman Y. R., Spencer L. H., Roberts S. B. (2019). Larval response to parental low pH exposure in the pacific oyster Crassostrea gigas. J. Shellfish Res. 38, 743–750. doi: 10.2983/035.038.0325
Venkataraman Y. R., White S. J., Roberts S. B.. (2022). Differential DNA methylation in pacific oyster reproductive tissue in response to ocean acidification. BMC Genomics. 23 (1), 1–16. doi: 10.1186/s12864-022-08781-5
Verkaik K., Hamel J. F., Mercier A. (2016). Carry-over effects of ocean acidification in a cold-water lecithotrophic holothuroid. Mar. Ecol. Prog. Ser. 557, 189–206. doi: 10.3354/meps11868
Vihtakari M., Havenhand J., Renaud P. E., Hendriks I. E. (2016). Variable individual- and population- level responses to ocean acidification. Front. Mar. Sci. 3. doi: 10.3389/fmars.2016.00051
Vihtakari M., Hendriks I. E., Holding J., Renaud P. E., Duarte C. M., Havenhand J. N. (2013). Effects of ocean acidification and warming on sperm activity and early life stages of the Mediterranean mussel (Mytilus galloprovincialis). Water 5, 1890–1915. doi: 10.3390/w5041890
Wang Q., Cao R., Ning X., You L., Mu C., Wang C., et al. (2016). Effects of ocean acidification on immune responses of the pacific oyster Crassostrea gigas. Fish Shellfish Immunol. 49, 24–33. doi: 10.1016/j.fsi.2015.12.025
Wang T., Kong H., Shang Y., Dupont S., Peng J., Wang X., et al. (2021). Ocean acidification but not hypoxia alters the gonad performance in the thick shell mussel Mytilus coruscus. Mar. pollut. Bull. 167, 112282. doi: 10.1016/j.marpolbul.2021.112282
Wang X., Shang Y., Kong H., Hu M., Yang J., Deng Y., et al. (2020). Combined effects of ocean acidification and hypoxia on the early development of the thick shell mussel Mytilus coruscus. Helgol. Mar. Res. 74, 1–9. doi: 10.1186/s10152-020-0535-9
Washington Sea Grant (2015) Shellfish aquaculture in Washington state. final report to the Washington state legislature. Available at: https://pdfs.semanticscholar.org/b833/e0fcb8a0459697f94fd86b9848ee0e59c0a2.pdf.
Wasson K., Gossard D. J., Gardner L., Hain P. R., Zabin C. J., Fork S., et al. (2020). A scientific framework for conservation aquaculture: A case study of oyster restoration in central California. Biol. Conserv. 250, 108745. doi: 10.1016/j.biocon.2020.108745
Weydmann A., Søreide J. E., Kwasniewski S., Widdicombe S. (2012). Influence of CO2-induced acidification on the reproduction of a key Arctic copepod Calanus glacialis. J. Exp. Mar. Bio. Ecol. 428, 39–42. doi: 10.1016/j.jembe.2012.06.002
White M. M., Mullineaux L. S., McCorkle D. C., Cohen A. L. (2014). Elevated pCO2 exposure during fertilization of the bay scallop Argopecten irradians reduces larval survival but not subsequent shell size. Mar. Ecol. Prog. Ser. 498, 173–186. doi: 10.3354/meps10621
Wippel B. J. T. (2017). Potential transgenerational effects of ocean acidification and hypoxia on the Olympia oyster ostrea lurida: A three-part experimental study (Seattle (WA: University of Washington). Available at: http://hdl.handle.net/1773/40227
Wong J. M., Kozal L. C., Leach T. S., Hoshijima U., Hofmann G. E. (2019). Transgenerational effects in an ecological context: Conditioning of adult sea urchins to upwelling conditions alters maternal provisioning and progeny phenotype. J. Exp. Mar. Bio. Ecol. 517, 65–77. doi: 10.1016/j.jembe.2019.04.006
Wood H. L., Spicer J. I., Widdicombe S. (2008). Ocean acidification may increase calcification rates, but at a cost. Proc. Biol. Sci. 275, 1767–1773. doi: 10.1098/rspb.2008.0343
Xu X., Yang F., Zhao L., Yan X. (2016). ). seawater acidification affects the physiological energetics and spawning capacity of the Manila clam Ruditapes philippinarum during gonadal maturation. Comp. Biochem. Physiol. A Mol. Integr. Physiol. 196, 20–29. doi: 10.1016/j.cbpa.2016.02.014
Yue C., Li Q., Yu H. (2018). Gonad transcriptome analysis of the pacific oyster Crassostrea gigas identifies potential genes regulating the sex determination and differentiation process. Mar. Biotechnol. 20, 206–219. doi: 10.1007/s10126-018-9798-4
Yusa Y. (2007). Causes of variation in sex ratio and modes of sex determination in the Mollusca–an overview*. Am. Malacol. Bull. 23, 89–98. doi: 10.4003/0740-2783-23.1.89
Zervoudaki S., Frangoulis C., Giannoudi L., Krasakopoulou E. (2013). Effects of low pH and raised temperature on egg production, hatching and metabolic rates of a Mediterranean copepod species (Acartia clausi) under oligotrophic conditions. Mediterr. Mar. Sci. 15, 74. doi: 10.12681/mms.553
Zervoudaki S., Krasakopoulou E., Moutsopoulos T., Protopapa M., Marro S., Gazeau F. (2017). Copepod response to ocean acidification in a low nutrient-low chlorophyll environment in the NW Mediterranean Sea. Estuar. Coast. Shelf Sci. 186, 152–162. doi: 10.1016/j.ecss.2016.06.030
Zhang D., Li S., Wang G., Guo D. (2011). Impacts of CO2-driven seawater acidification on survival, egg production rate and hatching success of four marine copepods. Acta Oceanologica Sin. 30, 86–94. doi: 10.1007/s13131-011-0165-9
Zhan Y., Hu W., Duan L., Liu M., Zhang W., Chang Y., et al. (2017). Effects of seawater acidification on early development of the sea urchin Hemicentrotus pulcherrimus. Aquac. Int. 25, 655–678. doi: 10.1007/s10499-016-0064-3
Zhan Y., Hu W., Duan L., Liu M., Zhang W., Chang Y., et al. (2018). Effects of seawater acidification on the early development of sea urchin Glyptocidaris crenularis. J. Oceanology Limnology 36, 1442–1454. doi: 10.1007/s00343-018-6317-4
Zhan Y., Hu W., Zhang W., Liu M., Duan L., Huang X., et al. (2016). The impact of CO2-driven ocean acidification on early development and calcification in the sea urchin Strongylocentrotus intermedius. Mar. pollut. Bull. 112, 291–302. doi: 10.1016/j.marpolbul.2016.08.003
Keywords: sperm, egg, fertilization, gametogenesis, fecundity, brooding, spawning, climate change
Citation: Padilla-Gamiño JL, Alma L, Spencer LH, Venkataraman YR and Wessler L (2022) Ocean acidification does not overlook sex: Review of understudied effects and implications of low pH on marine invertebrate sexual reproduction. Front. Mar. Sci. 9:977754. doi: 10.3389/fmars.2022.977754
Received: 24 June 2022; Accepted: 28 September 2022;
Published: 28 October 2022.
Edited by:
Hongsheng Yang, Institute of Oceanology (CAS), ChinaReviewed by:
Gael John Lecellier, Université de Versailles Saint-Quentin-en-Yvelines, FranceAlessandra Gallo, Stazione Zoologica Anton Dohrn Napoli, Italy
Copyright © 2022 Padilla-Gamiño, Alma, Spencer, Venkataraman and Wessler. This is an open-access article distributed under the terms of the Creative Commons Attribution License (CC BY). The use, distribution or reproduction in other forums is permitted, provided the original author(s) and the copyright owner(s) are credited and that the original publication in this journal is cited, in accordance with accepted academic practice. No use, distribution or reproduction is permitted which does not comply with these terms.
*Correspondence: Jacqueline L. Padilla-Gamiño, anBnYW1pbm9AdXcuZWR1
†These authors have contributed equally to this work