- 1Department of Marine Ecology, Institute of Oceanology Polish Academy of Sciences, Sopot, Poland
- 2Department of Marine Chemistry and Biochemistry, Institute of Oceanology Polish Academy of Sciences, Sopot, Poland
Macrobenthos is an important component of organic carbon storage and energy flow in marine systems, including these impacted by riverine discharge and high share of allochthonous organic matter (OM). Changing environmental conditions, such as lower precipitation and snow cover duration, may affect patterns of riverine input, therefore it is crucial to know how benthic communities utilize different OM sources in areas affected by rivers. Using Bayesian stable isotope mixing models, we assessed the diet composition of common macroinvertebrates forming benthic communities in the transition zone of one of the largest river outlets in the Baltic Sea region, the Vistula River prodelta. Furthermore, we analyzed the spatial and temporal variability in the biomass-weighted resource utilization by those communities. Contribution of riverine OM to the particulate OM in Vistula prodelta decreased with increasing depth. Despite similar distances to the river mouth, the relative contribution of riverine OM to the diet of all studied taxa also decreased with increasing depth, which corresponded to organic matter composition in prodelta waters. Nevertheless, due to synchronous structural changes in benthic communities, and changes in the feeding modes of dominant species, particularly Macoma balthica and Hediste diversicolor, the riverine organic carbon share in the biomass of benthic communities often remained the highest in the deepest prodelta parts. Our study corroborates that benthic primary consumers act as a gateway for riverine organic matter into the marine food web and illustrates that sediment properties and resource partitioning affects spatial structure of benthic communities and their abilities to utilize allochthonous OM.
1 Introduction
Organic matter (OM) transported to the sea by rivers constitutes a sizable energy source for coastal and estuarine food webs (Hoffman et al., 2008; Dunton et al., 2012). Together with nutrients, it promotes high primary and secondary production in river-ocean transition zones (Valiela and Bartholomew, 2015), making them one of the most productive areas of the world (Polis et al., 1997).
Benthic primary consumers are responsible for a large part of OM utilization, sustaining the carbon cycle and energy flow through the ecosystem (Middelburg, 2018; Snelgrove et al., 2018). Macrobenthic organisms not only sequester carbon in their tissues but also become prey for higher trophic levels, such as benthivorous fish (Darnaude et al., 2004; Nurkse et al., 2016; Silberberger et al., 2018). By transporting and irrigating sediments, benthic fauna takes part in nutrient retention and turnover (Snelgrove et al., 2018), and acts as a coastal filter for excessive loadings transported from land (Thoms et al., 2018). Therefore, vital and productive benthic communities are essential not only for effective carbon pathways but also for maintaining ecosystem health and function. This is especially important in river-impacted areas that are characterized by high sedimentation rates and hence intensive OM burial (Dunne et al., 2007; Damrat et al., 2013).
Riverine discharge may affect benthic communities and their food-web structure through many different factors, e.g., by the amount of OM load and its nutritive quality (Deegan and Garritt, 1997; Antonio et al., 2010). Earlier studies on estuarine food webs suggested that low-quality terrestrial OM (like leaves, wood and soil) is usually not a preferred food source for benthic consumers which rely mostly on autochthonous primary production (Deegan and Garritt, 1997; Peterson, 1999). However, some recent investigations revealed that terrestrial OM may state a substantial part of the benthos diet, especially in food-limited areas (Dunton et al., 2012; Kędra et al., 2012). Moreover, consumption of allochthonous OM by benthos may exceed its consumption by pelagic organisms like zooplankton and planktivorous fish (Hoffman et al., 2008; Bartels et al., 2018) and therefore reinforce the bottom-up transport of riverine OM through the marine food webs (Dias et al., 2016; Peller et al., 2020).
The incorporation of allochthonous riverine OM to benthic food webs depends also on the distance from the freshwater resource (Careddu et al., 2015), as well as pathways of sedimentation, which may vary with depth depending on local currents (Darnaude et al., 2004; Antonio et al., 2010; Bartels et al., 2018). Consequently, allochthonous OM consumption may be greatest close to river mouths in case of shallow estuaries overwhelmed by fluvial sedimentation (Chanton and Lewis, 2002; Vinagre et al., 2019). It can also increase with depth when allochthonous OM is transported to deeper, depositional zones (Bartels et al., 2018) which is characteristic of deltaic areas (Darnaude et al., 2004).
Studies on benthic food webs mostly concentrate on the proportions of different food sources to benthic consumers, putting aside the influence of different food quantities and compositions on the community structure (Arbi et al., 2018). Little is known about the influence of riverine OM supply on standing stocks and feeding habits of different benthic fauna species, which significantly reduces the possibility to predict the influence of hydrological changes (including riverine discharge) on the functioning of benthic faunal communities. In many areas of the world, climate change is predicted to influence freshwater runoff from land (Christensen and Christensen, 2007). These predictions raise concerns about potential effects on coastal food webs (Bartels et al., 2018; Vinagre et al., 2019). The Baltic Sea, a semi-enclosed sea with a large catchment area, is especially susceptible to warming and riverine regime shifts related to increasing precipitation in summer and decreasing snow cover in winter (Christensen et al., 2022). However, the potential impact of altered riverine discharge on benthic communities has not been recognized as a consequence of the ongoing climate change (Viitasalo and Bonsdorff, 2022).
The aim of the present work was to assess the spatio-temporal variability in marine and riverine OM utilization by benthic primary consumers inhabiting a temperate riverine-impacted coast (Vistula River prodelta, southern Baltic Sea). Specifically, we asked: [1] what is the seasonal variation of the quantity, quality, and composition of OM mixtures in the Vistula prodelta, [2] what is the relative contribution of riverine and marine OM to the diet of the nine most common benthic taxa, and [3] what is the contribution of riverine and marine organic carbon (Corg) to the total Corg comprised in benthic communities. The answers to these questions will provide new insight into allochthonous OM importance for the functioning of the dynamic, coastal ecosystem prone to environmental change and anthropogenic influence.
2 Materials and methods
2.1 Study area
The study area is located in the Gulf of Gdańsk, within the vicinity of the Vistula River mouth, in the eastern part of the prodelta (Figure 1). The Vistula River is the second biggest river entering the Baltic Sea (HELCOM, 2018), with a water discharge seasonally ranging from 250 to 8000 m3 s−1 [with the maximum outflow during spring and minimum in autumn (Cyberski et al., 2006; Kubiak-Wójcicka, 2020)]. The outflow contributes to 7% of the total annual freshwater discharge into the Baltic Sea (Majewski, 2013). The Vistula River is a very important source of suspended material to the Gulf of Gdańsk. The annual sediment transport into the Gulf ranges from 0.7 to 2.2 million tons of suspension (Emelyanov and Stryuk, 2002), which causes sedimentation rates of around 6600 g m−2 y−1 in the outer Vistula prodelta [the largest sediment load is deposited between February and May, and lowest during summer and autumn; (Damrat et al., 2013)]. The Vistula River is also one of the largest suppliers of OM and nutrients to the Baltic Sea. Kuliński and Pempkowiak (2012) estimated loads of total organic carbon to be 1.76 x 105 t y-1 and total inorganic carbon of.17 x 106 t y-1, which accounts for 5.3% and 21.3%, respectively, of the total amount delivered with rivers to the Baltic Sea. The average loads of nutrients to the Baltic Sea between 1995 and 2014 were 1.0 x 105 t y-1 for total nitrogen and 6.5 x 103 t y-1 for total phosphorus (HELCOM, 2018). The environmental variability generated by the Vistula River discharges (i.e., physical disturbance, quality and quantity of OM) has a huge impact in shaping benthic communities attributes [i.e., density, diversity and taxonomic composition (Włodarska-Kowalczuk et al., 2016)].
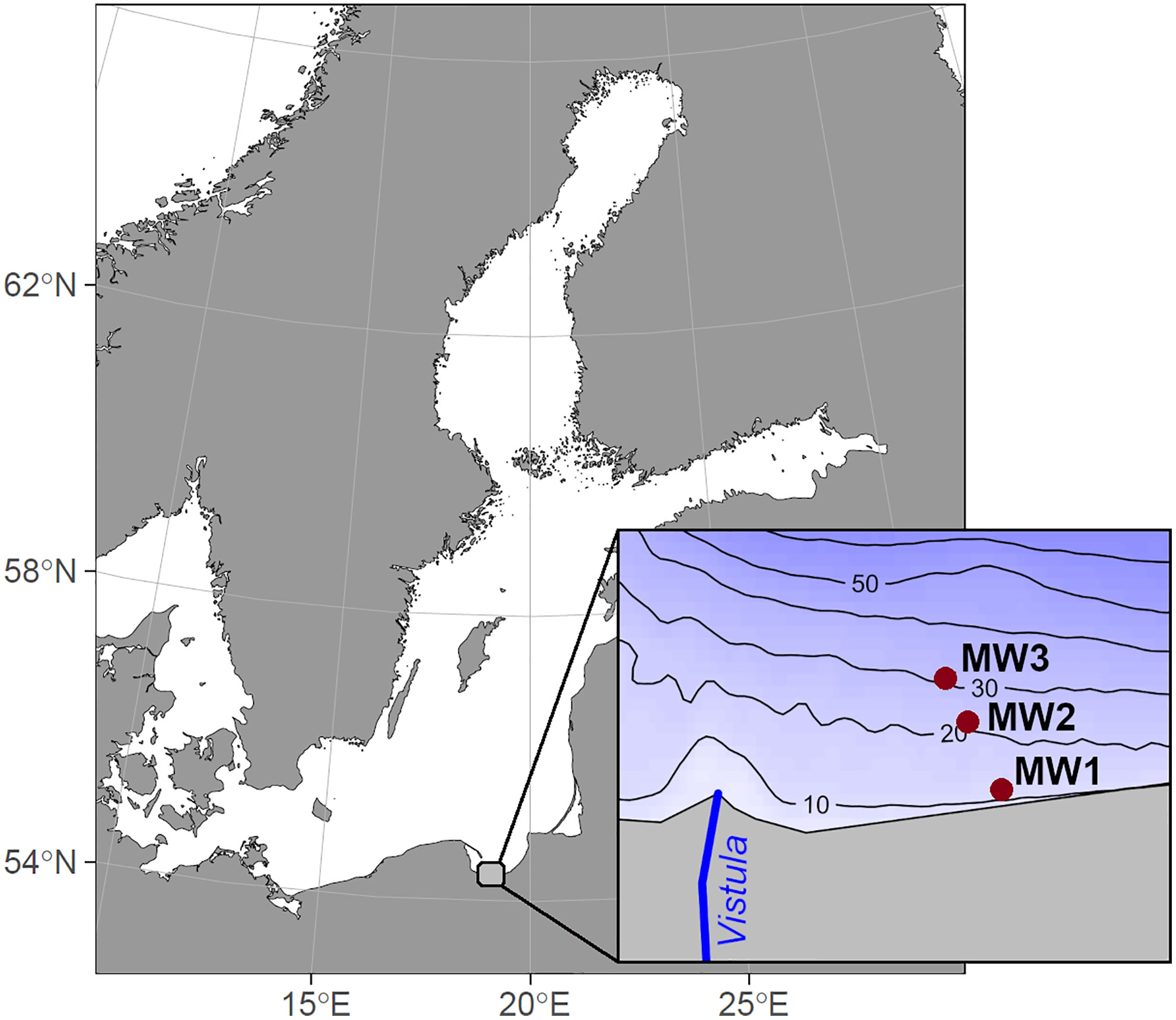
Figure 1 Map of the study area with sampling stations, overview map of the Baltic Sea region in the background.
2.2 Field Sampling
Samples for the study were collected in the Vistula River prodelta, Gulf of Gdańsk (Figure 1) from r/v Oceania, during 5 research cruises (October 2018, January 2019, March-April 2019, October 2019 and January 2020), covering two early autumns, one spring and two winters. Samples were collected at 3 stations – MW1, MW2 and MW3, located at similar distances to the river mouth but representing different depths (Table 1). At each station temperature and salinity profiles were measured with Sea-Bird SBE 19 plus CTD probe.

Table 1 Geographic coordinates and environmental conditions for each sampling station in the Vistula prodelta.
Samples of water for prodelta particulate OM (pPOM) were collected by a Niskin bottle (bottom water). The water was filtered through pre-weighted and pre-combusted Whatman GF/F filters (ø 47 mm; pore size: 0.7 μm) and frozen at -20°C until analysis. Additional water samples were filtered for phytopigments analysis [i.e., chlorophyll a (chl a) and phaeophytin (phaeo)] through Whatman GF/F filters (ø 25 mm; pore size: 0.7 μm) and frozen at -80°C.
At each sampling station surface sediments for grain size analysis, prodelta sedimentary OM (pSOM; upper 5 cm layer) and phytopigments (upper 1 cm layer) were collected from box corer and frozen at -20°C and -80°C, respectively.
Sediment samples for macrofauna were collected with a van Veen grab (0.1 m2) in four replicates at each station. Sediments were washed over a sieve with a mesh size of 0.5 mm. Three replicates were preserved in a 4% formaldehyde solution buffered with borax for later taxonomic analysis. One replicate sample was used to collect macrofauna for stable isotope analysis. This sample was kept in a fridge for 24h (allowing gut clearance) and then sorted under a stereomicroscope onboard. All taxa present in a sufficient amount for isotopic analysis (>2 mg dry weight) were collected and frozen at -80°C until further analysis. For bivalve species (size >1 cm) only muscle tissues were collected.
Additionally, samples for marine POM (mPOM) and riverine POM (rPOM) for stable isotope analysis were collected at the open coast of the southern Baltic (mPOM), and ~12 km upstream in the Vistula River (rPOM) respectively. Marine POM from the open coast was sampled at three stations (ML1: 54.78°N, 17.43°E; ML2: 54.84°N, 17.42°E; ML3: 54.97°N, 17.43°E) during the same research cruises and with the same methodology as in case of samples from prodelta. Samples of rPOM were collected in May 2019 (Silberberger et al., 2021) and November 2020, representing spring and autumn respectively, with water canisters directly from the river shore (54.25°N, 18.95°E).
2.3 Laboratory analysis
The analyses of Corg, as well as total nitrogen (Ntot) concentrations, and their stable isotopes (δ13C and δ15N) were performed in an Elemental Analyzer Flash EA 1112 Series combined with the Isotopic Ratio Mass Spectrometer IRMS Delta V Advantage (Thermo Electron Corp., Germany). Samples of benthic animals, sediments and filters with POM were freeze-dried, homogenized and weighed into silver capsules (about 1 mg, 75-80 mg and 35-45 mg, respectively). Afterward, samples were soaked with 2 M HCl to remove carbonates and dried at 60°C for 24 h [the procedure was repeated until a constant weight was obtained (Kuliński et al., 2014)]. Corg and Ntot measurements were calibrated against certified reference materials (marine sediments) provided by HEKAtech GmbH (Germany). δ13C results were calibrated against IAEA standards: CO-8 and USGS40, and δ15N results against N-1 and USGS40. Stable isotope ratios are given in the conventional delta notation, i.e., versus Vienna Pee Dee Belemnite for 13C and versus air for 15N as parts per thousand (‰).
The concentrations of chl a and phaeo in bottom water (filters) and surface sediments were analyzed following the EPA 445.0 method (Arar and Collins, 1997) with the use of a Fluorimeter Trilogy® (Turner Designs Inc.). Defrosted filters and freeze-dried and homogenized sediments were extracted in 10 ml of 90% acetone for approximately 24 h at 4°C. The centrifuged (3000 rpm for 2 min) supernatant was then analyzed before and after acidification with 100 µl of 0.1 M HCl (Arar and Collins, 1997).
Sediment samples for granulometric analysis were dried (48 h, 60°C) and sieved with a shaker through a set of standard test sieves with mesh diameters of 2, 1, 0.5, 0.25, 0.125 and 0.063 mm (Folk and Ward, 1957). Sediment parameters were calculated using the method of Folk and Ward (1957) in the program GRADISTAT 9.0 (Blott and Pye, 2001).
Benthic community samples were sorted under a stereomicroscope to the lowest possible taxonomic level, counted and weighed (blotted wet weight of formalin preserved samples). Additionally, the biomass/abundance (B/A) ratio for each taxon was calculated, which gives information about an average individual’s body size.
2.4 Data analysis
2.4.1 Organic matter properties
To visualize OM patterns among sampling stations and seasons, a Principal Component Analysis (PCA) was conducted in PRIMER v7 statistical software (Clarke and Gorley, 2015). In total, sixteen variables characterizing OM properties were used i.e., Corg, Ntot, δ13C, δ15N, Corg/Ntot, chl a, phaeo, and chl a/phaeo for both pPOM and pSOM. All data were scaled to 0 mean and unit variance (i.e., normalize option in PRIMER) prior to the analysis.
2.4.2 Mixing models
To quantify the contribution of mPOM and rPOM to the pPOM mixtures, a Bayesian mixing model approach was used (Parnell et al., 2013), which is a statistical framework for quantifying proportional contributions of various sources to a mixture, allowing for application of different extensions, such as trophic discrimination factors (TDFs), concentration dependence and various error structures (Stock et al., 2018). Our approach assumes that the pPOM collected at stations MW1-3 are mixtures of marine and river POM and that the local marine production in the Vistula prodelta is isotopically the same as in the open coast area. However, we are aware that some part of marine primary production may be supplied by riverine dissolved carbon and nitrogen, and if so, our model will take it as riverine source.
The modeling was conducted with the use of MixSIAR package (Stock and Semmens, 2016; Stock et al., 2018) in R version 4.1.2 (R Core Team, 2021). Due to relatively high seasonality of pPOM isotopic values in the study area (Szczepanek et al., 2021), four separate models were run, one for the pPOM data collected during the whole study period (5 sampling campaigns), and three separate models for autumn (2 sampling campaigns), winter (2 sampling campaigns), and spring (1 sampling campaign). The parameters of the model run were set to “extreme” (chain length = 3,000,000; burn = 1,500,000; thin = 500; chains = 3), meaning that 3 Markov chains were each sampled 3000000 times. The first half of the samples were discarded (burn-in) to ensure that the chains converged. This was followed by subsampling (thinning) of the retained second half of each Markov chain, meaning that only every 500th sample was kept, resulting in a total of 3000 samples per chain (i.e., 3 x 3000 = 9000 samples). The thinning is applied, since samples of a Markov chain are correlated, and thinning helps to reduce this correlation. Since the composition of pPOM is exclusively a mixing problem and does not involve a trophic transfer, TDFs for both isotopes were set to 0.
In order to select a group of reliable benthic taxa for the assessment of dietary contributions, the SIMPER analysis was conducted on the square-rooted biomass data that returned the percentage similarity of taxonomic composition within stations (Bray-Curtis similarity) (Table 2). The SIMPER analysis allowed to distinguish 11 common taxa, from which 9 were selected for further analyses, namely: Cerastoderma glaucum, Mya arenaria, Macoma balthica, Peringia ulvae, Corophium spp., Oligochaeta, Pygospio elegans, Marenzelleria spp., and Hediste diversicolor. The two remaining species, i.e. Diastylis rathkei and Streblospio shrubsoli were rejected due to an insufficient number of isotopic samples. SIMPER was conducted in PRIMER v7 statistical software (Clarke and Gorley, 2015).
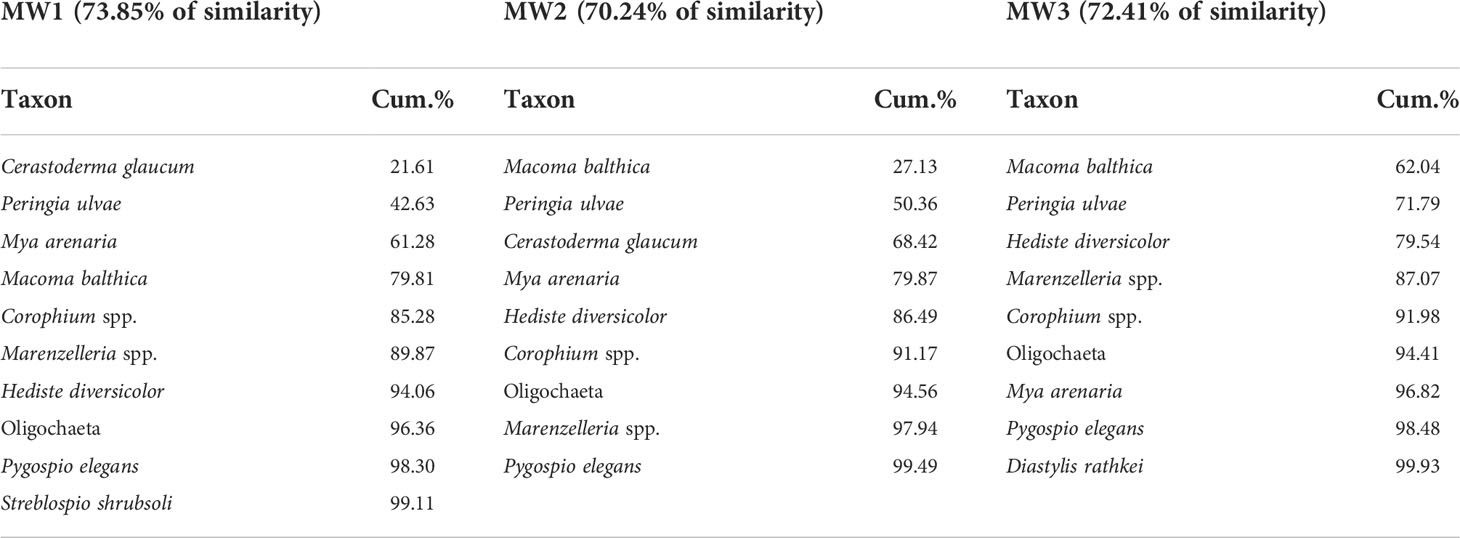
Table 2 The cumulative percentage similarity (Bray-Curtis) of taxonomic composition and biomass (√ transformed) at three sampling stations.
For each of the 9 taxa, a separate mixing model was conducted with a fixed effect: station ID in order to estimate the relative contribution of food sources (mPOM, rPOM and pSOM) to their diet at each sampling station (MW1, MW2, MW3). The parameters of the model runs were also set to “extreme”. Since differences between pSOM and mPOM values were not significant (Kruskal-Wallis test, p = 0.531 for δ13C and p = 0.474 for δ15N), resulting in high uncertainty of modeled probability distributions, those two sources were combined a posteriori (Phillips et al., 2005) into a “marine” source, whereas the rPOM represented the “riverine” source. The a posteriori summing of particular source proportions is recommended, when some sources are poorly differentiated, since it enables to include all potential sources without losing covariation structure among source proportions (Phillips et al., 2005; Phillips et al., 2014).
For mixing models of macroinvertebrates, we adapted TDFs that have been previously used for one of our sampling station (Silberberger et al., 2021). Accordingly, and were used for the first trophic transfer (i.e., from OM to primary consumers). Since δ15N indicated two species (H. diversicolor and Marenzelleria spp.) to be secondary consumers, and were used in mixing models for these species.
In our mixing models, we used the residual error structure that is appropriate in applications with an integrated sampling approach [i.e., when consumer sample is pooled from more than one individual (Stock et al., 2018)]. This error accounts for variation in consumer data originating from differences in biochemical reactions of individual consumers, assuming that all consumers equally feed on the source means (Stock and Semmens, 2016).
2.4.3 Relative Contribution Index
The measured wet weight (WW [g m-2]) of the 9 common taxa was converted into dry weight (DW) or shell-free DW (SFDW) in the case of mollusks, according to literature based conversion factors (Rumohr et al., 1987; Gogina et al., 2022). Next, the mean organic carbon content (Corg) for those taxa was quantified, based on the mean relative Corg measured for each species and station. Finally, to quantify the percentage contribution of marine and riverine food sources to the total organic carbon of benthic communities (represented by the common taxa), the relative contribution index (RCj) (Arbi et al., 2018) was calculated following equations (1) and (2):
where Corg is the organic carbon of the ith taxon per m2 and Pj is the percentage contribution of the jth source to the diet of ith taxon;
where BT is the total Corg [g m-2] in all 9 taxa. In other words, RCj is a percentage sum of organic carbon originating from j source, accumulated by all species in the community. The RC [%] for both food sources was calculated for each grab sample from the probability distribution of these food sources in the diet of each taxon (sum from all analyzed taxa). Additionally, the RCj index (median) was used to estimate the total content of marine and riverine Corg in benthic communities among seasons and sampling locations.
3 Results
3.1 Environmental conditions
A clear seasonal variation of OM quantity and quality was observed (Figure 2), both in the bottom water and in surface sediments of the Vistula prodelta (Supplementary Table 1). In winter, the water column at all stations was characterized by low values of chl a (0.2 – 1.2 µg L-1) and chl a/phaeo (0.12 – 0.32) and depleted δ13C (-29.7 – -26.4 ‰). In spring, the water at all stations was characterized by high concentrations of chl a (3.8 – 5.1 µg L-1), chl a/phaeo (2.01-2.49) and Corg (0.36 – 0.48 mg L-1). In contrast, during both autumns OM parameters were more variable among sampling stations, and chl a, chl a/phaeo and Corg in sediments were increasing with depth. Additionally, in autumn, δ15N values in the water column were depleted in comparison to other seasons (0.97 – 1.81 ‰, excluding MW1 in 2019 – 4.8 ‰).
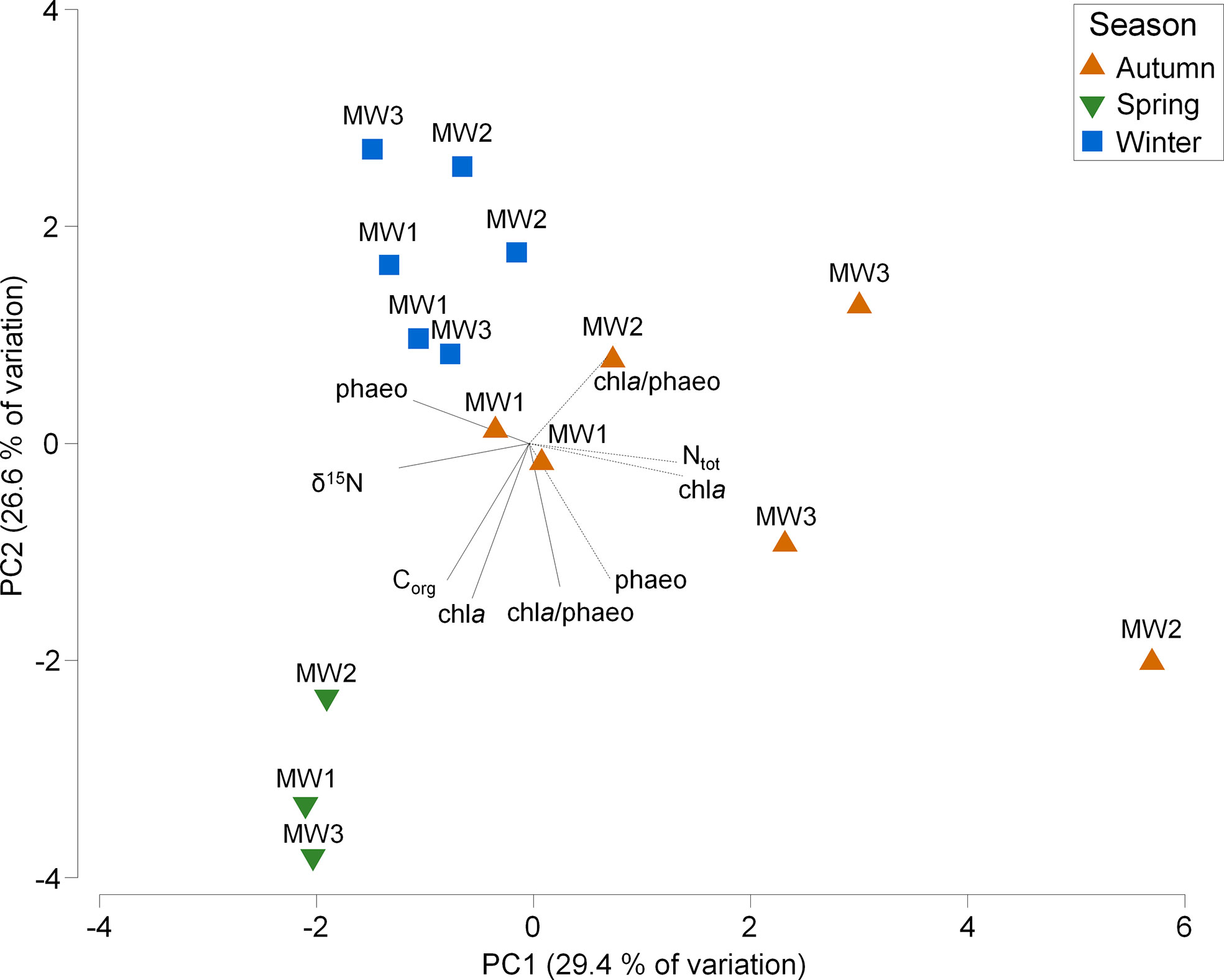
Figure 2 Principle Component Analysis (PCA) of organic matter properties in water and sediments among sampling stations (MW1, MW2, MW3) and seasons. Solid lines indicate important water variables, whereas dotted lines indicate important sediment variables.
3.2 Organic matter composition
The isotopic composition of rPOM did not differ among sampling campaigns (-31.94 ± 0.45 ‰ for δ13C and 9.96 ± 0.31 ‰ for δ15N). Marine POM was more variable among seasons, especially in case of δ15N values (-26.56 ± 0.89 ‰ for δ13C and 3.32 ± 1.61 ‰ for δ15N). In general, the contribution of rPOM to the mixtures of pPOM in the study area decreased from MW1 towards MW2 (from 27.1% to 4.3%), and only slightly further to MW3 (1.7%) (Figure 3). The relative contribution of rPOM at all stations was higher during winter in comparison to other seasons.
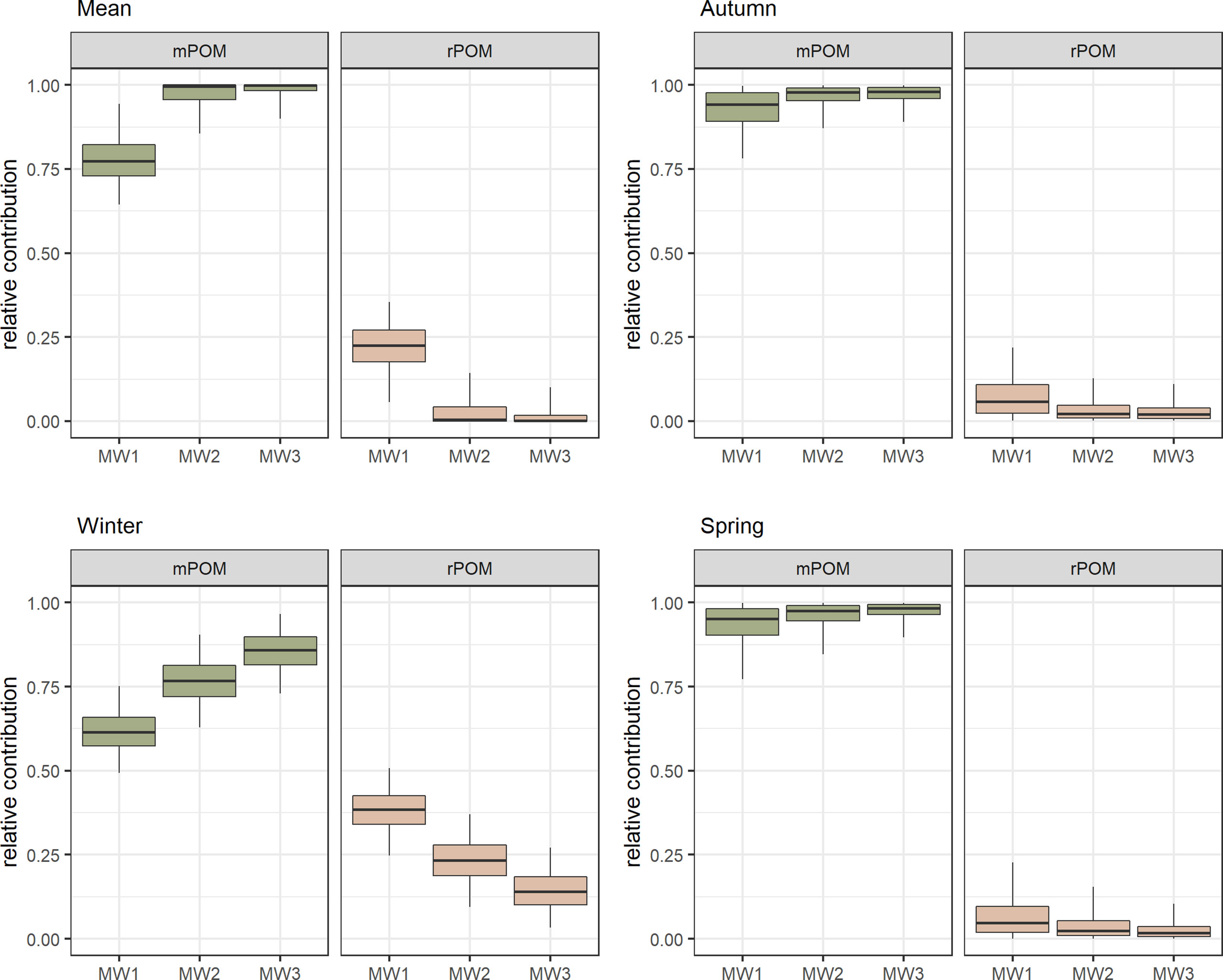
Figure 3 The probability distribution of relative contribution [%] of mPOM (collected at the open coast of western Poland) and rPOM (collected ~12 km upstream in the Vistula River) to the pPOM (prodelta POM, collected at sampling stations). Boxplots depict median (horizontal line) with 50% (box) and 95% credible intervals (vertical line).
3.3 Benthic community structure
The highest abundance was observed at station MW1 (up to 70,640 ind. m-2) and was associated with high numbers of M. arenaria, P. ulvae, C. glaucum and M. balthica (Table 3). Those four species also evenly dominated benthic biomass and benthic Corg at MW1. At stations MW2 and MW3 the abundance of macrofauna was lower (3387 – 10,683 ind. m-2 and 3617 – 10,840 ind. m-2, respectively) and the number of species that dominated the biomass decreased with depth – C. glaucum and M. balthica at MW2 and only M. balthica at MW3. The average individual body size (B/A ratio) was rather stable across depths for small-bodied taxa, whereas it varied for bivalve species and particularly for polychaete H. diversicolor with the highest values at MW3 (Table 3).
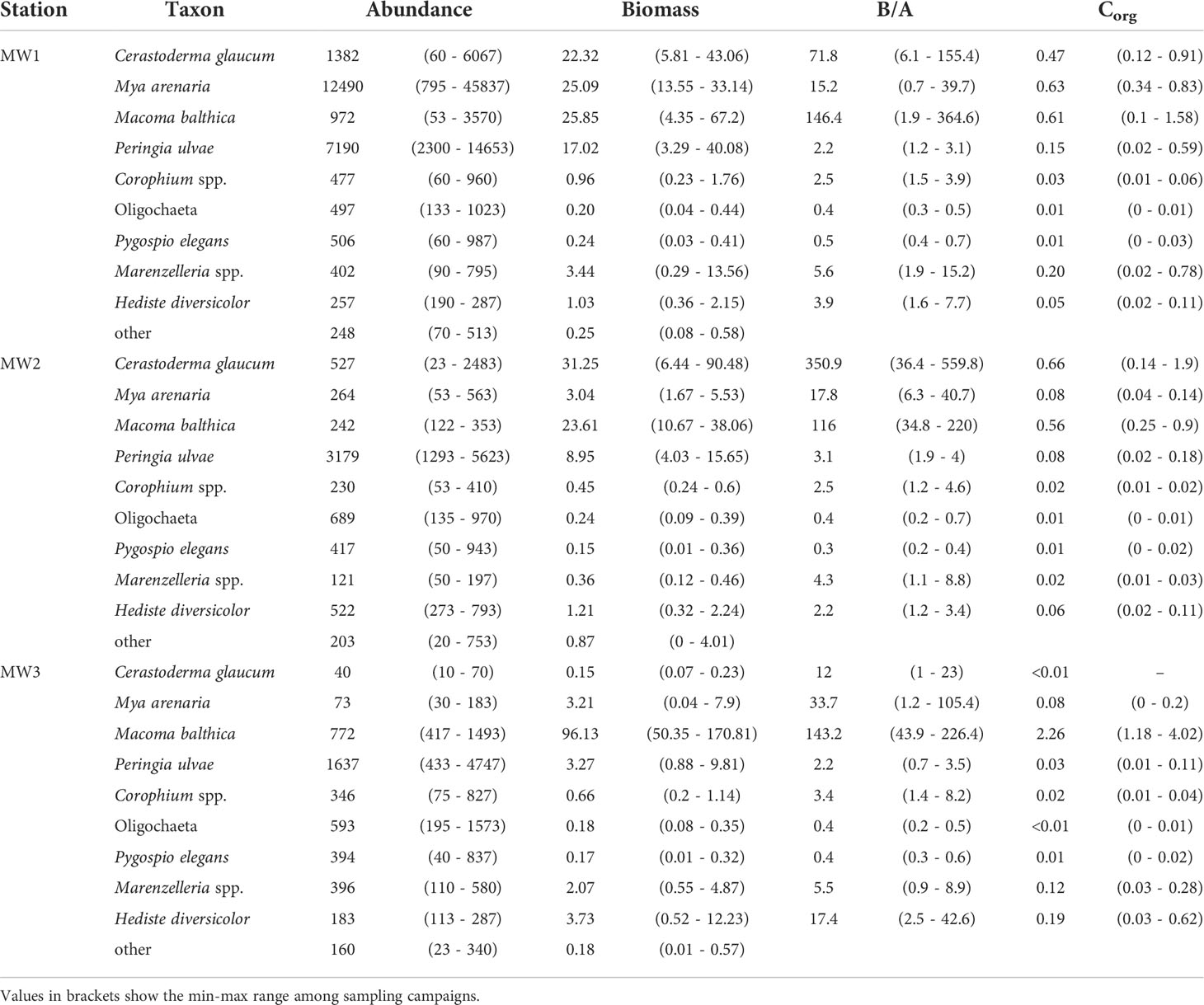
Table 3 The mean abundance [ind. m-2], biomass [WW g m-2], biomass/abundance ratio (B/A) [mg ind.-1], and Corg [DW g m-2; SFDW for mollusks species] of the common benthic taxa at the sampling stations.
3.4 Benthic food-web structure
The isotopic composition of the benthic fauna ranged from -24.64 to -22.21 ‰ and from 4.46 to 12.56 ‰ for δ13C and δ15N, respectively (Figure 4). The lowest δ15N values were characteristic for C. glaucum and M. arenaria whereas the highest for H. diversicolor and Marenzelleria spp.
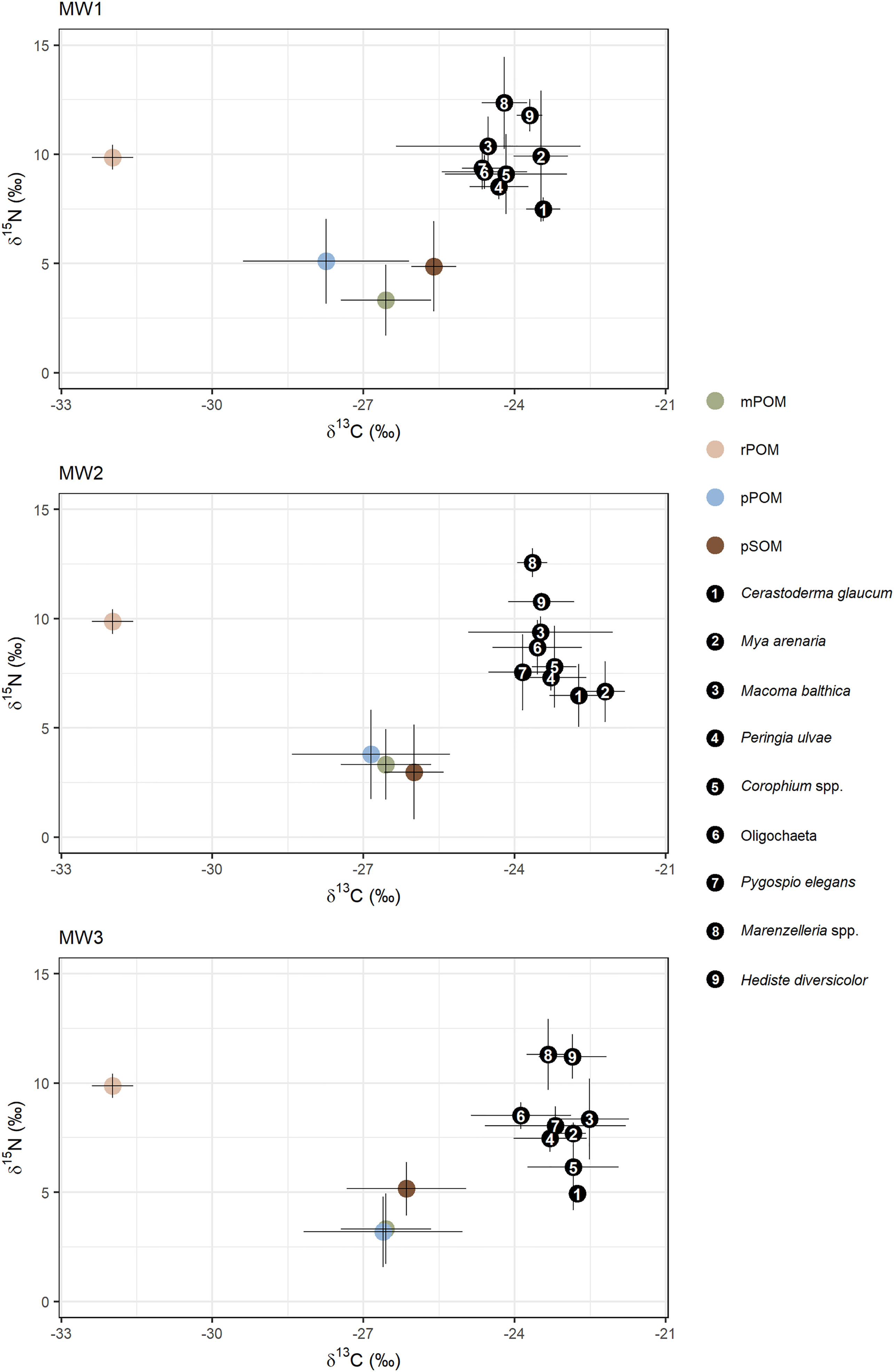
Figure 4 Biplots of carbon and nitrogen isotopic ratios of the common benthic taxa (indicated by numbers 1-9) and their food sources: mPOM – marine POM from the open Polish coast; rPOM – riverine POM from ~12 km upstream in the Vistula River; pPOM – prodelta POM collected at sampling stations; pSOM – prodelta sedimentary OM collected at sampling stations. Points indicate the mean (all sampling campaigns) and whiskers indicate standard deviation. Figure represent raw data before applying trophic enrichment factors.
The dietary contribution of rPOM for all taxa in this study was the highest at station MW1, with the highest values for M. balthica (47.7%) and Marenzelleria spp. (42.3%) (Figure 5). The contribution of rPOM decreased from MW1 toward MW2, and further toward MW3 for most species. C. glaucum, M. arenaria and P. ulvae had the lowest overall contribution of rPOM (2-12%).
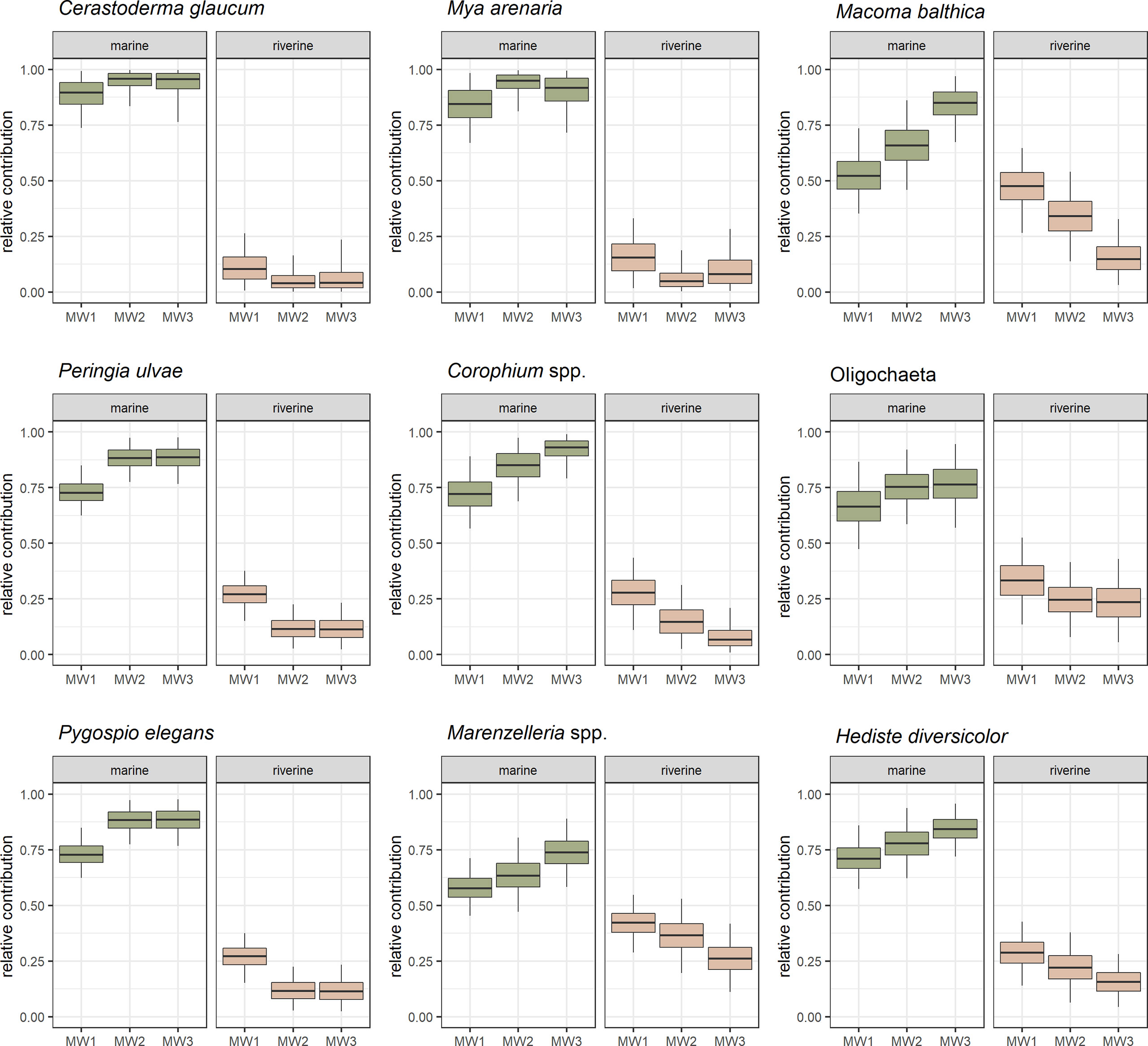
Figure 5 The probability distribution of relative contribution [%] of marine and riverine food sources in the diet of the common benthic taxa at sampling stations (MW1, MW2, MW3). Boxplots depict median (horizontal line) with 50% (box) and 95% credible intervals (vertical line).
3.5 Relative Contribution index
The average RC for rPOM decreased with depth and was 26.05% (10.97 – 42.35 [95% credible interval – CI95]) for MW1, 19.52% (6.98 – 35.50 [CI95]) for MW2 and 14.80% (3.55 – 31.49 [CI95]) for MW3. The total Corg content in benthic communities decreased after autumn 2018 at all stations (Table 4) and then increased next autumn at MW1 and MW3. In general, the total Corg content was the highest at MW3. Thus, although the RC for rPOM at this station was lower in comparison the station MW1, the riverine Corg at MW3 was the highest during three out of five campaigns.
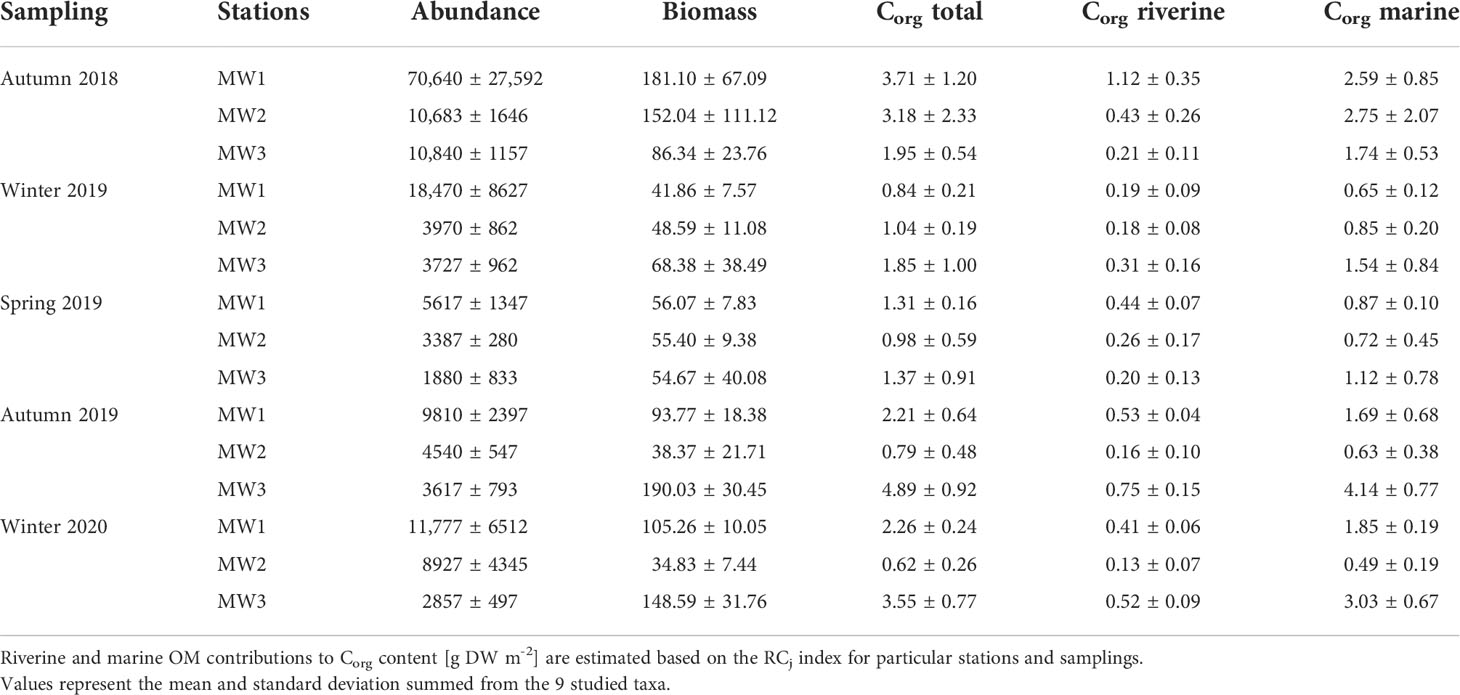
Table 4 The abundance [ind. m-2], biomass [g WW m-2] and total, riverine, and marine Corg contents [g DW m-2] in benthic communities among stations and sampling campaigns.
4 Discussion
Our study shows that utilization of riverine OM differed among feeding groups of benthic primary consumers in the Vistula River prodelta. Common species of obligatory and facultative deposit feeders i.e., Oligochaeta (Attrill et al., 2009), P. elegans (Brey, 1991), Marenzelleria spp. (Dauer, 1997) and H. diversicolor (Fauchald and Jumars, 1979) showed a higher contribution of rPOM in their diet in comparison to obligatory suspension feeders (C. glaucum, M. arenaria). Populations of M. balthica, which is known for the ability to switch between suspension- and deposit-feeding mode (Ólafsson, 1986), differed in the biomass and abundance as well as dietary proportions of food sources depending on depth, and the availability of fresh marine OM. Although the consumption of rPOM decreased with increasing depth for all species, changes in the community structure with depth resulted in similar levels of riverine Corg utilization in the shallow and deepest areas of this riverine impacted coast.
4.1 Contribution of riverine OM to benthic diet
The relative contribution of rPOM to the pPOM (prodelta POM) mixture decreased with increasing depth. Similarly, a decreasing contribution of riverine OM to the diet of benthic fauna was noted from the shallowest to deepest station. Similar pattern was observed in other riverine impacted areas e.g., in Chesapeake Bay (Hoffman et al., 2008) or Tyrrhenian Sea (Careddu et al., 2015). However, other studies observed also the opposite trend i.e., increasing contribution of allochthonous OM with increasing depth (Darnaude et al., 2004; Premke et al., 2015; Bartels et al., 2018). These contrary observations were suggested to result from different topology and hydrological conditions of estuaries and river deltas, which lead to higher sedimentation of allochthonous material in deeper deltaic areas. In the Vistula River prodelta, the highest sedimentation is observed close to the river mouth, however, the sedimented material largely becomes resuspended and transported to Gdańsk Deep, a depositional area outside the Gulf of Gdańsk (Damrat et al., 2013). This may explain lower abundance of riverine material at our outermost station.
Nevertheless, the contributions of rPOM to the pPOM at sampling stations were lower than those observed in the benthic fauna diet, especially at the deepest station. It is possible that we did not always detect riverine OM in pPOM, especially at deeper stations, since the vertical extent of Vistula waters was found to reach up to 10 m from the surface (Grelowski and Wojewódzki, 1996). However, in the long term, the suspended material from riverine flow can to some extent get to the bottom layer and surface sediments and affect the diet composition of fauna (Darnaude et al., 2004). It is worth mentioning that pycnocline was observed in the study area during both winter samplings, with less saline waters transported along the surface. However, differences in the isotopic values from bottom and surface water layers (δ13C = -28.39 ± 1.43 and δ15N = 4.70 ± 1.05 for bottom and δ13C = -27.19 ± 0.39 and δ15N = 3.89 ± 0.81 for surface waters respectively) indicated more allochthonous OM in the bottom water (Maksymowska et al., 2000), most probably originating from more intensive discharge typical for late autumn. In remaining seasons, water column was usually well-mixed and therefore ordinary sedimentation should be assumed. It is important to recognize that Gulf waters have naturally low salinity of approximately 7 and therefore both water masses usually mix immediately.
4.2 Depth-related patterns of rPOM availability
All stations in our study were located at a similar distance from the river mouth in the east of the outflow which is a prevailing direction in which riverine water masses are introduced into the Gulf (Grelowski and Wojewódzki, 1996; Voss et al., 2005). It is because of prevailing westerly winds that push riverine waters eastwards in this area. Thus, the observed decrease of riverine OM contribution with depth to both the pPOM mixture and the diet of macrofauna is most likely caused by an increasing contribution of marine primary production to the vertical OM flux with increasing volume of the water column (Careddu et al., 2015). Indeed, the most effective summer primary production in the study area is observed further in the offshore zone (Ochocki et al., 1995), and chl a concentrations in the surface layer were shown to increase with increasing bottom depth (and distance from the shore) during spring (this study) and autumn (Ochocki et al., 1995). Moreover, Corg concentrations in sediments were usually higher at MW3 in comparison to shallower stations (Supplementary Table 1), which supports this statement.
During calm weather and winds from the north, the outflow of the Vistula can be blocked to some extent close to the river mouth (Matciak and Nowacki, 1995; Grelowski and Wojewódzki, 1996). Under such conditions surface water pockets are formed and transported in both directions along the coast which prevents deeper areas from the river influence. These may additionally explain why the decrease of riverine food in the benthos diet is particularly prominent between MW1 and MW2, whereas it decreases further at MW3 only for particular taxa.
4.3 The role of feeding modes in rPOM consumption
In our study, deposit feeders (Oligochaeta and polychaetes, M. balthica) showed higher reliance on rPOM in their diet, which reflected the pPOM composition in winter. In contrast, the diet composition of suspension-feeding taxa (M. arenaria, C. glaucum) and selective feeding P. ulvae and Corophium spp. (Fenchel and Kofoed, 1976; Nielsen and Kofoed, 1982) resembled the pPOM composition during the productive period (spring and autumn), confirming their preference for higher-quality, autochthonous food source. Therefore, it is likely that the diet of deposit feeders originated from rPOM carried by riverine flow during periods of higher detrital abundance (winter) or that they were outcompeted by suspension feeders for fresh primary production (spring, autumn). This is further supported when differences in the response time to fresh primary production among different feeding groups are taken into account. Populations of deposit feeders were shown to react to primary production supply later than suspension feeders which was demonstrated by faster population growth of the latter (Lessin et al., 2019). Lessin et al. (2019) explained that it may be a mechanism of suspension-feeding fauna control on the fresh food supply to deposit feeders.
Deposit feeders rely on OM accumulated in or at the surface of the bottom and the quantity and nutritional value of accumulated OM depends on many environmental factors e.g., rates of sedimentation, amount of refractory compounds, and intensity of microbial remineralization (Levinton, 1989; Thornton and McManus, 1994). Thus, the fresh OM supply to this feeding group is potentially more limited than to suspension feeders (Levinton, 1972) which might cause increased importance of allochthonous riverine subsidy in their diet. This can be supported by rather low Corg concentrations in sediments throughout the year in contrast to water column, where Corg is only slightly higher during spring bloom comparing to other seasons (Supplementary Table 1; Szczepanek et al., 2021).
Previous findings also showed that deposit feeders may to some degree rely on terrigenous OM (Darnaude et al., 2004; McGovern et al., 2020) that is one of the main components of OM transported by rivers. Attrill et al. (2009) suggested that higher reliance of Oligochaeta on terrigenous matter is probably the result of their evolutionary association to freshwater ecosystems that to a large extent are subsidized by terrigenous detritus. Since most deposit feeders feed rather non-selectively on sedimentary detritus (Levinton, 1989), it is likely that terrigenous OM contribution in the diet of other deposit-feeding species is higher than for obligatory suspension feeders. This probability may additionally increase with getting closer to freshwater resource and associated increase of terrigenous OM to available sedimentary OM pool.
4.4 Changes in community structure with depth
In connection to changes in the diet composition of benthic fauna, the structural changes in community abundance and biomass among stations were also noticeable. The most prominent changes with depth were visible for the bivalve species. Suspension-feeding bivalves like M. arenaria and C. glaucum are found specifically in shallow sandy areas of the southern Baltic Sea (up to 25 m, Gic-Grusza et al., 2009; Gogina and Zettler, 2010). Thus, the limited abundance of M. arenaria and C. glaucum at station MW3 (32 m) could be related to limited depth range of those species. Kube (1996) also observed the highest abundance and biomass of M. arenaria close to the shore in sandy sediments of the Pomeranian Bay (southern Baltic Sea).
The absence of large individuals of suspension feeders at the shallowest station could be also the result of increased turbidity and resuspension by the river current which is unfavorable for their growth and survival (Archambault et al., 2004). However, the sampling stations in the study have been located by design at a greater distance from the river mouth, to not be directly affected by salinity changes or increased water turbidity and sedimentation (Włodarska-Kowalczuk et al., 2016).
Changes in the structure of benthic species populations across depth may be the result of sediment-dependent species distributions, together with their causes and effects (Snelgrove and Butman, 1994). Many previous studies reported that sediment grain size affects the distribution and densities of benthic species, including bivalves (Thrush et al., 2003; Compton et al., 2009; Gerasimova et al., 2019). It was shown that distribution of juvenile and adult populations of different bivalve species can be driven by sediment type selection during post-settlement migrations (Huxham and Richards, 2003; Compton et al., 2009). Compton et al. (2009) reported that while juvenile and adult individuals of deposit-feeding M. balthica inhabited separate sedimentary habitats across wide spatial scale, the same pattern was not observed for suspension feeders (C. edule and Scrobicularia plana). Similar effect on spatial distribution of M. balthica could be also induced by sediment-dependent feeding activity (Ólafsson, 1986; Kamermans et al., 1992). Ólafsson (1986) observed that in muddy sediments M. balthica preferred to feed on deposited material, whereas, in sediments dominated by sands, individuals actively suspension-fed. In our study, a positive correlation was observed between the biomass of M. balthica and mean grain size (Pearson’s r, 0.56), whereas weaker, negative correlations were observed for suspension feeders (-0.19 and -0.31 for C. glaucum and M. arenaria, respectively). This suggests that sediment grain size plays an important role in shaping populations of these species in the Vistula River prodelta.
Differences in spatial distributions could be also the result of resource partitioning between suspension-feeding and deposit-feeding species (Levinton, 1972; Ólafsson, 1986). It was shown that in areas of high abundance of bivalves, the interspecific competition between suspension feeders occured, leading to a switch of M. balthica to deposit-feeding (Kamermans et al., 1992). This may explain the much higher contribution of riverine OM to the diet of this species at the shallow station. In contrast, at deepest station, the diet of M. balthica was dominated by marine OM (this study, Silberberger et al., 2021). This suggests that the resource limitation for this species was no longer the case, and suspension-feeding strategy was predominant (Ólafsson, 1986). At a greater depth, marine food supply is larger and this decreases the level of intraspecific food competition. Interestingly, the biomass and individual body size of deposit-feeding H. diversicolor in our study also increased with depth. We suggest that this can be linked to the likely switch in the diet of M. balthica. When M. balthica feeds on suspended material at MW3 it results in a larger niche size and higher food availability for other deposit feeders (Levinton, 1972). As previously mentioned, at MW3 Corg concentrations in the sediment were higher throughout the year in comparison to shallower stations (Supplementary Table 1). Thus, higher biomass of H. diversicolor at MW3 could result from both lower competition from M. balthica and higher detrital abundance.
4.5 Riverine Corg utilization
In our study, the riverine share in the biomass of benthic communities remained similar among the shallow and deepest prodelta parts. This is a result of above-described structural changes in benthic communities, specifically for M. balthica and H. diversicolor which biomass and feeding habits switch between both areas. These suggests that the riverine supply plays an important role in the coastal system of the Gulf of Gdańsk. Although the riverine OM sustains around 15% of Corg demand (RCj) to the benthic species at 30 m depth, high biomass of M. balthica that effectively utilizes riverine Corg surely supports substantial transport of allochthonous Corg to higher trophic levels e.g., demersal fish (Nurkse et al., 2016). Additionally, due to their typically long life span (Gilbert, 1973; Kube, 1996) and production of calcium carbonate shells, marine bivalves act as an effective carbon sink supporting ecosystem functions and counteracting the negative effects of climate change (Filgueira et al., 2018).
The high content of riverine OM in benthic biomass shown in our study confirms that allochthonous OM is an important food subsidy to benthic secondary production in coastal riverine-impacted systems (Hoffman et al., 2008; Dunton et al., 2012). The observed and predicted climate change, and related decrease in snow cover and riverine discharge in Europe (Christensen and Christensen, 2007; Christensen et al., 2022) may lead to reduced allochthonous OM supply by the river (Vinagre et al., 2019). As shown in our study, changes in community structure and dietary contributions of benthic primary consumers may be directly linked to food composition. Thus, any changes in allochthonous OM supply may substantially affect the functioning and ecosystem services provided by coastal macrobenthic communities.
5 Conclusion
Our study shows that riverine OM supports benthic food web in the Vistula River prodelta. The importance of riverine OM decreases with depth affecting the community structure and functional feeding roles of benthic fauna. In the shallowest waters, the diet of benthic species is supported by riverine OM supply the most, and abundant populations of small-sized suspension feeders are favored most likely due to suitable depth range and sediment sorting. With increasing depth, the predominance of dietary-flexible M. balthica and H. diversicolor increases and we suggest that it is the result of the decrease of both inter- and intraspecific competition and increasing quantity of marine food supply.
Our results indicate that benthic primary consumers act as a gateway for riverine organic matter into the marine food web and that the structure of benthic fauna communities largely affects the ability and patterns of riverine Corg storage by benthos. Although benthic communities inhabiting the southern Baltic Sea show high plasticity of feeding behaviors (Szczepanek et al., 2021), the observed and predicted changes in the amount of riverine discharge (Christensen et al., 2022) and primary production patterns (Tamelander et al., 2017) may have profound consequences on the benthic community and food-web structure and thus the functioning of coastal riverine impacted ecosystems.
Data availability statement
The raw data supporting the conclusions of this article will be made available by the authors, without undue reservation.
Author contributions
MSz, MSi and MK conceptualized the design of the study. MSz, MSi and KK-M conducted filed and laboratory work. MSz analyzed data with contribution of KK-M. MSz wrote the original draft. All authors contributed substantially to the final version of the manuscript. MK acquired funding for this research. All authors contributed to the article and approved the submitted version.
Funding
This study was funded by Polish National Science Centre, project no 2017/26/E/NZ8/00496 (COmEBACk), and additional statutory IOPAN funding for r/v Oceania. MSz’s participation in this study was further supported by the Polish National Science Centre, project no 2020/37/N/NZ8/01418 (ISOFUN). KK-M’s participation in this study was further supported by the Foundation for Polish Science (FNP).
Acknowledgments
We would like to thank the captain and crew of r/v Oceania for help during sampling. Furthermore, we are grateful for help of Zuzanna Borawska and all undergraduate students during sampling and sample processing.
Conflict of interest
The authors declare that the research was conducted in the absence of any commercial or financial relationships that could be construed as a potential conflict of interest.
Publisher’s note
All claims expressed in this article are solely those of the authors and do not necessarily represent those of their affiliated organizations, or those of the publisher, the editors and the reviewers. Any product that may be evaluated in this article, or claim that may be made by its manufacturer, is not guaranteed or endorsed by the publisher.
Supplementary material
The Supplementary Material for this article can be found online at: https://www.frontiersin.org/articles/10.3389/fmars.2022.974539/full#supplementary-material
References
Antonio E. S., Kasai A., Ueno M., Won, N . il, Ishihi Y., Yokoyama H., et al. (2010). Spatial variation in organic matter utilization by benthic communities from yura river-estuary to offshore of tango Sea, Japan. Estuar. Coast. Shelf Sci. 86, 107–117. doi: 10.1016/j.ecss.2009.10.020
Arar E. J., Collins G. B. (1997). Method 445.0. In vitro determination of chlorophyll a and pheophytin a in marine and freshwater algae by fluorescence. (Washington, DC:U.S. Environmental Protection Agency).
Arbi I., Liu S., Zhang J., Wu Y., Huang X. (2018). Detection of terrigenous and marine organic matter flow into a eutrophic semi-enclosed bay by δ13C and δ15N of intertidal macrobenthos and basal food sources. Sci. Tot. Environ. 613–614, 847–860. doi: 10.1016/j.scitotenv.2017.09.143
Archambault M. C., Bricelj V. M., Grant J., Anderson D. M. (2004). Effects of suspended and sedimented clays on juvenile hard clams, mercenaria mercenaria, within the context of harmful algal bloom mitigation. Mar. Biol. 144, 553–565. doi: 10.1007/S00227-003-1222-5
Attrill M. J., Rundle S. D., Fraser A., Power M. (2009). Oligochaetes as a possible entry route for terrigenous organic carbon into estuarine benthic food webs. Mar. Ecol. Prog. Ser. 384, 147–157. doi: 10.3354/MEPS08019
Bartels P., Ask J., Andersson A., Karlsson J., Giesler R. (2018). Allochthonous organic matter supports benthic but not pelagic food webs in shallow coastal ecosystems. Ecosystems 21, 1459–1470. doi: 10.1007/s10021-018-0233-5
Blott S. J., Pye K. (2001). GRADISTAT: A grain size distribution and statistics package for the analysis of unconsolidated sediments. Earth Surf. Process. Landforms 26, 1237–1248. doi: 10.1002/esp.261
Brey T. (1991). Interactions in soft bottom benthic, communities: Quantitative aspects of behaviour in the surface deposit feeders Pygospio elegans (Polychaeta) and Macoma balthica (Bivalvia). Helgoländer Meeresunters 45, 301–316. doi: 10.1007/BF02365522
Careddu G., Costantini M. L., Calizza E., Carlino P., Bentivoglio F., Orlandi L., et al. (2015). Effects of terrestrial input on macrobenthic food webs of coastal sea are detected by stable isotope analysis in gaeta gulf. Estuar. Coast. Shelf Sci. 154, 158–168. doi: 10.1016/j.ecss.2015.01.013
Chanton J., Lewis F. G. (2002). Examination of coupling between primary and secondary production in a river-dominated estuary: Apalachicola bay, Florida, U.S.A. Limnol. Oceanogr. 47, 683–697. doi: 10.4319/LO.2002.47.3.0683
Christensen J. H., Christensen O. B. (2007). A summary of the PRUDENCE model projections of changes in European climate by the end of this century. Clim. Change 2007 81, 7–30. doi: 10.1007/S10584-006-9210-7
Christensen O. B., Kjellström E., Dieterich C., Gröger M., Meier H. E. M. (2022). Atmospheric regional climate projections for the Baltic Sea region until 2100. Earth Syst. Dyn. 13, 133–157. doi: 10.5194/ESD-13-133-2022
Compton T. J., Troost T. A., Drent J., Kraan C., Bocher P., Leyrer J., et al. (2009). Repeatable sediment associations of burrowing bivalves across six European tidal flat systems. Mar. Ecol. Prog. Ser. 382, 87–98. doi: 10.3354/MEPS07964
Cyberski J., Grześ M., Gutry-Korycka M., Nachlik E., Kundzewicz Z. W. (2006). History of floods on the river vistula. Hydrol. Sci. J. 51, 799–817. doi: 10.1623/hysj.51.5.799
Damrat M., Zaborska A., Zajączkowski M. (2013). Sedimentation from suspension and sediment accumulation rate in the river vistula prodelta, gulf of gdańsk (Baltic Sea). Oceanologia 55, 937–950. doi: 10.5697/oc.55-4.937
Darnaude A. M., Salen-Picard C., Harmelin-Vivien M. L. (2004). Depth variation in terrestrial particulate organic matter exploitation by marine coastal benthic communities off the Rhone river delta (NW Mediterranean). Mar. Ecol. Prog. Ser. 275, 47–57. doi: 10.3354/MEPS275047
Dauer D. M. (1997). Functional morphology and feeding behavior of Marenzelleria viridis (Polychaeta: Spionidae). Bull. Mar. Sci. 60, 512–516.
Deegan L. A., Garritt R. H. (1997). Evidence for spatial variability in estuarine food webs. Mar. Ecol. Prog. Ser. 147, 31–47. doi: 10.3354/meps147031
Dias E., Morais P., Cotter A. M., Antunes C., Hoffman J. C. (2016). Estuarine consumers utilize marine, estuarine and terrestrial organic matter and provide connectivity among these food webs. Mar. Ecol. Prog. Ser. 554, 21–34. doi: 10.3354/meps11794
Dunne J. P., Sarmiento J. L., Gnanadesikan A. (2007). A synthesis of global particle export from the surface ocean and cycling through the ocean interior and on the seafloor. Global Biogeochem. Cycles 21, 1–16. doi: 10.1029/2006GB002907
Dunton K. H., Schonberg S. V., Cooper L. W. (2012). Food web structure of the alaskan nearshore shelf and estuarine lagoons of the Beaufort Sea. Estuar. Coasts 35, 416–435. doi: 10.1007/S12237-012-9475-1
Emelyanov E. M., Stryuk V. L. (2002). Distribution of suspended matter. geology of the gdańsk basin. Baltic Sea (Kaliningrad: Yantarnyi skaz).
Fauchald K., Jumars P. A. (1979). The diet of worms: A study of polychaete feeding guilds. Oceanogr. Mar. Biol. Annu. Rev. 17, 193–284.
Fenchel T., Kofoed L. H. (1976). Evidence for exploitative interspecific competition in mud snails (Hydrobiidae). Oikos 27, 367. doi: 10.2307/3543455
Filgueira R., Strohmeier T., Strand Ø. (2018). “Regulating services of bivalve molluscs in the context of the carbon cycle and implications for ecosystem valuation,”. Smaal A., Ferreira J., Grant J, Petersen J, Strand Ø. (eds) Goods Serv. Mar. Bivalves, (Springer, Cham) 231–251. doi: 10.1007/978-3-319-96776-9_12
Folk R. L., Ward W. C. (1957). Brazos river bar [Texas]; A study in the significance of grain size parameters. J. Sediment. Res. 27, 3–26. doi: 10.1306/74D70646-2B21-11D7-8648000102C1865D
Gerasimova A. V., Filippova N. A., Lisitsyna K. N., Filippov A. A., Nikishina D. V., Maximovich N. V. (2019). Distribution and growth of bivalve molluscs Serripes groenlandicus (Mohr) and Macoma calcarea (Gmelin) in the Pechora Sea. Polar Biol. 42, 1685–1702. doi: 10.1007/S00300-019-02550-Z
Gic-Grusza G., Kryla-Straszewska L., Urbański J., Warzocha J., Węsławski J. M. (2009). Atlas of polish marine area bottom habitats. environmental valorization of marine habitats. Ed. Zgaińska D. (Gdynia: Institute of Oceanology, Polish Academy of Sciences).
Gilbert M. A. (1973). Growth rate, longevity and maximum size of Macoma balthica (L.). Biological Bulletin 145, 119–126. doi: 10.2307/1540352
Gogina M., Zettler M. L. (2010). Diversity and distribution of benthic macrofauna in the Baltic sea. data inventory and its use for species distribution modeling and prediction. J. Sea Res. 64, 313–321. doi: 10.1016/j.seares.2010.04.005
Gogina M., Zettler A., Zettler M. L. (2022). Weight-to-weight conversion factors for benthic macrofauna: Recent measurements from the Baltic and the North Seas. Earth Syst. Sci. Data 14, 1–4. doi: 10.5194/essd-14-1-2022
Grelowski A., Wojewódzki T. (1996). The impact of the Vistula River on the hydrological conditions in the Gulf of Gdansk in 1994. Bull. Sea Fish. Inst. 137, 23–33.
HELCOM (2018). “Input of nutrients by the seven biggest rivers in the Baltic Sea region,” in Baltic Sea Environment proceedings No.163. (Helsinki:Helsinki Commission)
Hoffman J. C., Bronk D. A., Olney J. E. (2008). Organic matter sources supporting lower food web production in the tidal freshwater portion of the York river estuary, Virginia. Estuar. Coasts 31, 898–911. doi: 10.1007/s12237-008-9073-4
Huxham M., Richards M. (2003). Can postlarval bivalves select sediment type during settlement? a field test with Macoma balthica (L.) and Cerastoderma edule (L.). J. Exp. Mar. Bio. Ecol. 288, 279–293. doi: 10.1016/S0022-0981(03)00023-6
Kamermans P., van der Veer H. W., Karczmarski L., Doeglas G. W. (1992). Competition in deposit- and suspension-feeding bivalves: Experiments in controlled outdoor environments. J. Exp. Mar. Bio. Ecol. 162, 113–135. doi: 10.1016/0022-0981(92)90127-V
Kedra M., Kuliński K., Walkusz W., Legezyńska J. (2012). The shallow benthic food web structure in the high Arctic does not follow seasonal changes in the surrounding environment. Estuar. Coast. Shelf Sci. 114, 183–191. doi: 10.1016/j.ecss.2012.08.015
Kube J. (1996). Spatial and temporal variations in the population structure of the soft-shell clam Mya arenaria in the pomeranian bay (southern Baltic sea). J. Sea Res. 35, 335–344. doi: 10.1016/S1385-1101(96)90760-1
Kubiak-Wójcicka K. (2020). Variability of air temperature, precipitation and outflows in the Vistula basin (Poland). Resour 9, 103. doi: 10.3390/RESOURCES9090103
Kuliński K., Kędra M., Legeżyńska J., Głuchowska M., Zaborska A. (2014). Particulate organic matter sinks and sources in high Arctic fjord. J. Mar. Syst. 139, 27–37. doi: 10.1016/j.jmarsys.2014.04.018
Kuliński K., Pempkowiak J. (2012). “Carbon cycling in the Baltic Sea,” in GeoPlanet (Berlin, Heidelberg: Springer Science and Business Media B.V). doi: 10.1007/978-3-642-19388-0
Lessin G., Bruggeman J., McNeill C. L., Widdicombe S. (2019). Time scales of benthic macrofaunal response to pelagic production differ between major feeding groups. Front. Mar. Sci. 6. doi: 10.3389/fmars.2019.00015
Levinton J. (1972). Stability and trophic structure in deposit-feeding and suspension-feeding communities. Am. Nat. 106, 472–486. doi: 10.1086/282788
Levinton J. (1989). “Deposit feeding and coastal oceanography,” in Ecology of marine deposit feeders. Eds. Lopez G., Taghon G., Levinton J. (New York: Springer-Verlag), 1–21.
Majewski W. (2013). General characteristics of the Vistula and its basin. Acta Energ. 2, 6–15. doi: 10.12736/issn.2300-3022.2013201
Maksymowska D., Richard P., Piekarek-Jankowska H., Riera P. (2000). Chemical and isotopic composition of the organic matter sources in the Gulf of Gdańsk (Southern Baltic Sea). Estuar. Coast. Shelf Sci. 51, 585–598. doi: 10.1006/ecss.2000.0701
Matciak M., Nowacki J. (1995). The Vistula River discharge front - surface observations. Oceanologia 37, 75–88.
McGovern M., Poste A. E., Oug E., Renaud P. E., Trannum H. C. (2020). Riverine impacts on benthic biodiversity and functional traits: A comparison of two sub-Arctic fjords. Estuar. Coast. Shelf Sci. 240, 106774. doi: 10.1016/j.ecss.2020.106774
Middelburg J. J. (2018). Reviews and syntheses: to the bottom of carbon processing at the seafloor. Biogeosciences 15, 413–427. doi: 10.5194/bg-15-413-2018
Nielsen M. V., Kofoed L. H. (1982). Selective feeding and epipsammic browsing by the deposit-feeding amphipod Corophium volutator. Mar. Ecol. Prog. Ser. 10, 81–88. doi: 10.3354/MEPS010081
Nurkse K., Kotta J., Orav-Kotta H., Ojaveer H. (2016). A successful non-native predator, round goby, in the Baltic Sea: Generalist feeding strategy, diverse diet and high prey consumption. Hydrobiologia 777, 271–281. doi: 10.1007/S10750-016-2795-6
Ochocki S., Nakonieczny J., Chmielowski H., Zalewski M. (1995). The hydrochemical and biological impact of the river vistula on the pelagic system of the gulf of gdansk in 1994. part 2. primary production and chlorophyll a. Oceanologia 37, 207–226.
Ólafsson E. B. (1986). Density dependence in suspension-feeding and deposit-feeding populations of the bivalve Macoma balthica: A field experiment. J. Anim. Ecol. 55, 517. doi: 10.2307/4735
Parnell A. C., Phillips D. L., Bearhop S., Semmens B. X., Ward E. J., Moore J. W., et al. (2013). Bayesian Stable isotope mixing models. Environmetrics 24, 387–399. doi: 10.1002/ENV.2221
Peller T., Andrews S., Leroux S. J., Guichard F. (2020). From marine metacommunities to meta-ecosystems: Examining the nature, scale and significance of resource flows in benthic marine environments. Ecosystems, 24, 1–14. doi: 10.1007/s10021-020-00580-x
Peterson B. J. (1999). Stable isotopes as tracers of organic matter input and transfer in benthic food webs: A review. Acta Oecologica 20, 479–487. doi: 10.1016/S1146-609X(99)00120-4
Phillips D. L., Inger R., Bearhop S., Jackson A. L., Moore J. W., Parnell A. C., et al. (2014). Best practices for use of stable isotope mixing models in food-web studies. Can. ournal Zool. 92, 823–835. doi: 10.1139/cjz-2014-0127
Phillips D. L., Newsome S. D., Gregg J. W. (2005). Combining sources in stable isotope mixing models: Alternative methods. Oecologia 144, 520–527. doi: 10.1007/s00442-004-1816-8
Polis G. A., Anderson W. B., Holt R. D. (1997). Toward an integration of landscape and food web ecology: the dynamics of spatially subsidized food webs. Annu. Rev. Ecol. Syst. 28, 289–316. doi: 10.1146/annurev.ecolsys.28.1.289
Premke K., Karlsson J., Steger K., Gudasz C., Von Wachenfeldt E., Tranvik L. J. (2015). Stable isotope analysis of benthic fauna and their food sources in boreal lakes. J. North Am. Benthol. Soc 29, 1339–1348. doi: 10.1899/10-002.1
R Core Team (2021) R: A language and environment for statistical computing. Available at: https://www.r-project.org/.
Rumohr H., Brey T., Ankar S. (1987). A compilation of biometric conversion factors for benthic invertebrates of the Baltic Sea. Balt. Mar. Biol. 9, 1–56.
Silberberger M. J., Koziorowska-Makuch K., Kuliński K., Kędra M. (2021). Stable isotope mixing models are biased by the choice of sample preservation and pre-treatment: Implications for studies of aquatic food webs. Front. Mar. Sci. 7. doi: 10.3389/fmars.2020.621978
Silberberger M. J., Renaud P. E., Kröncke I., Reiss H. (2018). Food-web structure in four locations along the European shelf indicates spatial differences in ecosystem functioning. Front. Mar. Sci. 5. doi: 10.3389/fmars.2018.00119
Snelgrove P. V. R., Butman C. A. (1994). “Animal-sediment relationships revisited: cause versus effect,” in Oceanography and marine biology: An annual review (London: UCL Press), 111–178.
Snelgrove P. V. R., Soetaert K., Solan M., Thrush S., Wei C. L., Danovaro R., et al. (2018). Global carbon cycling on a heterogeneous seafloor. Trends Ecol. Evol. 33, 96–105. doi: 10.1016/j.tree.2017.11.004
Stock B. C., Jackson A. L., Ward E. J., Parnell A. C., Phillips D. L., Semmens B. X. (2018). Analyzing mixing systems using a new generation of Bayesian tracer mixing models. PeerJ 6, e5096. doi: 10.7717/peerj.5096
Stock B. C., Semmens B. X. (2016) MixSIAR GUI user manual. version 3.1. Available at: https://github.com/brianstock/MixSIAR.
Szczepanek M., Silberberger M. J., Koziorowska-makuch K., Nobili E., Monika K. (2021). The response of coastal macrobenthic food-web structure to seasonal and regional variability in organic matter properties. Ecol. Indic. 132, 1–21. doi: 10.1016/j.ecolind.2021.108326
Tamelander T., Spilling K., Winder M. (2017). Organic matter export to the seafloor in the Baltic Sea: Drivers of change and future projections. Ambio 46, 842–851. doi: 10.1007/s13280-017-0930-x
Thoms F., Burmeister C., Dippner J. W., Gogina M., Janas U., Kendzierska H., et al. (2018). Impact of macrofaunal communities on the coastal filter function in the bay of gdansk, Baltic Sea. Front. Mar. Sci. 5. doi: 10.3389/fmars.2018.00201
Thornton S. F., McManus J. (1994). Application of organic carbon and nitrogen stable isotope and C/N ratios as source indicators of organic matter provenance in estuarine systems: Evidence from the Tay estuary, Scotland. Estuar. Coast. Shelf Sci. 38, 219–233. doi: 10.1006/ecss.1994.1015
Thrush S. F., Hewitt J. E., Norkko A., Nicholls P. E., Funnell G. A., Ellis J. I. (2003). Habitat change in estuaries: Predicting broad-scale responses of intertidal macrofauna to sediment mud content. Mar. Ecol. Prog. Ser. 263, 101–112. doi: 10.3354/MEPS263101
Valiela I., Bartholomew M. (2015). Land–Sea coupling and global-driven forcing: Following some of Scott nixon’s challenges. Estuar. Coasts 38, 1189–1201. doi: 10.1007/S12237-014-9808-3
Viitasalo M., Bonsdorff E. (2022). Global climate change and the Baltic Sea ecosystem: direct and indirect effects on species, communities and ecosystem functioning. Earth Syst. Dyn. Discuss., 13, 1–38. doi: 10.5194/esd-2021-73
Vinagre C., Madeira C., Dias M., Narciso L., Mendonça V. (2019). Reliance of coastal intertidal food webs on river input – current and future perspectives. Ecol. Indic. 101, 632–639. doi: 10.1016/j.ecolind.2019.01.064
Voss M., Liskow I., Pastuszak M., Rüæ D., Schulte U., Dippner J. W. (2005). Riverine discharge into a coastal bay: A stable isotope study in the Gulf of Gdańsk, Baltic Sea. J. Mar. Syst. 57, 127–145. doi: 10.1016/j.jmarsys.2005.04.002
Keywords: food webs, δ13C, δ15N, primary consumers, stable isotope mixing models, biomass-weighted resource utilization, Baltic Sea, Vistula River
Citation: Szczepanek M, Silberberger MJ, Koziorowska-Makuch K and Kędra M (2022) Utilization of riverine organic matter by macrobenthic communities in a temperate prodelta. Front. Mar. Sci. 9:974539. doi: 10.3389/fmars.2022.974539
Received: 21 June 2022; Accepted: 22 September 2022;
Published: 17 October 2022.
Edited by:
Jesper H. Andersen, NIVA Denmark Water Research, DenmarkReviewed by:
Laura Uusitalo, Finnish Environment Institute (SYKE), FinlandTorsten Berg, MariLim, Germany
Copyright © 2022 Szczepanek, Silberberger, Koziorowska-Makuch and Kędra. This is an open-access article distributed under the terms of the Creative Commons Attribution License (CC BY). The use, distribution or reproduction in other forums is permitted, provided the original author(s) and the copyright owner(s) are credited and that the original publication in this journal is cited, in accordance with accepted academic practice. No use, distribution or reproduction is permitted which does not comply with these terms.
*Correspondence: Marta Szczepanek, c2xvbWluc2thQGlvcGFuLnBs