- College of Earth Science and Engineering, Shandong University of Science and Technology, Qingdao, China
Tóthian theory refers to the gravity driven groundwater flow system (GFS) theory represented by Tóth, which mainly expounds the driving and distribution law of groundwater. The establishment and development of this theory not only deepened people’s understanding of the driving and distribution law of groundwater, but also greatly promoted the study of groundwater chemical evolution (GCE). Modern GCE research is mostly based on Tóthian theory, characterized by combining with advanced scientific and technological means. Based on the clue of time, this paper is divided into two parts. The first part mainly summarizes the establishment and development of Tóthian theory, including the exploration of groundwater driving force and distribution form by hydrogeologists before Tóthian theory, and the enrichment, development and application of Tóthian theory by geologists after its establishment. The second part mainly combs the main theories and application progress of GCE mechanism research, including the main theories and findings of GCE research before the emergence of Tóthian theory, as well as the research progresses of GCE after the emergence of Tóthian theory. With the flow of groundwater in GFS, groundwater undergoes continuous chemical evolution, which eventually leads to the transformation of hydrochemical types and the gradual increase of total dissolved solids (TDS). The distribution of GFS and GCE complement each other. The distribution of GFS directly determines the model of GCE, and the results of GCE also play a certain role in the distribution of GFS. GCE mainly includes dissolution, precipitation, cation exchange and adsorption, which is affected by the physical and chemical conditions of permeable media, organic matter content and microorganisms. GCE has the characteristics of universality, sustainability and diversity. With the increasing global population and the progresses of science and technology, the impact of human life, industrial and agricultural production on groundwater is deepening. The aggravation of pollution directly changes the chemical compositions of groundwater, resulting in changes of the law of GCE.
Introduction
Groundwater, a complex solution containing more than 80 elements, is an important geological force, which is widely involved in geological processes such as karst, sedimentation, diagenesis, metamorphism and mineralization (Zhang et al., 2018). Driven by gravity, density difference and heat, groundwater continuously reacts with the surrounding environment to complete the transportation and distribution of materials and energy, and its chemical properties continue to evolve (Tóth, 2009; Jiang et al., 2011; Zhang et al., 2015; Luan et al., 2017; Zhang et al., 2018; Meng, 2021; Zhao et al., 2021). According to Tóthian theory, the distribution pattern of gravity driven GFS is mainly controlled by the terrain. Regional GFS has multi-level nested systems, i.e., local-GFS, intermediate-GFS and regional-GFS. It is recognized that regional-GFS is the reason for the diversity of geological processes (Tóth, 1999; Jiang et al., 2010; Judit and Tóth, 2015; Jia et al., 2020). The discovery of Tóthian theory makes people master the general law of GFS. The combination of Tóthian theory and groundwater evolution research greatly promotes the study of GCE, which makes it possible to reveal the general law of GCE.
The long-term circulation makes the main components of groundwater controlled by the stratum. Under natural conditions, the main anions are bicarbonate (), sulfate (), chloride ion (Cl−) and carbonate (), and the main cations are calcium (Ca2+ n), magnesium (Mg2+), sodium (Na+) and potassium (K+) (Liang et al., 2012; Ren et al., 2014; Zhou et al., 2014; Zhang et al., 2018; Gao et al., 2020; Jia et al., 2020). Combined with the theory of GFS, the TDS of groundwater generally increases from the recharge area (RA) to the discharge area (DA), and the hydrochemical types often show horizontal zoning, gradually transforming from to , and eventually evolve to Cl−−Na+ (Hao et al., 2020; Wang et al., 2021a; Zhao et al., 2021). In some areas with special geological environment, the chemical evolution of groundwater deviates from the general law. For example, due to the different geological backgrounds of each region, the phenomenon of element process in groundwater in some areas leads to various endemic diseases, such as Sri Lanka, India, the United States and China (Gao et al., 2014; Kaur et al., 2019; Imbulana et al., 2020).
With the gradual expansion of the impact of human activities on nature, human activities continue to change the surrounding environment of groundwater, leading to obvious man-made interference characteristics in the chemical evolution of groundwater, resulting in obvious changes in the chemical components of groundwater. Such as nitrate () is added to the main ions in groundwater, the content of heavy metals in groundwater in some areas exceeds the background values, arsenic plumbum pollution is spread worldwide, and the change of chemical compositions of groundwater poses a threat to human health to a certain extent (Gao et al., 2014a; Nan et al., 2008; Sinha and Prasad, 2020; Paolalsiam and Mohan, 2021; Wang et al., 2021b; Xiao et al., 2021; Gao et al., 2022). In addition, with the development of industry and the growth of population, the natural recharge of groundwater is insufficient to meet people’s increasing demand for groundwater, the natural balance of groundwater is broken, and overexploitation leads to a series of problems such as land subsidence, ground fissures, land collapse, seawater intrusion and other problems (Zhang and Zhang, 2019).
Although the research on the chemical evolution of groundwater combined with Tóthian theory has achieved rich results which were widely recognized, the distribution law and chemical evolution law of groundwater have changed significantly under the influence of human activities. In the context of today’s industrial revolution, combined with Tóthian theory and human activities, mining the new laws of groundwater distribution and GCE under the influence of human activities can deepen our understanding of the diversity of geological processes, explain the chemical change process of groundwater more comprehensively and objectively, and better reveal the interaction relationship between groundwater and environment.
This paper fully integrates the relevant literatures, aiming at tracing the development and reform of the theory, focusing on combing the typical literature that can reflect the process of theoretical development, and analyzing the development of GCE theory and the main ways of GCE.
Establishment and development of Tóthian theory
Tóthian theory is the theory of gravity driven GFS firstly proposed by József Tóth, a Canadian hydrogeologist. Once published, it has been widely concerned. After the improvement and development of many hydrogeologists, it plays a leading role in hydrogeology. The theory was initially called “Groundwater multi-level nesting theory” (Tóth, 1963), and later evolved into “Gravity driven groundwater flow system theory” (Tóth, 2009). Finally, it was summarized as Tóthian theory in his article “The evolutionary concepts and practical utilization of the Tóthian Theory of regional groundwater flow”, published in 2016 (Tóth, 2016). This section mainly reviews the theoretical establishment and development of Tóthian theory.
Establishment of Tóthian theory
The GFS theory proposed by Tóthian comes from a large number of previous scientific researches. The main classical groundwater researches before Tóthian theory are shown in Table 1. In 1738, Daniel Bernoulli discovered the “Bernoulli theorem”, which had been widely used in hydraulic research. It was defined as that the total head of groundwater is the sum of its position head, pressure head and velocity head, and groundwater always flows from the position with high total head to the place with low total head (Maranzoni, 2020). In 1856, French hydraulic scientist Darcy found that the seepage velocity is directly proportional to the power of hydraulic gradient based on the concept of head, which is called Darcy’s Law (Darcy, 1857). In 1885, Chamberlin pointed out that the topographic elevation difference provided a driving force for groundwater flow (Chamberlin, 1885). King put forward the concept of gravity driven groundwater in 1899 (Figure 1A) (Cederstrom, 1946; Gao et al., 2014b), and Fourmarier proposed the concept of multistage GFS in 1939 (Figure 1b) (Zhang et al., 2015). Meinzer, Meinzer and Wenzel respectively discovered the three-dimensional properties of groundwater overflow and hydraulic gradient in 1936 and 1940 (Meinzer, 1936; Meinzer and Wenzel, 1940). Hubbert (1940) found that the driving force of groundwater was the negative value of the first derivative of fluid potential through Darcy’s law, and drew an approximate flow model of homogeneous permeable medium between gas-water interface potential source and valley potential sink - inter River block network flow diagram (Figure 1C) (Hubbert, 1940). In 1962, Tóth found the defects of Hubbert flow model in the actual hydrogeological work. Combined with the general relationship between piezometric tube surface and terrain in the same well depth in the same rock unit, he applied Laplace equation to solve the drainage problems of simple basin (Tóth, 1962). After continuous correction and improvement, he finally put forward the theory of “Nested multistage flow system” in 1963 (Figure 1D) (Tóth, 1963). Tóth believed that under the control of specific physical, geographical and geological conditions, the groundwater in the basin presented a multi-level flow mode (composed of local-GFS, intermediate-GFS and regional -GFS). In each GFS, from the supply source to the discharge sink, the flow changes from downward movement to horizontal movement, and then upward movement (Tóth, 1962; Tóth, 1963; Zhang et al., 2018).
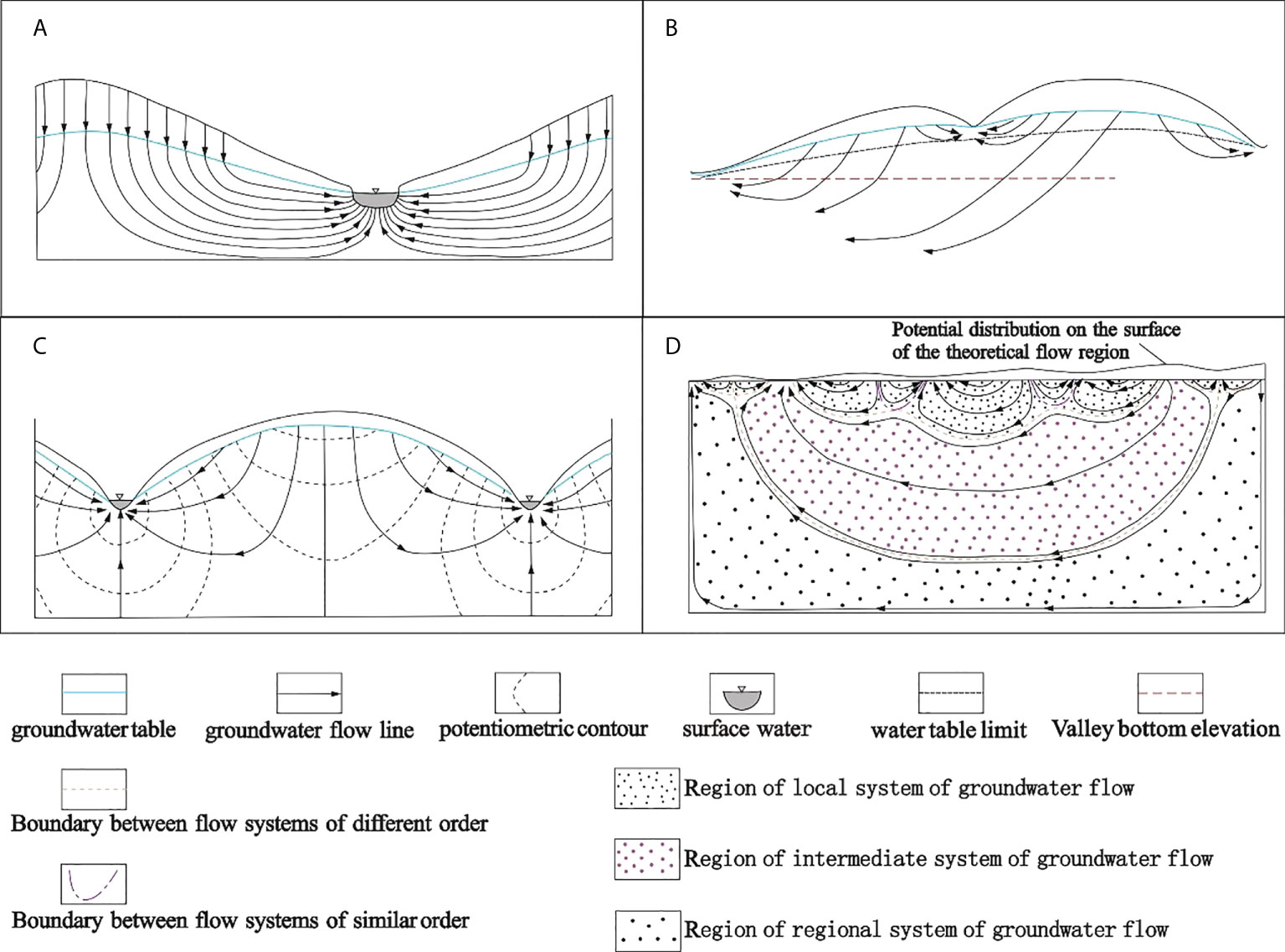
Figure 1 The evolution diagram of Tóthian Theory (A) The cross-section drawn of the gravity-driven groundwater flow by King in 1899; (B) A pattern of complex flow in unconfined aquifer by Fourmarier in 1939; (C) The groundwater flow net diagram of rivers block by Hubbert in 1940; (D) The use of upper head boundary for linear function and sine function superimposed conditions to conduct simulated groundwater flow field by Toth in 1963.
Development of Tóthian theory
Since 1961, Tóth has been committed to the research of GFS and continuously promoted Tóthian theory. The improvement of GFS and its environmental effects are shown in Figure 2. In 2009, Tóth systematically combed his scientific researches of nearly half a century and published the book “Graphic Systems of Ground Water Flow” (Tóth, 2009). During this period, Tóth’s achievements in gravity driven GFS could be briefly summarized as follows: (1) the main driving force of groundwater is gravity. The gravity penetration theory is proposed to expand the application scope of GFS theory from homogeneous small basins to heterogeneous large basins (Tóth, 1978; Tóth, 1979; Tóth, 1980; Tóth, 1995); (2) Reveal the role of GFS in material transport, hydrochemical evolution and thermal evolution (Tóth, 1966a; Tóth, 1984; Tóth, 1996); (3) It is considered that groundwater is an ubiquitous geological agent and the main reason for geological diversity (Tóth, 1971; Tóth, 1999; Tóth, 2016); (4) It promotes the application of GFS theory in practical work (Tóth, 1966b; Tóth, 1977; Tóth and Corbet, 1986).
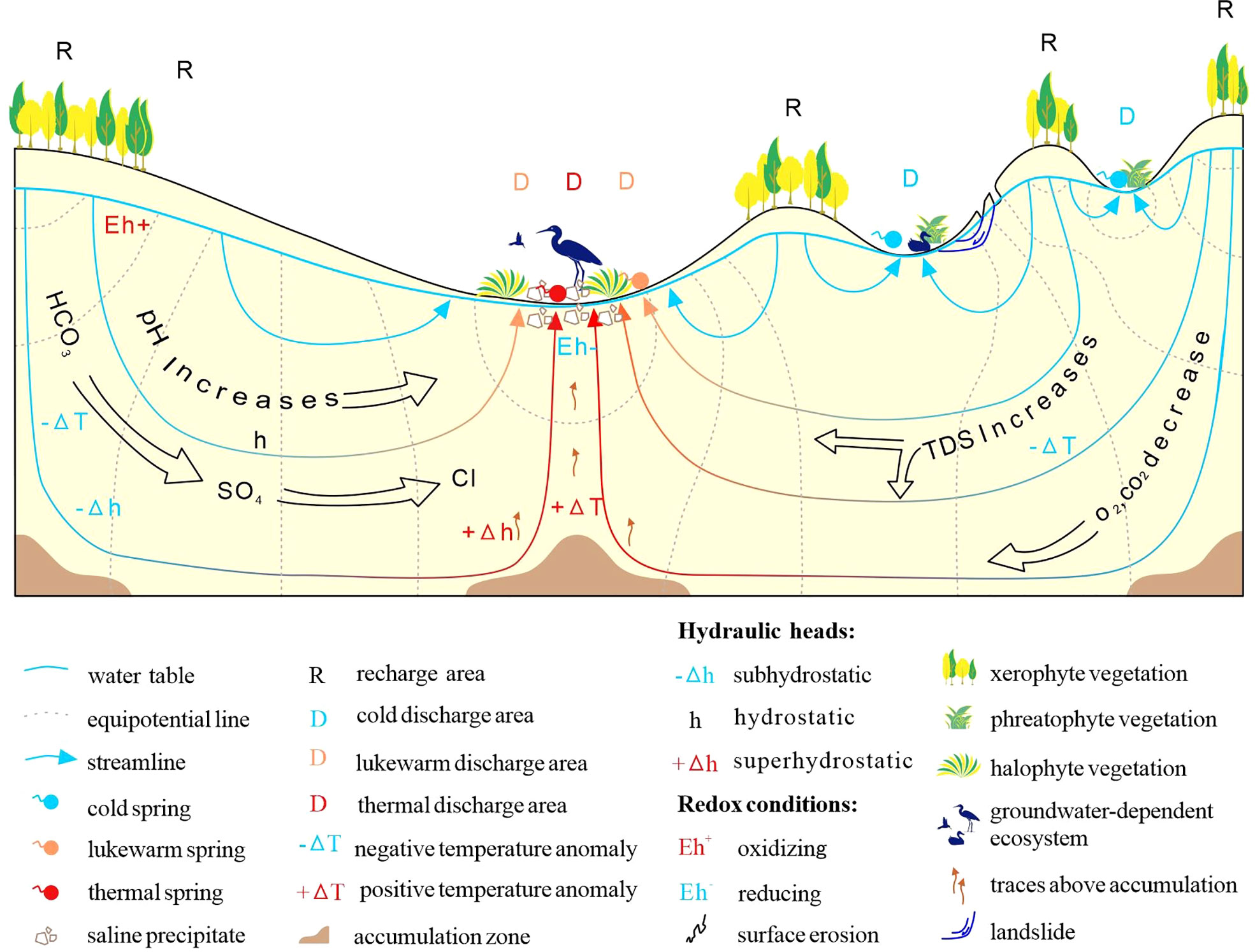
Figure 2 Natural conditions and phenomena due to environmental agency of flowing groundwater in drainage basin (modified after Tóth, 1999 and Tóth, 2016).
In addition to Tóth himself, other hydrogeological scholars have made continuous exploration based on Tóthian theory, which played an important role in the enrichment, development and promotion of the theory. Among them, the representative figures are Freeze and Witherspoon, Maclay, Jay, Schwartz, Engelen, Allen, Zijl, etc. Freeze and Witherspoon (1966;1967) extended the application scope of Tóthian theory from special cases to general cases through numerical methods, and explored the influence of water level and permeability coefficient on groundwater flow mode (Freeze and Witherspoon, 1966; Freeze and Witherspoon, 1967). Maclay and Winter (1967) combined Tóthian theory with the study of GCE. They found that the relative concentration of major ions in groundwater changed regularly from RA to DA. They believed that the combination of Tóthian theory and hydrochemical research could make people better understand the relationship between water quality and groundwater movement (Maclay and Winter, 1967). Lehr was one of early hydrogeological experts to carry out the indoor observation experiment of GFS. He used plexiglass to build a transparent box filled with sand and resin materials, simulated sandstone to build a hydraulic model, and clearly observed two main phenomena in the movement of groundwater: First, groundwater always moves from high head to low head and the second is the characteristics of layered movement of groundwater (Lehr, 1968). Schwartz and Domenico (1973) put forward the conceptual model of solute transport in GFS in combination with Tóthian theory, and summarized the law of groundwater evolution involved in the processes of groundwater flow (Schwartz and Domenico, 1973). Engelen and Jones (1986) participated in the compilation of the book “Developments in the Analysis of Ground Water Flow Systems” published by the International Association of Hydrological Sciences (Engelen and Jones, 1986). Later, Engelen and Kloosterman (1996) presided over the compilation of the book “Introduction to flow systems”. The publication of these two books not only affirms Tóthian theory, but also extends Tóthian theory to a wider world to a great extent (Engelen and Kloosterman, 1996). In 1993, Allen et al. explored the movement law of groundwater in convergent aquifer, and the main conclusions obtained are basically the same as Tóthian theory, which further confirms the applicability of Tóthian theory (Allen T. Hjelmfelt and Pi, 1993). Zijl (1999) explored the temporal and spatial problems of GFS theory and found that Tóthian theory was also very useful in analyzing the time and space of GFS and their relationship (Zijl, 1999). Jiang et al. (2010) found that due to the attenuation of hydraulic conductivity and porosity depth, the renewal and aging of groundwater in the basin exist at the same time (Jiang et al., 2010). Tóth (2016) combed the development of groundwater flow evolution theory and believed that the current GFS had broken the traditional concept of aquifer, and most of the current GFS were non-confined cross stratum flow systems (Tóth, 2016). Zhang et al. (2020a) found that the change of salinity in groundwater DA would change the position of stagnation point in GFS, thus changing the migration law of groundwater flow (Zhang et al., 2020a). With the maturity of GFS theory, people actively apply Tóthian theory to practical hydrogeological work. For example, people in countries such as Canada, the United States, China and Japan have widely used GFS theory in hydrogeological survey and mapping, water source and sewage discharge site selection, ecosystem protection and so on; and fruitful theoretical and practical results were achieved (Cloutier et al., 2006; Palmer et al., 2006; Han et al., 2009; Tóth, 2009; Lei et al., 2012; Zhang et al., 2015; Ono et al., 2019). By means of isotopes, it was found that there was not only a recharge relationship between surface water and groundwater, but also a GFS at the bottom of the ocean (Krall et al., 2017; Xu et al., 2018b; Danish et al., 2020). This discovery further promoted the application of GFS to a broader world.
In China, Tóthian theory has also been widely concerned and applied. Chen introduced Tóthian theory into China (Chen, 1987). Wang briefly reviewed the development of Tóthian theory in the Book “Fundamentals of Hydrogeology” and fully affirmed the significance of Tóthian theory in the history of hydrogeology (Wang, 1995). Later, the book “Fundamentals of Hydrogeology” compiled by Zhang et al. fully drew lessons from Tóthian theory (Zhang et al., 2018). The book of Zhang et al. also includes the multi-level GFS observed by Liang through the GFS demonstrator (Liang et al., 2010). Based on Tóthian theory, Jiang et al. (2009; 2011; 2012b) explored the relationship between permeability coefficient and depth, the distribution law of stagnation points in GFS, the spatial division characteristics of groundwater age, etc. It was found that the permeability coefficient in regional GFS decreased exponentially with the increasing depth, which would affect the distribution of stagnation points (Jiang et al., 2009; Jiang et al., 2011; Jiang et al., 2012b). Gao et al. (2013; 2014b; 2014c) successfully observed the multistage distribution of GFS, the refraction of groundwater in aquifers with different permeabilities and the driving of heat to groundwater through simple sand trough experiment (Gao, 2013; Gao et al., 2014b; Gao et al., 2014c). In 2015, Zhang et al. translated and published Tóth’s book “Gravitational Systems of Groundwater Flow”, which greatly improved the systematic dissemination of Tóthian theory in China (Zhang et al., 2015). Zhao et al. (2008) and Zhang et al. (2017) respectively investigated the GFS in Ordos Basin and they found that the regional - GFS was consistent with the GFS of Tóthian theory, which provides an excellent example for the research of Tóthian theory and is of great value to the research and practical application of Tóthian theory (Zhao et al., 2008; Zhang et al., 2017). Wang et al. (2017) proposed three-dimensional groundwater circulation units and combined these units into a three-dimensional GFS to obtain the fine structure characteristics of groundwater circulation (Wang et al., 2017).
Tóthian theory breaks through the concept of aquifer and makes people realize that there is no absolute water resisting body (layer) in nature. In the huge structural unit (groundwater aquifer system or hydrogeological unit), driven by the potential energy given by the terrain of the RA and the energy difference between it and the discharge point (place), the continuously moving groundwater flow runs in all directions of releasing energy, with low resistance and smooth flow speed. The gradient of potential energy reduction is large and so is the energy release, and vice versa (Gao et al., 2014b; Tóth, 1970; Tóth, 1978; Winter et al., 1998; Li and Hao, 1999; Tóth, 1999; Tóth, 2009; Liang et al., 2010; Jiang et al., 2011; Wang et al., 2011; Jiang et al., 2012a; Liang et al., 2013). If we combine the concept of GFS with hydrochemical methods, we can better understand the relationship between groundwater quality and movement, and better study the mechanism of GCE.
Chemical evolution of groundwater
Theoretical progress of groundwater chemical evolution
The theoretical study of GCE can be divided into two stages according to the establishment of Tóthian theory. The first stage is the study of GCE independent from Tóthian theory before 1963; The second stage is the study of GCE combined with Tóthian theory after 1963.
Before the emergence of Tóthian theory, the research on the chemical evolution of groundwater was generally limited to the aquifer, but a series of remarkable achievements were still made. For example, as early as the 1860s, geochemists established the basic principle of thermodynamic equilibrium that could be used to distinguish the types of natural water bodies (Glynn and Plummer, 2005). Early scientists believed that groundwater was partially balanced, and pH and redox conditions could reflect the ionic composition, mineral reaction and water stability of water (Korzhinskii, 1936; Krumbein and Garrels, 1952). There are many chemical classification methods for groundwater. In 1934, Schukarev took six main components in water as the basis of classification and proposed the classification method of natural water chemical analysis data, which is the most widely used classification method at present (Zhang and Zhang, 2019). In order to more conveniently represent the chemical types and characteristics of groundwater, Piper three-line diagram (1944) and Durov diagram (1948) appeared one after another. These two hydrochemical diagrams are still widely used in today’s hydrochemical research. Chadha (1999) proposed a rectangular chart that could be directly classified by Excel based on the above two hydrochemical charts (Chadha, 1999). The replacement order of cations is basically determined. Magnesium is (Mg2+ ) preferentially replaced in water containing calcium (Ca2+ ) and Mg2+ The order of relative replaceability of cations in clay is as follows: Li+>Na+>H+>K+>Mg2+>Ca2+ (Kelly, 1934; Ross, 1943). Cederstrom (1946) established a groundwater chemical model with the concept of aquifer (Cederstrom, 1946). It was found that the mineralization of groundwater in different aquifers decreased with the increase of depth. At the same time, combined with the sampling and analysis data, the groundwater near the coastal plain was divided into four zones from west to east: descending zone, hard water zone, soft water zone and high chlorine zone (Cederstrom, 1946). Back et al. (1960) proposed the concept of hydrochemical facies based on aquifer system to describe the main ions in groundwater. It was found that in shallow sediments of the North Atlantic coastal plain, the distribution of was wider than and Cl−, whereas was dominant in deeper sediments (Back, 1960; Back and Hanshaw, 1971b). In the 1950s, the study of groundwater isotopes began to show clues. Munnich and Vogel (1959) used the 14C method to determine the age of groundwater (Munnich and Vogel, 1959). Craig (1961) established the global atmospheric precipitation linear equation δD=8δ18O+10 to trace the source of groundwater (Craig, 1961). During this period, great progress were made in the theoretical research of GCE and many of them are still used today. However, the research on GCE is still limited, and the exploration of GCE law in different stages is insufficient.
The emergence of Tóthian theory has greatly promoted the development of GCE. For example, Maclay and Winter (1967) found that the evolution sequence of main hydrochemical types of groundwater from RA to runoff area to DA: (Maclay and Winter, 1967). Based on Tóthian Theory, Schwartz and Domenico (1973) proposed a conceptual model of solute transport in GFS, which could be defined as: the substance enters the GFS in the RA, then completes the transportation and distribution, and finally discharges in the DA; The chemical and biological effects continuously modify the dissolved substance throughout the process (as shown in Figure 3) (Schwartz and Domenico, 1973). Thorstenson put forward the concept of redox and its application in geochemistry; Then the sequence of redox reactions in GFS began to be studied (Thorstenson, 1984). Wang (1995) clearly distinguished the two concepts of aquifer system and GFS in his research, within which an ideal model diagram was proposed based on the hydrochemical evolution of GFS (as shown in Figure 4) (Wang, 1995). Due to the rapid development of computer technology, hydrogeochemical simulation based on GFS originated in the 1960s developed continuously, which played an important role in revealing the chemical evolution of groundwater. For example, the granite model proposed by Garrels and Mackenzi (1967) laid the foundation for hydrogeochemical simulation (Garrels and Mackenzie, 1967). In 1971, Back and Hanshaw (1971a) pointed out that the application of the basic principle of irreversible thermodynamics in GFS provided a theoretical basis for the construction of GCE and head distribution prediction model (Back and Hanshaw, 1971a). With the improvement of calculation level, PHREEQC began to be widely used in mass balance calculation based on hydrogen and oxygen in 1990 (Wolery et al., 1990). The technology of computer greatly promoted the research of GCE theory, and provided a new research tool for this theoretical research. In particular, the emergence of hydrochemical simulation promoted the hydrochemical research from summarizing the current situations and laws to predicting gradually.
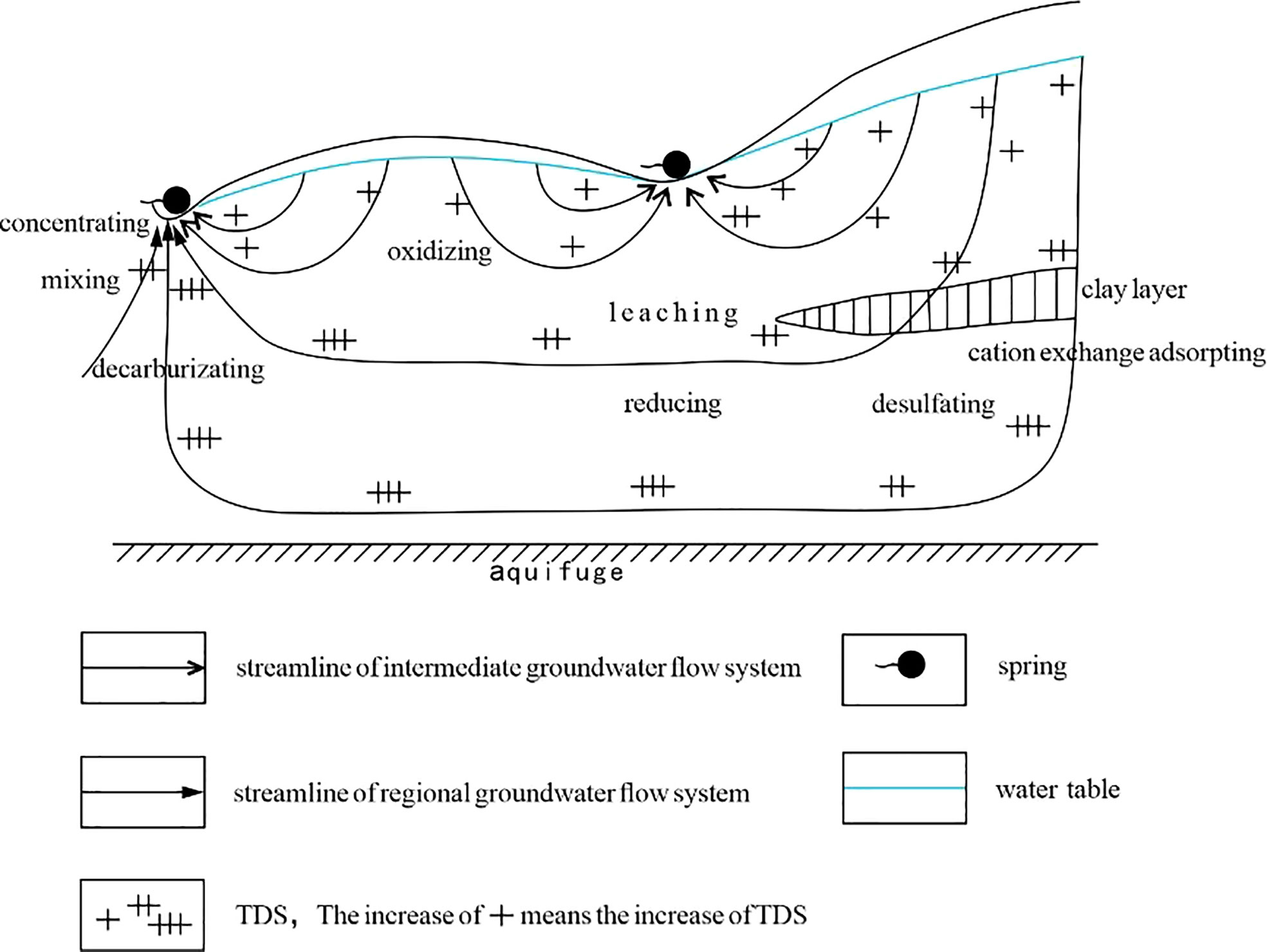
Figure 4 An ideal model for hydrochemical evolution of groundwater flow system (modified after Wang, 1995).
Main processes of groundwater chemical evolution based on Tóthian theory
In the local GFS which was widely distributed in the shallow part of the surface, the groundwater actively participating in the modern water cycle is discharged to the surface through short-distance, shallow burial and short-time runoff, and its hydrochemical type remains unchanged in bicarbonate type, mostly fresh water with low TDS. However, the groundwater in the regional GFS needs to go through runoff with long distance, deep burial and long time, and the hydrochemical type of groundwater gradually evolves. For example, the transformation from type to type and then to Cl− type mainly goes through certain evolution paths (as shown in Figure 5) (Li, 1991; Liang et al., 1991; Tóth, 1999; Chen et al., 2010; Liu et al., 2010; Jiang, 2013).
The chemical types of groundwater change with time, and vary from place to place. It is generally considered that groundwater experiences such actions as ion alternating adsorption, precipitation and dissolution of salt substances, microorganisms such as desulfurization bacteria and nitrifying bacteria participate in the action, and groundwater undergoes redox reaction, etc. (Gao et al., 2019; Chen et al., 2020a; Gao et al., 2020; Xia et al., 2020). The main evolution form of cations in groundwater are shown in Figure 6. In the RA, atmospheric precipitation enters the GFS through the aeration zone. In this process, leaching first occurs. Ca2+, Mg2+, Na+ in soil or rock are dissolved into groundwater. Due to the low content of CO2 in the RA and high content of TDS in atmospheric precipitation in the RA, the dissolution of Ca2+, Mg2+ in this period is greater than Na+. In the runoff area, with the migration of groundwater, TDS continues to increase and the content of CO2 decreases, along with the decrease of the solubility of Ca2+ and Mg2+, and the solubility of Na+ changes little. At the same time, Ca2+. and Mg2+ in groundwater exchange and adsorb with Na+ in surrounding geological bodies, so that the content of Ca2+ and Mg2+ in groundwater in the runoff area can either be greater or smaller than Na+. The TDS of groundwater in the DA continues to increase, the water pressure decreases during the discharge process, Ca2+ and Mg2+ precipitate, and Na+ continues to dissolve. Finally, the Na+ content in groundwar is greater than Ca2+ and Mg2+ (Ren et al., 2014; Wang, 1995; Zhang et al., 2015).
The evolution form of anions in GFS can be shown in Figure 7. In the local GFS, the groundwater actively participating in the modern water cycle is discharged to the surface through short-distance, shallow burial and short-time runoff, and its hydrochemical type remains unchanged in type, characterized by low TDS of most fresh water. However, the groundwater in the regional GFS needs to go through long-distance, deep burial and long-time runoff, and the hydrochemical type of groundwater gradually evolves from type to type and then to Cl− type (Wang, 1995; Ren et al., 2014; Zhang et al., 2018). When the RA is dominated by limestone and dolomite, the main chemical reactions are (Zhang et al., 2018):
When the RA is dominated by albite and calciclase, the main chemical reactions are:
Main chemical reactions in RA:
Main chemical reactions in the DA:
New theories and methods of groundwater chemical evolution
In the current situation of increasingly serious water pollution caused by the development of industry and agriculture, the traditional groundwater theories are facing enormous challenges. Therefore, new theories and wider research range related to groundwater arise. Due to the rapid development of computer level, the innovation of new testing methods like isotopic methods, the hydrogeochemical analysis has become more and more convenient and effective.
New theories of groundwater chemical evolution
(1) The reverse cation exchange mechanism has been gradually revealed. Monsoon climate (Kumar et al., 2009), environmental pollution (Reddy et al., 2012; Guma et al., 2021), water acidification (Zaidi et al., 2015), seawater intrusion (Chen et al., 2020b; Roy and Zahid, 2021) and other problems may become the trigger mechanism of the reverse cation exchange process. Through leaching experiment, David and Dimitrios (2002) found that when the soil leaching time reached 15-100h, reverse cation exchange occurred (David and Dimitrios, 2002). Reverse cation exchange can reduce the content of Ca2+ and increase the content of Na+ in groundwater (Askri, 2015), which can be expressed as: Ca2+(Mg2+)−water+2Na+−clay=2Na+−water+Ca2+(Mg2+)−clay (Chen et al., 2020b).
(2) The content of in groundwater increased significantly, and the traditional GCE theory encountered new challenges. Since the mid-20th century, nitrogen fertilizer has been widely used in agricultural production, resulting in the continuous increase of /Cl− content in groundwater in agricultural areas (Abascal et al., 2021) and affecting hydrochemical types (Xu et al., 2018a). In some areas, nitric acid water has appeared (Zhou and Zhu, 2014). The entry of into groundwater triggered a series of chemical reactions (Mahaqi et al., 2021), which changed the original chemical evolution of groundwater to a great extent (Zaryab et al., 2022; Zhang et al., 2022). For example, the nitrate could be eliminated from nitrate-polluted groundwater by this chemical reaction: 5 CH2O + 4 = 2N2 + + CO2+3H2O (Mahaqi et al., 2021). There is a strong correlation between and Cl−, and denitrification occurs when the ratio of /Cl− decreases (Rezaei et al., 2017; Su et al., 2020). content was negatively correlated with pH and Mn2+, and positively correlated with ORP (Oxidation-Reduction Potential) (Cong et al., 2021; Gómez et al., 2002). Nitrogen cycle will affect the migration and transformation of arsenic (As) and iron (Fe) (Gao et al., 2021b; Smith et al., 2017)
(3) The atmosphere is providing more Cl− for groundwater, resulting in significant changes in the hydrochemical type. Cl− in groundwater mainly comes from precipitation and salt dissolution (Zhang et al., 2018). With the growth of runoff path, Cl− in groundwater gradually occupies a dominant position (He et al., 2011). As mentioned above, most of the groundwater in the vadose zone is type r and Cl− type in the DA. However, with the intensification of industrial pollution, the concentration of Cl− in the atmosphere increases due to the massive emission or discharge of chlorinated compounds, and the Cl− supplied to the groundwater through rainfall continues to increase, which makes the groundwater in the vadose zone in some areas only present a Cl− type. For example, it’s found that Cl− of the groundwater in vadose zones in some valleys may come from the atmosphere rather than geological bodies (Gardner et al., 2020). In some coastal areas, the initial type of groundwater may also show Cl− type due to the influence of seawater aerosol (Gao et al., 2017).
(4) The research on the migration and transformation mechanism of heavy metals in groundwater has attracted more and more attention. With the rapid development of urbanization and industrialization, there are many forms of heavy metal pollution worldwide. The migration and transformation mechanism of heavy metals in groundwater have attracted extensive attention (Adimalla, 2019). For example, in recent years, scientists have found that the increase of Cl− concentration is conducive to the migration of Mn2+ , and seawater intrusion can promote the release of Mn2+ from rocks to groundwater and enrich Mn2+ in groundwater (Russak et al., 2016; Gao et al., 2021a). Fe and Mn2+ ions can form complexes with other anions in groundwater to increase the dissolution of As (Zhang et al., 2020b). Natural colloids are conducive to the diffusion of heavy metal ions (Cai et al., 2016), and low pH conditions are more conducive to the dissolution of heavy metals (Jacques et al., 2008).
New methods of groundwater chemical evolution
(1) More isotopes and isotope models are involved in the study of hydrochemical evolution.
In addition to the traditional 14C, 3H and 18O isotopes widely used in groundwater traceability analysis (Craig, 1961; Zhang et al., 2006), isotopes such as 32Si, 37Ar, 85Kr, 87Sr, 226Ra and 222Rn are gaining more and more popularity in terms of groundwater source and age analysis (Knies et al., 2000; Bauer et al., 2001; Krall et al., 2017; Danish et al., 2020; Seltzer et al., 2021), which makes the research on GCE more diversified. Isotope mixing model and Bayesian mixing model are also widely used in the identification of pollutants and the calculation of pollutant contribution rate (Zhang et al., 2022).
(2) Groundwater simulation is gradually moving towards artificial intelligence based on big data.
The traditional hydrogeochemical software such as MINTEQA2, WATEQ, Netpath, EQ3/6 and PHREEQC/QE are constantly upgraded (Zhang and Zhang, 2019), and the functions of groundwater flow numerical simulation software such as GMS, MODFLOW and FEFLOW are more and more perfect (Li et al., 2021; Liu et al., 2022a). Neural network learning, deep learning and machine learning based on big data have been widely applied to solve the problem of high-dimensional uncertain GCE (Mo, 2019; El Bilali et al., 2021). For example, Liu et al. (2022) explored the low-temperature hydrogeological process of frozen soil environment through three-dimensional numerical simulation (Liu et al., 2022b). Meanwhile, it is a significant trend that big data models were employed to study the groundwater pollution. Podgorski and Berg created a global prediction map of groundwater arsenic exceeding 10 mg/L using a random forest machine-learning model based on geospatial environmental parameters and more than 50,000 aggregated data points of measured groundwater arsenic concentration (Podgorski and Berg, 2020). Keesari et al. studied big data and environmental sustainability based integrated framework for isotope hydrology applications in India (Keesari et al., 2021). Correa et al. proposed and Embedded Edge-processing IoT-based Water Quality Monitoring system for the sake of proper decision-making (Correa et al., 2022). Wang et al. applied more than 4600 water samples for the artificial neural network to predict genesis, occurrence and distribution of high iodine groundwater in China (Wang et al., 2022). Using the similar method to Wang et al. (2022), Cao et al. predicted geogenic groundwater fluoride contamination throughout China (Cao et al., 2022). Xiong et al. (2022) applied machine learning to optimize the groundwater pollution monitoring network (Xiong et al., 2022). It is also noted that geological big data sharing service has received great attention from the governments. For example, the British Geological Survey, supported by the British government, is the first country to build the geological database and they are at the forefront of sharing data worldwide through a comprehensive system in this decade (Normile, 2019; Wen et al., 2021). In China, the Chinese Geological Survey also shows the sharing attitude by developing and opening a geological database called “GeoCloud”, within which a wealth of groundwater data was accessible (Lei et al., 2021). By sharing the groundwater database between different regions, hydrologists can use different types of databases from similar geological background for reference and achieve comparative results of GFS and GCE (Normile, 2019).
In short summary, implementation of big-data methodology in terms of isotope hydrology, water pollution and environmental sustainability has drawn more and more attention. The connected and sharing geological database throughout China or other regions in the world makes it more convenient to carry out big-data based groundwater research.
Conclusion
From Darcy’s Law (1856) to GFS theory (1963), then to Tóthian theory (2016), scientists have been exploring the flow law of water for more than 160 years and will continue to do so. In the more than 50 years since the establishment of GFS force theory to finally become Tóthian theory, its connotation has been gradually enriched through the continuous development, enrichment and practice of many hydrogeologists. Tóthian theory mainly covers the distribution and driving mechanism of GFS, the transmission and distribution of matter and energy by GFS, the interaction between groundwater and environment, and the environment associated phenomena brought by groundwater. Tóthian theory is a milestone in the history of hydrogeology, which breaks the concept of aquifer and promotes the development of hydrogeology from small-scale homogeneous basin to large-scale heterogeneous basin. As a universal geological agent, the environmental effect of groundwater is gradually accepted by people. With the progresses of science and technology, the research scopes of GFS have been extended from the original saturated zone to the non-saturated zone, realizing the leap from two-dimensional space to three-dimensional space, and describing the structural characteristics of GFS more finely.
The research on the mechanism of GCE based on GFS theory and Tóthian theory have promoted each other and grown together in the past 50 years. People have mastered some general laws of GCE, such as: (1) the replacement order of cations in the process of cation exchange adsorption, (2) the main water rock reactions and the main hydrochemical types in the RA, runoff area and DA under different geological backgrounds, (3) horizontal zoning of GCE. (4) in GFS, groundwater TDS generally increases from RA to DA, (5) sources of groundwater in GFSs in different regions, (6) location and sequence of redox reaction. With the expansion of the research scopes of GFS to the unsaturated zone, soil microorganisms and CO2 have become a research hotspot in the study of GCE. The maturity of traditional isotopic methods and the addition of new isotopes have greatly improved the reliability of the research conclusion of hydrochemical evolution. The maturity of hydrochemical evolution software and the application of neural network learning and deep learning have solved the high-dimensional uncertainty problem.
With the deepening of theoretical research, more and more scientists are aware of the interaction between the distribution of GFS and GCE. The theoretical problems of GFS and the research of GCE are interdependent and inseparable. In order to better deal with the problems of GFS distribution change, GCE environment change and groundwater pollution caused by the further intensification of human transformation of nature in the 21st century, it is also a difficult problem for hydrogeologists to comprehensively use the scientific research results of Tóthian theory and GCE mechanism to promote the harmonious coexistence between man and nature.
Author contributions
HD, original draft preparation, software, and visualization. ZG, review. All authors contributed to the article and approved the submitted version.
Funding
This review was supported by the Natural Science Foundation of Shandong Province (ZR2020MD109); National Natural Science Foundation of China (40772145, 41641022).
Conflict of interest
The authors declare that the research was conducted in the absence of any commercial or financial relationships that could be construed as a potential conflict of interest.
Publisher’s note
All claims expressed in this article are solely those of the authors and do not necessarily represent those of their affiliated organizations, or those of the publisher, the editors and the reviewers. Any product that may be evaluated in this article, or claim that may be made by its manufacturer, is not guaranteed or endorsed by the publisher.
References
Abascal E., Gómez-Coma L., Ortiz I., Ortiz A. (2021). Global diagnosis of nitrate pollution in groundwater and review of removal technologies. Sci. Total Environ. 810, 152233. doi: 10.1016/j.scitotenv.2021.152233
Adimalla N. (2019). Heavy metals contamination in urban surface soils of medak province, India, and its risk assessment and spatial distribution. Environ. Geochem. Health42 (1), 59–75. doi: 10.1007/s10653-019-00270-1
Allen T. Hjelmfelt J., Pi Z. (1993). Movement of ground water in converging aquifer. J. Irrig. Drain. Eng.119 (2), 312–322. doi: 10.1061/(ASCE)0733-9437(1993)119:2(312)
Askri B. (2015). Hydrochemical processes regulating groundwater quality in the coastal plain of al musanaah, sultanate of oman. J. Afr. Earth Sci.106, 87–98. doi: 10.1016/j.jafrearsci.2015.03.009
Back W. (1960). Hydrochemical Facies and Ground-Water Flow Patterns in Northern Atlantic Coastal Plain: ABSTRACT. AAPG Bulletin, 44(7), 1244–1245.
Back W., Hanshaw B. B. (1971a). Geochemical interpretations of groundwater flow systems. J. Am. Water Resour. Assoc.7 (5), 1008–1016. doi: 10.1111/j.1752-1688.1971.tb05021.x
Back W., Hanshaw B. B. (1971b). Rates of Physical and Chemical Processes in a Carbonate Aquifer Washington:American Chemical Society. doi: 10.1021/ba-1971-0106.ch003
Bauer S., Fulda C., Schäfer W. (2001). A multi-tracer study in a shallow aquifer using age dating tracers 3H, 85Kr, CFC-113 and SF6 — indication for retarded transport of CFC-113. J. Hydrol.248 (1), 14–34. doi: 10.1016/S0022-1694(01)00381-X
Cai L., Peng S., Wu D., Tong M. (2016). Effect of different-sized colloids on the transport and deposition of titanium dioxide nanoparticles in quartz sand. Environ. pollut.208 (JAN.PT.B), 637–644. doi: 10.1016/j.envpol.2015.10.040
Cao H. L., Xie X. J., Wang Y. X., Liu H. X. (2022). Predicting geogenic groundwater fluoride contamination throughout China. J. Environ. Sci.115, 140–148. doi: 10.1016/j.jes.2021.07.005
Cederstrom D. J. (1946). Genesis of ground waters in the coastal plain of Virginia. Econ. Geol.41 (3), 218–245. doi: 10.2113/gsecongeo.41.3.218
Chadha D. K. (1999). A proposed new diagram for geochemical classification of natural waters and interpretation of chemical data. Hydrogeol. J.7 (5), 431–439. doi: 10.1007/s100400050216
Chamberlin T. C. (1885). U.S. Geol. Survey Annual Report. 5, 131–175, U.S. Geological Survey, Washington.
Chen M. (1987). Study on groundwater resources and groundwater system (Changchun: Editorial Committee of Journal of Changchun Institute of Geology).
Chen Q., Hao D. H., Gao Z. J., Shi M. J., Wang M., Feng J .G., et al. (2020a). The enrichment process of groundwater fluorine in sea water intrusion area of gaomi city, china. Ground Water58 (6), 882–891. doi: 10.1111/gwat.12990
Chen Q., Jia C. P., Wei J. C. (2020b). Geochemical process of groundwater fluoride evolution along global coastal plains: Evidence from the comparison in seawater intrusion area and soil salinization area. Chem. Geol.552, 119779. doi: 10.1016/j.chemgeo.2020.119779
Chen Z., Wang Y., Liu J., Wei W. (2010). Evolution characteristics of groundwater in typical areas of northern China in recent 50 years. Quat. Sci.30 (01), 115–126. doi: 10.3969/j.issn.1001-7410.2010.01.11
Cloutier V., Lefebvre R., Savard M. M., Bourque É., Therrien R. (2006). Hydrogeochemistry and groundwater origin of the basses-laurentides sedimentary rock aquifer system, st. lawrence lowlands, québec, canada. Hydrogeol. J.14 (4). doi: 10.1007/s10040-005-0002-3
Cong X., Zhang S. S., Xu Z. H., Xu L. R., Pan W. Y. (2021). Spatio-temporal variation and driving factors of “three nitrogen”in shallow groundwater in typical over-exploited areas in north china. Earth Environ.49 (06), 603–614. doi: 10.14050/j.cnki.1672-9250.2021.49.051
Correa C., Dujovne D., Bolano F. (2022). Design and implementation of an Embedded Edge-processing Water Quality Monitoring System for underground waters. IEEE Embedded Systems Letters, 1.
Craig H. (1961). Isotopic variations in meteoric waters. Science133, 1702–1703. doi: 10.1126/science.133.3465.1702
Danish M., Tripathy G. R., Rahaman W. (2020). Submarine groundwater discharge to a tropical coastal lagoon (Chilika lagoon, india): An estimation using Sr isotopes. Mar. Chem.224 (C). doi: 10.1016/j.marchem.2020.103816
Darcy H. (1857). Recherches expérimentales relatives au mouvement de l’eau dans les tuyaux (Paris, France: Mallet-Bachelier).
David R., Dimitrios P. (2002). Diffusion and cation exchange during the reclamation of saline-structured soils. Geoderma107 (3), 271–279. doi: 10.1016/S0016-7061(01)00152-5
El Bilali A., Taleb A., Brouziyne Y. (2021). Comparing four machine learning model performances in forecasting the alluvial aquifer level in a semi-arid region. J. Afr. Earth Sci.181, 104244. doi: 10.1016/j.jafrearsci.2021.104244
Freeze R. A., Witherspoon P. A. (1966). Theoretical analysis of regional groundwater flow: 1. analytical and numerical solutions to the mathematical model. Water Resour. Res.2 (4).doi: 10.1029/WR002i004p00641
Freeze R. A., Witherspoon P. A. (1967). Theoretical analysis of regional groundwater flow: 2. effect of water-table configuration and subsurface permeability variation. Water Resour. Res. 3 (2). doi: 10.1029/wr003i002p00623
Gao Z. (2013). Experimental demonstration and significance of groundwater flow system differentiation. J. Shandong Univ. Sci. Technol.32 (02), 17–24. doi: 10.16452/j.cnki.sdkjzk.2013.02.020
Gao Y., Chen J., Qian H., Wang H., Ren W., Qu W. (2022). Hydrogeochemical characteristics and processes of groundwater in an over 2260year irrigation district: a comparison between irrigated and nonirrigated areas. J. Hydrol.606, 127437. doi: 10.1016/j.jhydrol.2022.127437
Gao Z., Zhang F., An Y., Feng J., Wang M., Han K., et al. (2014a). The Formation and model of highly-concentrated fluorite groundwater and in-situ fluoride dispelling assumption in Gaomi City of Shandong Province. Earth Science Frontiers (China University of Geosciences (Beijing); Peking University) 21 (4), 50–58. doi: 10.13745/j.esf.2014.04.005
Gao Z., Wang S., Li J., Zhu X., Zheng Q. (2014b). Study on difference of groundwater movement in seepage flow field based on experiment sand tank. J. OF China Hydrol. 34 (01), 14–19+13. doi: 10.3969/j.issn.1000-0852.2014.01.003
Gao Z., Wang S., Xu C., Dong H., Zheng Q. (2014c). A study of the discharge controlling the differentiation of groundwater flow systems. Hydrogeol. & Eng. Geol. 41 (04), 6–10. doi: 10.16030/j.cnki.issn.1000-3665.2014.04.005
Gao Z., Xu H., Zhang P., Ji D., Xia L., Wang X., et al. (2020). Variations in bacterial community structures during geothermal water recharge-induced bioclogging. J. Environ. Sci. Health Part a-Tox./Hazard. Subst. Environ. Eng. 55 (5), 629–637. doi: 10.1080/10934529.2020.1724744
Gao Z., Wang Z., Wang S., Fu T., Liu W. (2021a). Characteristics of heavy metals and health risk assessment of groundwater in tianjin coastal area. Mar. Environ. Sci. 40 (03), 384–391. doi: 10.13634/j.cnki.mes.2021.03.008
Gao C., Feng C., Liu W., Ji A., Yoshihiro K., Iwao K. (2014). Patterns of arsenic cycle and groundwater arsenic contamination on the earth’s surface. Acta Geosci. Sin.35 (06), 741–750. doi: 10.3975/cagsb.2014.06.10
Gao Y., Qian H., Ren W., Wang H., Liu F., Yang F. (2020). Hydrogeochemical characterization and quality assessment of groundwater based on integrated-weight water quality index in a concentrated urban area. J. Cleaner Production260, 121006. doi: 10.1016/j.jclepro.2020.121006
Gao Z., Sun Z., Yang Y., Mou L., CUI Y. (2019). Occurrence characteristics and hydrochemical characteristics of geothermal water in shandong province. Sci. Technol. Eng. 19 (20), 85–90. doi: 10.3969/j.issn.1671-1815.2019.20.012
Gao Z., Weng H., Guo H. (2021b). Unraveling influences of nitrogen cycling on arsenic enrichment in groundwater from the hetao basin using geochemical and multi-isotopic approaches. J. Hydrol.595, 125981. doi: 10.1016/j.jhydrol.2021.125981
Gao Z., Zhang H., Sun M., Xu H., Yao D. (2017). The chemical characteristics and causes of shallow groundwater in the Rizhao coastal area of Shandong province are discussed. Ground Water39 (04), 1–4. doi: 10.3969/j.issn.1004-1184.2017.04.001
Gardner P. M., Nelson N. C., Heilweil V. M., Solder J. E., Solomon D. K. (2020). Rethinking a groundwater flow system using a multiple-tracer geochemical approach: A case study in moab-Spanish valley, Utah. J. Hydrol.590, 125512. doi: 10.1016/j.jhydrol.2020.125512
Garrels R. M., Mackenzie F. T. (1967). Origin of the chemical compositions of some springs and lakes. Equilib. Concepts Natural Water Systems. doi: 10.1021/ba-1967-0067.ch010
Glynn P. D., Plummer L. N. (2005). Geochemistry and the understanding of ground-water systems. Hydrogeol. J.13 (1), 263–287. doi: 10.1007/s10040-004-0429-y
Gómez M. A., Hontoria E., González-López J. (2002). Effect of dissolved oxygen concentration on nitrate removal from groundwater using a denitrifying submerged filter. J. Hazard. Mater.90, 267–278. doi: 10.1016/S0304-3894(01)00353-3
Guma B. E., Muwanga A., Owor M. (2021). Hydrogeochemical evolution and contamination of groundwater in the albertine graben, Uganda. Environ. Earth Sci.80 (8). doi: 10.1007/s12665-021-09587-6
Han D., Liang X., Jin M., Currell M., Han Y., Song X., et al. (2009). Hydrogeochemical indicators of groundwater flow systems in the yangwu river alluvial fan, xinzhou basin, shanxi, china. Environ. Manage.44 (2). doi: 10.1007/s00267-009-9301-0
Hao Y., Wang P., Zhang M., Zhang J., Li D. (2020). Hydrochemical characteristic and its driving force of groundwater in the covered karst in pearl river basin ecology and environmental sciences 29, 02, 337–344. doi: 10.16258/j.cnki.1674-5906.2020.02.015
He J., Zhang J., Ding Z., Ma J. (2011). Evolution of groundwater geochemistry in the minqin basin: taking chloride as an indicator of groundwater recharge. Resour. Sci.33 (3), 416–421. doi: 10.1007/s12182-011-0123-3
Hubbert M. K. (1940). The theory of ground-water motion. J. Geol. 48 (8, Part 1), 785–944. doi: 10.1097/00010694-194105000-00015
Imbulana S., Oguma K., Takizawa S. (2020). Evaluation of groundwater quality and reverse osmosis water treatment plants in the endemic areas of chronic kidney disease of unknown etiology (ckdu) in sri lanka. Sci. Total Environ.745 (3), 140716. doi: 10.1016/j.scitotenv.2020.140716
Jacques D., SimuNek J., Mallants D., Genuchten M. (2008). Modelling coupled water flow, solute transport and geochemical reactions affecting heavy metal migration in a podzol soil. Geoderma145 (3-4), 449–461. doi: 10.1016/j.geoderma.2008.01.009
Jia H., Ken H., Qian H. (2020). Use of multiple isotopic and chemical tracers to identify sources of nitrate in shallow groundwaters along the northern slope of the qinling mountains, china. Appl. Geochem.113, 104512. doi: 10.1016/j.apgeochem.2019.104512
Jiang X. (2013). A review of regional groundwater flow theory (Beijing: Geological Publishing House).
Jiang X., Wan L., Ge S., Cao G., Hou G., Hu F., et al. (2012a). A quantitative study on accumulation of age mass around stagnation points in nested flow systems. Water Resour. Res. 48 (12), W12502. doi: 10.1029/2012WR012509
Jiang X., Wan L., Cardenas M. B., Ge S., Wang X. (2010). Simultaneous rejuvenation and aging of groundwater in basins due to depth-decaying hydraulic conductivity and porosity. Geophys. Res. Lett.37 (5). doi: 10.1029/2010GL042387
Jiang X., Wan L., Ge S. (2011). An analytical study on stagnant points in nested flow systems in basins with depth-decaying hydraulic conductivity. Water Resour. Res.47 (1), 128–139. doi: 10.1029/2010WR009346
Jiang X., Wan L., Wang X., Ge S., Liu J. (2009). Effect of exponential decay in hydraulic conductivity with depth on regional groundwater flow. Geophys. Res. Lett.36 (24). doi: 10.1029/2009GL041251
Jiang X., Wan L., Wang X., Li H. (2012b). Distribution of groundwater age in drainage basins. Hydrogeol. & Eng. geol.39 (04), 1–6. doi: 10.16030/j.cnki.issn.1000-3665.2012.04.014
Jia H., Qian H., Zheng L., Feng W., Wang H., Gao Y. (2020). Alterations to groundwater chemistry due to modern water transfer for irrigation over decades. Sci. Total Environ.717, 137170. doi: 10.1016/j.scitotenv.2020.137170
Judit M.-S., Tóth A. (2015). Basin-scale conceptual groundwater flow model for an unconfined and confined thick carbonate region. Hydrogeol. J.23 (7), 1–22. doi: 10.1007/s10040-015-1274-x
Kaur G., Sharma S., Garg U. K. (2019). Assessment of ground water contamination by inorganic impurities in ferozepur district of punjab state, India. Asian J. Chem.31 (3), 515–521. doi: 10.14233/ajchem.2019.21601
Keesari T., Goyal M. K., Gupta B., Kumar N., Roy A., Sinha U. K., et al. (2021). Big data and environmental sustainability based integrated framework for isotope hydrology applications in India. Environ. Technol. Innovation24, 101889. doi: 10.1016/j.eti.2021.101889
Kelly W. P. (1934). Base exchange in relation to composition of clay with special reference to effect of sea water. Bull. Amer. Assoc. Petrol. Geol.18 (3), 358–367. doi: 10.2110/pec.55.04.0454
Knies D. L., Grabowski K. S., Hubler G. K. Jr., D., Deturck T. M., Mignerey A. C., et al. (2000). Determination of 32Si by AMS at the US naval research laboratory. Nucl. Instrum. Methods Phys. Res.172 (1), 321–327. doi: 10.1016/S0168-583X(00)00378-5
Korzhinskii D. (1936). Mobility and inertia of components in metasomatism (in Russian). Izv. AN USSR Ser. Geol1 (1), 35–60.
Krall L., Trezzi G., Garcia-Orellana J., Rodellas V., Morth C. M., Andersson P., et al. (2019). Submarine groundwater discharge at forsmark, gulf of bothnia, provided by Ra isotopes. Mar. Chem. 196, 162–172. doi: 10.1016/j.marchem.2017.09.003
Krumbein W. C., Garrels R. M. (1952). Origin and classification of chemical sediments in terms of pH and oxidation–reduction potentials. J. Geol60, 1–30. doi: 10.1086/625929
Kumar M., Kumari K., Singh U. K., Ramanathan A. L. (2009). Hydrogeochemical processes in the groundwater environment of muktsar, punjab: conventional graphical and multivariate statistical approach. Environ. Geol.57 (4), 873–884. doi: 10.1007/s00254-008-1367-0
Lehr J. H. (1968). Ground water movement. Am. Water Works Assoc.60, 281–285. doi: 10.1002/j.1551-8833.1968.tb03545.x
Lei C. Y., Liu Z. X., Wang B., Fan M., Xie H. Y., Yin X. K. (2021). Research on geological big data sharing service under the background of “Internet plus. Natural Resource Econ. China11, 22–27. doi: 10.19676/j.cnki.1672-6995.000626
Lei J., Zhang L., Deng Y., Huang Z. (2012). Research on utilization and protection of groundwater resources in Yangtze river basin. Yangtze River43 (03), 48–51. doi: 10.16232/j.cnki.1001-4179.2012.03.004
Li W. (1991). Origin of deep fresh groundwater and shallow saline groundwater in the minming basin gansu province. Geol Rev.37 (06), 546–554. doi: 10.16509/j.georeview.1991.06.007
Liang X., Liu Y., Jin M., Lu X., Zhang R. (2010). Direct observation of complex tothian groundwater flow systems in the laboratory. Hydrol. Processes. doi: 10.1002/hyp.7758
Liang X., Quan D., Jin M., Liu Y., Zhang R. (2013). Numerical simulation of groundwater flow patterns using flux as upper boundary. Hydrol. Processes27 (24), 3475–3483. doi: 10.1002/hyp.9477
Liang X., Sun L., Zhao F., Li Z. (1991). The study of groundwater quality problem based on the groundwater flow system theory. Earth Sci. -J. China Univ. Geosci.16 (01), 43–50.
Liang X., Zhang R., Niu H., Jin M., SUN R. (2012). Development of the theory and research method of groundwater flow system. Geol Sci. Technol. Inf.031 (005), 143–151.
Li W., Hao A. (1999). Formation and evolution model of groundwater in inland arid basins in northwest china and its significance. Hydrogeol. Eng. Geol.26 (04), 30–34. doi: 10.16030/j.cnki.issn.1000-3665.1999.04.009
Liu L., Chen J., Niu H., Li L., Yin L. (2022a). Numerical simulation of three-dimensional soil-groundwater coupled chromium contamination based on FEFLOW. Hydrogeol. Eng. Geol. 49 (01), 164–174. doi: 10.16030/j.cnki.issn.1000-3665.202102008
Liu W., Fortier R., Molson J., Lemieux J. (2022b). Three-dimensional numerical modeling of cryo-hydrogeological processes in a river-talik system in a continuous permafrost environment. Water Resour. Res.58 (3). doi: 10.1029/2021WR031630
Liu Y., Liang X., Quan D., Jin M. (2010). Experiments of groundwater flow patterns under changes of infiltration intensity. Earth Sci. Front.17 (06), 111–116.
Li Y., Zhang Q., Li J., Wang Y. (2021). Overview of research on visual modflow and gms. Yellow River43 (04), 89–93+130. doi: 10.3969/j.issn.1000-1379.2021.04.016
Luan F., Zhou J., Jia R., Lu C., Bai M. (2017). Hydrochemical characteristicsand formation mechanism of groundwater in plain areas of barkol-YiwuBasin, xinjiang. Envionmental Chem. 36 (02), 380–389. doi: 10.7524/j.issn.0254-6108.2017.02.2016062001
Maclay R. W., Winter T. C. (1967). Geochemistry and ground-water movement in northwestern minnesotaa. Ground Water5 (1), 11–19. doi: 10.1111/j.1745-6584.1967.tb01233.x
Mahaqi A., Mehiqi M., Moheghy M. A., Moheghi M. M., Hussainzadeh J. (2021). Nitrate pollution in Kabul water supplies, afghanistan; sources and chemical reactions: a review. Int. J. Environ. Sci. Technol. doi: 10.1007/s13762-021-03551-4
Maranzoni A. (2020). Galilean-Invariant expression for bernoulli’s equation. J. Hydraulic Eng.146 (2), 04019061.1–04019061.8. doi: 10.1061/(ASCE)HY.1943-7900.0001680
Meinzer O. E. (1936). Movement of ground-water. Eos Trans. Am. Geophys. Union17. doi: 10.1029/TR017i002p00478
Meinzer O. E., Wenzel L. K. (1940). Present status of our knowledge regarding the hydraulics of ground water. Eos Trans. Am. Geophys. Union35 (2), 915–941. doi: 10.1029/TR021i002p00648-2
Meng Q. (2021). Hydrochemical characteristics and controlling factors of the shallow groundwater in the midstream and downstream areas of shiyang river basin. J. Arid Land Resources Environ.35 (03), 80–87. doi: 10.13448/j.cnki.jalre.2021.072
Mo S. (2019). Towards efficient high-dimensional uncertainty quantification and inverse analysis in groundwater modeling using deep learning (Nanjing University).
Munnich K. O., Vogel J. C. (1959). The recent increase in the c*sup 14/content of the atmosphere, the biosphere and the ocean. report on the measurements of the C*SUP 14/Laboratory (Heidelberg: Zweites Physikalisches Institut der Universitat).
Nan J., Wang H., Lian X. (2008). Progr ess on technology for pb contamination of groundwater remediation. Environ. Sci. Technol.31 (02), 56–60. doi: 10.19672/j.cnki.1003-6504.2008.02.015
Normile D. (2019). Earth scientist plan a’Geological google’. Science363 (6430), 917. doi: 10.1126/science.363.6430.917
Ono M., Machida I., Ikawa R., Kamitani T., Oyama K., Muuranaka Y., Ito A., Marui A. (2019). Regional groundwater flow system in a stratovolcano adjacent to a coastal area: a case study of mt. Fuji and suruga bay, Japan: Springer Berlin Heidelberg, 27(2), 717–730.
Palmer P. C., Gannett M. W., Hinkle S. R. (2006). Isotopic characterization of three groundwater recharge sources and inferences for selected aquifers in the upper klamath basin of Oregon and California, USA. J. Hydrol.336 (1). doi: 10.1016/j.jhydrol.2006.12.008
Paolalsiam V. S., Mohan K. R. (2021). Hydrochemical characteristics and nitrate health risk assessment of groundwater through seasonal variations from an intensive agricultural region of upper Krishna river basin, telangana, India. Ecotoxicol. Environ. Saf. 213. doi: 10.1016/j.ecoenv.2021.112073
Podgorski J., Berg M. (2020). Global threat of arsenic in groundwater. Science368, 845–850. doi: 10.1126/science.aba1510
Reddy A. G. S., Saibaba B., Sudarshan G. (2012). Hydrogeochemical characterization of contaminated groundwater in patancheru industrial area, southern India. Environ. Monit. Assess.184 (6), 3557–3576. doi: 10.1007/s10661-011-2208-2
Ren J., Wu Q., Gao Z. (2014). Fundamentals of hydrogeochemistry (1st edition) (Beijing, China: Geological publishing house).
Rezaei M., Nikbakht M., Shakeri A. (2017). Geochemistry and sources of fluoride and nitrate contamination of groundwater in lar area, south Iran. Environ. Sci. pollut. R24 (18), 15471–15487. doi: 10.1007/s11356-017-9108-0
Ross C. S. (1943). Clays and soils in relation to geologic processes. Jour. Wash. Acad. Sci.33, 225–235. doi: 10.2307/24531882
Russak A., Sivan O., Yechieli Y. (2016). Trace elements (Li, B, Mn and Ba) as sensitive indicators for salinization and freshening events in coastal aquifers. Chem. Geol. 441, 35–46.
Roy S. K., Zahid A. (2021). Assessment of river water–groundwater–seawater interactions in the coastal delta of Bangladesh based on hydrochemistry and salinity distribution. SN Appl. Sci.3 (4), 411. doi: 10.1007/s42452-021-04389-8
Schwartz F. W., Domenico P. A. (1973). Simulation of hydrochemical patterns in regional groundwater flow. Water Resour. Res.9 (3), 707–720. doi: 10.1029/WR009i003p00707
Seltzer A. M., Krantz J. A., Ng J., Danskin W. R., Bekaert D. V., Barry P. H. (2021). The triple argon isotope composition of groundwater on ten-thousand-year timescales. Chem. Geol. 583, 120458. doi: 10.1016/j.chemgeo.2021.120458
Sinha D., Prasad P. (2020). Health effects inflicted by chronic low-level arsenic contamination in groundwater: A global public health challenge. J. Appl. Toxicol.40 (1). doi: 10.1002/jat.3823
Smith R. L., Kent D. B., Repert D. A., Böhlke J. K. (2017). Anoxic nitrate reduction coupled with iron oxidation and attenuation of dissolved arsenic and phosphate in a sand and gravel aquifer. Geochim. Cosmochim. Acta196, 102–120. doi: 10.1016/j.gca.2016.09.025
Su C., Zhang F., Cui X., Cheng Z., Zheng Z. (2020). Source characterization of nitrate in groundwater using hydrogeochemical and multivariate statistical analysis in the muling-xingkai plain, northeast China. Environ. Monit. Assess.192 (7), 456. doi: 10.1007/s10661-020-08347-6
Thorstenson D. C. (1984). The concept of electron activity and its relation to redox potentials in aqueous geochemical systems. Open-File Rep. doi: 10.3133/ofr8472
Tóth J. (1962). A theory of groundwater motion in small drainage basins in central Alberta, Canada. J. Geophys. Res.67 (11), 4375–4388. doi: 10.1029/JZ067i011p04375
Tóth J. (1963). A theoretical analysis of groundwater flow in small drainage basins Vol. 68 (John Wiley & Sons, Ltd).
Tóth J. (1966a). Groundwater Geology, Movement, Chemistry and Resources Near Olds. Alberta, Canada:Research Council of Alberta, RCA/AGS Bulletin 17, p 148.
Tóth J. (1966b). Mapping and interpretation of field phenomena for groundwater reconnaissance in a prairie environment, Albert, Canada. Int. Assoc. Sci. Hydrology. Bull.11 (2), 20–68. doi: 10.1080/02626666609493458
Tóth J. (1970). A conceptual model of the groundwater regime and the hydrogeologic environment. J. Hydrol.10 (2), 164–176. doi: 10.1016/0022-1694(70)90186-1
Tóth J. (1971). Groundwater discharge: a common generator of diverse geologic and morphologic phenomena. international association of scientific hydrology. Bulletin16, 7–24. doi: 10.1080/02626667109493029
Tóth J. (1977). The hydrogeological reconnaissance maps of Alberta. Alberta Res. council Bull.35, 1–11.
Tóth J. (1978). Gravity-induced cross-formational flow of formation fluids, red earth region, Alberta, Canada: Analysis, patterns, and evolution. Water Resour. Res.14 (5). doi: 10.1029/WR014i005p00805
Tóth J. (1979). Patterns of dynamic pressure increment of formation-fluid flow in large drainage basins, exemplified by the red earth region, Alberta (Canada: China Social Sciences Pub. House).
Tóth J. (1980). Cross-formational gravity-flow of groundwater: a mechanism of the transport and accumulation of petroleum (the generalized hydraulic theory of petroleum migration). problems of petroleum migration. Washington:American Association of Petroleum Geologists.
Tóth J. (1984). The role of regional gravity flow in the chemical and thermal evolution of ground water. Proceedings of the First Canadian/american Conference on Hydrogeology. Alterta, Canada, 22–26 June 1984.
Tóth J. (1995). Hydraulic continuity in large sedimentary basins. Hydrogeol. J.3 (4), 4–16. doi: 10.1007/s100400050250
Tóth J. (1996). Thoughts of a hydrogeologist on vertical migration and near-surface geochemical exploration for petroleum. AAPG Memoir66 (20), 279–283. doi: 10.1306/M66606C20
Tóth J. (1999). Groundwater as a geologic agent: An overview of the causes, processes, and manifestations. Hydrogeol. J.7 (1), 1–14. doi: 10.1007/s100400050176
Tóth J. (2009). Gravitational systems of groundwater flow:Theory, evaluation and utilization (Cambridge, U.K.: Cambridge University Press). doi: 10.1017/CBO9780511576546
Tóth J. (2016). The evolutionary concepts and practical utilization of the tóthian theory of regional groundwater flow. Int. J. Earth Environ. Sci.1, 111. doi: 10.15344/2456-351X/2016/111
Tóth J., Corbet T. (1986). Post-Paleocene evolution of regional groundwater flow-systems and their relation to petroleum accumulations, Taber area, southern Alberta, Canada. Bull. Can. Petrol. Geol.75 (2), 331–335. doi: 10.35767/gscpgbull.34.3.339
Wang D. (1995). Fundamentals of hydrogeology (Fourth edition) (Beijing: Geological Publishing House).
Wang X. S., Wan L., Jiang X. W., Li H., Zhou Y., Wang J. (2017). Identifying three-dimensional nested groundwater flow systems in a tóthian basin. Adv. Water Resour. 108 (oct.), 139–156. doi: 10.1016/j.advwatres.2017.07.016
Wang W., Li W., Cai Y., An Y., Shao X., Wu X., Yin D. (2021a). A study of the hydrogeochemical evolution of groundwater in the middle reaches of the heihe river basin. Earth Sci. Front. 28 (04), 184–193. doi: 10.13745/j.esf.sf.2021.5.28
Wang Z., Wang S., Fu T., Gao Z., Xu X. (2021b). Characteristics and health risk assessment of heavy metals in groundwater of qinhuangdao coastal zone. Environ. Chem.40 (04), 1157–1166. doi: 10.7524/j.issn.0254-6108.2020091405
Wang X. S., Jiang X. W., Wan L., Ge S., Li H. (2011). A new analytical solution of topograghy-driven flow in drainage basin with depth-dependent anisotropy of permeability. Water Resour. Res.47 (9). doi: 10.1029/2011WR010507
Wang Y. X., Li J. X., Xie X. J. (2022). Genesis and occurrence of high iodine groundwater. Earth Sci. Front.29 (3), 001–010. doi: 10.13745/j.esf.sf.2022.1.47
Wen D., Huang H., Shi Y. K., Yang S. R., Yang J., Wang Y., et al. (2021). Big data management and sharing for earth sciences: An paradigm from British geological survey. Geol J. China Universities27 (1), 45–57. doi: 10.16108/j.issn1006-7493.2020098
Winter T. C., Harvey J. W., Franke O. L., Alley W. M. (1998). Ground water and surface water: A single resource. U.S. Geological Survey Circular 1139. Colorado Geological Survey.
Wolery T. J., Jackson K. J., Bourcier W. L., Bruton C. J., Delany J. M. (1990). Current status of the EQ3/6 software package for geochemical modeling. Chem. Modeling Aqueous Syst. II. doi: 10.1021/bk-1990-0416.ch008
Xia L., Gao Z., Xu H., Feng G. (2020). Variations in bacterial community during bioclogging in managed aquifer recharge (MAR): A laboratory study. Int. Biodeterior. Biodegradation147. doi: 10.1016/j.ibiod.2019.104843
Xiao J., Wang L., Chai N., Liu T., Rinklebe J. (2021). Groundwater hydrochemistry, source identification and pollution assessment in intensive industrial areas, eastern chinese loess plateau. Environ. pollut.278, 116930. doi: 10.1016/j.envpol.2021.116930
Xiong Y., Luo J., Liu X., Liu X., Xin X. (2022). Machine learning-based optimal design of groundwater pollution monitoring network. Environ. Res. 211, 113022. doi: 10.1016/j.envres.2022.113022
Xu N., Gong J., Yang G. (2018b). Using environmental isotopes along with major hydro-geochemical compositions to assess deep groundwater formation and evolution in eastern coastal China. J. contam. hydrol. 208. doi: 10.1016/j.jconhyd.2017.11.003
Xu J., He J., Peng C., Zeng Y. (2018a). Characteristics and genesis of no_3 type water in shallow groundwater in liujiang basin. Environ. Sci.39 (9), 4142–4149. doi: 10.13227/j.hjkx.201712082
Zaidi F. K., Nazzal Y., Jafri M. K., Naeem M., Ahmed I. (2015). Reverse ion exchange as a major process controlling the groundwater chemistry in an arid environment: a case study from northwestern Saudi Arabia. Environ. Monit. Assess.187 (10), 607. doi: 10.1007/s10661-015-4828-4
Zaryab A., Nassery H. R., Knoeller K., Alijani F., Minet E. (2022). Determining nitrate pollution sources in the Kabul plain aquifer (Afghanistan) using stable isotopes and Bayesian stable isotope mixing model. Sci. Total Environ.823, 153749. doi: 10.1016/j.scitotenv.2022.153749
Zhang J., Cao M., Jin M., Huang X., Zhang Z., Kang F. (2022). Identifying the source and transformation of riverine nitrates in a karst watershed, north China: Comprehensive use of major ions, multiple isotopes and a Bayesian model. J. Contam. Hydrol.246, 103957. doi: 10.1016/j.jconhyd.2022.103957
Zhang J., Hou R., Yin L., Ma H., Huang J. (2017). Formation and influencing factors of regional groundwater flow systems. Hydrogeol. & Eng. Geol.44 (04), 8–14. doi: 10.16030/j.cnki.issn.1000-3665.2017.04.02
Zhang X., Jiao J. J., Li H., Luo X., Kuang X. (2020a). Effects of downward intrusion of saline water on nested groundwater flow systems. Water Resour. Res.56 (10). doi: 10.1029/2020WR028377
Zhang R., Liang X., Jin M., Tóth József (2015). Gravitational systems of groundwater flow theory, evaluation, utilization (Beijing: Geological Publishing House).
Zhang R., Liang X., Jin M., Wan L., Yu Q. (2018). General hydrogeology. Seventh Edition (Beijing China: Geological Publishing House).
Zhang Y., Wu Y., Wen X., Su J. (2006). Application of environmental isotopes in water cycle. Adv. In Water Sci.17 (05), 738–747. doi: 10.14042/j.cnki.32.1309.2006.05.025
Zhang Z., Xiao C., Adeyeye O., Yang W., Liang X. (2020b). Source and mobilization mechanism of iron, manganese and arsenic in groundwater of shuangliao city, northeast china. Water12 (2), 534. doi: 10.3390/w12020534
Zhang B., Zhang C. (2019). Progress on hydrogeochemical method applied in groundwater study. Yellow River41 (10), 135–142. doi: 10.3969/j.issn.1000-1379.2019.10.023
Zhao Z., Wang D., Tao Z., Li Y. (2008). Multi- layer circulation model of groundwater flow systems on the ordos plateau, China: evidence from water head measurements at different depths of a deep borehole by the packer system. Geol Bull. China 27 (08), 1131–1137. doi: 10.1016/S1872-5791(08)60056-1
Zhao N., Yan X., Li Q., Fu S., Chu X. (2021). Hydrochemical characteristics and formation mechanism of shallow groundwater in around-lake area of poyang lake. Yangtze River52 (01), 44–48. doi: 10.16232/j.cnki.1001-4179.2021.01.008
Zhou X., HU F., He J. (2014). Introduction to groundwater science (2nd edition) (Beijing, China: Geological publishing house).
Zhou X., Zhu C. (2014). The nitrate content characteristics of shallow groundwater in jinjiang city, fujian province, and their hydrochemical indication significance. Acta Geosci. Sin.35 (2), 177–182. doi: 10.3975/cagsb.2014.02.08
Keywords: Tóthian theory, groundwater chemical evolution, groundwater flow system, gravity driven, human factor
Citation: Dong H and Gao Z (2022) Theoretical progress of groundwater chemical evolution based on Tóthian theory: A review. Front. Mar. Sci. 9:972426. doi: 10.3389/fmars.2022.972426
Received: 18 June 2022; Accepted: 03 August 2022;
Published: 22 August 2022.
Edited by:
Yanpeng Cai, Guangdong University of Technology, ChinaReviewed by:
Jun Xiao, Institute of Earth Environment, (CAS), ChinaChemseddine Fehdi, University of Tébessa, Algeria
Hui Qian, Chang’an University, China
Copyright © 2022 Dong and Gao. This is an open-access article distributed under the terms of the Creative Commons Attribution License (CC BY). The use, distribution or reproduction in other forums is permitted, provided the original author(s) and the copyright owner(s) are credited and that the original publication in this journal is cited, in accordance with accepted academic practice. No use, distribution or reproduction is permitted which does not comply with these terms.
*Correspondence: Zongjun Gao, Z2Fvem9uZ2p1bkAxMjYuY29t