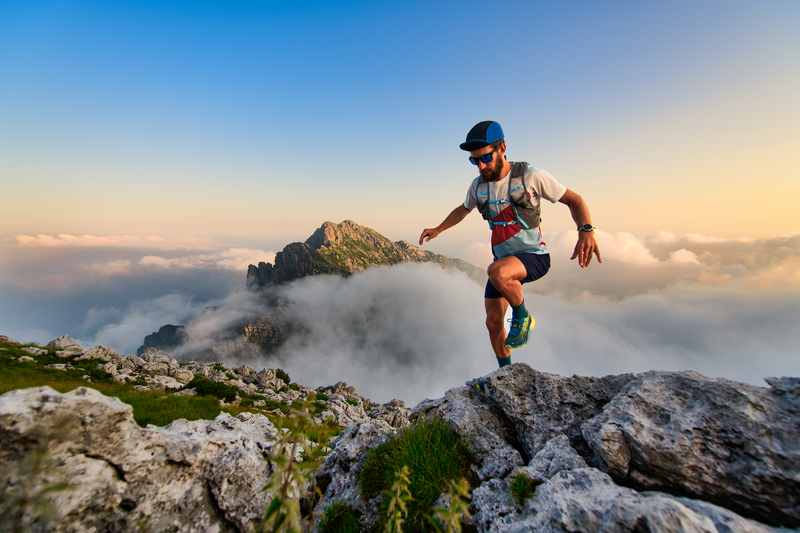
94% of researchers rate our articles as excellent or good
Learn more about the work of our research integrity team to safeguard the quality of each article we publish.
Find out more
BRIEF RESEARCH REPORT article
Front. Mar. Sci. , 04 October 2022
Sec. Marine Fisheries, Aquaculture and Living Resources
Volume 9 - 2022 | https://doi.org/10.3389/fmars.2022.971441
This article is part of the Research Topic Exploration and Utilization of Marine and Freshwater High-Value Biological Resources View all 12 articles
High bicarbonate levels and low temperature may have an impact on microalgae cultivation. However, changes in cellular composition in response to the combination of the above stresses are still poorly understood. In this study, the combined effects of bicarbonate and low temperature on biochemical changes in alkaliphilic microalgae Dunaliella salina HTBS were investigated. Comparing to the control condition of 25°C without bicarbonate, the cell density was increased from 0.69 to 1.18 in the treatment condition of 0.15 M bicarbonate and low temperature (16 °C) while the lipid\protein\carbohydrate contents were increased from 34.71% to 43.94%, 22.44% to 26.03%, 22.62% to 29.18%, respectively. Meanwhile, the PUFAs, arachidonic acid (AA) and docosahexaenoic acid (DHA) contents reached to 3.52% and 4.73% with the combination of low temperature and bicarbonate, respectively, whereas they were not detected when the cells were treated with single condition. Moreover, both the chlorophyll and carotenoid contents were also detected with increased profiles in the combined treatments. As a result, the maximum photochemical efficiency but not reduced non-photochemical quenching was strengthened, which enhanced the photosynthetic performance. Additionally, our results indicated that D. salina HTBS could acclimate to the combined stress by up-regulating the activity of SOD\CAT and reducing MDA content. These findings demonstrated that the addition of a certain bicarbonate under low temperature could effectively enhance the biomass production and accumulation of AA and DHA, which would benefit the development of the microalgae industry in value-added products.
Microalgae, as photosynthetic organisms, are utilized as potential candidates for carbon sequestration and valuable compounds production with a higher growth rate and efficiency in CO2 fixation than terrestrial plants (Chaunhan et al., 2022; Jakhwal et al., 2022). Among the great diversity of metabolites in microalgal cells, polyunsaturated fatty acids (PUFAs), such as arachidonic acid (AA) and docosahexaenoic acid (DHA), have been widely used as the key ingredients for nutrient supplements and pharmaceutical industrial products. Although both AA and DHA are reported to be accumulated in microalgae under certain conditions, their biosynthesis could be greatly affected by culture medium modifications, such as nutrients supplementation, limitation, and abiotic condition changes (Almutairi, 2020).
Biosynthesis of AA and DHA is closely related to carbon metabolism and temperature changes. Studies have shown that the PUFAs are increased from 32.3% to 37.9% in Pavlova lutheri with the addition of bicarbonate from 2 mM to 18 mM in the medium, which are also the case with both biomass and lipid contents (Guihéneuf and Stengel, 2013). The biosynthesis of both lipid and AA is active in Parietochloris Incisa under high C/N conditions (Khozin-Goldberg et al., 2002). Interestingly, bicarbonate is convenient to transport and cost effective when compared with CO2, it can be considered as an excellent carbon resource for microalgae cultivation with high carbon utilization. Therefore, bicarbonate supplementation has been regarded as an effective way for the enhancement of secondary metabolites biosynthesis and growth acceleration. In contrast, PUFAs were detected with reduced content under high carbon conditions (Morales et al., 2021), and both the PUFAs biosynthesis and cell growth varied depending on the bicarbonate concentration and microalgae strain (Nunez et al., 2016). When added with bicarbonate, a large amount of cations are accumulated in both the microalgae cells and medium, which may inhibit cell division, resulting in cell death (Chen et al., 2009; Chi et al., 2014; Srinivasan et al., 2015; Ratomski et al., 2021). Due to the lack of strains tolerant to high-concentration cations, the effects of high bicarbonate on facilitating PUFAs biosynthesis in microalgae have been rarely reported.
Temperature is the other key factor which affects the biosynthesis of both AA and DHA. Low temperatures ranging from 10 °C to 25 °C trigger the PUFAs metabolic pathway, which leads to a 120% increase in PUFAs content (Jakhwal et al., 2022), and the accumulated PUFAs improve the cell membrane fluidity, which reduces the damage to cells caused by low temperatures (Lu et al., 2017). The PUFAs content increased to 35.23% when the temperature was decreased from 45 °C to 25 °C in Galdieria sp (Lu et al., 2021). Similarly, the positive effects of low temperature on PUFAs biosynthesis were also observed in the cultivation of Nannochloropsis, Isochrysis, Rhodomonas, and Dixioniella grisea (Aussant et al., 2018; Lu et al., 2021). However, microalgal growth is inhibited when PUFAs accumulate under low temperature conditions. Therefore, the strains with high bicarbonate and low-temperature tolerance are urgently needed to solve the previously mentioned problems.
In our previous study, Dunaliella salina strain HTBS, with a high tolerance to bicarbonate, was reported to be able to grow well under 70 g/L bicarbonate and low temperature. Nevertheless, the combined effects of bicarbonate supplementation and low temperature on the biochemical composition of HTBS changes are still unclear. Therefore, the objective of this study was to investigate the role of the combination of high bicarbonate and low temperature in physiological and biochemical changes in HTBS. Changes of cell density, pigments, lipid, carbohydrate, and protein contents, particularly the high value products AA and DHA content were initially monitored. Then, stress biomarkers like antioxidative enzyme superoxide dismutase (SOD), catalase (CAT) and malondialdehyde (MDA), along with Ci and nitrogen consumption curve were evaluated to elucidate the physiological mechanism of HTBS in response to the combined stress as well as to study their effects on PUFAs. These results will provide valuable information to produce PUFAs using microalgae and will benefit industrial development.
Dunaliella salina strain HTBS with high tolerance to HCO3- and low temperature was obtained and cultured to an early stationary phase, then centrifuged and washed twice with sterile seawater for inoculation based on our previous study (Hou et al., 2016). For evaluating the effects of different bicarbonate contents at low temperature on physiological and biochemical changes in HTBS, the cells were inoculated in the modified f/2 medium (750 mg/L NaNO3) under a light intensity of 80 μmol m-2 s-1 containing various bicarbonate concentrations ranging from 0 M to 0.6 M at 16°C.
During the cultivation, the cell growth was evaluated by measuring the optical density (OD) at 680 nm using a spectrometer. The algae suspension was centrifuged to harvest the cell pellets at 8,000 rpm for 5 min, the pellets were freeze dried using a vacuum freeze-drying machine. About 50 mg of cells were resuspended with 4 mL of chloroform and 2 mL of methanol, then incubated at 30°C for 16 h for lipid extraction. Then the mixture was centrifuged at 5,000 rpm for 10 min after adding another 2 mL of methanol and 3.6 mL of ddH2O. Subsequently, the organic phase was transferred to a pre-weighed glass tube and dried with N2 protection under 65 °C. The lipid was dissolved in 2.5 mL of 2% (v/v) H2SO4-methanol solution and heated at 85°C for 2.5 h. The fatty acid methyl ester (FAME) was obtained with 1 mL of n-hexane and 1 mL of saturated sodium chloride solution addition. FAME analysis was carried out using GC (GC2010, Shimadzu; SP-2560, 100m×0.25mm×0.2µm, Supelco, USA) (Zhang et al., 2020a).
The cell pellets were also used for other metabolites analysis. For pigment measurement, the cells at 8th day were mixed with 80% (v/v) acetone and ethanol solution and incubated in the dark at 4°C for 60 min, then centrifuged at 7,500 rpm for 5 min to obtain the supernatant for chlorophyll and carotenoid content detection with ultraviolet spectrophotometer (Thermo Scientific, USA). 0.5 M NaOH was used to extract the protein by boiling for 10 min, then the supernatant after centrifugation was measured using the Bradford assay (Chen et al., 2012). For carbohydrate analysis, the cell pellets were re-suspended in 4 mL of ddH2O and transferred to a tube with 1 mL of 5% (w/v) phenol solution and 5 mL of HCl. The tube was incubated at 25°C for 10 min, then at 30°C for 20 min, and OD483 was then measured (Liang et al., 2020). For fluorescence parameters measurement, 3 mL of algal suspension were adapted for 15 min in dark place then measured by Palm Water Chlorophyll Fluorometer (Aquapen-C AP-C 100, Photonic System Instrument, Czech Republic) (Zhang et al., 2020b).
To investigate the effect of the antioxidase system in cells, the harvested cells were broken using an Ultrasonic Cell Disruption System (Nanjing XinChen JY96-II, Nainjing, China) at 4°C for 15 min (3 s on and 3 s off). Then the supernatant obtained by centrifugation (12,000 rpm at 4°C for 10 min) was assayed according to Solarbio BC0170, BC0200 and BC0020 for SOD, CAT and MDA, respectively. (Zhang et al., 2020b).
The supernatant was detected by inorganic carbon content analyzer (MULTI N/C 2100 N5 221/I). For nitrogen concentration determination, the obtained supernatant was mixed with 20 μM HCl and 0.08% sulfamic acid according to Liu’s report (Liu et al., 2013).
All the experiments in this study were conducted in triplicate. Data is represented as the mean value with standard deviation (error bars). SPSS Statistics software program was used for one-way ANOVA at the significance level of 0.05 to calculate the salient difference between treatments.
Growth of the strain HTBS with high tolerance to low temperature and high bicarbonate content was evaluated under the combination of these above conditions. As shown in Figure 1A, the growth was inhibited slightly when cells were cultured at 16°C. However, cell density increased significantly with the addition of bicarbonate (0-0.15), then decreased when the bicarbonate content exceeded 0.15 M. The OD680 reached to 1.18 under the condition of 0.15 M bicarbonate on day 7 when cultured at 16°C, which was 60.9% and 69.6% higher than cells under 25°C and 16°C without bicarbonate treatment, respectively. These results suggest that temperature and bicarbonate may affect the growth rate in microalgae cultivation. Previous study indicated the underlying mechanism that low temperature can inhibit the metabolic activity and result in the rigidification of the bilayer lipid membrane, which consequently affects the nutrient permeability and utilization (Chua et al., 2020). Furthermore, low temperature decreases the intracellular enzyme activity, which results in low cell density (Yang et al., 2019). Meanwhile, the addition of bicarbonate brings a high concentration of Na+, 0.2 M of which can inhibit many algal strains (Srinivasan et al., 2015). Therefore, it seems as if the growth might be inhibited significantly by the combination of high HCO3− and low temperature. Interestingly, the strain HTBS exhibited good bicarbonate-tolerance ability under low temperature condition in this study. The results suggested that the addition of bicarbonate was beneficial for algal strains to overcome the bottleneck caused by low temperature.
Figure 1 Effects of different combinations of bicarbonate and temperature on growth (A) and major metabolites (B, lipid; C, carbohydrate; D, protein) accumulation in HTBS.
Bicarbonate and temperature conditions can also affect the biosynthesis of cellular components. As shown in Figure 1B, the lipid content of the group with 16°C and 0 M bicarbonate was 1.7% higher than treatment with 25°C and 0 M bicarbonate (control), then increased from 36.41% to 43.94% when the bicarbonate supplement increased from 0 M to 0.15 M under 16°C. Carbohydrate as the other carbon storage material of HTBS increased from 24.67% to 29.18% (Figure 1C), which was consistent with previous results that carbon sources are positively related to carbohydrate content (Xu et al., 2017). Similar results were also obtained for protein content analysis (19.66% to 26.03%) (Figure 1D). With the addition of bicarbonate, the increased C/N ratio results in more lipid and carbohydrate biosynthesis for carbon storage with the excess energy (Peng et al., 2019; Singh et al., 2022; Xie et al., 2022). Although the lipid accumulation and protein biosynthesis present competitive relationships with each other, the increased profile of both lipid and protein content were observed in this study, possibly owing to the modified nitrogen content (750 mg/L), which was 10-fold higher than f/2 medium and promotes more intracellular conversion to protein (Vishwakarma et al., 2019). Hence, we speculate that the combination of stress could be used as an effective method for regulating the biosynthesis of main intracellular metabolites in HTBS.
The effects of different bicarbonate contents on the profile of fatty acids were investigated at 25°C and 16°C at 8 days after inoculation (Table 1). The results showed that C16:0 was the main saturated fatty acids in various conditions. C16:0 decreased slightly from 31.5% to 28.5% when cells were transferred from 25°C to 16°C without the addition of bicarbonate, whereas the unsaturated fatty acids, C18:1n9c significantly increased from 5.9% to 9.1% with an increment of 54.2%. A similar trend was observed at 25°C with 0.15 M bicarbonate addition. In contrast, the highly valued components C20:4 (AA) and C22:6 (DHA) were not found neither in both treatments at 25°C nor in treatment at low temperature without bicarbonate. Previous studies have emphasized the important effect of bicarbonate and low temperature on microalgae metabolites production (Chua et al., 2020). Lower temperature affects lipid composition, which is important to maintain membrane fluidity, and brings in an increase in the content of unsaturated fatty acids (Gao et al., 2018). The strain HTBS appears to be incapable of biosynthesizing AA and DHA under either low temperature or high bicarbonate conditions. Interestingly, the combination resulted in AA and DHA accumulation. Moreover, the profiles of the two fatty acids varied in different bicarbonate content treatments at 16°C. AA concentration increased from 0.38% to 3.52% with the content of bicarbonate increased from 0.075 M to 0.3 M, then decreased to 2.0% when bicarbonate reached to 0.6 M. Similarly, the relative percentages of DHA were 1.8%, 2.1%, 4.7% and 4.6% for 0.075 M, 0.15 M, 0.3 M and 0.6 M bicarbonate under 16°C, respectively. The highest AA and DHA concentrations in total fatty acids were found in 0.3 M bicarbonate condition, which accounted for 8.2% of the total fatty acids. In contrast, the PUFA (EPA, C20:5) of Nannochloropsis oculata was observed in normal culture condition and increased obviously in the group at 15°C (Willette et al., 2018; Chua et al., 2020). Another D. salina strain was reported to produce AA and DHA at 25°C, and the content of AA and DHA increased slightly with carbon stress (Almutairi, 2020). By contrast, AA and DHA were undetectable in D. salina Y6 under various conditions, such as high-light, nitrogen-depleted and high-salt conditions. In general, microalgae cells accumulate more PUFAs to increase membrane fluidity to counteract the negative effect caused by lower temperatures (Lu et al., 2017). Nevertheless, with the good ability of tolerance to cold treatment, the membrane fluidity of HTBS is activated as the cells grow well at 16°C and 4°C, thus the PUFAs such as AA and DHA are unnecessary to be synthesized (Hou et al., 2016; Wu et al., 2020). Then, more carbon resource is provided with the supplement of bicarbonate, which might be used for secondary metabolites conversion (Chua et al., 2020; Wu et al., 2021). In addition, bicarbonate may induce ROS production, which could be counteracted by increasing PUFA synthesis (Xie et al., 2021; Ju et al., 2022; Vinuganesh et al., 2022). As a result, AA and DHA are accumulated with the combinatorial stresses.
To illustrate the potential reason for the effects of various bicarbonate contents with low temperature on growth and metabolite biosynthesis, the photosynthetic performance and carbon\nitrogen utilization rate were investigated (Figure 2). The maximum photochemical efficiency (Fv/Fm) was reduced by 19.35% compared to the control culture at 25 °C due to the low temperature and lack of bicarbonate supply. The addition of bicarbonate alleviated low temperature-induced photosynthesis impairment, as Fv/Fm increased by 54.67%, 34.22%, 14.67% and 12% at 0.075 M, 0.15 M, 0.3 M and 0.6 M bicarbonate, respectively, which was consistent with the previous study (Sun et al., 2020). Similarly, microalgae cells with an increased bicarbonate conditions showed a gradually significant rise in Fv/Fm, which suggested an increase in photosynthetic carbon fixation and metabolic activity (Singh et al., 2022). With the sustained addition of bicarbonate, the salinity increases to suppress of photosynthetic activity (Salbitani et al., 2020). Luckily, the extracellular carbon anhydrases in HTBS are sufficient to provide enough Ci for the growth under high bicarbonate levels (Hou et al., 2016). Then the carboxylation reaction catalyzed by Rubisco in the dark reaction could be enhanced, which resulted in ATP and NADPH consumption. Hence, Fv/Fm was increased to promote electron transfer to generate more energy for carbon fixation, which resulted in a higher carbon removal rate (Figure 2C) and higher biomass (Figure 1) (Xie et al., 2022). The increased non-photochemical quenching (NPQ) means that the cells are suffering from environmental stress (Xue et al., 2022). Therefore, the reductions of NPQ at 0.075 M and 0.15M bicarbonate groups suggest that a certain bicarbonate content can help to relieve the stress caused by low temperature, which is consistent with the report that a high concentration of CO2 declines NPQ to benefit carbon and nitrogen sequestration, resulting in more metabolite synthesis (Singh et al., 2022). As a consequence, the carbon and nitrogen removal rates of the combined groups were significantly increased compared to low temperature treatment without bicarbonate supply. The visible nutrient utilization might be attributed to the increased Fv/Fm and decreased NPQ. Overall, these results indicated that HTBS with the combination of optimum bicarbonate concentration and low temperature showed good photosynthetic performance, which resulted in a significant increase in cell proliferation.
Figure 2 Effects of various combinations on HTBS photosynthetic performance [(A), Fv/Fm; (B), NPQ] and nutrient removal rate [(C), carbon; (D), nitrogen]. Data were mean ± SD of three replicates.
As shown in Figure S1, low temperature without bicarbonate affected chlorophyll (Chl) biosynthesis slightly. However, the additional bicarbonate showed a dose-dependent effect. Chl was gradually increased by 9.67%, 27.43% and 43.58% with the added bicarbonate content increasing from 0.075 M to 0.3 M, while the extremely high content of bicarbonate (0.6 M) inhibited Chl biosynthesis. It means that a certain bicarbonate content accelerates Chl synthesis to maintain photosynthetic activity and alleviate the stress caused by low temperature (Xie et al., 2022). Meanwhile, carotenoids, another important pigment for light harvest and energy transfer, were affected by the combination of low temperature and bicarbonate. The carotenoid contents of the combined stress groups increased compared with the low temperature without bicarbonate, which indicated that more energy is required for carbon utilization under high carbon resources in HTBS (Ding et al., 2017).
To respond to the stress-induced ROS, the antioxidant system is activated to protect cells from oxidative damage (Rezayian et al., 2019). Superoxide dismutase (SOD) and catalase (CAT) were up-regulated to scavenge superoxide radicals and hydrogen peroxide at low temperature. In this study, SOD and CAT decreased under low temperature without bicarbonate, probably due to the good low-temperature tolerance of HTBS (Hou et al., 2016). SOD activity increased and reached its maximum at the 0.15 M bicarbonate group, which was 89% higher than that in the group without carbon supply, followed by a decrease in activity (Figure 3A). The increased SOD activity resulted in H2O2 accumulation, which could be converted to H2O by CAT. CAT activity was closely associated with SOD activity (Figure 3B). On the other hand, MDA, the biomarker for evaluating oxidative damage, was also assessed. With the addition of bicarbonate at 16 °C, MDA decreased slightly and then significantly increased (Figure 3C). These results suggested that HTBS adapted to the environment by regulating specific enzymes in response to the combined stresses (Srinivasan et al., 2018).
Figure 3 Changes of SOD (A), CAT (B) activities and MDA (C) content in HTBS cells grown under different stresses.
In this study, the effects of a combination of low temperature and bicarbonate on physiological and biochemical changes in HTBS were investigated. Compared to single stress (low temperature or bicarbonate), the content of pigment and, Fv/Fm was increased while NPQ was decreased under the combination of low temperature and bicarbonate stress. All of the above changes benefited carbon and nitrogen absorption and utilization, which resulted in an increase in cell growth, lipid, protein and carbohydrate with combined conditions. Moreover, AA and DHA reached 3.52% and 4.73%, respectively, whereas they were not detected with single treatment. The present study demonstrates that supplementing bicarbonate under low temperature could effectively enhance the biomass production and accumulation of AA and DHA in D. salina HTBS, which benefits the development of the microalgae industry in value-added products.
The original contributions presented in the study are included in the article/Supplementary Material. Further inquiries can be directed to the corresponding authors.
WW, ZL, CF and HY conceived the ideas and the experimental design. GZ, LZ, GT and MY collected the data. HT, HN, YY and LC analyzed the data. HY, GZ and GT drafted the manuscript. MS, WW, ZL and CF reviewed and edited the manuscript. All authors contributed to the article and approved the submitted version.
This work was supported by the National Key R&D Program of China (2019YFA0904600; 2018YFE0107200), the Science and Technology Partnership Program, Ministry of Science and Technology of China (KY202001017), the Tianjin Synthetic Biotechnology Innovation Capacity Improvement Project (TSBICIP-IJCP-001; TSBICIP-CXRC-027), and the TIB-VIB Joint Center of Synthetic Biology (TSBICIP-IJCP-002).
The authors declare that the research was conducted in the absence of any commercial or financial relationships that could be construed as a potential conflict of interest.
All claims expressed in this article are solely those of the authors and do not necessarily represent those of their affiliated organizations, or those of the publisher, the editors and the reviewers. Any product that may be evaluated in this article, or claim that may be made by its manufacturer, is not guaranteed or endorsed by the publisher.
The Supplementary Material for this article can be found online at: https://www.frontiersin.org/articles/10.3389/fmars.2022.971441/full#supplementary-material
Almutairi A. W. (2020). Effects of nitrogen and phosphorus limitations on fatty acid methyl esters and fuel properties of Dunaliella salina. Environ. Sci. pollut. Res. 27 (26), 32296–32303. doi: 10.1007/s11356-020-08531-8
Aussant J., Guihéneuf F., Stengel D. (2018). Impact of temperature on fatty acid composition and nutritional value in eight species of microalgae. Appl. Microbiol. Biotechnol. 102 (12), 5279–5297. doi: 10.1007/s00253-018-9001-x
Chauhan D., Sahoo L., Mohanty K. (2022). Maximize microalgal carbon dioxide utilization and lipid productivity by using toxic flue gas compounds as nutrient source. Bioresour. Technol. 348, 126784. doi: 10.1016/j.biortech.2022.126784
Chen H., Jiang J. (2009). Osmotic responses of Dunaliella to the changes of salinity. J. Cell Physiol. 219 (2), 251–258. doi: 10.1002/jcp.21715
Chen F., Liu Z., Li D., Liu C., Zheng P., Chen S. (2012). Using ammonia for algae harvesting and as nutrient in subsequent cultures. Bioresour. Technol. 121, 298–303. doi: 10.1016/j.biortech.2012.06.076
Chi Z., Elloy F., Xie Y., Hu Y., Chen S. (2014). Selection of microalgae and cyanobacteria strains for bicarbonate-based integrated carbon capture and algae production system. Appl. Biochem. Biotechnol. 172, 447–457. doi: 10.1007/s12010-013-0515-5
Chua E. T., Dal'Molin C., Thomas-Hall S., Netzel M. E., Netzel G., Schenk P. M. (2020). Cold and dark treatments induce omega-3 fatty acid and carotenoid production in Nannochloropsis oceanica. Algal Res. 51, 102059. doi: 10.1016/j.algal.2020.102059
Ding Y., Li X., Li Z., Wang Z., Li Z., Geng Y., et al. (2017). Ammonium bicarbonate supplementation as carbon source in alkaliphilic Spirulina mass culture. Aquac. Res. 48 (9), 4886–4896. doi: 10.1111/are.13308
Gao G., Clare .A S., Chatzidimitriou E., Rose C., Caldwell G. S. (2018). Effects of ocean warming and acidification, combined with nutrient enrichment, on chemical composition and functional properties of Ulva rigida. Food Chem. 258, 71–78. doi: 10.1016/j.foodchem.2018.03.040
Guihéneuf F., Stengel D. B. (2013). LC-PUFA-enriched oil production by microalgae: accumulation of lipid and triacylglycerols containing n-3 LC-PUFA is triggered by nitrogen limitation and inorganic carbon availability in the marine haptophyte Pavlova lutheri. Mar. Drugs 11, 4246–4266. doi: 10.3390/md11114246
Hou Y., Liu Z., Zhao Y., Chen S., Zheng Y., Chen F. (2016). CAH1 and CAH2 as key enzymes required for high bicarbonate tolerance of a novel microalga Dunaliella salina HTBS. Enzyme. Microb. Tech. 87-88, 17–23. doi: 10.1016/j.enzmictec.2016.02.010
Jakhwal P., Biswas J. K., Tiwari A., Kwon E. E., Bhatnagar A. (2022). Genetic and non-genetic tailoring of microalgae for the enhanced production of eicosapentaenoic acid (EPA) and docosahexaenoic acid (DHA) - a review. Bioresour. Technol. 344 (Pt B), 126250. doi: 10.1016/j.biortech.2021.126250
Ju Z., Feng T., Feng J., Lv J., Xie S., Liu Q. (2022). Physiological response of an oil-producing microalgal strain to salinity and light stress. Foods. 11 (2), 11020215. doi: 10.3390/foods11020215
Khozin-Goldberg I., Bigogno C., Shrestha P., Cohen Z. (2002). Nitrogen starvation induces the accumulation of arachidonic acid in the freshwater green alga parietochloris incisa (trebuxiophyceae). J. Phycol. 38, 991–994. doi: 10.1046/j.1529-8817.2002.01160.x
Liang C., Yang X., Wang L., Fan X., Zhang X., Xu D., et al. (2020). Different physiological and molecular responses of the green algae Chlorella variabilis to long-term and short-term elevated CO2.J. Appl. Phycol. 32 (2), 951–966. doi: 10.1007/s10811-019-01943-1
Liu Z., Liu C., Hou Y., Chen S., Xiao D., Zhang J., et al. (2013). Isolation and characterization of a marine microalga for biofuel production with astaxanthin as a co-product. Energies. 6 (6), 2759–2772. doi: 10.3390/en6062759
Lu Q., Li J., Wang J., Li K., Li J. J., Han P., et al. (2017). Exploration of a mechanism for the production of highly unsaturated fatty acids in scenedesmus sp. at low temperature grown on oil crop residue based medium. Bioresour. Technol. 244 (Pt 1), 542–551. doi: 10.1016/j.biortech.2017.08.005
Lu Q., Li H., Xiao Y., Liu H. (2021). A state-of-the-art review on the synthetic mechanisms, production technologies, and practical application of polyunsaturated fatty acids from microalgae. Algal Res. 55, 102281. doi: 10.1016/j.algal.2021.102281
Morales M., Aflalo C., Bernard O. (2021). Microalgal lipids: A review of lipids potential and quantification for 95 phytoplankton species. Biomass. Bioenerg. 150, 106108. doi: 10.1016/j.biombioe.2021.106108
Nunez M., Quigg A. (2016). "Changes in growth and composition of the marine microalgae Phaeodactylum tricornutum and Nannochloropsis salina in response to changing sodium bicarbonate concentrations." appl. Phycol. 28 (4), 2123–2138. doi: 10.1007/s10811-015-0746-7
Peng Y. Y., Gao F., Hang W., Yang H., Jin W., Li C. (2019). Effects of organic matters in domestic wastewater on lipid/carbohydrate production and nutrient removal of Chlorella vulgaris cultivated under mixotrophic growth conditions. J. Chem. Technol. Biot. 94 (11), 3578–3584. doi: 10.1002/jctb.6161
Ratomski P., Hawrot-Paw M., Koniuszy A. (2021). Utilisation of CO2 from sodium bicarbonate to produce Chlorella vulgaris biomass in tubular photobioreactors for biofuel purposes. Sustainability 13 (16), 9118. doi: 10.3390/su13169118
Rezayian M., Niknam V., Ebrahimzadeh H. (2019). Oxidative damage and antioxidative system in algae. Toxicol. Rep. 6, 1309–1313. doi: 10.1016/j.toxrep.2019.10.001
Salbitani G., Bolinesi F., Affuso M., Carraturo F., Mangoniand O., O.Carfagna S. (2020). Rapid and positive effect of bicarbonate addition on growth and photosynthetic efficiency of the green microalgae Chlorella sorokiniana (Chlorophyta, trebouxiophyceae). Appl. Sci. 10 (13), 4515. doi: 10.3390/app10134515
Singh R. P., Yadav P., Kumar A., Hashem A., Al-Arjani A. F., Allah E. F. A, et al. (2022). Physiological and biochemical responses of bicarbonate supplementation on biomass and lipid content of green algae scenedesmus sp. BHU1 isolated from wastewater for renewable biofuel feedstock. Front. Microbiol. 13, 839800. doi: 10.3389/fmicb.2022.839800
Srinivasan R., Kumar V. A., Kumar D., Ramesh N., Babu S., Gothandam K. M., et al. (2015). Effect of dissolved inorganic carbon on beta-carotene and fatty acid production in dunaliella sp. appl. biochem. Biotechnol. 175 (6), 2895–2906. doi: 10.1007/s12010-014-1461-6
Srinivasan R., Mageswari A., Subramanian P., Suganthi C., Chaitanyakumar A., Aswini V., et al. (2018). Bicarbonate supplementation enhances growth and biochemical composition of Dunaliella salina V-101 by reducing oxidative stress induced during macronutrient deficit conditions. Sci. Rep. 8 (1), 6972. doi: 10.1038/s41598-018-25417-5
Sun H., Ren Y., Mao X., Li X., Zhang H., Lao Y., et al. (2020). Harnessing C/N balance of Chromochloris zofingiensis to overcome the potential conflict in microalgal production. Commun. Biol. 3 (1), 186. doi: 10.1038/s42003-020-0900-x
Vishwakarma J., Parmar V., Vavilala S. L. (2019). Nitrate stress-induced bioactive sulfated polysaccharides from Chlamydomonas reinhardtii. Biomed. Res. J. 6 (1), 7–16. doi: 10.4103/BMRJ.BMRJ_8_19
Vinuganesh A., Kumar A., Prakash S., Alotaibi M., Saleh A., Mohammed A., et al. (2022). Influence of seawater acidification on biochemical composition and oxidative status of green algae Ulva compressa. Sci. Total. Environ. 806 (Pt 1), 150445. doi: 10.1016/j.scitotenv.2021.150445
Willette S., Gill S. S., Dungan B., Schaubb T. M., Jarvisb J. M., Hilairea R. S., et al. (2018). Alterations in lipidome and metabolome profiles of Nannochloropsis salina in response to reduced culture temperature during sinusoidal temperature and light. Algal Res. 32, 79–92. doi: 10.1016/j.algal.2018.03.001
Wu M., Zhu R., Lu J., Lei A., Zhu H., Hu Z., et al. (2020). Effects of different abiotic stresses on carotenoid and fatty acid metabolism in the green microalga Dunaliella salina Y6. Ann. Microbiol. 70, 48. doi: 10.1186/s13213-020-01588-3
Wu M., Gao G., Jian W., Xu J. (2021). High CO2 increases lipid and polyunsaturated fatty acid productivity of the marine diatom Skeletonema costatum in a two-stage model. J. Appl. Phycol. 34 (1), 43–50. doi: 10.1007/s10811-021-02619-5
Xie G., Ding K., Liu W., Zheng Z., Liu Y., Wang Y. (2021). Characteristics of lipid biosynthesis in Chlorella pyrenoidosa as subjected to nutrient deficiency stress. Phycologia. 60 (4), 384–393. doi: 10.1080/00318884.2021.1948751
Xie S., Lin F., Zhao X., Gao G. (2022). Enhanced lipid productivity coupled with carbon and nitrogen removal of the diatom Skeletonema costatum cultured in the high CO2 level. Algal. Res. 61, 102589. doi: 10.1016/j.algal.2021.102589
Xue S., Zang Y., Chen J., Shang S., Tang X. (2022). Effects of enhanced UV-b radiation on photosynthetic performance and non-photochemical quenching process of intertidal red macroalgae Neoporphyra haitanensis. Environ. Exp. Bot. 199, 104888. doi: 10.1016/j.envexpbot.2022.104888
Xu Z., Gao G., Xu J., Wu H. (2017). Physiological response of a golden tide alga (Sargassum muticum) to the interaction of ocean acidification and phosphorus enrichment. Biogeosciences 14 (3), 671–681. doi: 10.5194/bg-14-671-2017
Yang J., Yu D., Ma Y., Yin Y., Shen S. (2019). Antioxidative defense response of Ulva prolifera under high or low-temperature stimulus. Algal Res. 44, 101703. doi: 10.1016/j.algal.2019.101703
Zhang S., Hou Y., Liu Z., Ji X., Wu D., Wang W., et al. (2020a). Electro-fenton based technique to enhance cell harvest and lipid etraction from microalgae. Energies 13 (15), 3813. doi: 10.3390/en13153813
Keywords: Dunaliella salina HTBS, bicarbonate, low temperature, arachidonic acid, docosahexaenoic acid
Citation: Guo Z, Hou Y, Liu Z, Ma Y, Han T, Hao N, Yao Y, Lan C, Ge T, Safavi M, Wang W, Zhao L and Chen F (2022) Combination of bicarbonate and low temperature stress induces the biosynthesis of both arachidonic and docosahexaenoic acids in alkaliphilic microalgae Dunaliella salina HTBS. Front. Mar. Sci. 9:971441. doi: 10.3389/fmars.2022.971441
Received: 17 June 2022; Accepted: 21 September 2022;
Published: 04 October 2022.
Edited by:
Wei Liu, Qilu University of Technology (Shandong Academy of Sciences), ChinaReviewed by:
Tonmoy Ghosh, Indian Institute of Technology Indore, IndiaCopyright © 2022 Guo, Hou, Liu, Ma, Han, Hao, Yao, Lan, Ge, Safavi, Wang, Zhao and Chen. This is an open-access article distributed under the terms of the Creative Commons Attribution License (CC BY). The use, distribution or reproduction in other forums is permitted, provided the original author(s) and the copyright owner(s) are credited and that the original publication in this journal is cited, in accordance with accepted academic practice. No use, distribution or reproduction is permitted which does not comply with these terms.
*Correspondence: Weijie Wang, d2Vpamlld2FuZ0BuY3N0LmVkdS5jbg==; Lei Zhao, emhhb2xAdGliLmNhcy5jbg==; Fangjian Chen, Y2hlbl9makB0aWIuY2FzLmNu
†These authors have contributed equally to this work
Disclaimer: All claims expressed in this article are solely those of the authors and do not necessarily represent those of their affiliated organizations, or those of the publisher, the editors and the reviewers. Any product that may be evaluated in this article or claim that may be made by its manufacturer is not guaranteed or endorsed by the publisher.
Research integrity at Frontiers
Learn more about the work of our research integrity team to safeguard the quality of each article we publish.