- School of Biology and Environmental Science, University College Dublin, Dublin, Ireland
Wetland ecosystems, particularly coastal vegetated ecosystems, play a vital role in climate mitigation and adaptation. Coastal vegetated habitats (including coastal wetlands such as seagrass meadows, saltmarshes, and mangroves) are some of the most efficient ecosystems for storing carbon on a per hectare basis, retaining organic carbon for centuries to millennia. We conducted carbon stock assessments, to a depth of 1 m, across four young saltmarsh sites and the adjacent mudflats in Dublin, Ireland. Soil cores and vegetation samples were taken across each site in order to analyze the carbon content of each carbon pool. The carbon density of Dublin’s saltmarshes was 112.1 ± 10.1 Mg Corg ha−1. The dominant plant species were Spartina anglica, Plantago maritima, and Atriplex portulacoides. The soil carbon pool of these saltmarshes contributes 87% to the total carbon stock, with living plant biomass contributing the remaining 13%. Saltmarshes are important ecosystems for climate mitigation and can provide nature-based solutions to reduce net carbon emissions; however, their degradation will lead to the loss of climate-relevant carbon pools.
1. Introduction
Wetland ecosystems, particularly coastal vegetated ecosystems, play a vital role in climate mitigation and adaptation (Kirwan and Megonigal, 2013; Were et al., 2019). Coastal vegetated habitats (including coastal wetlands such as seagrass meadows, saltmarshes, and mangroves) are some of the most efficient ecosystems for storing carbon on a per hectare basis, retaining organic carbon for centuries to millennia (Chmura et al., 2003; Duarte et al., 2005; Fourqurean et al., 2012; Ellison and Beasy, 2018). The carbon stored in these ecosystems has been termed “blue carbon” (Nelleman et al., 2008; Windham-Myers et al., 2018). In addition to carbon storage, coastal vegetated habitats, also known as blue carbon ecosystems (BCEs), provide a lot of co-benefits. They are valued for their ability to mitigate the effects of sea-level rise and their ability to protect the shoreline from erosion and storm surge (Gedan et al., 2011; Shepard et al., 2011; Möller et al., 2014; Giuliani and Bellucci, 2019; Unsworth and Butterworth, 2021). BCEs serve as a nursery ground for nekton and avian species (Beck et al., 2001; Minello et al., 2003; Spencer et al., 2009; Minello et al., 2012) and play a key role in nutrient cycling (Saunders and Kalff, 2001; Mcleod et al., 2011). The many ecological services of BCEs contribute to the protection, maintenance, and restoration of these unique ecosystems. These ecosystems can provide nature-based solutions to reducing net carbon emissions and aid in climate mitigation (Macreadie et al., 2021; Macreadie et al., 2022). Furthermore, their role in the global carbon cycle has enabled their protection and restoration in carbon crediting programs and climate change policy frameworks (Herr et al., 2012).
Coastal wetlands store as much as 50% of the carbon found in ocean sediments, although covering less than 0.5% of the seabed (Nelleman et al., 2008). They sequester carbon through the photosynthesis of vegetation and store it in the biomass of plants and sediments (Howard et al., 2014). Dead plant tissue is degraded or exported from BCEs; however, some amount contributes to sediment accumulation (Middelburg et al., 1997; Duarte et al., 2005). In addition to this, the vegetation of coastal wetlands has dense shoot systems and complex root and rhizome structures that are efficient at trapping and stabilizing suspended particles and organic matter in tidal waters, therefore aiding in the accumulation of carbon (Leonard and Luther, 1995; Duarte et al., 2005; Wang et al., 2021). Carbon decomposition is slowed due to the anoxic conditions of the environment, resulting in the long-term storage and preservation of carbon (Lo Iacono et al., 2008). The lack of oxygen in the sediment—due to the waterlogged conditions of all BCEs and the impermeable clay sediment found in many saltmarshes and mangroves—suppresses the microbial oxidation of carbon. The inability of microbes to break down organic carbon and the continuous accumulation of sediments result in high carbon stocks (Ouyang and Lee, 2013). When coastal wetlands are degraded (e.g., drained of water), the carbon stored in the soil is consumed by microorganisms. These microorganisms respire and release CO2 as a metabolic waste product. The anthropogenic activity that degrades BCEs or changes the land use (e.g., impoundment and agriculture) results in a reduction in the uptake of CO2 due to the loss of vegetation and the release of greenhouse gases (Howard et al., 2017). Given that these ecosystems contain substantial amounts of carbon, their degradation would greatly impact global CO2 emissions; thus, they can be considered as climate-relevant carbon pools.
Studies worldwide have conducted assessments to quantify the carbon stocks of BCEs (Chmura et al., 2003; Ouyang and Lee, 2014). These studies assessed the carbon accumulation rates (CARs) and volumetric soil carbon density (SCD) (in grams per cubic meter) in global saltmarshes. Of the sites included in these studies, only 20% were in Europe, with no information regarding Irish saltmarshes. Saltmarshes have significant data gaps for carbon stocks and accumulation rates, with most of the studies so far conducted in Australia and the United States (Ellison and Beasy, 2018). Improving the global estimates of the carbon stocks and sequestration rates in saltmarshes will allow a further understanding of the potential of and the degree to which these BCEs can mitigate climate change.
Saltmarshes are located along the entire coastline of Ireland comprising approximately 10,000 ha over at least 250 sites (Curtis and Sheehy Skeffington, 1998; Mcowen et al., 2017). These saltmarshes are documented and classified according to their location and substrate types (Curtis and Sheehy Skeffington, 1998). These include estuarine, bay, sandflats, lagoons, and fringe saltmarshes. The focus of this study is County Dublin, where areas of saltmarsh habitat have developed over the last 200 years within estuaries (Farrington and Haughton, 1947; McCorry, 2007). These sites are classified as estuarine saltmarshes and have fine sediment. The clay and silt sediments of the estuarine and bay saltmarshes and the peat substrate of the fringe saltmarshes are expected to have high quantities of organic carbon, in contrast to the sandy soils found in sandflats (Yang et al., 2016; Cott et al., 2021). The carbon concentrations and a preliminary carbon stock have been determined for Irish saltmarshes (i.e., 57.75 Mg Corg ha−1) using soil samples to a depth of 10 cm from sites across the east and south coasts of Ireland (Penk, 2019; Penk and Perrin, 2021). However, the complete carbon stock, to a 1m depth, and the variability between the substrate types of Ireland’s saltmarshes are yet to be determined.
The aim of this study was to quantify the carbon stock of the saltmarshes of Dublin and to establish whether they contain climate-relevant pools of carbon. Determining the soil carbon stock of the aforementioned saltmarsh types in Ireland will contribute to our understanding of the carbon storage potential of these ecosystems. We compared the carbon density and storage capacity to other saltmarshes of similar geographical location and to other ecosystems within Ireland.
2. Methods
2.1. Study sites
We conducted carbon stock assessments across four sites and the adjacent mudflats in Dublin: North Bull Island (NBI; 53.37078° N, 6.15014° W), Baldoyle Estuary (BE; 53.41857° N, 6.12991° W), Malahide Estuary (ME; 53.46837° N, 6.13269° W), and Rogerstown Estuary (RE; 53.50331° N, 6.17048° W) (Figure 1). These saltmarshes cover a total area of 267.51 ha: of which 120.25 ha cover NBI [divided into North Lagoon (NL NBI, 41.91 ha) and South Lagoon (SL NBI, 78.34 ha)], while the remaining sites occupy 54.13, 39.85, and 53.28 ha, respectively. The growth of each saltmarsh in Dublin has notably increased over the past 200 years and can be observed through aerial imagery and historic maps (OSi, 2021). NBI has developed over the past 200 years since 1820–1823, after the building of the North Bull wall in Dublin port (Farrington and Haughton, 1947). In addition to this, the growth of the seaward side of ME saltmarsh accelerated after the construction of the Dublin to Drogheda train line in the late 1800s, although much of the saltmarsh habitat at the mouth of the estuary has been affected by the development of the motorway bridge (McCorry, 2007). BE, although present on historic maps dating to the early 1800s, has seen recent growth (past 100 years) due to the spread of Spartina anglica and its colonization of the surrounding mudflat habitats (McCorry, 2007). The swards of S. anglica have led to an additional 38 ha of the vegetated ecosystem—70% of Baldoyle’s current saltmarsh area (McCorry, 2007). Some areas of RE are possibly the oldest of the four studied saltmarshes, with sections being used for grazing and others previously as agricultural land. S. anglica swards have also developed in RE within the past 100 years (McCorry, 2007); however, there is no definite timescale for this.
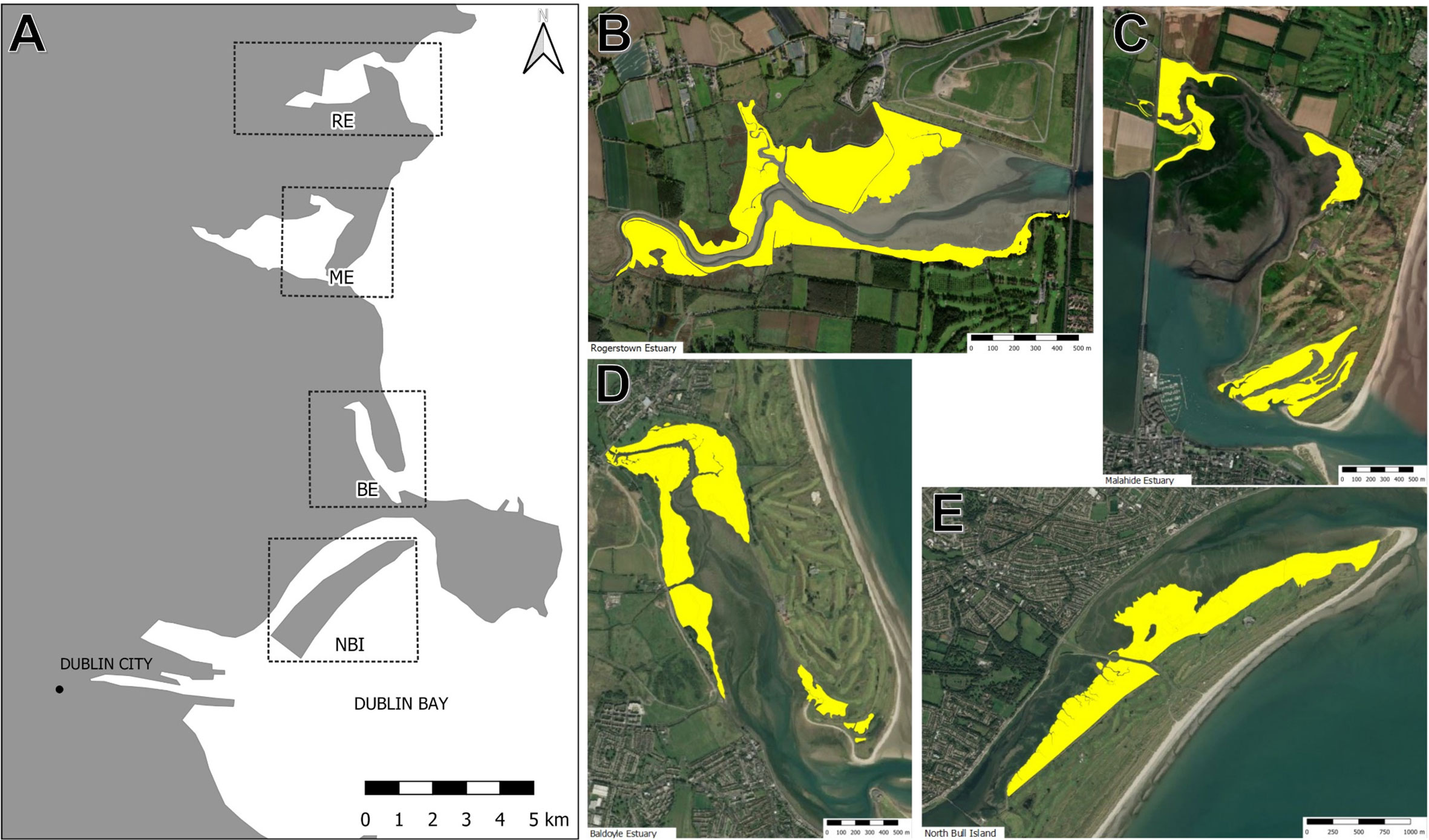
Figure 1 The location of each studied site in Dublin City and County (A) with the saltmarsh area highlighted in yellow; where (B) is Rogerstown Estuary (RE), (C) is Malahide Estuary (ME), (D) is Baldoyle Estuary (BE), and (E) is North Bull Island (NBI). Background maps: Bing satellite map. Shapefiles adapted from npws.ie/maps-and-data.
Three different habitat types are found within these areas, classified by the EU Habitats Directive as Salicornia mudflats, Atlantic salt meadows, and Mediterranean salt meadows. These habitats are differentiated based on their dominant vegetation species: Salicornia spp., Puccinellia maritima, and Juncus maritimus and/or Juncus acutus. Areas of the invasive S. anglica species are also included in the overall area of saltmarshes as they are abundant in the Atlantic salt meadow habitats. The most prominent saltmarsh habitat among the NBI, RE, and ME sites is Atlantic salt meadow, whereas approximately 70% of the BE site is Spartina swards.
The climate of Dublin is temperate, without a dry season, and with a warm summer. The mean daily minimum and maximum temperatures of Dublin are 6.4°C and 13.3°C, respectively, and the mean annual precipitation is 758 mm (1981–2010, Dublin Airport station, Met Éireann).
2.2. Field sampling
Soil and vegetation sampling for carbon analysis took place over 7 days during the period from September 2019 to November 2021. One set of samples (i.e., a soil core, a root biomass core, and a vegetation clip plot) was taken from between 8 and 12 plots in each saltmarsh and from four plots in each mudflat. Each plot was chosen primarily using satellite imagery and QGIS software (https://www.qgis.org/) and readjusted on site depending on the distribution of vegetation and the access on the saltmarsh. At each plot on the saltmarsh and on the mudflat, two soil cores and one soil core, respectively, were collected using a gouge or Russian peat auger of 6 cm diameter and up to 100 cm in depth; in cases where the auger did not reach 100 cm, the depth at refusal was recorded. Specific care was taken to avoid sediment compaction during the core insertion. No sediment compaction was observed during the sampling process. One soil core was used for the soil carbon analysis, while the other was used for belowground living biomass analysis. Belowground living biomass cores are not necessary for the mudflat plots as these were unvegetated. Each core was divided into at most five sections (i.e., 0–5, 5–15, 15–30, 30–50, and 50–100 cm) and further divided into subsamples of the center 5 cm of each section, which were retained for analysis. A maximum of five subsamples were taken from each core.
To analyze the aboveground living biomass, a quadrat (30 × 30 cm) of vegetation was removed from each plot. The shoots of living plant material were cut from the base of the plant as close to the sediment as possible without removing the roots or rhizomes. Any algae and dead plant litter found in between the vegetation were removed from the sample before calculation of the biomass.
Each sample was stored in airtight plastic bags while on site and later stored at 4°C until they could be further analyzed. Further laboratory analyses followed the protocols outlined by the Coastal Blue Carbon manual (Howard et al., 2014).
2.3. Lab analysis
2.3.1. Soil carbon analysis
The subsamples taken from the soil core were weighed before drying at 60°C for a period of 72 h until the mass of each sample was stable. The subsamples were re-weighed and the mass of the dried sample used to determine the dry bulk density of the soil. A subsample of the dried soils was processed to remove live roots, shells, or stones and ground using a mortar and pestle to a finer particle size. A 5-g portion of each ground subsample was ignited to combustion in a muffle furnace at 450°C for 4 h; this temperature was used to ensure that only organic carbon was oxidized as inorganic carbon may be lost at temperatures as low as 425°C (Heiri et al., 2001; Howard et al., 2014). This combustion method provided the percentage loss on ignition of organic matter (%LOI) (see Equation 1). All %LOI values were entered into Equation 2 to determine a conservative percentage of organic carbon (%Corg) (Craft et al., 1991).
When scaling these values to consider the entire saltmarsh, the average carbon density (CD) per hectare (CD per hectare, Mg Corg ha−1) was determined before multiplying by the size of the saltmarsh (in hectares) to calculate the total organic carbon content (Mg Corg). The avoided emissions for all carbon pools were calculated by multiplying the total carbon content by 3.67 (converting from C to CO2 molar mass). When uncertainty estimates are presented, these represent the standard error of all samples.
2.3.2. Belowground living biomass
The roots were removed from the belowground biomass soil cores by washing each subsample under water over a fine mesh sieve (2 mm). Living roots were differentiated from dead roots based on the appearance of the material: live roots were observed to be pale in color and were turgid, whereas dead roots were dark or gray in color and were flattened. After separation, the living roots were weighed before drying at 60°C for at least 24 h until the mass of each sample was stable. The biomass (dry weight) per core was determined and multiplied by a conversion factor of 0.34 to determine the organic carbon content (Mg Corg ha−1) of the belowground biomass component (Duarte, 1990; Howard et al., 2014). This was scaled up to the area of the saltmarsh using the method outlined above.
2.3.3. Aboveground living biomass
The vegetation samples were divided depending on the species type (woody shrubs or herbaceous plants), and any algae, dead plant litter, and non-plant material were removed before weighing and drying at 60°C for at least 24 h or until the mass of each sample was stable. The biomass was multiplied by carbon conversion factors of 0.45 for marsh grasses (including herbaceous plants) and 0.46 for woody shrubs to determine the carbon content of each vegetation type (Fang et al., 1996; Howard et al., 2014). This was scaled up to the area of the saltmarsh using the method outlined above, assuming that the variability and the dominance of vegetation species were adequately captured during plot selection.
3. Results
3.1. Aboveground living biomass carbon pool
The species distribution of each site was highly variable, although the most prominent habitat type was Atlantic salt meadows (Glauco-Puccinellietalia maritimae) (Doody, 2008). The dominant vegetation species was recorded at each plot and was found to consist of seven distinct species: S. anglica, Atriplex portulacoides, Armeria maritima, Phragmites australis, J. maritimus, Plantago maritima, and other Poaceae species (e.g., P. maritima, Festuca rubra, and Agrostis stolonifera, among others). The most common species sampled throughout the sites were S. anglica and P. maritima.
The total organic carbon (TOC) content of the aboveground carbon pool across all sites was 1,364.5 ± 141.67 Mg Corg. The aboveground living biomass carbon density per hectare (aboveground CD per hectare, Mg Corg ha−1) for each site and the total are shown in Figure 2A and Table 1. The highest mean aboveground CD per hectare was observed in BE, with almost twice the average across the sites in Dublin. The most frequently sampled vegetation species in BE were A. portulacoides, and S. anglica, which had mean aboveground CDs of 11.0 ± 0.7 and 7.2 ± 0.8 Mg Corg ha−1, respectively.
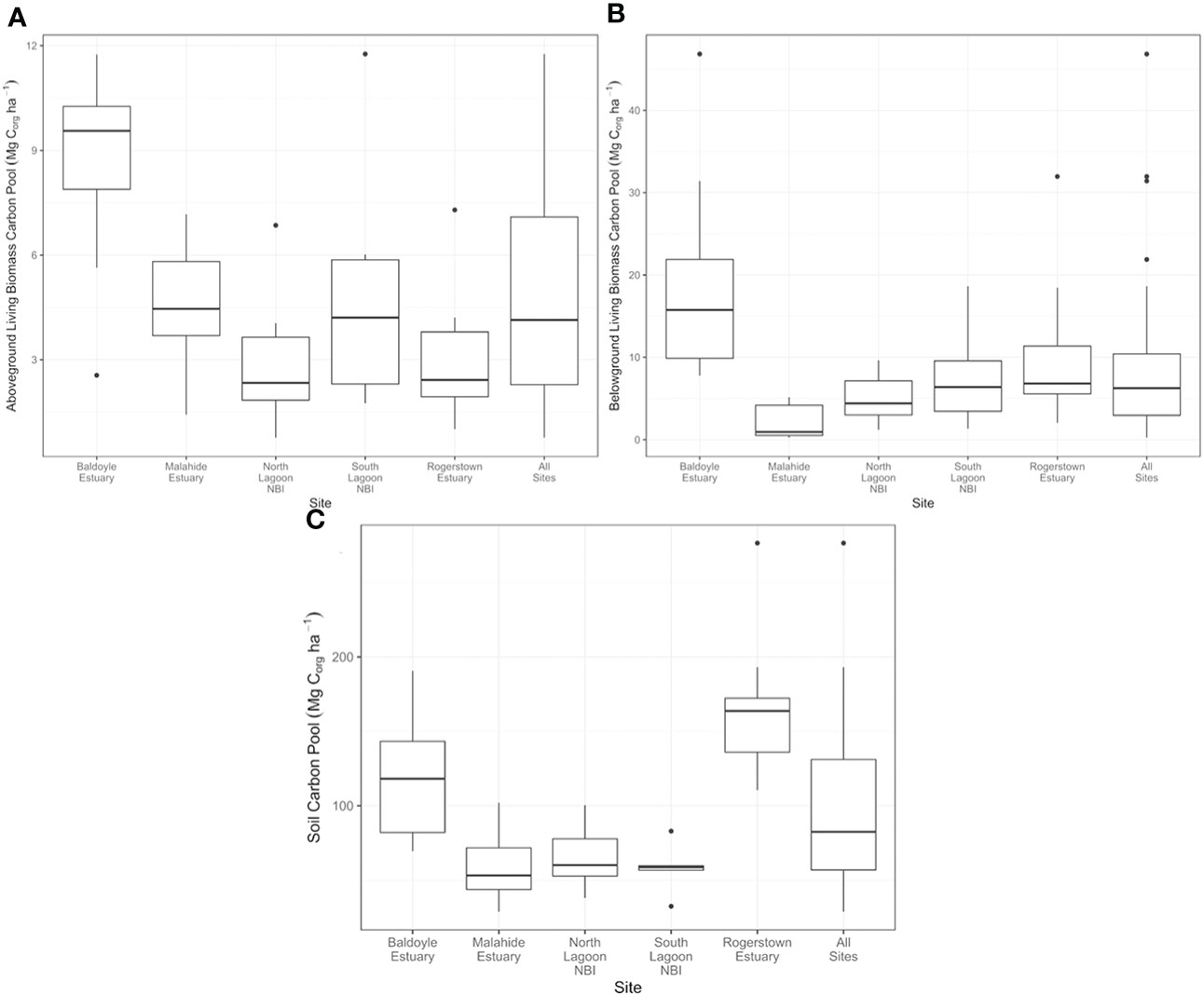
Figure 2 The carbon densities per hectare (Mg Corg ha−1) of each carbon pool and of each site (L-R: Baldoyle Estuary, Malahide Estuary,North Lagoon of North Bull Island, South Lagoon of North Bull Island, Rogerstown Estuary, and all sites combined) where (A) is the aboveground carbon pool, (B) is the belowground carbon pool, and (C) is the soil carbon pool.
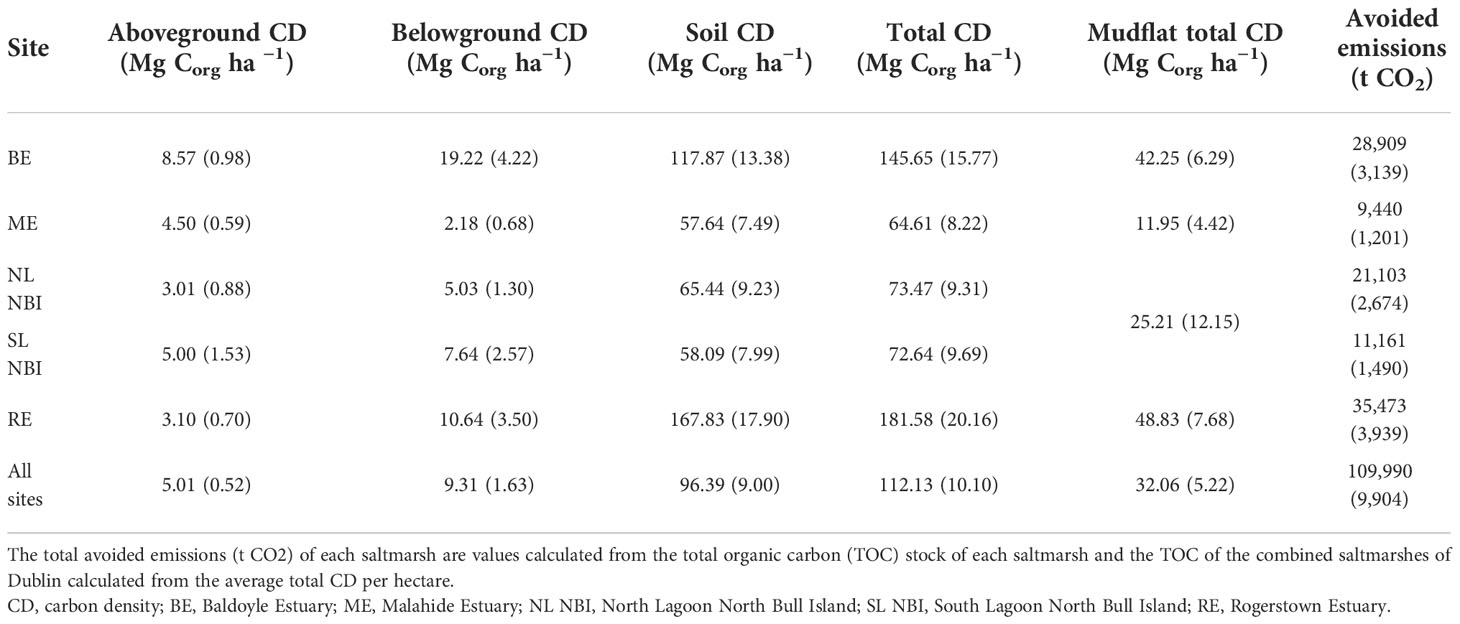
Table 1 Mean carbon density per hectare (Â ± standard error) for each carbon pool of each site and the average across all sites.
3.2. Belowground living biomass carbon pool
The TOC content of the belowground living biomass carbon pool across all sites was 2,547.6 ± 443.9 Mg Corg−1. The belowground biomass carbon density per hectare (belowground CD per hectare, Mg Corg ha−1) for each site and the total are shown in Figure 2B and Table 1. The highest mean belowground CD per hectare was observed in BE. The BE site was dominated by S. anglica, where thick and long (>2 mm) root systems were observed.
3.3. Soil carbon pool
The soil organic carbon (SOC) stock was 26,085.2 ± 2,442.6 Mg Corg. The soil CD per hectare for each site and the total are shown in Figure 2C and Table 1. The soil CD per hectare of the adjacent mudflats of each site were two- to fourfold lower than those of the corresponding saltmarshes. RE was found to have the highest mean SCD (volumetric), with a value of 0.03 ± 0.003 g cm−3, which was 50% greater than the Dublin average (0.02 ± 0.001 g cm−3). The soil CD per hectare (Mg Corg ha−1) was also highest in RE.
3.4. Total carbon stock
The TOC stock of all pools in the Dublin saltmarshes was estimated as 29,997.2 ± 2,701.1 Mg Corg−1. The total CD per hectare was found to be lowest in ME and highest in RE (Table 1). The largest carbon pool among the sites was in RE, which contributed to 20% of the saltmarsh area in Dublin and 32% of the blue carbon pool.
These data are available through the figshare repository (https://doi.org/10.6084/m9.figshare.20506713).
4. Discussion
Our results have shown that the young saltmarshes of Dublin contain climate-relevant pools of carbon. The soil carbon pool of these saltmarshes contributed 87% to the TOC, with living plant biomass contributing the remaining 13%. With the high abundance of saltmarshes along the coast of Ireland, there is the potential of climate-relevant carbon hotspots.
The estimated total CD of Dublin’s saltmarshes was 112.13 ± 10.10 Mg Corg ha−1, which was twice that of the carbon stock reported for Ireland’s south and east coast saltmarshes calculated up to a depth of 10 cm (Penk and Perrin, 2021). The aboveground carbon pools for the saltmarshes of Dublin (5.01 ± 0.5 Mg Corg ha−1), other Irish sites (4.98 ± 0.2 Mg Corg ha−1) (Penk and Perrin, 2021), and globally (4.3 ± 0.10 Mg Corg ha−1) (Alongi, 2020) were all similar to each other despite differences in the species composition of each site.
The belowground living biomass carbon pool was 9.3 ± 1.6 Mg Corg ha−1, which contributed to<1% of the total CD per hectare. The global average value of the belowground biomass carbon content was 12.9 ± 1.2 Mg Corg ha−1, which was similar to the combined saltmarshes of Dublin (Alongi, 2020). The method we followed for the calculation of the belowground biomass included only live roots, while dead roots were included in the calculation of soil carbon stock. The method used differs among studies, including those mentioned in the saltmarsh global synthesis by Chmura et al. (2003) and in the study by Penk and Perrin (2021).
The difference in the belowground biomass carbon pools may also be due to the differing species composition of each saltmarsh. The species compositions of the saltmarshes in Dublin are not identical and show great variability between sites; the belowground living biomass ranged from 0.007 to 0.55 g cm−2. Species such as S. anglica were observed to have thicker roots and less dense root systems (0.085 ± 0.010 g cm−2), whereas the upper marsh areas consisted of a mosaic of other species (other Poaceae species, P. maritima, A. maritima, and J. maritimus) with finer roots and a denser root system (0.114 ± 0.017 g cm−2). P. australis, which has high above and belowground biomass (Moore et al., 2012), was sampled in BE, but not at other sites, thus affecting both the above- and belowground biomass.
Although CHN (carbon, hydrogen, and nitrogen) analysis of the sampled biomass may have provided more accurate estimates of the aboveground and belowground CDs per hectare, this was not conducted due to limited resources. Following the guidelines of Howard et al. (2014), the carbon conversion factors used were 0.45 for the aboveground herbaceous saltmarsh species, 0.46 for the aboveground woody species, and 0.34 for belowground roots. To assess the variations in the results from the use of different conversion factors, the carbon content percentages were extracted from four studies (Cartaxana and Catarino, 1997; Sutter et al., 2014; Ma et al., 2018; Penk and Perrin, 2022). The above- and belowground CDs were reanalyzed to assess for significant differences between the carbon contents. We multiplied the previously determined vegetation biomass by the different carbon conversion factors from each of the aforementioned studies to recalculate the above- and belowground carbon pools. We performed Mann–Whitney–Wilcoxon tests with Bonferroni-adjusted alphas [aboveground CD: α = 0.01 (0.05–5); belowground CD: α = 0.0167 (0.05–3)] using RStudio (version 2022.07.1554) with R (version 4.1.2) (R Core Team, 2021), which directly compared each study to the coastal conversion factors (Howard et al., 2014). No statistically significant differences were found between values.
The soil CDs per hectare per hectare differed greatly between this study and the average for Ireland’s south and east coast saltmarshes (Penk and Perrin, 2021). The significance of soil depth when calculating ecosystem carbon stocks was highlighted by this difference. The soil CDs per hectare for the southern and eastern Irish saltmarshes was 38.54 ± 0.76 Mg Corg ha−1 (Penk and Perrin, 2021), which was less than half of that determined through this study. Similarly, in Great Britain, the saltmarshes were shown to have an soil CDs per hectare of 51.4 ± 10.4 Mg Corg ha−1 in the top 10 cm (Smeaton et al., 2022). The 10cm sampling depth used in these studies omitted a large proportion of the C stock and potential CO2 emissions. Using the soil CDs per hectare value for Ireland, i.e., 38.54 ± 0.76 Mg Corg ha−1, the SOC stock would be 10,309.8 ± 203.3 Mg Corg or 37,803 ± 745 tonnes of CO2, a difference of almost 20,000 Mg Corg or over 72,000 tonnes of CO2 from our estimate. In studies where carbon stock to a depth of 1 m is presented and calculated by extrapolation, i.e., assuming the carbon concentration does not decrease with depth, the calculation could lead to overestimating the carbon stock, as, from our study, we saw a rapid decline in carbon concentration after 20 cm (Figure 3). This shows the importance of sampling to at least 1 m or to the depth at refusal in order to produce a comprehensive carbon stock assessment (Howard et al., 2014). Additional sampling is currently being conducted across Ireland at sites with a broader geomorphic range and sediment composition.
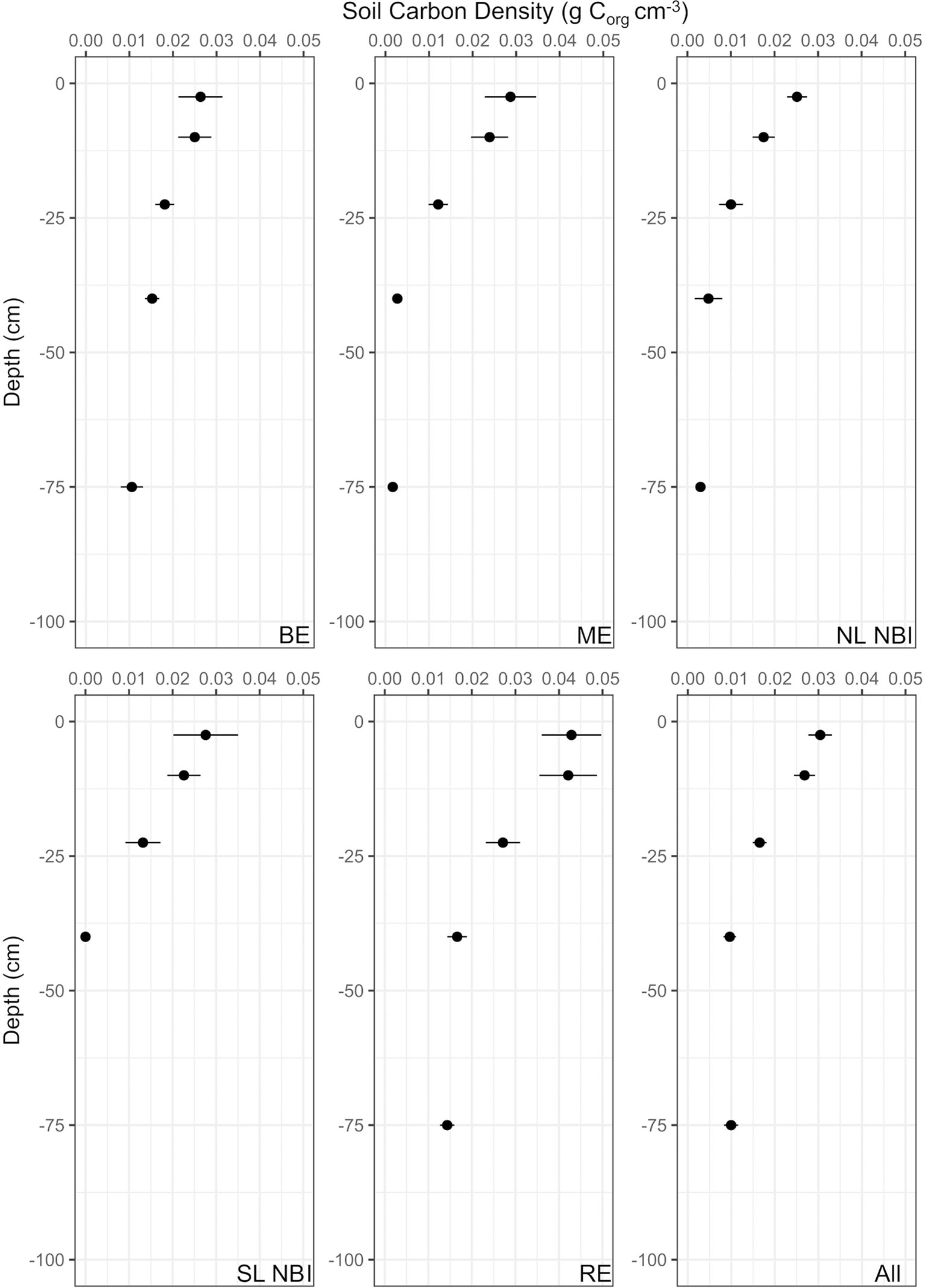
Figure 3 The soil carbon density (g Corg cm−3) at the midpoint of each sampling depth (0-5 cm, 5-15 cm, 15-30 cm, 30-50 cm, and 50-100 cm) of each Dublin site and the average of all sites (mean ± standard error).Top L-R: Baldoyle Estuary (BE), Malahide Estuary (ME), North Lagoon of North Bull Island (NL NBI); Bottom L-R: South Lagoon of NBI (SL NBI), Rogerstown Estuary (RE), and All Sites (All).
The CD per hectare estimates can be influenced by the age and the CAR of the saltmarsh, i.e., the older the saltmarsh, the longer the sediment has to accumulate. Most of the saltmarsh areas in this study can be seen, through historic maps and aerial imagery, to have developed mainly over the last 200 years. As NBI developed through anthropogenic means, we know that the accumulation of sediments began around the time the North Bull wall was built, approximately 200 years ago (Farrington and Haughton, 1947). The SOC of NL NBI was 5,125.81 ± 723.21 Mg Corg, which would show an average soil CAR of 32.71 ± 4.62 g Corg m−2 yr−1, and that of SL NBI was 2,434.20 ± 334.91 Mg Corg, with an average CAR of 29 ± 4.0 g Corg m−2 yr−1. Based on the construction of the Dublin–Drogheda train line, ME would have an average soil CAR of 38.43 ± 5.0 g Corg m−2 yr−1 over the past 150 years, using the SOC of 2,296.88 ± 298.57 Mg Corg determined in this study. The global CAR of saltmarshes was averaged at 244.7 g Corg m−2 yr−1 (Ouyang and Lee, 2014), which is considerably higher than what we observed in NBI and ME. This global accumulation rate ranged from 18 to 1,713 g C m−2 yr−1 (Chmura et al., 2003), an almost 100-fold difference, and conveyed the extensive variability among saltmarshes. There are several factors that can impact the accumulation rate in addition to the age of the saltmarsh, including vegetation type, tidal range, and carbon inputs (Arriola and Cable, 2017). The age of the saltmarshes, as well as the rate at which sediments accumulate, was not addressed in this study or in the other global syntheses of saltmarsh carbon stores by Alongi (2020) and Chmura et al. (2003). The CAR, if similar throughout all sites, could partially explain the sizeable difference in the CD per hectare values between the Dublin average and the global average (334.4 ± 3.5 Mg C ha−1) (Alongi, 2020).
The age of saltmarshes also considerably impacts the SCD per hectare of saltmarshes and other BCEs (Dontis et al., 2020). In a study by Ruiz-Fernández et al. (2018), the soil CDs per hectare were 117.8 and 144.7 Mg Corg ha−1, up to a depth of 40 cm, for saltmarshes that were 127 ± 5 and 125 ± 7 years old, respectively (Ruiz-Fernández et al., 2016). These values were closer to those found among the saltmarshes in Dublin, where the saltmarshes were estimated to be 200 years old. In contrast, a different study by Drexler (2011) determined the soil CDs per hectare of sites that were 6,000 years old to be between 1,550 and 3,780 Mg Corg ha−1. The values for these sites were more than 10-fold those found in Dublin saltmarshes. The depths of these sites were also strikingly different, as the average depth sampled in our study was 58 cm, while it was 48 cm in Ruiz-Fernández et al. (2018) and was 670 cm in Drexler (2011), a difference of over 600 cm. Although age can be seen to have a noticeable impact, the sediment type and the particle size can still greatly affect the carbon storage capacity.
The sediment particle size has been shown to be a major predictor of carbon storage in saltmarshes (Dahl et al., 2016; Kelleway et al., 2016). The below average SCD per hectare in Dublin’s saltmarshes may also be influenced by the sediment properties observed in each saltmarsh. In a study of NBI’s substrates by Grey et al. (2021), the sediments (up to a depth of 10 cm) comprised 49.35% silt, 33.95% sand, and 16.01% clay. Fine sediments, such as silt and clay, contain higher concentrations of carbon than coarse, sandy sediments (Kelleway et al., 2016). As shown in NBI, the high percentage of fine sediments in the top 10 cm would explain the higher %Corg compared to the lower %Corg found at lower depths, where our samples mainly consisted of sand. The particle size distribution of a whole core would have to be analyzed for each site to further understand how the sediment makeup impacts the distribution of carbon throughout the depth of the soil profile. The difference in particle size distribution could also explain the large variability in the soil CDs per hectare value for each site and the range of values observed (28.73–276.45 Mg Corg ha−1).
The volumetric SCD averaged across sites (0.020 ± 0.001 g cm−3) was substantially lower than the mean SCD of global saltmarshes (0.039 ± 0.003 g cm−3) (Chmura et al., 2003) and the SCD of the southern and eastern saltmarshes in Ireland (0.039 ± 0.0008 g cm−3) (Penk and Perrin, 2021). The SCD in our study was calculated using the whole core (100 cm) or the depth at refusal, whereas in the global synthesis by Chmura et al. (2003), the depths at which the SCD was calculated varied with each study. Most of the global density data, where depth was accounted for, were taken from the top 30 cm (Chmura et al., 2003). Additionally, the carbon stocks of Ireland’s southern and eastern saltmarshes were based on a depth of 10 cm (Penk and Perrin, 2021). If the surface-level SCD (0–15 cm) were used in this study, the mean SCD across Dublin’s saltmarshes (0.029 ± 0.001 g cm−3) would be closer to that of the global average for saltmarshes.
The mudflat habitats in Dublin were shown to store three times less carbon than the saltmarshes, thus exhibiting the effect vegetated ecosystems have on the carbon accumulation in these areas. Comparably, at a site where mudflats are found adjacent to mangroves, the soil CDs per hectare of the mudflats were also three times less than those of the interior mangrove (Sasmito et al., 2020). These differences were due to the carbon inputs of the vegetated and non-vegetated habitats. Saltmarshes and mangroves have a substantial input of autochthonous carbon, whereas mudflats are more reliant on allochthonous carbon inputs.
Seagrass meadows, another BCE, had similar TOC stock estimates to those of the saltmarshes of Dublin (Fourqurean et al., 2012). Seagrass meadows were estimated to store between 9.1 and 628.1 Mg Corg ha−1, with a median value of 139.7 Mg Corg ha−1 (Fourqurean et al., 2012). A study by Arias-Ortiz et al. (2018) calculated the SCD of seagrass meadows to be 128 ± 7 Mg C ha−1, with a range of 23–322 Mg C ha−1. The range of values observed among seagrass meadows was similar to that found among Dublin’s saltmarshes (28.7–276.5 Mg Corg ha−1). The extensive variation among the seagrass meadows and saltmarshes of Dublin showed the extent of the effect to which factors such as sediment properties can have on the carbon storage of each ecosystem.
Within Ireland, carbon stock estimations have been made for various habitats, including other wetlands and peatlands (Cruickshank et al., 1998; Eaton et al., 2008). The CD was highest in peatland ecosystems, with a range from 705 ± 420 to 1,160 ± 520 Mg Corg ha−1 (Wellock et al., 2011). Other wetland ecosystems were estimated have 320 Mg Corg ha−1; however, this value was not based on actual wetland data, but on the assumption of other semi-natural habitats (Eaton et al., 2008). These values were three times those found for Dublin’s saltmarshes, and using these would significantly overestimate the CDs of other coastal wetlands. This further highlighted the need for regional studies on wetland ecosystems around Ireland.
The TOC stock of Dublin’s saltmarshes was equivalent to almost 110,000 tonnes of CO2 (Table 1). With complete degradation of these habitats and the subsequent release of CO2 into the atmosphere, the management, protection, and restoration of these saltmarshes are essential. Managing and restoring BCEs will allow for continued carbon sequestration, thus aiding in the mitigation of CO2 emissions and ultimately providing a climate benefit.
5. Conclusion
This study highlights the importance of Dublin’s saltmarsh carbon stock and contributes to our understanding of the blue carbon potential of Ireland. We found that the aboveground and belowground living biomass carbon stocks were similar to those found globally in saltmarshes; however, the SCD was substantially lower due to several aforementioned factors. We estimated the TOC stock (112.13 ± 10.10 Mg Corg ha−1) to be twice the preliminary estimated stock of Ireland (57.75 Mg Corg ha−1) (Penk, 2019). However, this estimate by Penk (2019) measured carbon to a depth of 10 cm and therefore vastly underestimated the contribution Ireland makes to global blue carbon stocks.
In 2013, the Intergovernmental Panel on Climate Change (IPCC) released a supplement to the guidelines for national greenhouse gas inventories focusing on wetlands, including coastal wetlands, where the default tier 1 estimate for tidal marsh soil carbon stock (up to 1 m) is 255 Mg C ha−1 and ranges from 15.6 to 623 Mg C ha−1 (Hiraishi et al., 2014). This emphasizes the need for further exploration into local and country-specific saltmarsh carbon stocks, as we have shown that Dublin’s saltmarshes have values within the range of the IPCC guidelines, but contain half that of the IPCC’s mean estimate. Dublin has 267 ha of saltmarshes that contain an average total carbon stock of almost 30,000 Mg Corg. The degradation of these habitats would lead to the emission of 110,000 tonnes of CO2 to the atmosphere. This clearly indicates the importance of the conservation of these climate-relevant carbon pools.
Data availability statement
The original contributions presented in the study are included in the article/supplementary material, further inquiries can be directed to the corresponding author.
Author contributions
GC obtained the funding for the project. SB, GC, and EE were involved in the conceptualization of the study. SB collected data, performed laboratory analysis, and wrote the manuscript—original draft. JM collected data and performed laboratory analysis. EE and GC contributed to writing the manuscript—review and editing. All authors contributed to the article and approved the submitted version.
Funding
This research was gratefully supported by Science Foundation Ireland (grant no. 18/SIRG/5614) and the School of Biology and Environmental Science, University College Dublin.
Acknowledgments
The authors would like to thank Sadhbh McCarrick, Martina Caplice, Oscar Flynn, and Lisa Jessen for their assistance during data collection.
Conflict of interest
The authors declare that the research was conducted in the absence of any commercial or financial relationships that could be construed as a potential conflict of interest.
Publisher’s note
All claims expressed in this article are solely those of the authors and do not necessarily represent those of their affiliated organizations, or those of the publisher, the editors and the reviewers. Any product that may be evaluated in this article, or claim that may be made by its manufacturer, is not guaranteed or endorsed by the publisher.
References
Alongi D. M. (2020). Carbon balance in salt marsh and mangrove ecosystems: A global synthesis. J. Mar. Sci. Eng. 8, 767. doi: 10.3390/jmse8100767
Arias-Ortiz A., Serrano O., Masqué P., Lavery P. S., Mueller U., Kendrick G. A., et al. (2018). A marine heatwave drives massive losses from the world’s largest seagrass carbon stocks. Nat. Climate Change 8, 338–344. doi: 10.1038/s41558-018-0096-y
Arriola J. M., Cable J. E. (2017). Variations in carbon burial and sediment accretion along a tidal creek in a florida salt marsh. Limnology Oceanography 62, S15–S28. doi: 10.1002/lno.10652
Beck M. W., Heck K. L., Able K. W., Childers D. L., Eggleston D. B., Gillanders B. M., et al. (2001). The identification, conservation, and management of estuarine and marine nurseries for fish and invertebrates: a better understanding of the habitats that serve as nurseries for marine species and the factors that create site-specific variability in nursery quality will improve conservation and management of these areas. Bioscience 51, 633–641. doi: 10.1641/0006-3568(2001)051[0633:TICAMO]2.0.CO;2
Cartaxana P., Catarino F. (1997). Allocation of nitrogen and carbon in an estuarine salt marsh in portugal. J. Coast. Conserv. 3, 27–34. doi: 10.1007/BF02908176
Chmura G. L., Anisfeld S. C., Cahoon D. R., Lynch J. C. (2003). Global carbon sequestration in tidal, saline wetland soils. Global biogeochemical cycles 17, 4. doi: 10.1029/2002GB001917
Cott G., Beca-Carretero P., Stengel D. (2021). Blue carbon and marine carbon sequestration in Irish waters and coastal habitats. Tech. Rep. Marine Institute.
Craft C., Seneca E., Broome S. (1991). Loss on ignition and kjeldahl digestion for estimating organic carbon and total nitrogen in estuarine marsh soils: calibration with dry combustion. Estuaries 14, 175–179. doi: 10.2307/1351691
Cruickshank M., Tomlinson R., Devine P., Milne R. (1998). “Carbon in the vegetation and soils of northern ireland,” in Biology and environment: Proceedings of the royal Irish academy (JSTOR), 9–21.
Curtis T., Sheehy Skeffington M. J. (1998). “The salt marshes of ireland: an inventory and account of their geographical variation,” in Biology and environment: Proceedings of the royal Irish academy (JSTOR), 87–104.
Dahl M., Deyanova D., Gütschow S., Asplund M. E., Lyimo L. D., Karamfilov V., et al. (2016). Sediment properties as important predictors of carbon storage in zostera marina meadows: a comparison of four european areas. PloS One 11, e0167493. doi: 10.1371/journal.pone.0167493
OSi (2021) Geohive hub. Available at: https://www.geohive.ie/ (Accessed 2022-05-25).
Dontis E. E., Radabaugh K. R., Chappel A. R., Russo C. E., Moyer R. P. (2020). Carbon storage increases with site age as created salt marshes transition to mangrove forests in tampa bay, florida (usa). Estuaries Coasts 43, 1470–1488. doi: 10.1007/s12237-020-00733-0
Doody J. P. (2008). Management of natura 2000 habitats. 1330 Atlantic salt meadows (Glauco-puccinellietalia maritimae). Tech. Rep. European Commission.
Drexler J. Z. (2011). Peat formation processes through the millennia in tidal marshes of the sacramento–san joaquin delta, california, usa. Estuaries Coasts 34, 900–911. doi: 10.1007/s12237-011-9393-7
Duarte C. M. (1990). Seagrass nutrient content. Mar. Ecol. Prog. series. Oldendorf 6, 201–207. doi: 10.3354/meps067201
Duarte C. M., Middelburg J. J., Caraco N. (2005). Major role of marine vegetation on the oceanic carbon cycle. Biogeosciences 2, 1–8. doi: 10.5194/bg-2-1-2005
Eaton J. M., McGoff N. M., Byrne K. A., Leahy P., Kiely G. (2008). Land cover change and soil organic carbon stocks in the republic of ireland 1851–2000. Climatic Change 91, 317–334. doi: 10.1007/s10584-008-9412-2
Ellison J. C., Beasy K. M. (2018). Sediment carbon accumulation in southern latitude saltmarsh communities of tasmania, australia. Biology 7, 27. doi: 10.3390/biology7020027
Fang J., Liu G., Xu S. (1996). Carbon pools in terrestrial ecosystems in china, in emissions and their relevant processes of greenhouse gases in china. China Environ. Sci. Press Beijing.
Farrington A., Haughton J. (1947). The north bull island, co. dublin. Irish Naturalists’ J. 9, 46–48.
Fourqurean J. W., Duarte C. M., Kennedy H., Marbà N., Holmer M., Mateo M. A., et al. (2012). Seagrass ecosystems as a globally significant carbon stock. Nat. Geosci. 5, 505–509. doi: 10.1038/ngeo1477
Gedan K. B., Kirwan M. L., Wolanski E., Barbier E. B., Silliman B. R. (2011). The present and future role of coastal wetland vegetation in protecting shorelines: answering recent challenges to the paradigm. Climatic Change 106, 7–29. doi: 10.1007/s10584-010-0003-7
Giuliani S., Bellucci L. G. (2019). “Salt marshes: their role in our society and threats posed to their existence,” in World seas: An environmental evaluation (Elsevier), 79–101.
Grey A., Cunningham A., Lee A., Monteys X., Coveney S., McCaul M. V., et al. (2021). Geochemical mapping of a blue carbon zone: Investigation of the influence of riverine input on tidal affected zones in bull island. Regional Stud. Mar. Sci. 45, 101834. doi: 10.1016/j.rsma.2021.101834
Heiri O., Lotter A. F., Lemcke G. (2001). Loss on ignition as a method for estimating organic and carbonate content in sediments: reproducibility and comparability of results. J. paleolimnology 25, 101–110. doi: 10.1023/A:1008119611481
Herr D., Pidgeon E., Laffoley D. d. (2012). Blue carbon policy framework 2.0: based on the discussion of the international blue carbon policy working group (International Union for Conservation of Nature).
Hiraishi T., Krug T., Tanabe K., Srivastava N., Baasansuren J., Fukuda M., et al. (2014). 2013 supplement to the 2006 ipcc guidelines for national greenhouse gas inventories: Wetlands (Switzerland: IPCC).
Howard J., Hoyt S., Isensee K., Telszewski M., Pidgeon E. (2014). Coastal blue carbon: methods for assessing carbon stocks and emissions factors in mangroves, tidal salt marshes, and seagrasses (International Union for Conservation of Nature).
Howard J., Sutton-Grier A., Herr D., Kleypas J., Landis E., Mcleod E., et al. (2017). Clarifying the role of coastal and marine systems in climate mitigation. Front. Ecol. Environ. 15, 42–50. doi: 10.1002/fee.1451
Kelleway J. J., Saintilan N., Macreadie P. I., Ralph P. J. (2016). Sedimentary factors are key predictors of carbon storage in se australian saltmarshes. Ecosystems 19, 865–880. doi: 10.1007/s10021-016-9972-3
Kirwan M. L., Megonigal J. P. (2013). Tidal wetland stability in the face of human impacts and sea-level rise. Nature 504, 53–60. doi: 10.1038/nature12856
Leonard L. A., Luther M. E. (1995). Flow hydrodynamics in tidal marsh canopies. Limnology oceanography 40, 1474–1484. doi: 10.4319/lo.1995.40.8.1474
Lo Iacono C., Mateo M. A., Gracia E., Guasch L., Carbonell R., Serrano L., et al. (2008). Very high-resolution seismo-acoustic imaging of seagrass meadows (mediterranean sea): Implications for carbon sink estimates. Geophysical Res. Lett. 35. doi: 10.1029/2008GL034773
Macreadie P. I., Costa M. D., Atwood T. B., Friess D. A., Kelleway J. J., Kennedy H., et al. (2021). Blue carbon as a natural climate solution. Nat. Rev. Earth Environ. 2, 826–839. doi: 10.1038/s43017-021-00224-1
Macreadie P. I., Robertson A. I., Spinks B., Adams M. P., Atchison J. M., Bell-James J., et al. (2022). Operationalizing marketable blue carbon. One Earth 5, 485–492. doi: 10.1016/j.oneear.2022.04.005
Ma S., He F., Tian D., Zou D., Yan Z., Yang Y., et al. (2018). Variations and determinants of carbon content in plants: a global synthesis. Biogeosciences 15, 693–702. doi: 10.5194/bg-15-693-2018
McCorry M. (2007). Saltmarsh monitoring project 2006. Unpublished Rep. to Natl. Parks Wildlife Service Dublin.
Mcleod E., Chmura G. L., Bouillon S., Salm R., Björk M., Duarte C. M., et al. (2011). A blueprint for blue carbon: toward an improved understanding of the role of vegetated coastal habitats in sequestering co2. Front. Ecol. Environ. 9, 552–560. doi: 10.1890/110004
Mcowen C. J., Weatherdon L. V., Van Bochove J.-W., Sullivan E., Blyth S., Zockler C., et al. (2017). A global map of saltmarshes. Biodiversity Data J 5. doi: 10.3897/BDJ.5.e11764
Middelburg J., Nieuwenhuize J., Lubberts R., Van de Plassche O. (1997). Organic carbon isotope systematics of coastal marshes. Estuarine Coast. Shelf Sci. 45, 681–687. doi: 10.1006/ecss.1997.0247
Minello T. J., Able K. W., Weinstein M. P., Hays C. G. (2003). Salt marshes as nurseries for nekton: testing hypotheses on density, growth and survival through meta-analysis. Mar. Ecol. Prog. Ser. 246, 39–59. doi: 10.3354/meps246039
Minello T. J., Rozas L. P., Baker R. (2012). Geographic variability in salt marsh flooding patterns may affect nursery value for fishery species. Estuaries Coasts 35, 501–514. doi: 10.1007/s12237-011-9463-x
Möller I., Kudella M., Rupprecht F., Spencer T., Paul M., Van Wesenbeeck B. K., et al. (2014). Wave attenuation over coastal salt marshes under storm surge conditions. Nat. Geosci. 7, 727–731. doi: 10.1038/ngeo2251
Moore G. E., Burdick D. M., Peter C. R., Keirstead D. R. (2012). Belowground biomass of phragmites australis in coastal marshes. Northeastern Nat. 19, 611–626. doi: 10.1656/045.019.0406
Nelleman C., Corcoran E., Duarte C. M., Valdes L., DeYoung C., Fonseca L., et al. (2008). Blue carbon: The role of healthy oceans in binding carbon (UNEP/FAO/UNESCO/IUCN/CSIC).
Ouyang X., Lee S. (2013). Carbon accumulation rates in salt marsh sediments suggest high carbon storage capacity. Biogeosciences Discussions 10, 19–155. doi: 10.5194/bgd-10-19155-2013
Ouyang X., Lee S. (2014). Updated estimates of carbon accumulation rates in coastal marsh sediments. Biogeosciences 11, 5057–5071. doi: 10.5194/bg-11-5057-2014
Penk M. R. (2019). Preliminary carbon stocks of Irish saltmarshes. Tech. Rep. Environmental Protection Agency.
Penk M., Perrin P. (2021). Data from: Penk and perrin estuaries and coasts. doi: 10.6084/m9.figshare.17212202.v1Penk2021
Penk M. R., Perrin P. M. (2022). Variability of plant and surface soil carbon concentration among saltmarsh habitats in ireland. Estuaries Coasts 45, 1–15. doi: 10.1007/s12237-021-01042-w
R Core Team (2021). R: A language and environment for statistical computing (Vienna, Austria: R Foundation for Statistical Computing).
Ruiz-Fernández A., Carnero-Bravo V., Sanchez-Cabeza J., Pérez-Bernal L., Amaya-Monterrosa O., Bojórquez-Sánchez S., et al. (2018). Carbon burial and storage in tropical salt marshes under the influence of sea level rise. Sci. total Environ. 630, 1628–1640. doi: 10.1016/j.scitotenv.2018.02.246
Ruiz-Fernández A. C., Sanchez-Cabeza J.-A., Serrato de la Pena J. L., Perez-Bernal L. H., Cearreta A., Flores-Verdugo F., et al. (2016). Accretion rates in coastal wetlands of the southeastern gulf of california and their relationship with sea-level rise. Holocene 26, 1126–1137. doi: 10.1177/0959683616632882
Sasmito S. D., Kuzyakov Y., Lubis A. A., Murdiyarso D., Hutley L. B., Bachri S., et al. (2020). Organic carbon burial and sources in soils of coastal mudflat and mangrove ecosystems. Catena 187, 104414. doi: 10.1016/j.catena.2019.104414
Saunders D., Kalff J. (2001). Nitrogen retention in wetlands, lakes and rivers. Hydrobiologia 443, 205–212. doi: 10.1023/A:1017506914063
Shepard C. C., Crain C. M., Beck M. W. (2011). The protective role of coastal marshes: a systematic review and meta-analysis. PloS One 6, e27374. doi: 10.1371/journal.pone.0027374
Smeaton C., Burden A., Ruranska P., Ladd C. J., Garbutt A., Jones L., et al. (2022). Using citizen science to estimate surficial soil blue carbon stocks in great british saltmarshes. Front. Mar. Sci. 1461. doi: 10.3389/fmars.2022.959459
Spencer J., Monamy V., Breitfuss M. (2009). Saltmarsh as habitat for birds and other vertebrates. Aust. saltmarsh Ecol. N. Saintilan (Ed.), (Collingwood: CSIRO Publishing) 7, 149–165.
Sutter L. A., Perry J. E., Chambers R. M. (2014). Tidal freshwater marsh plant responses to low level salinity increases. Wetlands 34, 167–175. doi: 10.1007/s13157-013-0494-x
Unsworth R. K., Butterworth E. G. (2021). Seagrass meadows provide a significant resource in support of avifauna. Diversity 13, 363. doi: 10.3390/d13080363
Wang F., Sanders C. J., Santos I. R., Tang J., Schuerch M., Kirwan M. L., et al. (2021). Global blue carbon accumulation in tidal wetlands increases with climate change. Natl. Sci. Rev. 8, nwaa296. doi: 10.1093/nsr/nwaa296
Wellock M. L., Reidy B., Laperle C. M., Bolger T., Kiely G. (2011). Soil organic carbon stocks of afforested peatlands in ireland. Forestry 84, 441–451. doi: 10.1093/forestry/cpr046
Were D., Kansiime F., Fetahi T., Cooper A., Jjuuko C. (2019). Carbon sequestration by wetlands: a critical review of enhancement measures for climate change mitigation. Earth Syst. Environ. 3, 327–340. doi: 10.1007/s41748-019-00094-0
Windham-Myers L., Crooks S., Troxler T. G. (2018). A blue carbon primer: the state of coastal wetland carbon science, practice and policy (CRC Press).
Keywords: coastal wetland, saltmarsh, carbon stock, blue carbon, Ireland
Citation: Burke SA, Manahan J, Eichelmann E and Cott GM (2022) Dublin’s saltmarshes contain climate-relevant carbon pools. Front. Mar. Sci. 9:976457. doi: 10.3389/fmars.2022.976457
Received: 23 June 2022; Accepted: 28 November 2022;
Published: 19 December 2022.
Edited by:
Juan Jose Munoz-Perez, University of Cádiz, SpainReviewed by:
Amrit Kumar Mishra, The University of Hong Kong, Hong Kong SAR, ChinaMatteo Postacchini, Marche Polytechnic University, Italy
Copyright © 2022 Burke, Manahan, Eichelmann and Cott. This is an open-access article distributed under the terms of the Creative Commons Attribution License (CC BY). The use, distribution or reproduction in other forums is permitted, provided the original author(s) and the copyright owner(s) are credited and that the original publication in this journal is cited, in accordance with accepted academic practice. No use, distribution or reproduction is permitted which does not comply with these terms.
*Correspondence: Shannon A. Burke, c2hhbm5vbi5idXJrZS4xQHVjZGNvbm5lY3QuaWU=