- 1Marine Research Station (MRS), Institute of Cellular and Organismic Biology, Academia Sinica, I-Lan County, Taiwan
- 2Department of Life Science, College of Life Science, National Taiwan University, Taipei City, Taiwan
Cephalopods are ancient mollusks that can be found in many different ecological niches in the ocean ranging from the intertidal zone to the deep-sea abyss. In order to adapt to a lifestyle in various habitats, cephalopods have evolved a variety of locomotory modes to accommodate their respective habitats. Most cephalopods have relatively high metabolic rates due to their less efficient swimming mode by jet propulsion. This lifestyle is characterized by a high level of energy expenditure, fueled exclusively by protein diets that are rapidly digested and may produce metabolic nitrogenous waste NH3/accumulation and acid-base disturbances. This study observed that the transport rate in pelagic bigfin reef squid (Sepioteuthis lessoniana) is two times faster than benthic common octopus (Octopus vulgaris). Inhibition of Na+/H+ exchangers (NHEs) showed significant disruption of and H+ excretory processes in gills of octopus but not in squid. However, inhibition of vacuolar-type H+-ATPase (VHA) significantly disrupts and H+ transport rates in gills of both animals. Accordingly, for and H+ homeostasis, benthic octopus with lower aerobic respiration rates utilize both active and Na+-driven secondary transport machinery. In order to avoid accumulated in the blood, pelagic squids with higher aerobic respiration rates prefer active and H+ transport mechanisms that consume ATP intensively.
Introduction
The marine ecosystem was dominated by cephalopods during the Ordovician period, primarily by primitive nautiloidea (Kroeger and Yun-Bai, 2009; Histon, 2012). In place of nautiloidea, modern coleoid cephalopods, such as octopuses and squids, still serve as apex predators at the top of the food chain (Villanueva et al., 2017). They have evolved advanced sensory and locomotor abilities, which are regarded as physiological traits in terms of convergent evolution for competition with fish in the marine environment (Hu et al., 2014; Hu et al., 2015; Chung et al., 2020). Due to their well-developed nervous system and specialized locomotion patterns (crawling and jet propulsion), coleoid cephalopods have the advantage over most marine invertebrates or even teleosts (Xavier et al., 2015). Today, over 800 species of coleoid cephalopods have evolved a variety of lifestyles in order to survive in the different ecological niches of the ocean system (Wood et al., 2000; Jereb and Roper, 2010). Octopod and loliginid cephalopods have been observed in distinct ecological niches across all families. Octopodidae animals are mainly benthic and cave-dwelling cephalopods that crawl and rest in a restful posture, thus avoiding predation risk or waiting for prey (Scheel and Bisson, 2012). Additionally, loliginids are pelagic cephalopods with locomotive flexibility. The jet-propulsion swimming pattern enables them to maintain substantial advantages that confer maneuverability for a variety of athletic activities, such as chasing prey, projecting ink for escape, and irrigation of egg capsules (Wells, 1990). Furthermore, most loliginid cephalopods possess fin-like extensions on their mantle. Therefore, in addition to jet propulsion, fin locomotion allows loliginid cephalopods to move in a manner similar to that of fish. However, fin-derived locomotion creates more drag while shifting distantly. Therefore, pelagic loliginid cephalopods evolved relatively small lobe fins for hovering and mainly used jet-propulsion when making long migrations (O’Dor, 2002; Mather et al., 2010).
There is also the fact that cephalopods have comparative high metabolic rates compared to other marine animals, which is a result of their athletic locomotion (Potts, 1965). As an example, cephalopods swim by jet propulsion, which is an inefficient and energy-consuming mode of locomotion (Seibel and Drazen, 2007). It should also be noted that the energy sources of cephalopods are primarily derived from protein metabolism rather than carbohydrate or lipid metabolism (Boucher-Rodoni and Mangold, 1989; Lee, 1995). Protein metabolism generates nitrogenous compounds such as ammonia (NH3)/ammonium (), urea, uric acid, and so on (Wright, 1995). Therefore, the metabolically-derived nitrogenous wastes could accumulate in blood to the lethal level, causing further metabolic dysregulation and extracellular acid-base disturbances (Boucher-Rodoni and Mangold, 1995; Lee, 1995; Wells and Clarke, 1996). And the conversion of ammonium into free amino acids by specific metabolic pathways is thought to be an important means of maintaining ammonium homeostasis. A close relationship exists between the synthesis of glutamate (Glu) and glutamine (Gln) and the production and utilization of ammonium (Huang et al., 2020). As a consequence, most modern coleoid cephalopods would have a mechanism for excreting or utilizing nitrogenous waste, and they should also have remarkable features for regulating metabolic ammonium and acidosis. According to earlier studies, branchial hearts, renal appendages, and gills would be involved in the excretion of ammonium for the maintenance of homeostasis in cephalopods (Potts, 1965). However, there is still uncertainty about the homeostatic trade-offs between adaptive energy metabolism and movement by fins and jet propulsion, or even crawling locomotion, in coleoid cephalopods, which may reflect species distribution from natural selection.
Acid-base and ammonium regulation are essential features of aquatic animals and contribute to a range of intracellular pH values (Hwang and Perry, 2010; Hwang and Lin, 2013; Hu et al., 2015). It relates to a wide range of physiological processes, including signaling transduction, metabolic adjustments, and animal activities (Tseng and Hwang, 2008; Chang and Hwang, 2011; Tovey and Brauner, 2018). Early studies in teleosts indicated that the gill epithelium is the primary tissue responsible for gas exchange, ion and acid-base regulation, and nitrogenous product excretion (Evans et al., 2005; Hwang and Lin, 2013). In a perturbed aquatic system, those transepithelial ion regulatory proteins play the most important role in maintaining ion and pH homeostasis. For example, the vacuole H+-ATPase (VHA), Na+/H+ exchanger (NHE), rhesus protein (RhP), Na+/K+ pump (NKA) and other ion regulatory proteins have been demonstrated to be involved in blood pH stabilization and ammonium excretion in teleosts (Liu et al., 2013; Tseng et al., 2013; Liu et al., 2016). In eukaryotic organisms, the VHA is an essential component of the acid-base regulation, as it drives proton flux on the epithelial membrane coupled to ATP hydrolysis (Nishi and Forgac, 2002). And the NHE is a membrane protein that maintains the acid-base and balance in teleosts by facilitating transepithelial Na+ uptake and H+ and/or secretion (Tseng et al., 2020). Using the advanced homeostasis model in teleosts, (Hwang et al., 2011) further delineated the functions of specialized epithelial ionocytes that maintain acid-base and ammonium balance by active or passive transport. Fish-derived homeostasis mechanisms have also been identified in other aquatic invertebrates, such as cephalopods and crustaceans (Wright, 1995; Wright and Wood, 2009; Hu et al., 2014; Hu et al., 2015). Recently, a study of gills, liver and blood of cuttlefish (Sepia pharaonis) demonstrated that this sepiid cephalopod family could convert ammonia to urea via uricolysis of the ornithine-urea cycle (OUC) and glutamine formation pathway for ammonia detoxification under high ambient ammonia nitrogen stress. (Peng et al., 2017). Moreover, convergent evolution between fish and cephalopods has led to the development of a well-developed branchial acid-base regulatory system coordinated by specific regulatory proteins in the gill tissue of modern coleoid cephalopods, such as VHA, NHE, RhP and other ion regulatory proteins, which is the primary site for ammonium regulation (Hu et al., 2014; Hu et al., 2015; Hu et al., 2017).
Due to the fact that cephalopods require sufficient energy to achieve their respective locomotion types, the gill tissue is thought to be the most sensitive organ for and H+ regulation (Hu et al., 2014). It is still unclear how they may differ in relation to different behavioral paradigms. The present study examined the metabolic features and responses of gill epithelial and H+ transport in pelagic and benthic cephalopods. The gills are relatively complex organs compared to other excretory organs since they contain ctenidial arteries and ctenidial veins associated with branchial and systemic hearts, respectively. Based on our previous study on common octopus (Hu et al., 2017), a gill perfusion approach with specific inhibitors against regulatory proteins was applied to determine the functions of responsive proteins in the acid-base regulation of the coleoid squid, S. lessoniana and octopus, O. vulgaris. As a way to better understand the and acid-base regulation mechanisms in cephalopod gills, the perfusion saline was prepared according to the osmolarity, ion content, and pH value (~pH 7.6) of blood from octopus and squid. This study hypothesizes that the distinct ammonium and acid-base homeostasis abilities of pelagic squid and benthic octopus may be related to their different locomotion patterns and lifestyles, which may explain why these two cephalopod species remain as the apex marine predators in the open marine system.
Materials and methods
Experimental animals
The adult bigfin reef squid (Sepioteuthis lessoniana; mantle lengths ranging from 200 to 250 mm) and common octopus (Octopus vulgaris; mantle lengths ranging from 150 to 180 mm) were collected from Bisha and Heping Island harbors in Keelung, Taiwan. The bigfin reef squid and common octopus were raised at the Marine Research Station, ICOB, Academia Sinica, and were kept respectively in aerated circulatory fiber reinforced plastics (FRP) buckets (total volume approximately 1000 L seawater, pH 8.0 to 8.1, sand filters, constant 12/12h light-dark cycle) and in flow-through systems (total volume approximately 37000 L, pH 8.0. to 8.1). The animals were fed twice daily with a diet consisting of white leg shrimp (Litopenaeus vannamei) and Japanese horse mackerel (Trachurus japonicus). Animals weighing 500 to 800 grams were kept for at least two months before they were sacrificed for experiments. The experimental protocols were approved by the Biosafety Committee and Institutional Animal Care and Use Committee of Academia Sinica (approval no. BSF17-12-1158).
Oxygen consumption estimations
The resting metabolic rates (RMRs) of octopus (Octopodidae) and squid (Loliginidae) cephalopods were determined by observing the oxygen consumption rate when the animals were incubated in a closed swim tunnel (an intermittent-flow respirometry system) with continuous water flow (as shown in Supplementary Figure S1). During the experiment, the oxygen consumption of animals was measured until the air saturation level dropped to 70%, a criteria level applicable to embryonic squid and adult fish (Kuan et al., 2022; Wang et al., 2022). In the beginning, the animals were gently transferred to the swimming tunnel and were temporarily adapted for ten minutes. The estimations would begin after habituation, and the intact system would be maintained at 26°C. The oxygen probes were calibrated according to the manufacturer’s instructions (available on the PreSens website https://www.presens.de/products/detail/oxy-4-mini) and then placed in the swimming tunnel and connected to a mini channel fiber-optic oxygen transmitter (PreSens, Regensburg, Germany). Additionally, the bacteria control would be tested under the same conditions as the background for 30 minutes to ensure that the respiration of the bacteria could be ignored.
Examination of amino acids content in blood collected from the vena cava
After dissecting the funnel and mantle from the ventral side, blood samples were taken at approximately 500 μL from different blood transit stations (anterior vena cava, ctenidial artery or ctenidial vein) of the circulatory system (Figure 1A) by using syringes. In this study, glutamate and glutamine levels in blood were determined from samples collected from the anterior vena cava in order to determine the levels of these substances before entering the systemic heart of cephalopods. In order to extract the amino acids in the blood of anterior vena cava, ethanol was used, along with norvaline as an internal standard, and the sample was centrifuged at 4300 g for 10 minutes. The 2 mL fixed quantity supernatant was transferred to a new tube and dried in a vacuum concentrator. The dried samples were reconstituted in 100 mL of 8 mM HCl, filtered with a 0.2 micron syringe filter, and then analyzed using the AccQ tag Ultra Reagent Kit (Water, Milford, MA, USA). Derivatized samples were injected into a high-performance liquid chromatography (HPLC) system (ACQUITY UPLC H-Class System, Waters) equipped with a TUV detector. The amino acids were identified and measured by comparing with the retention times and peak areas of the standards (WAT088122, Waters).
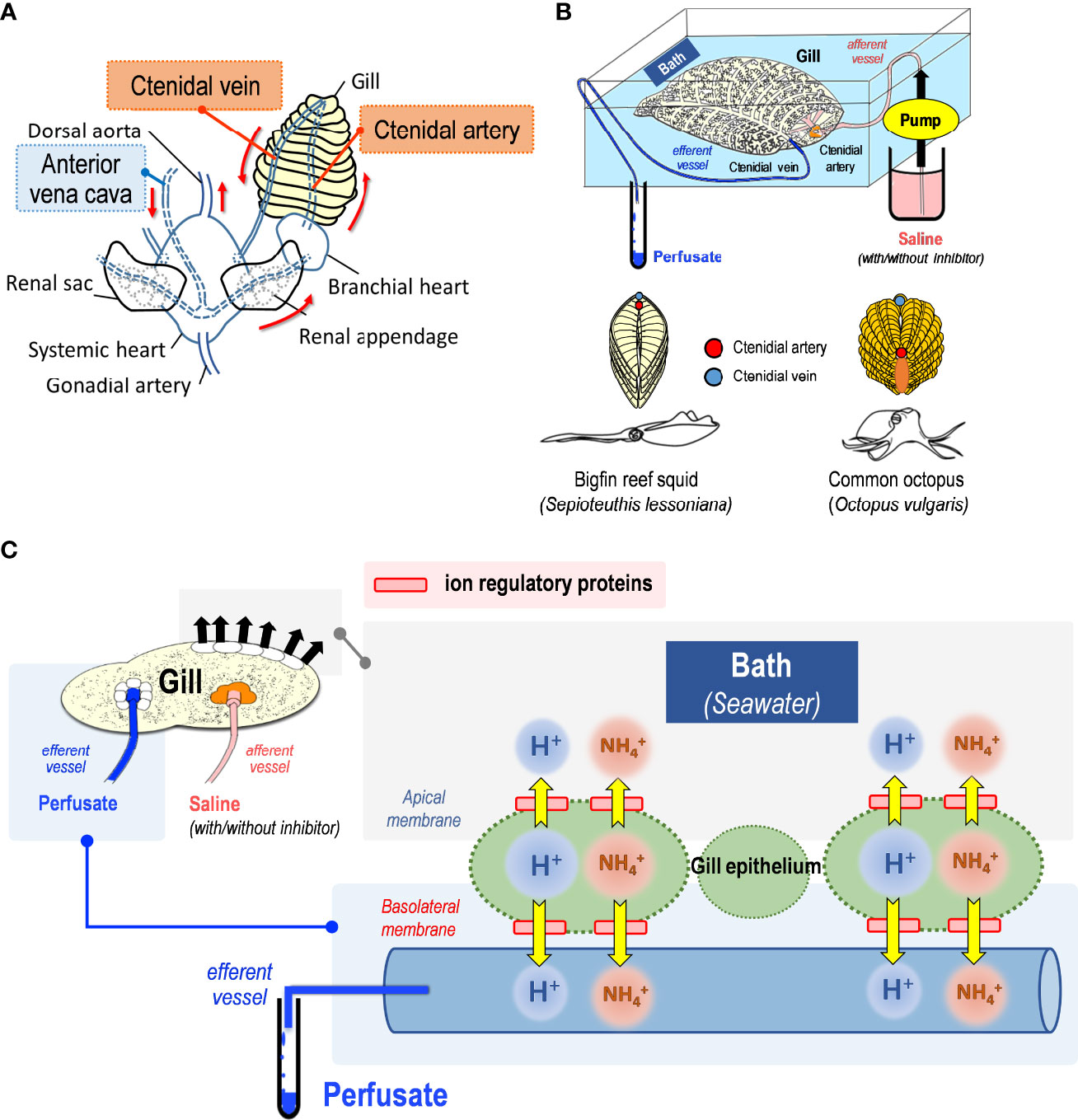
Figure 1 Representative images of blood circulation in cephalopod gills and ex-vivo approach. (A) The closed circulation system of cephalopods. The arrows indicate the direction of blood flow in the vessels. Vessels (anterior vena cava, ctenidial artery and ctenidial vein) where blood samples were taken were highlighted in background colors. (B) The schematic model of the ex-vivo perfusion experiment in the gills. During the experiments, the gills were incubated in a filtering seawater bath. The perfusate was collected from the efferent side of the tubing. (C) The sample collected from perfusate represents the fluid transport from the basolateral side of the gill epithelium to the circulating blood via the ctenidial vein.
Evaluation of NH4+ concentrations in blood of gill artery and vein
For the measurement of NH4+ concentrations, 25 μL of blood was mixed with 100 μL of reagent containing orthophthaldialdehyde, sodium sulphite, and sodium borate. After incubation in the dark for two hours at room temperature, the fluorescence was measured at excitation and emission wavelengths of 350 and 420 nm using a microplate reader (Moleculer Device, Spectra Max, M5) (Holmes et al., 1999; Hu et al., 2014).
Preparation of perfusion salines
The perfusion salines have similar ingredients and osmolarity to real cephalopod blood (Hu et al., 2017) depending on the native ion concentration and pH value (pH 7.6) in the in vivo blood test for different cephalopods. The blood sample extraction procedure begins with centrifugation of the samples for one minute at 12000 rpm. The supernatant was then diluted with double-deionized water (1:10000). Afterwards, major cation contents (Na+, K+, Ca2+, Mg2+) were determined using an atomic absorption spectrophotometer (Hitachi Z-8000, Tokyo, Japan). For the measurement of anion ion Cl-, a double-beam spectrophotometer was used (NanoDrop 2000/2000c UV-Vis Spectrophotometer, Thermo Scientific). For the generation of the standard curve, the standard solutions of major ions (Na+, K+, Ca2+, Mg2+, Cl-; Merck Darmstadt, Germany) were used. Corning 965 carbon dioxide analyzer (Olympic Analytical Service, England) was used to estimate concentration from total dissolved carbon measurements. The perfusion salines used in the cephalopod perfusion experiments are different between bigfin reef squid and common octopus, as shown in Supplementary Table S1.
Gill perfusion experiments and pharmacological evaluations
Gills were carefully removed from the mantle cavity and connective tissues for the perfusion experiments (Figure 1A). The gills were carefully cut at the 1st order ctenidial arteries and veins that transport blood between the gills and branchial heart. After the gills had been completely dissected from the mantle cavity, ex-vivo gill perfusion experiments could be performed. The ctenidial arteries and veins were connected with one end of special-treated polyethylene tubes (0.86 mm inner diameter*1.52 mm outer diameter, PE-100) and tied tightly by woven strings. Another end of the polyethylene tubes was attached to PVC tubes connected to thicker silicon tubes (2 mm in diameter). Silicon tubes fitted with a peristaltic pump (Multi-channel peristaltic system, MINIPULS® Evolution) was used to pump the perfusion saline into the gill at a rate of 12 mL per hour by setting the pump to 10 revolutions per minute (rpm). Perfusion experiments were conducted by incubating the gills in an aerated container filled with 50 mL of filtering seawater (Figure 1B).
For the purpose of ensuring that the regulatory proteins are inhibited by the specific inhibitors (ethylisopropyl amiloride (EIPA) for NHE; bafilomycin A1 (BafA1) for VHA), the sample collection should begin after the saline has been in the gill for at least 30 minutes. We prepared perfusion saline by adding different dosages of EIPA that could compete for Na+ binding sites to determine whether NHEs are responsible for regulating cephalopod gill function (Schaffhauser et al., 2016) and could be acted on squid as well (Hu et al., 2011). The role of VHA in the regulation of homeostasis in the gills of squid and octopus was examined by preparing perfusion saline containing different dosages of BafA1, which is noncovalently binding with VHA to prohibit H+ conduction. As part of the perfusion experiments, the pH value of saline would be immediately measured by a pH meter and adjusted with 0.1 M hydrochloric acid and NaOH(aq). After the perfusion experiments had been completed, the perfusate and bath were collected and immediately measured for pH and NH4+ concentration. The sample collected from the perfusate represents the fluid that would be transported back to the body via the ctenidial vein. This represents the functions of the basolateral membrane side to the perfusate blood (Figure 1C). Alternatively, the sample collected from the bath represents the fluid that would be expelled or exchanged by the apical side of gills with the surrounding environment (seawater, bath) (Figure 1C).
Statistical analysis
GraphPad Prism 7.00 (GraphPad, San Diego, CA, USA) was used for statistical analyses. Data are presented as mean ± S.D. Student’s t-test was used to test for significance between squid and octopus in terms of oxygen consumption rates, glutamate/glutamine contents, ammonium transport rates, and ammonium concentration between the ctenidial artery and ctenidial vein. The effect of different dosages of EIPA and BafA1 on and H+ transport rates in gills was determined using one-way ANOVA followed by Tukey’s pairwise comparison. Differences were considered to be significant at p< 0.05.
Results
The resting metabolic appearances of octopus and squid
The resting metabolic rates (RMRs) were determined by observing the oxygen consumption rates of experimental animals. The oxygen consumption rate of octopus (33.69 ± 6.51 µmol O2/h g) is significantly lower (p<0.001) than that of squid (183.23 ± 95.67 µmol O2/h g) (Figure 2A). During the experimental process, both species remain calm and do not exhibit any active behavior with distance movement.
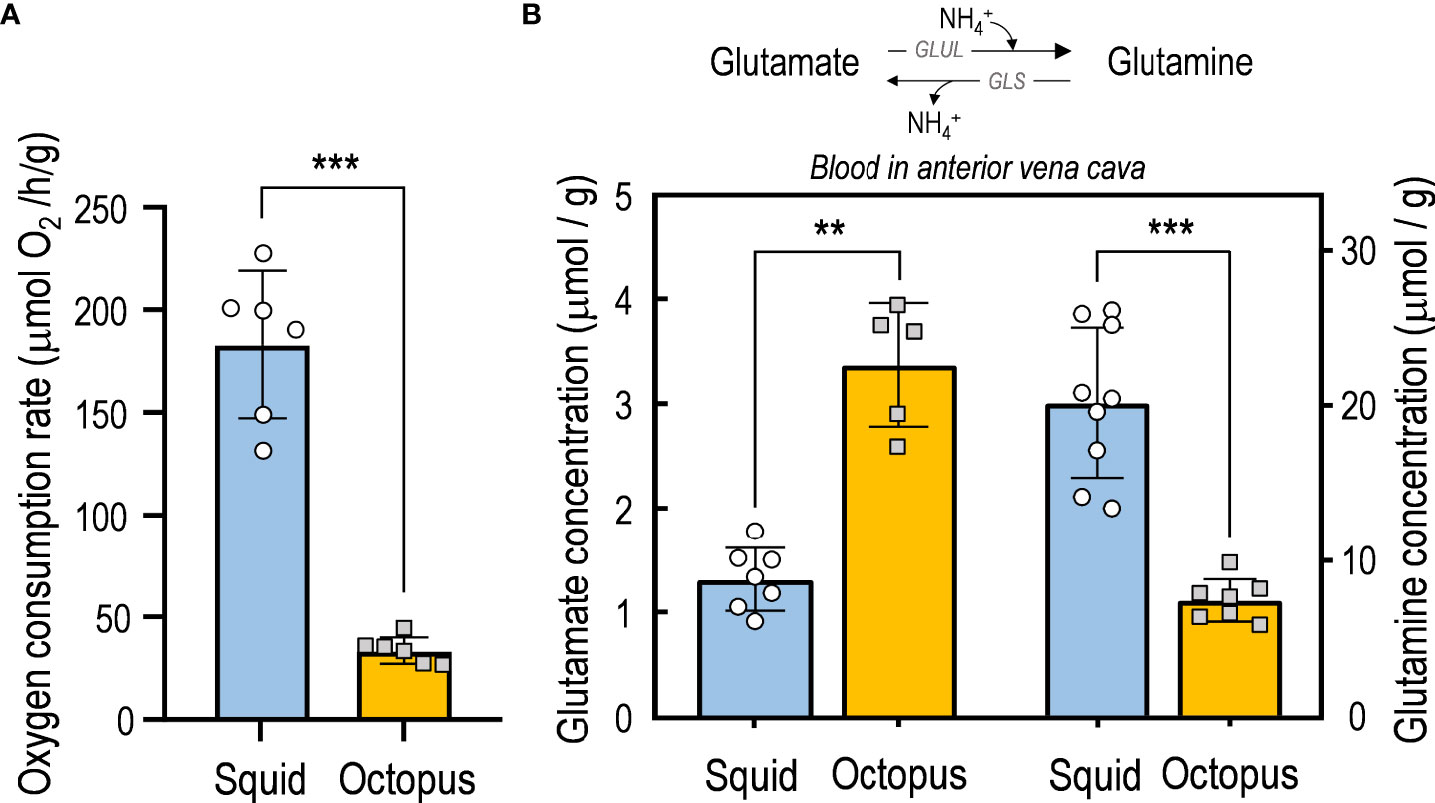
Figure 2 Metabolic features in squid and octopus. (A) The resting metabolic rates (RMRs) of adult bigfin reef squid (S. lessoniana) and common octopus (O. vulgaris) were evaluated by measuring their oxygen consumption rates under the animals were incubated in a closed swim tunnel with continuous water flow. (B) The glutamate and glutamine contents in blood were collected from the anterior vena cava. The estimated values are presented as mean ± SD (n = 5~9). Asterisks indicate significance levels of p < 0.01 (**) and p < 0.001 (***).
Glutamate and glutamine contents in blood collected from anterior vena cava
The glutamine concentrations in blood collected from the anterior vena cava of octopus (7.42 ± 1.27 μmol/g) and squid (20.19 ± 4.54 μmol/g) were both relatively higher than glutamate concentrations (3.23 ± 0.83 μmol/g in octopus; 1.67 ± 0.77 μmol/g in squid) (Figure 2B). Notably, the glutamate content in blood collected from the anterior vena cava of octopus is 1.9-fold higher than that of squid (Figure 2B). By contrast, the glutamine content in the anterior vena cava blood of octopus is 2.7-fold lower than that of squid (Figure 2B).
NH4+concentrations in blood of octopus and squid gills
Blood was collected from the ctenidial arteries and veins of octopus and squid, respectively, in order to better understand the NH4+ concentrations in the gills. The NH4+ concentration in ctenidial veins (~250 μM) of octopus was lower than that in ctenidial arteries (~300 μM) (Figure 3A). Moreover, in squid, the concentration in ctenidial veins (~20 μM) was found to be also lower than that in ctenidial arteries (~25 μM) (Figure 3A). Furthermore, the concentration in the blood of octopus gills (250~300 μM) is approximately ten times higher than that of squid (20~25 μM).
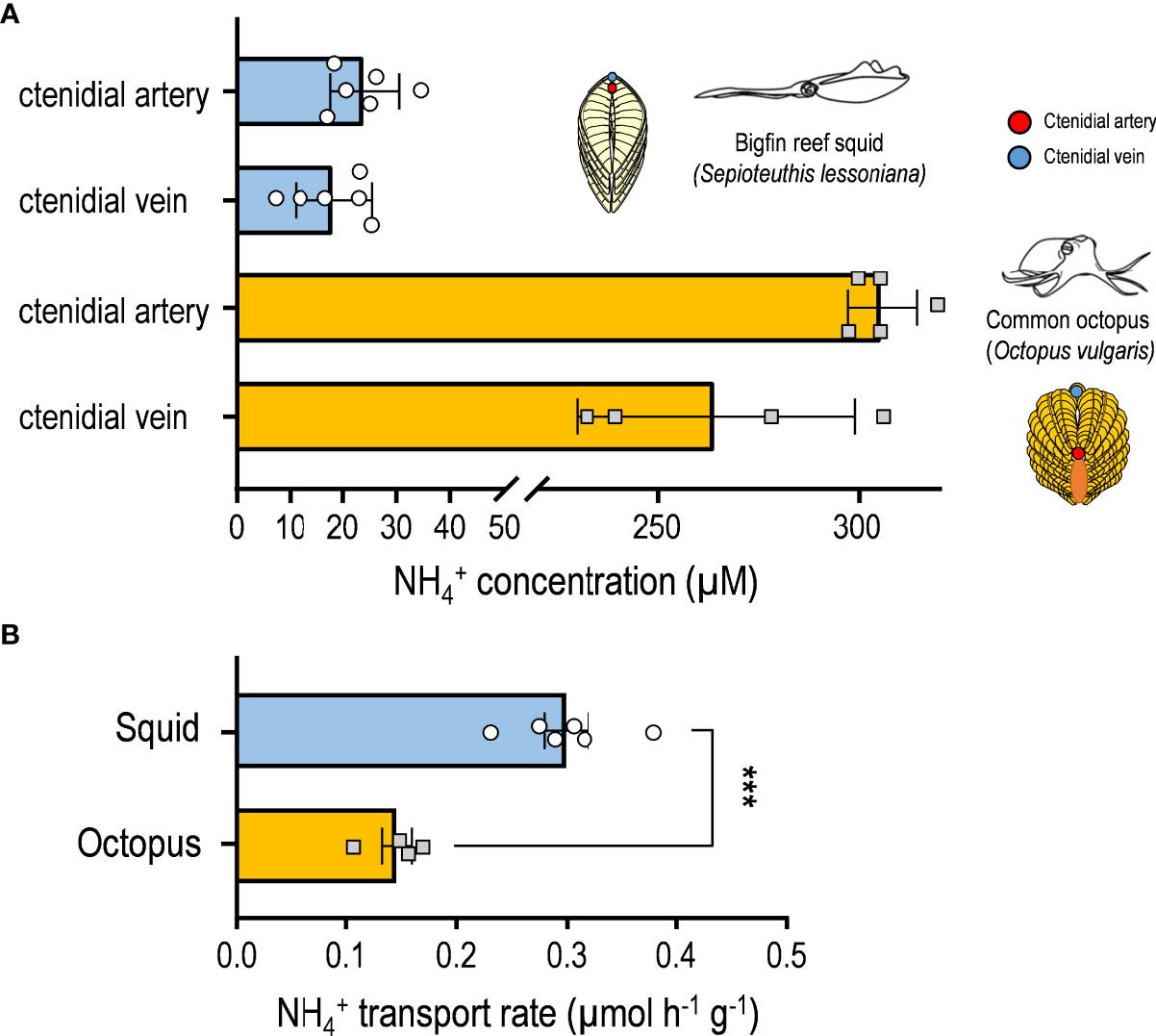
Figure 3 concentration and transport rates in cephalopod gills. (A) The concentration in blood collected from the ctenidial artery and ctenidial vein of squid and octopus. (B) The transport rates were determined using the fluid collected from the perfusate in perfused gills. The estimated values are presented as mean ± SD (n = 4~6). Asterisks indicate significance levels of p < 0.001 (***).
NH4+ transport patterns of gills by the perfusion approach
As shown in Figure 3B, the transport rate in the epithelium from the basolateral site to the perfusate blood of octopus was 0.1105 ± 0.0023 μmol NH4+ h-1 , and 0.2795 ± 0.1249 μmol h-1 in S. lessoniana. Squid gills showed a higher transport rate into perfusate blood than octopus gills.
Pharmacological studies of sodium-hydrogen exchanger (NHE) in NH4+ and H+ transport on cephalopod gills
In squid gills, the average values of transport rates from the basolateral side to the perfusate blood were 0.251~0.301 μmol h-1 under 2.5, 5 and 10 μM EIPA treatments, which were not significantly different from the control group (Figure 4A; Supplementary Table S2). Besides, the average values of transport rates from the apical side to the bath seawater under 2.5, 5 and 10 μM EIPA treatments were 0.081~0.173 μmol h-1 (Figure 4A; Supplementary Table S2), which were also not significantly different from the control value (0.141 ± 0.066 μmol h-1 ). Additionally, the average values of H+ transport rates in squid gills were maintained within the range of 0.001~0.008 μmol H+ h-1 and 0.006~0.015 μmol H+ h-1 from the basolateral side to the perfusate and from the apical side to the bath, respectively, under EIPA treatments (Figure 4B; Supplementary Table S2).
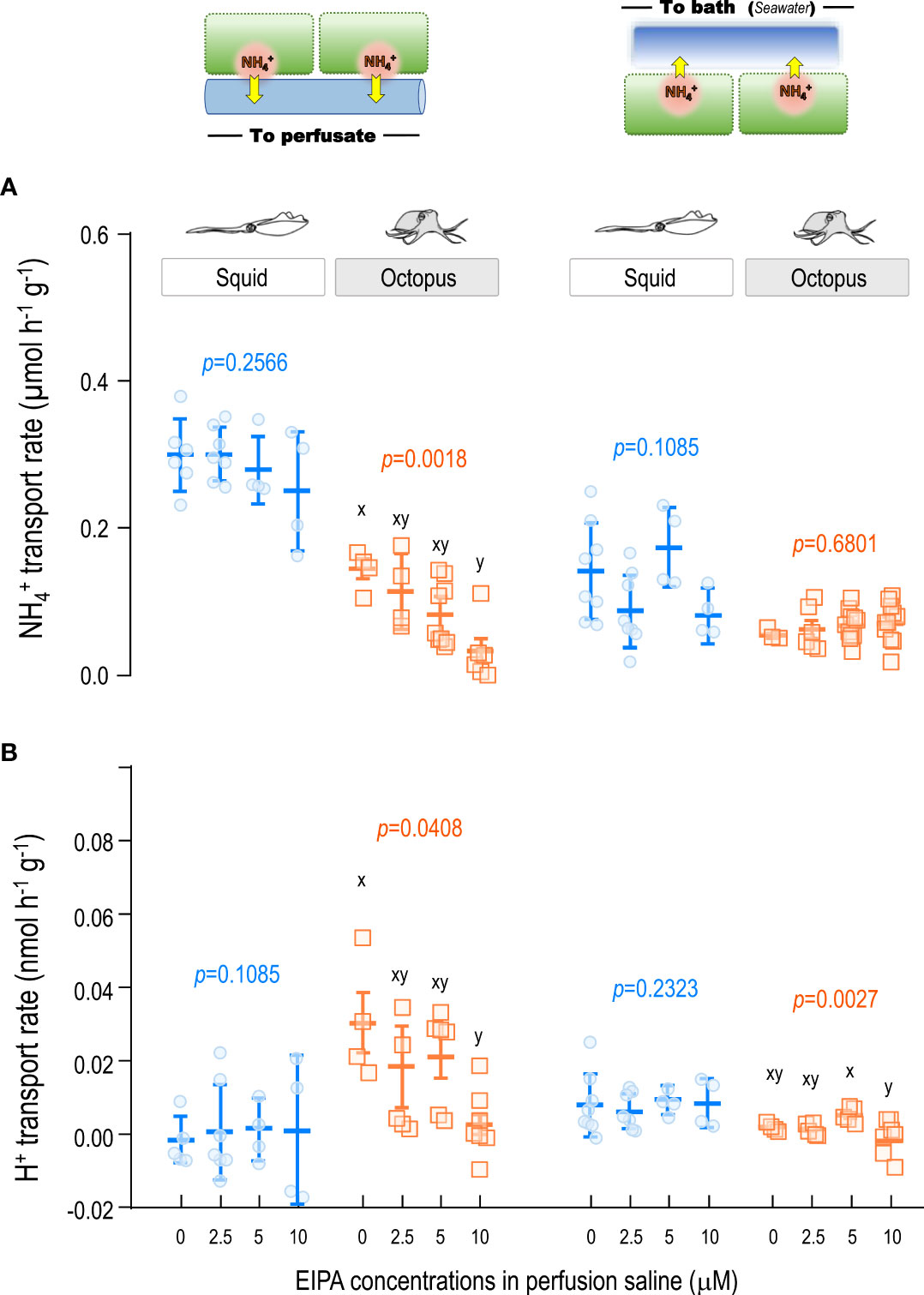
Figure 4 Effects of NHE inhibitor EIPA on the and H+ transport rates in gill epithelium of squid and octopus by ex-vivo perfusion approach. Different dosages of the NHE inhibitor EIPA were tested for their effects on the (A) and H+ (B) transport rates in squid and octopus gills by ex-vivo perfusion. Different letters indicate significant differences among perfused inhibitor dosages (p < 0.05; One-way ANOVA, Tukey’s pairwise comparisons).
Moreover, EIPA was also perfused to examine the effects of NHE inhibition on the and H+ transport in octopus gills. The NH4+ transport rates from the basolateral side to the perfusate blood decreased by approximately 30%, 45% and 84% under 2.5, 5 and 10 μM EIPA treatments, respectively, which showed a significantly decreased tendency (p=0.0018) compared with the control group (Figure 4A; Supplementary Table S2). Moreover, the transport rates from the apical side to the bath were 0.062~0.070 μmol h-1 under different dosages of EIPA treatments, which were not significantly different from the control ones (0.054 ± 0.006 μmol h-1 ) (Figure 4A; Supplementary Table S2). For the estimation of H+ transport rates, compared to the control group, a decrease of approximately 39%, 30%, and 91% from the basolateral side to the perfusate blood was observed under 5, 7.5 and 10 μM EIPA perfused octopus gills, respectively. It was also observed that the H+ transport from the apical side to the bath was reduced by approximately 32%~95% under different dosages of EIPA treatment (Figure 4B; Supplementary Table S2).
Pharmacological studies of vacuolar H+ ATPase (VHA) in NH4+ and H+ transport on cephalopod gills
In squid gills, under 2.5 and 5 μM of BafA1 treatment, the transport rate from the basolateral side to the perfusate was significantly increased by about 93% and 91%, respectively, compared with the control counterpart. However, the dosage of 7.5 μM of BafA1 treatment would not affect the transport rate (Figure 5A; Supplementary Table S3). While estimating the transport rate from the apical side to the bath seawater, the 2.5 μM of BafA1 treatment would result in an increased level (0.268 ± 0.072 μmol h-1 ) of about 2.88-fold higher than the control counterpart (0.093 ± 0.034 μmol h-1 ). Other dosages (5 and 7.5 μM) of BafA1 treatments would not affect the transport from the apical side to the bath (Figure 5A). While we further examined the H+ transport rates from the basolateral side to the perfusate blood, the 2.5 and 5 μM of BafA1 treatment would apparently result in about 9.0- and 4.9-fold decrease compared to the control group, respectively (Figure 5A). And the dosage of 7.5 μM of BafA1 treatment would not affect the H+ transport from the basolateral side to the perfusate. Besides, the average values of H+ transport rates from the apical side to the bath seawater were 0.005~0.009 μmol H+ h-1 under 2.5, 5 and 7.5 μM BafA1 treatments, which were not significantly different from the control group (Figure 5A; Supplementary Table S3).
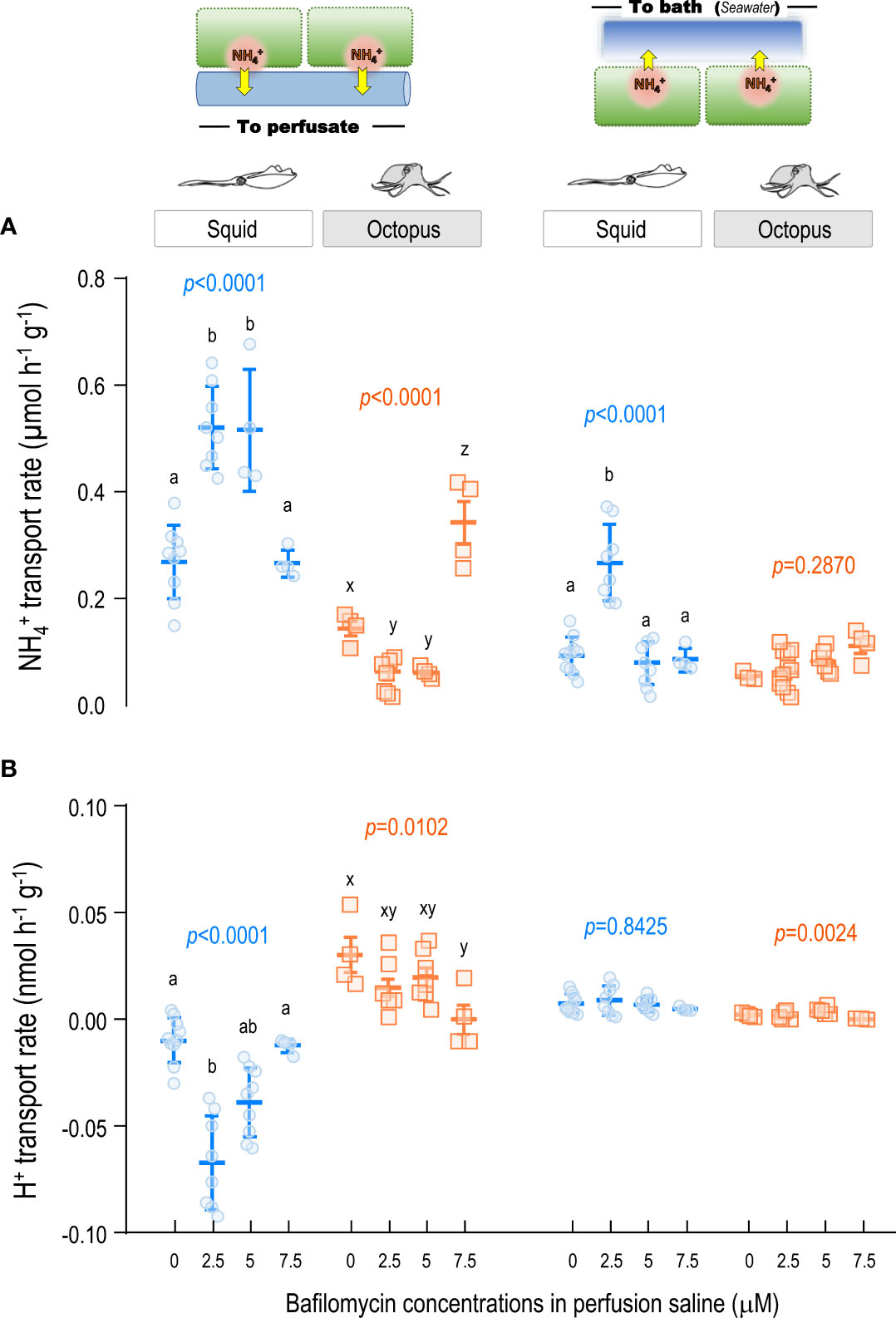
Figure 5 Effects of VHA inhibitor bafilomycin on the and H+ transport rates in gill epithelium of squid and octopus by ex-vivo perfusion approach. Different dosages of the VHA inhibitor bafilomycin were tested for their effects on the (A) and H+ (B) transport rates in squid and octopus gills by ex-vivo perfusion. Different letters indicate significant differences among perfused inhibitor dosages (p < 0.05; One-way ANOVA, Tukey’s pairwise comparisons).
Moreover, in octopus gills, the transport rate from the basolateral side to the perfusate would decrease to 0.064 ± 0.042 μmol h-1 (42% decrement) and 0.061 ± 0.010 μmol h-1 (44% decrement) under 2.5 and 5 μM of BafA1 treatment, respectively. The 7.5 μM of BafA1 treatment would reversely increase (0.343 ± 0.081 μmol h-1 ) the transport from the basolateral side to the perfusate blood (Figure 5A). And the BafA1 treatments would not apparently affect the transport from the apical side to the bath seawater (p=0.2870) (Supplementary Table S3). As for the BafA1 treatment effects on the H+ transport rates in octopus gills, the BafA1 treatment would result in a significantly decreased fashion in H+ transport rates (p=0.0102) from the basolateral side to the perfusate blood (Figure 5B; Supplementary Table S3). Moreover, the H+ transport rates from the apical side to the bath seawater were also significantly affected by the BafA1 treatment (p=0024) (Figure 5B; Supplementary Table S3).
Discussion
The distinct strategies in cephalopods for NH4+ regulation and homeostasis
The modern coleoid cephalopods are widely distributed in the marine ecosystem, from the abyssal regions to the intertidal zones. By virtue of their particular locomotion patterns and intelligence, they behave with greater dominance than most marine animals of similar size (Villanueva et al., 2017). Octopods animals can swim by contracting their arms with their velar skins and thus expelling water or crawling as their primary modes of movement. In addition, the modern loliginid squids use their muscular mantle to pressurize the mantle cavity water ejecting through the siphons; therefore, they are able to move relatively rapidly with a jet propulsion pattern and discharge ink as a decoy or smoke screen (Bone et al., 1981). Several studies have proposed that cephalopods’ jet propulsion or crawling motion patterns create high energy demands as a compensatory measure for their inferior competitive ability with other marine vertebrates (O’Dor et al., 1995; Webber et al., 2000). As a result, these special locomotion types may act as a double-edged sword, causing huge nitrogenous wastes or productions from protein catabolism, which may adversely affect body growth, nervous system abnormalities, or other possible intrinsic activities imbalances. Consequently, they must adopt different behaviors and adapt their homeostasis regulation strategies to discard or exploit nitrogenous wastes in comparison with other marine animals, even between the Octopod and loliginid cephalopods.
As cephalopod species behave differently in their locomotion patterns, their natural habitats vary accordingly (O’Dor et al., 2002). The resting metabolism of Octopod and loliginid cephalopods may reflect differences in their respective life histories (Potts, 1965). In this study, the resting metabolic rate of octopus O. vulgaris was found to be significantly lower than that of squid S. lessoniana (Figure 2A). This feature suggested that their different locomotion strategies, such as crawling or jet-motion, may correlate with their different metabolic strategies. Despite the fact that jet propulsion is not an efficient locomotion pattern that requires too much energy from aerobic respiration, this pattern is capable of rapid acceleration and locomotion (O’Dor and Webber, 1991; Gemmell et al., 2021). Octopuses, on the other hand, are considered to be jet-propulsion abandonment members (Wells, 1990; Huffard, 2006). This species is an ambush predator with excellent camouflage and maneuverability, allowing it to stay in mud bottoms and caves for a long time (Kayes, 1973; Caldwell et al., 2015). In this respect, Octopods may be exposed to hypoxic and high ammonium conditions (Wells and Wells, 1983; Okutani, 1990; Seibel and Childress, 2000) and should therefore demonstrate extraordinary capacities for nitrogenous detoxification. In this study, the gills of Octopoda O. vulgaris was found to accumulate higher levels of in blood compared to that of the pelagic loliginid bigfin reef squid, S. lessoniana (Figure 4A). As a result of the “ammonium-keeping” ability of octopus blood, a chemical gradient against ambient high ammonium levels may be generated. A higher intact aerobic respiration rate in pelagic squid, and a higher transport rate to the perfusate, may result in a lower accumulation in the gills. Apart from that, is an intermediate metabolite that can be converted into urea or amino acids for maintaining intact homeostasis or buoyancy capacity (Seibel et al., 2004; Peng et al., 2017). Consequently, further studies are needed to explore those nitrogen pathways in terms of ammonium regulation in cephalopods with different lifestyles.
Among these metabolic processes, ammonia-related amino acid synthesis is one of the most important biochemical processes for maintaining organismic homeostasis (Levitt and Levitt, 2018; Voss et al., 2021). Under the Glu-Gln cycle, glutamate (Glu) is catabolized to glutamine (Gln), which coordinates with . Compared to benthic octopus, pelagic squid blood contains more glutamine, a potential carrier, while octopus retains more glutamate for the potential binding plasticity toward . In terms of the metabolic trade-off concept, benthic octopus with lower aerobic respiration rates would prefer to utilize glutamate as an alternative metabolic substrate for energy supply. In contrast, pelagic squids would eliminate accumulation by binding to glutamine and increasing transport through their gills. The metabolic trade-off adjustment may help prevent nitrogenous wastes from accumulating and may also benefit the Glu/Gln balance. Additionally, those bicarbonate-buffering molecules, such as carbonic acid (H2CO3), bicarbonate ion () and carbon dioxide (CO2), are essential for the acid-base homeostatic mechanism in both aquatic vertebrates and invertebrates (Melzner et al., 2020; Tresguerres et al., 2020). However, ammonia could also serve as the non-bicarbonate buffer molecule binding to H+, which may play a role in the acid-base balance (Hu et al., 2014; Tseng et al., 2020). Cephalopods are capable of producing and accumulating large quantities of nitrogenous , in comparison with most marine fishes of similar body weight (Packard, 1972; Lee, 1995). Consequently, cephalopods must be equipped with efficient machinery for acid-base regulation and detoxification of nitrogenous products in comparison to their athletic competitor teleosts in similar ocean habitats. In this way, cephalopod mollusks may be able to alleviate abiotic perturbations resulting from high metabolic-derived cellular acidification and nitrogenous waste accumulation. It is unclear whether there is a relationship between contents/transport significance and different locomotion types in cephalopods. The estimations of Glu and Gln levels in the blood of squids and octopuses may therefore reflect their lifestyles and locomotion patterns. Additional evidence is needed to prove this notion.
Moreover, we should also be aware that vertical migration in the ocean system is a major metabolic expenditure for many pelagic organisms, and a special mechanism for neutral buoyancy in cephalopods has been proposed (Seibel et al., 2004). In pelagic deep-sea crustacean species, the use of has been reported to be effective in maintaining buoyancy (Sanders and Childress, 1988). Cranchiidae would also store ammoniacal fluid in their unique coelomic cavity, and is subsequently accumulated in vacuoles in body tissues such as the mantle and arms (Voight et al., 1995; Seibel et al., 2004). This means nitrogenous is not a metabolic waste product for cephalopods but a useful molecule that participates in amino acid metabolism, acid-base regulation, and even buoyancy locomotion.
NH4+ and H+ transport machinery in the branchial epithelium of squid and octopus
In most aquatic animals, ammonia is a toxic chemical that has detrimental effects on the nervous system, metabolic disorders, and other severe pathological conditions (Wood et al., 1995; Weiner and Verlander, 2016). For marine animals with an active lifestyle, gills serve as an efficient organ to deal with this biological stressor. Based on earlier findings in cephalopods, the renal appendages and gills are the most important organs for regulating levels. In addition to secreting ammonia, the renal appendages are also believed to produce urine by combining urea and water to regulate osmotic pressure (Potts, 1965). However, renal appendages are able to excrete less than 1% of ammonium. Therefore, it was concluded that the gill was the primary organ for excreting ammonium in cephalopods (Potts, 1965).
The excretion of ammonia can occur either in the form of non-ionized NH3 or ammonium () by direct diffusion through paracellular pathways or through Rhesus (Rh) glycoproteins that work with apical NHEs (Randall and Ip, 2006; Wright and Wood, 2009; Liu et al., 2013; Hu et al., 2017; Clifford et al., 2022), and the acid-trapping mechanism is hypothesized to be important. Under conditions of high environmental ammonia, the consumption of metabolic acid in pacific hagfish (Eptatretus stoutii) was significantly increased, accompanied by the conversion of NH3 to (Clifford et al., 2015). The mRNA and protein levels of renal Rhcg1 were stimulated in common carp (Cyprinus carpio) under acidic conditions, along with upregulation of several ion transporters, including the NHE, VHA, and NKA (Wright et al., 2014). Besides, the basolateral sodium-potassium ATPase (NKA) actively creates the concentration gradient of Na+ (energy coming from ATP consumption), and the driving force to process the NHEs works by Na+ gradient. Therefore, the secondary active transport manner by NHE homologs is essential for acid-base regulation; moreover, coordination of excretion by combining H+ with NH3. According to a previous study in the crustacean Carcinus maenas, H+ would trap NH3 within intracellular vesicles to form , thereby allowing H+ secretion through this potential pathway for ammonia excretion (Weihrauch et al., 2002). As for cephalopods, recent molecular insights into the regulation of and H+ have also been well demonstrated (Hu et al., 2014; Hu et al., 2015; Hu et al., 2017). Coleoid cephalopods possess sophisticated vertebrate-like /H+ regulatory mechanisms and rely extensively upon branchial epithelia for homeostasis control. In the semitubular environment of cephalopod gills, an acid-trapping mechanism for has also been proposed based on morphological, functional, and molecular studies (Hu and Tseng, 2017). Thus, homeostasis in aquatic organisms is not only controlled by direct diffusion or by transepithelial transporters but also by the H+ secretion machinery.
As part of this study, EIPA and BafA1 were applied to pharmacological perfusion experiments on squid and octopus gills. Inhibition of NHEs by EIPA appeared to affect and H+ transport in octopus gills. However, inhibition of NHE by EIPA appears to have no significant effect on either or H+ transport in the squid epithelium from basolateral or apical sites. In this regard, compared to the pelagic species, the crawling cephalopod octopus would rely on the secondary active transport route (NHE) via the electrochemical Na+ gradient to maintain and H+ transport. In case of elevated ambient and H+ concentrations, octopuses with less aerobic respiration capacity have an energy-saving strategy to maintain homeostasis and hunt prey with less energy consumption. It should be noted that, while this pharmacological trial utilized the NHE inhibitor EIPA in octopus gills, we found that the transport towards the apical site was not significantly affected as was shown toward the basolateral site and the effective feature in H+ transport towards the apical site. Accordingly, it appears that another apical transporter, such as the RhP homolog, that can facilitate transport in the gill epithelium of octopus (Hu et al., 2017). Moreover, it is also inferred that the level of excreted by the octopus gills is primarily determined by the transport of from the branchial cells into the blood. It is possible that this basolateral machinery may be operated by other transport molecules, such as Rhp1b, which has been suggested to be present in the branchiae of marine polychaetes (Eurythoe complanata) (Weihrauch and Allen, 2018). The “ammonium-keeping” significance of chemical gradients against environmental nitrogenous toxicity is the reason for a higher concentration in the ctenidial vein of octopus than in squid. It would be physiologically meritorious to discover what caused the difference in transport rates between octopus and squid. A possible explanation for this significance may be the presence of different transport molecules with different isoforms (Weihrauch and Allen, 2018). transport molecules have different functional characteristics, enabling organisms to adapt to a variety of habitats with different mobility styles, as well as have significantly greater physiological plasticity due to the well-regulated content, essential for maintaining intact homeostasis and metabolic balance in various environmental conditions.
Apart from H+ transport by NHE, the primary active transport by VHA with ATP-dependent operation appears to be irreplaceable. Observing the effects of the VHA inhibitor bafilomycin (BafA1) on and H+ transport in the gills of squid and octopus, the influence patterns were quite apparent. The BafA1 inhibits the H+ transport rate toward the blood in squid gills, but the transport rates in gill epithelium are reversely stimulated, suggesting that other alternative molecules, such as the RhP homologs, may contribute to the efficient transport capacity of squid to maintain acid-base equilibrium (Hu et al., 2014). Meanwhile, the fact that BafA1 would inhibit both and H+ transport in octopus gills indicates that VHA is also essential for homeostasis in crawling cephalopods. Additionally, octopus VHA may be involved in the post-translational control of vesicular excretion via a membrane trafficking pathway that represents an alternative method of excreting vesicular (Hu et al., 2017). As for the transport towards the apical site, it was not significantly affected. This may also be due to the fact that the excretion in the octopus gills is predominantly transported from the branchial cells into the blood, and/or the apical NHE may also be involved in the excretion of . More importantly, the VHA in the gills of octopus showed distinct distribution within the endothelial cells of blood vessels (Hu et al., 2017). Accordingly, our preliminary data indicate that high concentrations (10 μM in saline) of perfused BafA1 affect the morphology of the gills of octopuses during the perfusion procedure. Therefore, the maximum inhibitory concentration of 7.5 μM BafA1 was used in both species during the VHA-inhibition studies.
It is true that the epithelial basolateral NKA actively creates the concentration gradient of Na+ (energy derived from ATP consumption); however, the SW habitat provides a natural Na+-rich driving force for the NHEs to work. Natural Na+ gradients in SW drive the secondary active transport mechanism through the NHE homologs, which is crucial for the regulation of and H+. As a result of the energy constraint concerns, the implementation of NHE traits, as well as the energy-consuming VHA in the gills for body fluid homeostasis, offers a potential benefit for reducing energy consumption. It was evident from this feature that the octopus would take advantage of both secondary and primary active transport mechanisms to maintain and H+ homeostasis. As energy is a major constraint on physiological processes, trade-offs between energy allocations to different physiological processes under the concern of energy efficiency could be considered to be a cellular adjustment. In order to achieve regular homeostasis, the benthic crawling octopuses may act as “misers,” which means that they deliberate with the amount of energy consumed (Semmens et al., 2004; Onthank and Cowles, 2011). As an alternative, the acid-base and ammonium regulation in pelagic squid could be largely controlled by primary active transport in an energy-intensive manner since they possess higher aerobic respiration rate and more efficient regulation machinery than benthic crawling octopus. Due to their similar athletic hunting strategies and jet propulsion locomotion pattern, the pelagic squid may behave as a “spendthrift” for energy consumption that is not concerned with the food source (Shulman et al., 2002; Gao et al., 2022). This characteristic implies that pelagic squids are able to generate enough energy for intact physiological adjustments, such as body growth and nitrogenous waste elimination.
Conclusion
Cephalopods are ancient mollusks that can be found in a wide variety of ecological niches in the ocean, ranging from the intertidal zone to the deep ocean. To adapt to their lifestyles in various types of habitats, modern cephalopods have evolved a variety of locomotory modes. In general, cephalopods have relatively high metabolic rates due to their less efficient swimming mode by jet propulsion. This energy-intensive lifestyle is primarily fueled by the rapid digestion of protein diets, which may lead to metabolic ammonia/ammonium accumulation and acid-base disturbances. The inhibition of secondary active transport machinery significantly impaired and H+ excretory processes in the gills of benthic octopus, but not in pelagic squid. However, the inhibition of primary active transport machinery significantly impairs the excretion of and H+ in both benthic and pelagic coleoid cephalopods. Pelagic squids prefer active transport for /H+ excretion in an energy-dependent manner, whereas benthic octopuses use both secondary and primary active transport for homeostasis. Consequently, the pelagic squid, in contrast to the benthic octopus, may have developed /H+-transport mechanisms in an energy-intensive manner to avoid toxic nitrogenous effects in the blood.
Data availability statement
The original contributions presented in the study are included in the article/Supplementary Material. Further inquiries can be directed to the corresponding author.
Ethics statement
The animal study was reviewed and approved by Academia Sinica Institutional Animal Care and Utilization Committee (approval no. BSF17-12-1158).
Author contributions
M-WL, P-LK, and P-HS conceived the study, designed, and carried out the metabolic and perfusion analysis. M-WL and Y-CT wrote the first draft of the paper, and all co-authors commented on and approved the manuscript.
Funding
This study was financially supported by grants to Y-CT from the Ministry of Science and Technology, Taiwan, Republic of China (MOST 107-2311-B-001 -015 -MY3).
Acknowledgments
The authors express their appreciation to the Marine Research Station (ICOB, Academia Sinica) for squid and octopus rearing during the experiments.
Conflict of interest
The authors declare that the research was conducted in the absence of any commercial or financial relationships that could be construed as a potential conflict of interest.
Publisher’s note
All claims expressed in this article are solely those of the authors and do not necessarily represent those of their affiliated organizations, or those of the publisher, the editors and the reviewers. Any product that may be evaluated in this article, or claim that may be made by its manufacturer, is not guaranteed or endorsed by the publisher.
Supplementary material
The Supplementary Material for this article can be found online at: https://www.frontiersin.org/articles/10.3389/fmars.2022.971764/full#supplementary-material
References
Bone Q., Pulsford A., Chubb A. D. (1981). Squid mantle muscle. J. Mar. Biol. Assoc. U. K. 61, 327–342. doi: 10.1017/S0025315400046981
Boucher-Rodoni R., Mangold K. (1989). Respiration and nitrogen excretion by the squid Loligo forbesi. Mar. Biol. 103, 333–338. doi: 10.1007/BF00397267
Boucher-Rodoni R., Mangold K. (1995). Ammonia production in cephalopods, physiological and evolutionary aspects. Mar. Freshw. Behav. Physiol. 25, 53–60. doi: 10.1080/10236249409378907
Caldwell R. L., Ross R., Rodaniche A., Huffard C. L. (2015). Behavior and body patterns of the larger pacific striped octopus. PloS One 10, e0134152. doi: 10.1371/journal.pone.0134152
Chang W. J., Hwang P. P. (2011). Development of zebrafish epidermis. Birth Defects Res. C Embryo Today 93, 205–214. doi: 10.1002/bdrc.20215
Chung W.-S., Kurniawan N. D., Marshall N. J. (2020). Toward an MRI-based mesoscale connectome of the squid brain. Iscience 23, 100816. doi: 10.1016/j.isci.2019.100816
Clifford A. M., Goss G. G., Wilkie M. P. (2015). Adaptations of a deep sea scavenger: High ammonia tolerance and activeexcretion by the pacific hagfish (Eptatretus stoutii). Comp. Biochem. Physiol. A Mol. Integr. Physiol. 182, 64–74. doi: 10.1016/j.cbpa.2014.12.010
Clifford A. M., Wilkie M. P., Edwards S. L., Tresguerres M., Goss G. G. (2022). Dining on the dead in the deep: Active Excretion via Na+/H+ exchange in the highly ammonia tolerant pacific hagfish, Eptatretus stoutii. Acta Physiol. 00, e13845. doi: 10.1111/apha.13845
Evans D. H., Piermarini P. M., Choe K. P. (2005). The multifunctional fish gill: Dominant site of gas exchange, osmoregulation, acid-base regulation, and excretion of nitrogenous waste. Physiol. Rev. 85, 97–177. doi: 10.1152/physrev.00050.2003
Gao X., Gong Y., Chen X., Li Y. (2022). Dietary shifts and niche partitioning throughout ontogeny reduce intraspecific competition in a pelagic generalist predator. Mar. Ecol. Prog. Ser. 692, 81–97. doi: 10.3354/meps14079
Gemmell B. J., Dabiri J. O., Colin S. P., Costello J. H., Townsend J. P., Sutherland K. R. (2021). Cool your jets: Biological jet propulsion in marine invertebrates. J. Exp. Biol. 224, jeb222083. doi: 10.1242/jeb.222083
Histon K. (2012). Paleoenvironmental and temporal significance of variably colored Paleozoic orthoconic nautiloid cephalopod accumulations. Palaeogeogr. Palaeoclimatol. Palaeoecol. 367, 193–208. doi: 10.1016/j.palaeo.2012.07.008
Holmes R. M., Aminot A., Kérouel R., Hooker B. A., Peterson B. J. (1999). A simple and precise method for measuring ammonium in marine and freshwater ecosystems. Can. J. Fish Aquat. Scci. 56, 1801–1808. doi: 10.1139/f99-128
Huang P. C., Liu T. Y., Hu M. Y., Casties I., Tseng Y. C. (2020). Energy and nitrogenous waste from glutamate/glutamine catabolism facilitates acute osmotic adjustment in non-neuroectodermal branchial cells. Sci. Rep. 10, 1–17. doi: 10.1038/s41598-020-65913-1
Huffard C. (2006). Locomotion by Abdopus aculeatus (Cephalopoda: Octopodidae): Walking the line between primary and secondary defenses. J. Exp. Biol. 209, 3697–3707. doi: 10.1242/jeb.02435
Hu M. Y., Guh Y.-J., Stumpp M., Lee J.-R., Chen R.-D., Sung P.-H., et al. (2014). Branchial -dependent acid–base transport mechanisms and energy metabolism of squid (Sepioteuthis lessoniana) affected by seawater acidification. Front. Zool. 11, 1–17. doi: 10.1186/s12983-014-0055-z
Hu M. Y., Hwang P.-P., Tseng Y.-C. (2015). Recent advances in understanding trans-epithelial acid-base regulation and excretion mechanisms in cephalopods. Tissue Barriers 3, e1064196. doi: 10.1080/21688370.2015.1064196
Hu M. Y., Sung P.-H., Guh Y.-J., Lee J.-R., Hwang P.-P., Weihrauch D., et al. (2017). Perfused gills reveal fundamental principles of pH regulation and ammonia homeostasis in the cephalopod Octopus vulgaris. Front. Physiol. 8, 162. doi: 10.3389/fphys.2017.00162
Hu M., Tseng Y.-C. (2017). “Acid–base regulation and ammonia excretion in cephalopods: An ontogenetic overview,”, in Acid-base balance and nitrogen excretion in invertebrates (Cham: Springer), 275–298. doi: 10.1007/978-3-319-39617-0_11
Hu M. Y., Tseng Y. C., Lin L. Y., Chen P. Y., Charmantier-Daures M., Hwang P. P., et al. (2011). New insights into ion regulation of cephalopod molluscs: A role of epidermal ionocytes in acid-base regulation during embryogenesis. Am. J. Physiol. Regul. Integr. Comp. Physiol. 301, R1700–R1709. doi: 10.1152/ajpregu.00107.2011
Hwang P. P., Lee T. H., Lin L. Y. (2011). Ion regulation in fish gills: Recent progress in the cellular and molecular mechanisms. Am. J. Physiol. Regul. Integr. Comp. Physiol. 301, R28–R47. doi: 10.1152/ajpregu.00047.2011
Hwang P.-P., Lin L.-Y. (2013). “Gill ionic transport, acid-base regulation, and nitrogen excretion”. The Physiology of Fishes 4th Edn, eds Evans D. H., Claiborne J. B (Boca Raton, FL: CRC Press) 4, 205–233.
Jereb P., Roper C. F. (2010). “Cephalopods of the world-an annotated and illustrated catalogue of cephalopod species known to date,” in Myopsid and oegopsid squids, Vol 2. (Rome: Fao).
Kayes R. (1973). The daily activity pattern of Octopus vulgar is in a natural habitat. Mar. Freshw. Behav. Physiol. 2, 337–343. doi: 10.1080/10236247309386935
Kroeger B., Yun-Bai Z. (2009). Pulsed cephalopod diversification during the Ordovician. Palaeogeogr. Palaeoclimatol. Palaeoecol. 273, 174–183. doi: 10.1016/j.palaeo.2008.12.015
Kuan P.-L., You J.-Y., Wu G.-C., Tseng Y.-C. (2022). Temperature increases induce metabolic adjustments in the early developmental stages of bigfin reef squid (Sepioteuthis lessoniana). Sci. Total Environ. 844, 156962. doi: 10.1016/j.scitotenv.2022.156962
Lee P. (1995). Nutrition of cephalopods: Fueling the system. Mar. Freshw. Behav. Physiol. 25, 35–51. doi: 10.1080/10236249409378906
Levitt D. G., Levitt M. D. (2018). A model of blood-ammonia homeostasis based on a quantitative analysis of nitrogen metabolism in the multiple organs involved in the production, catabolism, and excretion of ammonia in humans. Clin. Exp. Gastroenterol. 11, 193–215. doi: 10.2147/CEG.S160921
Liu S. T., Horng J. L., Chen P. Y., Hwang P. P., Lin L. Y. (2016). Salt secretion is linked to acid-base regulation of ionocytes in seawater-acclimated medaka: New insights into the salt-secreting mechanism. Sci. Rep. 6, 31433. doi: 10.1038/srep31433
Liu S. T., Tsung L., Horng J. L., Lin L. Y. (2013). Proton-facilitated ammonia excretion by ionocytes of medaka (Oryzias latipes) acclimated to seawater. Am. J. Physiol. Regul. Integr. Comp. Physiol. 305, R242–R251. doi: 10.1152/ajpregu.00047.2013
Mather J. A., Griebel U., Byrne R. A. (2010). Squid dances: An ethogram of postures and actions of sepioteuthis sepioidea squid with a muscular hydrostatic system. Mar. Freshw. Behav. Physiol. 43, 45–61. doi: 10.1080/10236241003660771
Melzner F., Mark F. C., Seibel B. A., Tomanek L. (2020). Ocean acidification and coastal marine invertebrates: Tracking CO2 effects from seawater to the cell. Annu. Rev. Mar. Sci. 12, 499–523. doi: 10.1146/annurev-marine-010419-010658
Nishi T., Forgac M. (2002). The vacuolar (H+)-ATPases–nature’s most versatile proton pumps. Nat. Rev. Mol. Cell Biol. 3, 94–103. doi: 10.1038/nrm729
O’Dor R. (2002). Telemetered cephalopod energetics: Swimming, soaring, and blimping. Integr. Comp. Biol. 42, 1065–1070. doi: 10.1093/icb/42.5.1065
O’Dor R., Adamo S., Aitken J., Andrade Y., Finn J., Hanlon R., et al. (2002). Currents as environmental constraints on the behavior, energetics and distribution of squid and cuttlefish. Bull. Mar. Sci. 71, 601–617.
O’Dor R., Hoar J., Webber D., Carey F., Tanaka S., Martins H., et al. (1995). Squid (Loligo forbesi) performance and metabolic rates in nature. Mar. Freshw. Behav. Physiol. 25, 163–177. doi: 10.1080/10236249409378915
O’Dor R., Webber D. (1991). Invertebrate athletes: Trade-offs between transport efficiency and power density in cephalopod evolution. J. Exp. Biol. 160, 93–112. doi: 10.1242/jeb.160.1.93
Okutani T. (1990). Squids, cuttlefish and octopuses. Mar. Freshw. Behav. Physiol. 18, 1–17. doi: 10.1080/10236249009378778
Onthank K. L., Cowles D. L. (2011). Prey selection in Octopus rubescens: Possible roles of energy budgeting and prey nutritional composition. Mar. Biol. 158, 2795–2804. doi: 10.1007/s00227-011-1778-4
Packard A. (1972). Cephalopods and fish: The limits of convergence. Biol. Rev. 47, 241–307. doi: 10.1111/j.1469-185X.1972.tb00975.x
Peng R. B., Le K. X., Wang P. S., Wang Y., Han Q. X., Jiang X. M. (2017). Detoxification pathways in response to environmental ammonia exposure of the cuttlefish, Sepia pharaonis: Glutamine and urea formation. J. World Aquac Soc. 48, 342–352. doi: 10.1111/jwas.12341
Potts W. T. W. (1965). Ammonia excretion in Octopus dolfeini. Comp. Biochem. Physiol. 14, 339–354. doi: 10.1016/0010-406X(65)90209-4
Randall D. J., Ip Y. K. (2006). Ammonia as a respiratory gas in water and air-breathing fishes. Respir. Physiol. Neurobiol. 154, 216–225. doi: 10.1016/j.resp.2006.04.003
Sanders N., Childress J. (1988). Ion replacement as a buoyancy mechanism in a pelagic deep-sea crustacean. J. Exp. Biol. 138, 333–343. doi: 10.1242/jeb.138.1.333
Schaffhauser D., Fine M., Tabata M., Goda T., Miyahara Y. (2016). Measurement of rapid amiloride-dependent pH changes at the cell surface using a proton-sensitive field-effect transistor. Biosensors 6, 11. doi: 10.3390/bios6020011
Scheel D., Bisson L. (2012). Movement patterns of giant pacific octopuses, Enteroctopus dofleini (Wülker 1910). J. Exp. Mar. Biol. Ecol. 416, 21–31. doi: 10.1016/j.jembe.2012.02.004
Seibel B. A., Childress J. J. (2000). Metabolism of benthic octopods (Cephalopoda) as a function of habitat depth and oxygen concentration. Deep Sea Res. Part I Oceanogr. Res. Pap 47, 1247–1260. doi: 10.1016/S0967-0637(99)00103-X
Seibel B. A., Drazen J. C. (2007). The rate of metabolism in marine animals: Environmental constraints, ecological demands and energetic opportunities. Philos. Trans. R Soc. Lond B Biol. Sci. 362, 2061–2078. doi: 10.1098/rstb.2007.2101
Seibel B. A., Goffredi S. K., Thuesen E. V., Childress J. J., Robison B. H. (2004). Ammonium content and buoyancy in midwater cephalopods. J. Exp. Mar. Biol. Ecol. 313, 375–387. doi: 10.1016/j.jembe.2004.08.015
Semmens J., Pecl G., Villanueva R., Jouffre D., Sobrino I., Wood J., et al. (2004). Understanding octopus growth: Patterns, variability and physiology. Mar. Freshw. Res. 55, 367–377. doi: 10.1071/MF03155
Shulman G., Chesalin M., Abolmasova G., Yuneva T., Kideys A. (2002). Metabolic strategy in pelagic squid of genus Sthenoteuthis (Ommastrephidae) as the basis of high abundance and productivity: An overview of the soviet investigations. Bull. Mar. Sci. 71, 815–836.
Tovey K. J., Brauner C. J. (2018). Effects of water ionic composition on acid–base regulation in rainbow trout, during hypercarbia at rest and during sustained exercise. J. Comp. Physiol. B 188, 295–304. doi: 10.1007/s00360-017-1129-y
Tresguerres M., Clifford A. M., Harter T. S., Roa J. N., Thies A. B., Yee D. P., et al. (2020). Evolutionary links between intra-and extracellular acid–base regulation in fish and other aquatic animals. J. Exp. Zool. A Ecol. Integr. Physiol. 333, 449–465. doi: 10.1002/jez.2367
Tseng Y.-C., Hu M. Y., Stumpp M., Lin L.-Y., Melzner F., Hwang P.-P. (2013). CO2-driven seawater acidification differentially affects development and molecular plasticity along life history of fish (Oryzias latipes). Comp. Biochem. Physiol. A Mol. Integr. Physiol. 165, 119–130. doi: 10.1016/j.cbpa.2013.02.005
Tseng Y. C., Hwang P. P. (2008). Some insights into energy metabolism for osmoregulation in fish. Comp. Biochem. Physiol. C Toxicol. Pharmacol. 148, 419–429. doi: 10.1016/j.cbpc.2008.04.009
Tseng Y. C., Yan J. J., Furukawa F., Hwang P. P. (2020). Did acidic stress resistance in vertebrates evolve as Na+/H+ exchanger-mediated ammonia excretion in fish? BioEssays 42, e1900161. doi: 10.1002/bies.201900161
Villanueva R., Perricone V., Fiorito G. (2017). Cephalopods as predators: A short journey among behavioral flexibilities, adaptions, and feeding habits. Front. Physiol. 8, 598. doi: 10.3389/fphys.2017.00598
Voight J. R., Pörtner H. O., O’dor R. K. (1995). A review of ammonia-mediated buoyancy in squids (cephalopoda: Teuthoidea). Mar. Freshw. Behav. Physiol. 25, 193–203. doi: 10.1080/10236249409378917
Voss C. M., Arildsen L., Nissen J. D., Waagepetersen H. S., Schousboe A., Maechler P., et al. (2021). Glutamate dehydrogenase is important for ammonia fixation and amino acid homeostasis in brain during hyperammonemia. Front. Neurosci. 15, 646291. doi: 10.3389/fnins.2021.646291
Wang M.-C., Hsu M.-T., Lin C.-C., Hsu S.-C., Chen R.-D., Lee J.-R., et al. (2022). Adaptive metabolic responses in a thermostabilized environment: Transgenerational trade-off implications from tropical tilapia. Sci. Total Environ. 806, 150672. doi: 10.1016/j.scitotenv.2021.150672
Webber D. M., Aitken J. P., O’dor R. K. (2000). Costs of locomotion and vertic dynamics of cephalopods and fish. Physiol. Biochem. Zool. 73, 651–662. doi: 10.1086/318100
Weihrauch D., Allen G. J. (2018). Ammonia excretion in aquatic invertebrates: New insights and questions. J. Exp. Biol. 221, jeb169219. doi: 10.1242/jeb.169219
Weihrauch D., Ziegler A., Siebers D., Towle D. W. (2002). Active ammonia excretion across the gills of the green shore crab Carcinus maenas: Participation of Na+/K+-ATPase, V-type h+-ATPase and functional microtubules. J. Exp. Biol. 205, 2765–2775. doi: 10.1242/jeb.205.18.2765
Weiner I. D., Verlander J. W. (2016). Recent advances in renal ammonia metabolism and transport. Curr. Opin. Nephrol. Hypertens. 25, 436. doi: 10.1097/MNH.0000000000000255
Wells M. (1990). Oxygen extraction and jet propulsion in cephalopods. Can. J. Zool. 68, 815–824. doi: 10.1139/z90-117
Wells M. J., Clarke A. (1996). Energetics: The costs of living and reproducing for an individual cephalopod. Philos. Trans. R Soc. Lond B Biol. Sci. 351, 1083–1104. doi: 10.1098/rstb.1996.0095
Wells M., Wells J. (1983). The circulatory response to acute hypoxia in octopus. J. Exp. Biol. 104, 59–71. doi: 10.1242/jeb.104.1.59
Wood J. W., Day C. L., Lee P., O’dor R. K. (2000). CephBase: Testing ideas for cephalopod and other species-level databases. Oceanography 13, 14–20. doi: 10.5670/oceanog.2000.03
Wood C. M., Pärt P., Wright P. A. (1995). Ammonia and urea metabolism in relation to gill function and acid-base balance in a marine elasmobranch, the spiny dogfish (Squalus acanthias). J. Exp. Biol. 198, 1545–1558. doi: 10.1242/jeb.198.7.1545
Wright P. A. (1995). Nitrogen excretion: Three end products, many physiological roles. J. Exp. Biol. 198, 273–281. doi: 10.1242/jeb.198.2.273
Wright P. A., Wood C. M. (2009). A new paradigm for ammonia excretion in aquatic animals: Role of rhesus (Rh) glycoproteins. J. Exp. Biol. 212, 2303–2312. doi: 10.1242/jeb.023085
Wright P. A., Wood C. M., Wilson J. M. (2014). Rh Versus pH: The role of rhesus glycoproteins in renal ammonia excretion during metabolic acidosis in a freshwater teleost fish. J. Exp. Biol. 217, 2855–2865. doi: 10.1242/jeb.098640
Keywords: cephalopods, ecological niches, acid-base regulations, ammonium excretion, perfusion
Citation: Lin M-W, Kuan P-L, Sung P-H and Tseng Y-C (2022) Metabolic trade-offs associated with homeostatic adjustments in pelagic and benthic cephalopods: Comparative evaluations of NH4+/H+ transport machinery in gills. Front. Mar. Sci. 9:971764. doi: 10.3389/fmars.2022.971764
Received: 17 June 2022; Accepted: 16 September 2022;
Published: 05 October 2022.
Edited by:
Anna Di Cosmo, University of Naples Federico II, ItalyReviewed by:
Susumu Hyodo, The University of Tokyo, JapanJaruwat Nabhitabhata, Prince of Songkla University, Thailand
Copyright © 2022 Lin, Kuan, Sung and Tseng. This is an open-access article distributed under the terms of the Creative Commons Attribution License (CC BY). The use, distribution or reproduction in other forums is permitted, provided the original author(s) and the copyright owner(s) are credited and that the original publication in this journal is cited, in accordance with accepted academic practice. No use, distribution or reproduction is permitted which does not comply with these terms.
*Correspondence: Yung-Che Tseng, eWN0c2VuZ0BnYXRlLnNpbmljYS5lZHUudHc=