- 1Department of Environment and Energy, Sejong University, Seoul, South Korea
- 2Korea Polar Research Institute (KOPRI), Incheon, South Korea
- 3Department of Ocean Environmental Sciences, Chungnam National University, Daejeon, South Korea
- 4Environmental Science and Engineering Research Group, Guangdong Technion - Israel Institute of Technology, Shantou, China
The warming of the Arctic Ocean impacts the dissolved organic matter (DOM) imports into the Arctic region, which affects the local bacterial communities. This review addressed the current status of DOM inputs and their potential influences on bacteria data (e.g., population, production, and metabolic activity of bacteria), as well as the projected changes of DOM inputs and bacterial communities as a result of climate warming. Microbial communities are likely affected by the warming climate and the transport of DOM to the Arctic Ocean. Imported DOM can alter Arctic bacterial abundance, cell size, metabolism, and composition. DOM fluxes from Arctic River runoff and adjacent oceans have been enhanced, with warming increasing the contribution of many emerging DOM sources, such as phytoplankton production, melted sea ice, thawed permafrost soil, thawed subsea permafrost, melted glaciers/ice sheets, atmospheric deposition, groundwater discharge, and sediment efflux. Imported DOM contains both allochthonous and autochthonous components; a large quantity of labile DOM comes from emerging sources. As a result, the Arctic sea water DOM composition is transformed to include a wider range of various organic constituents such as carbohydrates (i.e., glucose), proteinaceous compounds (i.e., amino acid and protein-like components) and those with terrigenous origins (i.e., humic-like components). Changes to DOM imports can alter Arctic bacterial abundance, cell size, metabolism, and composition. Under current global warming projections, increased inflow of DOM and more diverse DOM composition would eventually lead to enhanced CO2 emissions and frequent emergence of replacement bacterial communities in the Arctic Ocean. Understanding the changes in DOM fluxes and responses of bacteria in the Arctic broadens our current knowledge of the Arctic Ocean’s responses to global warming.
1 Introduction
The annual average Arctic temperature has risen approximately twice as fast as global temperatures in recent decades and is expected to increase by an average of 8.5 ± 2.1°C over the duration of the twenty-first century (Kaplan and New, 2006; Bintanja and Andry, 2017). Continued warming is likely to have profound effects on a variety of Arctic Ocean processes, including Arctic River runoff (Raymond et al., 2007; Behnke et al., 2021), Atlantic and Pacific inflow (Jones et al., 2008; Vetrov and Romankevich, 2019) to the Arctic Ocean, phytoplankton production (Kirchman et al., 2009; Osburn et al., 2019), sea ice melting (Maranger et al., 2015; Piontek et al., 2021), permafrost soil thawing (Serreze and Barry, 2011; Turetsky et al., 2020), subsea permafrost thawing (Chen et al., 2021), glacier/ice sheet melting (Hood et al., 2015), atmospheric deposition (Ruppel et al., 2015; Fang et al., 2016), groundwater discharge (Connolly et al., 2020), and efflux from sediment (Chen et al., 2016; Rossel et al., 2016; Rossel et al., 2020). These environmental processes contain and generate high quantities of dissolved organic matter (DOM). Climate change-related repercussions have led these processes to become DOM generators, accumulating substantial volumes of DOM in the Arctic Ocean, particularly in surface water (Tanaka et al., 2016; Stedmon et al., 2021). The role of DOM in marine ecosystems, notably as a substrate for bacterial communities, is highly dependent on its concentration and composition, both of which are closely related to its sources (Carlson, 2014). Therefore, it is critical to keep track of all the sources of DOM that contribute to the Arctic Ocean and to assess how climate warming is impacting and will continue to impact DOM input.
Numerous bacterial species thrive in the cold conditions of the Arctic Ocean, where Alphaproteobacteria, Gammaproteobacteria, and Bacteroidetes are dominant (Bano and Hollibaugh James, 2002; Alonso-Sáez et al., 2008; Comeau et al., 2011; Lee et al., 2019; Sanz-Sáez et al., 2020). Bacteria in the Arctic are evolutionarily unique compared to all other isolates, as evidenced by genetic analysis (Royo-Llonch et al., 2021). Bacterial populations in the Arctic Ocean are especially vulnerable to environmental changes caused by external factors (Fadeev et al., 2018; Carter-Gates et al., 2020; Cardozo-Mino et al., 2021; Ramachandran et al., 2021). The Arctic Ocean is known to be an oligotrophic marine environment with low levels of carbon substrates (Sala et al., 2008; Sert et al., 2022). Thus, perturbations in DOM flux caused by rising temperatures can significantly affect bacterial communities in the Arctic. DOM provides energy and carbon to bacteria, and bacteria participate in carbon cycling within aquatic environments, functioning as a sink (mineralization of DOM to CO2) or a link (generation of biomass that may be transported through the microbial food web) (Cotner et al., 2002). Bacteria play a key role in the marine environment because they are critical components of the microbial loop; bacteria use available DOM in the surrounding water to grow, and are subsequently ingested by grazers, which are eventually consumed by bigger organisms in the Arctic Ocean (Kirchman et al., 2009). Thus, changes in bacterial communities may have a domino effect on the entire Arctic ecosystem. The response of bacteria to DOM changes in the Arctic can be considered as the first signal of changes within the entire Arctic ecosystems caused by warming climate effects. Therefore, it is an important topic that deserves extensive research.
In this study, we collected present data regarding DOM inputs and their likely effects on bacteria, as well as projected changes as a result of the impacts of climate warming. First, we identified the possible sources of DOM discharged into the Arctic Ocean, as well as the origins and composition of DOM. In addition, updated data on bacterial abundance, metabolic activity, and composition in Arctic sea waters were compiled to gain a better understanding of the particular features that may operate as an adaptive mechanism for Arctic bacterial populations. We also summarize the consequences of DOM changes on domestic bacterial populations in the Arctic. Finally, we discuss the potential impact of warming on DOM quality and Arctic bacteria. The research region for this study covers the entire Arctic Ocean (Figure 1), with a particular emphasis on surface seawater, which receives a larger volume of DOM and varies to a greater extent as a result of environmental changes compared to Arctic deep water (Bano and Hollibaugh James, 2002; Benner et al., 2005; Tanaka et al., 2016). This study is expected to expand and deepen our knowledge of how the Arctic Ocean changes in response to global warming, in terms of DOM influx and bacterial populations.
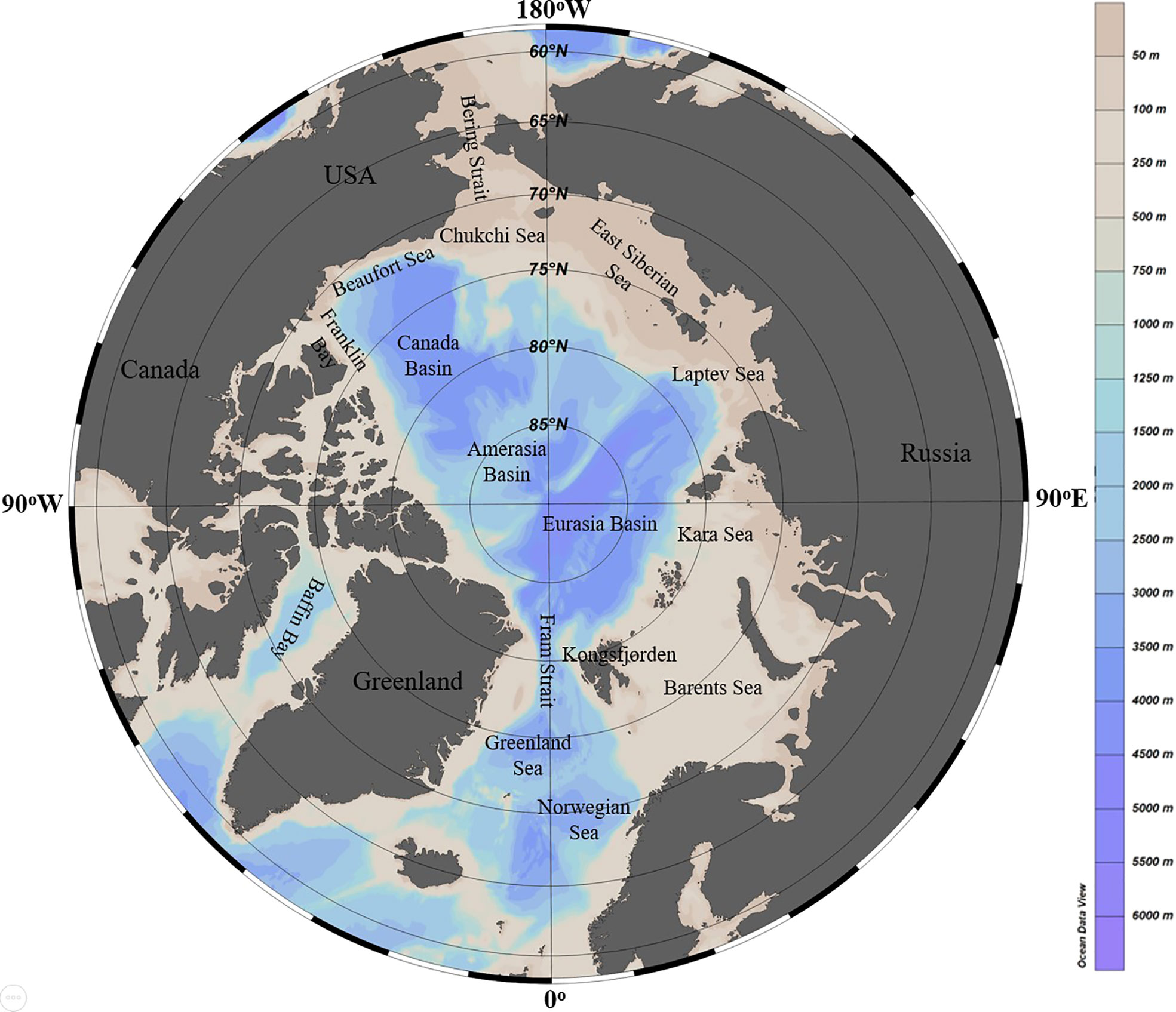
Figure 1 Arctic Ocean and its constituent seas. The map was created by using the Ocean Data View software (Schlitzer, Reiner, Ocean Data View, odv.awi.de, 2021).
2 Sources of DOM to the Arctic Ocean
DOM is transported to the Arctic Ocean via multiple sources. Arctic rivers, as well as the inflow from the Atlantic and Pacific, are the main transporters of DOM to the Arctic Ocean. The level of transported DOM can be intensified by heavier precipitation that is caused by warming climate (Raymond et al., 2007; Jones et al., 2008; Vetrov and Romankevich, 2019; Behnke et al., 2021). In addition, many other DOM sources have recently been identified as a result of the warming in the Arctic Ocean, including plankton production, melted sea ice, thawed permafrost soil, thawed subsea permafrost, melted ice sheets/glaciers, atmospheric deposition, groundwater discharge, and enhanced efflux from sediment. Ten sources of present DOM influxes to the Arctic Ocean are illustrated in Figure 2 and the corresponding DOM information (origin, concentration, and composition) are listed in Table 1. This section provides in-depth description concerning how these sources are influenced by warming climate.
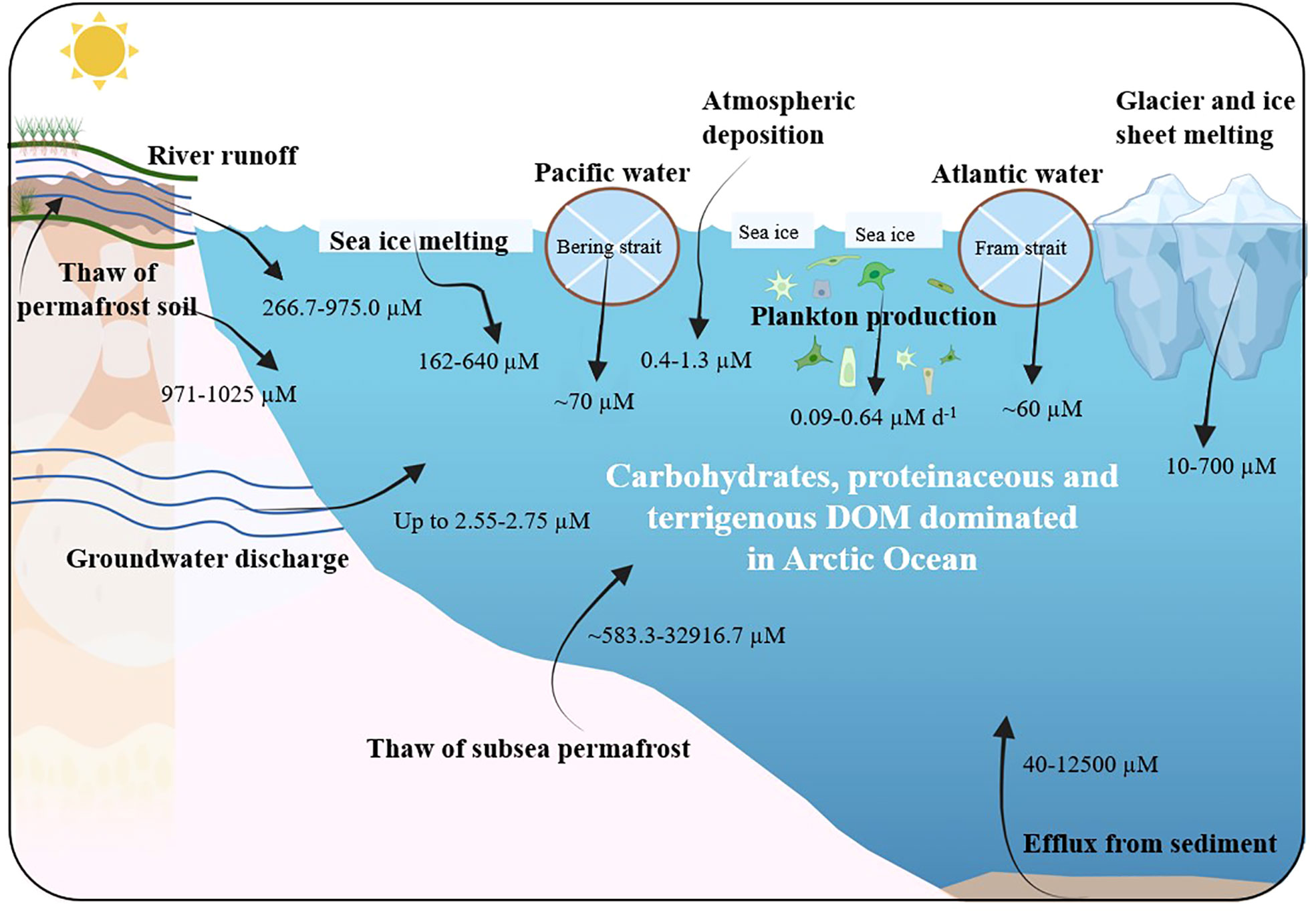
Figure 2 Sources of DOM imports into the Arctic Ocean. Concentration of DOM was derived the previous studies: river discharge (Behnke et al., 2021), Atlantic and Pacific water intrusion (Borsheim and Drinkwater, 2014; Jørgensen et al., 2014; Anderson and Amon, 2015), plankton production (Poulton et al., 2016), melted sea ice (Retelletti Brogi et al., 2018), thawed permafrost soil (Ward and Cory, 2015), melted ice sheets and glaciers (Bhatia et al., 2010; Bhatia et al., 2013; Kellerman et al., 2021), thawed subsea permafrost (Overduin et al., 2015; Chen et al., 2021), atmospheric deposition (Osterholz et al., 2014; Nakane et al., 2017; Fang et al., 2021), groundwater discharge (Connolly et al., 2020), efflux from Arctic sediment (Chen et al., 2016; Rossel et al., 2016; Rossel et al., 2020). Carbohydrates (i.e., glucose), proteinaceous (i.e., amino acid and protein-like components), and terrigenous characteristics (i.e., humic-like components) of DOM are predominant in surface water of Arctic Ocean as a result of increasing DOM input due to warming impacts.
2.1 Arctic River discharges
The increase in freshwater flow from rivers into the Arctic Ocean as a consequence of global warming was previously estimated to be 7% with a yearly rate of increase of ~2.0 km3 year-1 during 1936–1999 (Peterson Bruce et al., 2002). Recent measurements at regional and pan-Arctic scales also revealed a significant increase at a rate of ~2.7 km3 year-1 in the total annual river flow to the Arctic Ocean during 1936–2015 (Shiklomanov et al., 2021). The riverine DOM input to the Arctic Ocean has been estimated as approximately 25–36 Tg C yr-1 (Raymond et al., 2007) based on the discharge from the five largest rivers (i.e., the Mackenzie, Yukon, Ob’, Lena, and Yenisey Rivers) in 2004–2005. In the same vein, approximately 5–33% of the total DOC in Arctic surface waters is considered to be terrigenous in origin and is delivered via Arctic rivers (Opsahl et al., 1999). The DOM flux from Arctic rivers to the Arctic Ocean is expected to rise with increased water flow due to warming. A 6-year study conducted from 2012 to 2017 reported DOC concentrations in six major rivers varying from 3.2 mg L-1 to 11.7 mg L-1 (Behnke et al., 2021). The discharge of Arctic rivers is highly seasonal, with maximum fluxes in the spring and the lowest fluxes in the winter (Mann et al., 2012; Walker et al., 2013). River runoff during spring freshet is primarily affected by modern plant cover, topsoil, and surface litter (Benner et al., 2004; Walker et al., 2013); thus, the bulk of DOM exported during spring is relatively young and includes newly fixed carbon (Benner et al., 2004; Raymond et al., 2007; Holmes et al., 2008; Wickland et al., 2012), which is considered to be relatively labile DOM. During summer and winter, water from deeper soil layers percolates to the rivers; incorporating old, relatively non-labile DOM with relatively humified structures and low content of lignin phenols (Stedmon et al., 2011; Mann et al., 2012). For organic compounds derived from Arctic rivers, terrestrial humic-like fluorophores are the most prevalent fluorescent DOM (FDOM) component (Walker et al., 2013). Enhanced river flow can lead to increases of several DOM optical indices such as the absorption coefficient at 350 nm (a350), which serves as a measure of lignin content and tDOM intake, and the average specific UV absorbances at 254 nm (SUVA254), which is a measure of aromaticity from allochthonous inputs (Walker et al., 2013). The biological index (BIX) values can also increase [e.g., from 0.45 to 0.68 (Birdwell and Engel, 2010)], indicating that the DOM in the Arctic rivers contains a relatively low abundance of autochthonous DOM. The core Arctic riverine fingerprint (CARF), which was proposed based on the molecular analysis of DOM from the six major rivers discharging to the Arctic Ocean over several years (2012–2017), was found to consist of 1,328 formulas (Behnke et al., 2021). The CARF contains a broad range of different substances with potential autochthonous origins, such as aliphatics and sugar-like formulas, as well as those with potential terrestrial sources, such as condensed aromatics and polyphenolics (Behnke et al., 2021). With continual global warming, the Arctic Ocean is thus anticipated to receive an increase in the supply of diverse organic compounds, particularly terrestrial-derived DOM compounds (humic-like and other aromatic components) along with a quantitative increase of DOM imports from the Arctic rivers.
2.2 Inflow waters from adjacent oceans
DOM is transported to the Arctic Ocean by inflows from the Pacific and Atlantic Oceans, and the inflow volume is on the rise owing to the warming effect. Long-term in situ Bering Strait mooring data (1990–2015) recorded nearly a doubling of the volume transport of Pacific waters into the Arctic from 2001 (0.7 Sv) to 2011 (1.1 Sv) (1 Sv = 106 m3 s-1) (Woodgate, 2018). The Atlantic water volume transport to the Arctic Ocean has also been expanded (Årthun et al., 2012; Wang et al., 2020), which provides not only DOM but also heat and salinity, causing the so-called “Atlantification” of the Arctic Ocean (Quadfasel et al., 1991; Lind et al., 2018). The seasonally accumulated inventory of DOC in the upper Northeast Pacific Ocean varied by a factor of ten across years (2009 to 2015), changing from 0.078 to 0.75 mol C m-2 (Bif and Hansell, 2019). Thus, the DOC concentrations in the North Pacific and North Atlantic waters to the Arctic Ocean tend to increase, thus increasing the likelihood of enhanced transport of DOM to the Arctic (Bif and Hansell, 2019). The fluxes of DOM into the Arctic Ocean were estimated to be 23 ± 2 Tg C yr-1 and 155 ± 13 Tg C yr-1 by inflow from the Pacific Ocean and the North Atlantic, respectively (Monteith et al., 2007; Bif and Hansell, 2019; Vetrov and Romankevich, 2019). Meanwhile, the concentration of DOM from the Pacific was estimated to be ~70 µM (Anderson and Amon, 2015), which is dominated by allochthonous DOM with a strong aliphatic character with very low light absorptivity (Pugach et al., 2018) and FDOM (~0.017 RU) (Stedmon et al., 2021; Cai et al., 2022). The DOC concentration in Atlantic seawater input was reported to be approximately 60 µM (Borsheim and Drinkwater, 2014; Jørgensen et al., 2014), which is characterized by a relatively low abundance of chromophoric DOM (cDOM) (Paulsen et al., 2018) and FDOM (~0.01 RU) (Stedmon et al., 2021), suggesting a lower contribution from terrestrial DOM compared to the Pacific seawater. The DOM from the surface waters of both the Atlantic and Pacific was found to be relatively bio-refractory, with only 10% of the bio-labile fraction in the total pool (Amon and Benner, 2003). Thus, it is worth noting that the influx of water from the Pacific and Atlantic is a considerable factor influencing the distribution of relatively bio-refractory DOM in the Arctic Ocean.
2.3 Plankton production
Plankton is a significant source of DOM production in the Arctic Ocean (Wheeler et al., 1997; Osburn et al., 2019). The annual DOM input from fresh phytoplankton is estimated to be approximately 66 Tg C y–1 over the entire Arctic Ocean (Stein et al., 2004). Phytoplankton photosynthesizes inorganic carbon (i.e., CO2) and transforms it into organic carbon, which releases 2–50% of the total fixed carbon as DOM to seawater via passive diffusion and active exudation (Thornton, 2014). The physical breaking of phytoplanktonic cells by predators and virus lysis also contributes to DOM production (Urban-Rich et al., 2006; Saba et al., 2011). Phytoplankton development is on the rise as a result of the accelerating loss of sea ice cover in the Arctic Ocean, which is experiencing a warming climate (Dadaglio et al., 2018; Lewis et al., 2020). Spring was formerly thought to be the only season for phytoplankton blooms; however, autumn blooms have also been reported in Arctic seas (Uchimiya et al., 2016; Chen et al., 2017). Integrated primary production in the Arctic Ocean increased by 56.5% from 1998 to 2018, with an average annual increase of 6.79 Tg C (Lewis et al., 2020). In particular, sea ice and under-ice algae play substantial roles in primary production in the Arctic Ocean, accounting for 3–60% of the total production in the Arctic Ocean (Legendre et al., 1992; Gosselin et al., 1997; Ambrose et al., 2005; Mundy et al., 2009). It was previously reported that the amount of DOC generated by phytoplankton was 0.09–0.64 mmol C m-3 day-1 in Nordic waters of the Arctic Ocean during the summer of 2012 (Poulton et al., 2016). Carbohydrates, nitrogenous substances, lipids, and organic acids constitute phytoplankton-derived DOM, which is highly labile (Lancelot, 1984; Emerson and Hedges, 2008). Protein-like fluorescent DOM (FDOM) has been suggested to be closely linked to the fall phytoplankton bloom in the Chukchi Sea and East Siberian Sea (Chen et al., 2017). Tyrosine- and tryptophan-like fluorophores are recognized as FDOM components in the polar ocean, which represent proteins (Chen et al., 2018; Retelletti Brogi et al., 2019; Jung et al., 2021). Additionally, phytoplankton blooms are significant producers of particulate and extracellular carbon in the Barents Sea (Vernet et al., 1998), which may serve as a major source of labile organic carbon for pelagic bacterioplankton (Poulton et al., 2016). In other words, marine phytoplankton is also thought to be the source of the Chukchi Sea’s marine humic-like fluorescence (Chen et al., 2018). Taken together, the enhanced primary production in the Arctic Ocean seems to result in increased distributions of diverse labile organic components with a predominance of carbohydrates, amino acids, and protein-like molecules in Arctic sea water.
2.4 Melted sea ice
When sea ice melts, it releases a large quantity of DOM into the surface waters of Arctic seas (Niemi et al., 2014; Retelletti Brogi et al., 2018; Retelletti Brogi et al., 2019; Piontek et al., 2021). Since 1980, both the permanent and annual central Arctic sea ice zones have diminished by approximately 13–15% every decade (Vaughan et al., 2013). The DOM content in sea ice is affected by allochthonous DOM, such as that trapped in brine channels and pores within the ice matrix (Petrich and Eicken, 2010), as well as autochthonous DOM, which is generated by bacteria and algae in the sea ice (Stedmon et al., 2007; Underwood et al., 2013). DOM is more enriched in the sea-ice matrix than in the surrounding seawater (Giannelli et al., 2001; Muller et al., 2013; Retelletti Brogi et al., 2018). For example, in Cambridge Bay, Canada, the concentration of DOM in sea ice (162-640 µM) was ~6.2 times higher than that in the surrounding seawater (Retelletti Brogi et al., 2018). The DOM extracted from sea ice was found to be labile, mostly comprising low molecular weight (LMW) molecules (<350 Da), such as amino acids and glucose (Muller et al., 2013; Jorgensen et al., 2015; Retelletti Brogi et al., 2018; Retelletti Brogi et al., 2019; Piontek et al., 2021). Protein-like compounds have been reported to be the most abundant constituents of DOM in sea ice cores (Retelletti Brogi et al., 2018; Zabłocka et al., 2020). The mean humification index (HIX) and SUVA254 values of sea ice were in low levels, ranging from 0.454 to 0.629 and 1.137 to 1.390 m2 g-1 C, respectively, indicating mostly autochthonous origin for CDOM (Zabłocka et al., 2020). Thus, the melting of Arctic sea ice caused by rising temperatures is likely to supply a large quantity of labile DOM to Arctic seawater.
2.5 Thawed permafrost soil
Under the climate warming effects, a substantial quantity of ancient frozen carbon from the permafrost soil may be mobilized and transferred to the Arctic seas (Schuur et al., 2015; O'Donnell et al., 2016; Turetsky et al., 2020; Behnke et al., 2021). It was projected that without noticeable reductions in human greenhouse gas emissions, up to 80% of near-surface permafrost is expected to disintegrate by 2100 (Slater and Lawrence, 2013). The remnants of plants and animals that have accumulated over thousands of years in permanently frozen soil are known as permafrost carbon (Zimov et al., 2006; Tarnocai et al., 2009). Permafrost is found across the Northern Hemisphere, especially on terrain surrounding the Arctic Ocean (Pugach et al., 2018; Obu et al., 2019). Permafrost covers approximately 1300–1700 Pg of soil organic carbon in the Northern Hemisphere, almost half of the total belowground organic carbon stock (Tarnocai et al., 2009; Schuur et al., 2015; Turetsky et al., 2020). The amount of DOC leached from permafrost soil has been estimated at 13.3 ± 0.4 mmol-DOC g-soil−1 day−1, according to a study based on the present permafrost at 95–105 cm depth on the North Slope of Alaska’s Imnavait Creek watershed (Ward and Cory, 2015). The DOM leached from the permafrost soil is expected to increase under a warming scenario because of deeper permafrost soil layer thawed (Slater and Lawrence, 2013). Permafrost DOM is labile to bacteria. For example, DOM in Yedoma permafrost, which is widespread in Siberia, Alaska, and Yukon, Canada (Strauss et al., 2017), contains a substantial proportion (34–62%) of highly biodegradable organic carbon (Vonk et al., 2013; Anthony et al., 2014; Spencer et al., 2015). The labile DOM comprises highly saturated and aliphatic structures (i.e., amino acids, proteins, and peptides) as well as carbohydrates (Ward and Cory, 2015; Heslop et al., 2019; Bruhn et al., 2021; MacDonald et al., 2021). Research on Canadian permafrost soil revealed that the LMW proteinaceous molecules with low aromaticity (i.e., tyrosine- and tryptophan-like components) were abundant in the permafrost leachates (Fouché et al., 2020). According to Fouché et al. (2020), permafrost stores more protein-derived DOM with a low molecular weight than the active soil layer. This was confirmed by the fluorescence index (FI) and the ratio of spectral slopes (SR), which both increased with soil core depth (upper 3 m). The Arctic sea water is likely to contain more labile organic substances (such as amino acids, polysaccharides, and protein-like components) as a result of increasing breakdown of the permafrost soil in the region.
2.6 Melted glaciers and ice sheets
Glaciers and ice sheets constitute the world’s second largest water storage area, and changes in the glacier volume driven by global warming may significantly affect the amount of DOM entering the Arctic Ocean. By 2050, the loss of global glacier mass is anticipated to result in a reduction of 15 Tg in glacial carbon reserves owing to climate change (Hood et al., 2015). Each year, the Greenlandic glacier releases DOC at a range of 0.2–0.5 Tg (Lawson et al., 2014; Pain et al., 2020). The DOC of supraglacial water and glacially fed river samples usually varies from 10 µM to 700 µM (Bhatia et al., 2010; Bhatia et al., 2013; Kellerman et al., 2021). DOM origins include aerosol and wind-blown organic material deposition on glacier surfaces, ancient terrestrial sources, inputs carried from the surface into glacier beds, and products of in situ microbial synthesis (Bhatia et al., 2010; Lawson et al., 2014; Wadham et al., 2019). 26-53% of glacial DOM is bioavailable (Lawson et al., 2014; Musilova et al., 2017). The vast majority of DOM produced by glaciers consists of protein-like, nitrogen-rich compounds with low molecular weights (Hood et al., 2009; Bhatia et al., 2010; Lawson et al., 2014; Lawson et al., 2014). Simultaneously, lignin-like and previously overridden soil and plant-derived compounds can be liberated from glacier meltwater during the late melt season (Bhatia et al., 2010). Compared to other aquatic systems (such as the Greenland and Svalbard rivers), glacial DOM is notably richer in aliphatic and peptide-like organic materials (Kellerman et al., 2021). Increased contribution of bioavailable DOM to the Arctic sea water is anticipated as a consequence of continued mass loss of the glaciers and ice sheets by the rising temperature.
2.7 Thawed subsea permafrost
Permafrost in Arctic shelf areas stores 1400 Pg C of carbon (Shakhova et al., 2010; Vonk et al., 2012). If thawed, the subsea permafrost could be an important carbon source for the Arctic Ocean. Arctic subsea permafrost consists of frozen sediment interlayered with inundated peatland that has developed in the Arctic shelf area since the Holocene transgression of 7–15 kyrs BP, which was submerged due to glacier melting and is primarily located in the Beaufort, Chukchi, Laptev, and East Siberian Seas (Romanovskii et al., 2005; Shakhova et al., 2010; Overduin et al., 2015). Recently, numerous studies have reported the possibility of the rapid thawing of subsea permafrost in the Arctic Ocean (Vonk et al., 2012; Shakhova et al., 2017; Angelopoulos et al., 2019; Semenov et al., 2020; Steinbach et al., 2021). A study conducted in the East Siberian Arctic reported 9–2240 g C m-2 y-1 of DOM flux from the Arctic shelf underlain by undersea permafrost, with the most pronounced DOM release occurring at the continuous-discontinuous transition zone of the subsea permafrost. (Chen et al., 2021). Also, the entire Arctic shelf with offshore permafrost was projected to supply DOM discharge at rates of ~0.7–1.0 Pg C yr-1 (Chen et al., 2021). DOC concentrations in sediment pore fluids in a shelf with subsea permafrost vary from ~7 mg L-1 to 395 mg L-1, with LMW (<350 Da) compounds being dominant (Overduin et al., 2015; Chen et al., 2021). Humic-like and protein-like fluorescent components have also been found in pore water collected from subsea permafrost (Chen et al., 2021). As a result, the Arctic sea water may receive a benthic outflow of various organic components from thawed subsea permafrost.
2.8 Atmospheric deposition
Melted sea ice creates an extended open sea in the Arctic Ocean, which is projected to increase DOM intake in ice-free waters through atmospheric deposition. Atmospheric particulate organic carbon of terrigenous origin, such as particulate black carbon (BC), generated from biomass and fossil fuel combustion, is a possible source of DOM in the Arctic Ocean (Khan et al., 2017; Mori et al., 2020). Globally, biomass burning produces 40–250 Tg C yr-1 of BC (Jaffé et al., 2013; Jones et al., 2020), which can be transported to the Arctic from mid- and high latitudes. This is the reason why the Arctic is recognized as a pollution receptor zone (Shindell et al., 2008; Hall and Loboda, 2017; Backman et al., 2021). Increased BC deposition has been observed in parts of the European Arctic over the last few decades (Ruppel et al., 2015). In the central Arctic Ocean, BC concentrations of 0.021 ± 0.016 μmol L−1 have been detected in seawater (Fang et al., 2016). In such an aquatic environment, BC may be oxidized by microbial or photochemical pathways, releasing DOM into the surrounding environment (Kim et al., 2004). In addition, BC is deposited on Arctic snow and sea ice (Doherty et al., 2010; Wang et al., 2011), where it is then imported into seawater when snow and sea ice melt. Recent research has shown that the concentration of dissolved BC in the Arctic Ocean ranges between 4.8 µg C L-1 and 15.5 µg C L-1 (Osterholz et al., 2014; Nakane et al., 2017; Fang et al., 2021). A fraction of condensed hydrocarbons, which are compounds deficient in oxygen and hydrogen and typically contain aromatic ring structures in DOM molecules, can be sourced from black carbon-like compounds (Kim et al., 2004; Bhatia et al., 2010). Atmospheric deposition has been established as the primary route by which terrigenous POM is directly transported to the open parts of the North Atlantic Ocean (Hernes and Benner, 2006). Thus, if the melting of sea ice in the Arctic Ocean accelerates in response to climate change scenarios, the flux of terrestrial DOM (tDOM) imported via atmospheric deposition is expected to become more significant.
2.9 Groundwater discharge
Groundwater may serve as an important source of DOM to Arctic coastal waters as permafrost thaws. A previous study reported that suprapermafrost groundwater (SPGW) can directly enter nearshore coastal waters in the Arctic (Connolly et al., 2020). DOC concentrations in the SPGW near the land-sea interface along the Alaska Beaufort Sea were up to two orders of magnitude greater than those in nearby rivers during the sampling periods in August 2011 and 2012, reaching 33 ± 2 mg C L−1. Approximately 14–71 kg of DOC can be supplied by the SPGW to the coastal ocean per kilometer of coastline every day in late summer (Connolly et al., 2020). This DOM originates from easily leachable organic matter in surface soils, as well as deeper centuries-to-millennia-old soils that extend into melting permafrost (Connolly et al., 2020). It was reported that DOC leached from permafrost zones like suprapermafrost aquifer consisted of old radiocarbon, high aromatic compounds, and hydrophobic acids (O'Donnell et al., 2012). In addition, the groundwater level has been on the rise in the Arctic region, implying an increased input of groundwater to the streamflow (Smith et al., 2007; Walvoord and Striegl, 2007; O'Donnell et al., 2012), which eventually flows into the Arctic seas. The long-term streamflow record (>30 years) in the Yukon River Basin indicates a general increasing trend in the groundwater contribution to streamflow at 0.7–0.9% yr-1 (Walvoord and Striegl, 2007). In general, it is presumed that the increase in groundwater discharge in the warming Arctic could result in a greater fraction of DOM being exported to the Arctic Ocean. Groundwater is a source of “island of stability” (IOS), which denotes the last products of DOM disintegration and includes chemically stable molecular formulas formed following DOM processing by biological and physical pathways (Lechtenfeld et al., 2014; Kellerman et al., 2018; Behnke et al., 2021). Therefore, if groundwater flow increased, the export of stable DOM/IOS to the Arctic Ocean could also rise, which adds the oceanic carbon sink the stable DOM pool (Jiao et al., 2010; Behnke et al., 2021).
2.10 Efflux from Arctic sediments
DOM flux from Arctic sediment can be another DOM input source to Arctic seawater. A previous study has shown that the heating of near-surface marine sediment causes the bulk generation and release of reactive DOM (Lin et al., 2017). Advective fluid flow (i.e., cold seeps) from the seabed may facilitate the efflux of DOM from the sediment to the overlying water (Pohlman et al., 2011; Lin et al., 2017). In addition, several studies observed DOM efflux from the Arctic sediment to the overlying water column (Chen et al., 2016; Rossel et al., 2016; Rossel et al., 2020); all suggested that the release of DOM from sediment might increase as the Arctic warms. When the Arctic is subjected to a warming climate, the release of DOM from sediment could be considerable, which is supported by several studies that mention DOM efflux from the Arctic sediment to the overlying water column (Chen et al., 2016; Rossel et al., 2016; Rossel et al., 2020). DOM in Arctic sediment originates mainly from sedimented materials of primary production and terrigenous deposits (Boucsein and Stein, 2000; Belicka et al., 2004; Chen et al., 2016; Grosse et al., 2016). The deposited DOM is further reworked in the sediment via a complex combination of physical, chemical, and biological processes (Burdige and Komada, 2015; Chen et al., 2016). It was reported that DOC concentrations in the porewater of surficial sediment (0–10 cm) exhibited a wide range of spatial variations, ranging from 0.04 mM to 1.0 mM in the Eurasian Basin and HAUSGARTEN (Fram Strait) (Rossel et al., 2016; Rossel et al., 2020) and from 1.0 mM to 12.5 mM in the Chukchi-East Siberian Seas (Chen et al., 2016). The porewater DOM extracted from surficial sediment in the Eurasian Basin was characterized by an abundance of peptide and aliphatic molecular formulas in productive ice edges, but relatively more aromatic structures in multiyear ice-covered stations (Rossel et al., 2016). In addition, the properties of the porewater DOM changed with depth in the Fram Strait. For example, surface porewaters at shallower stations (1500 m water depth) exhibited a signal of a higher and more recent OM input, which was associated with higher abundances of unsaturated aliphatic and N-containing formulas, whereas those from deeper stations presented a molecular signature of a lower and older OM input, which was linked with higher abundances of aromatic and oxygen-deficient compounds (Rossel et al., 2016; Rossel et al., 2020). With the spatial variation in porewater DOM taken into account, the warming effect can generate a benthic flux of DOM containing diverse chemical compositions to the Arctic sea water.
Overall, DOM from various sources enters the Arctic Ocean, and DOM concentrations are expected to rise owing to global warming. Imported DOM consists of both allochthonous and autochthonous components. A significant amount of labile DOM, such as amino acids, carbohydrates, glucose, LMW compounds (<350 Da), protein-like fluorescent components, and aliphatic and peptide components, are imported from the emerging sources highlighted in this article (i.e., phytoplankton, melted sea ice, melted ice sheets/glaciers, and thawed permafrost) (Table 1). Additionally, bio-refractory DOM, which includes humic-like fluorescent and aromatic compounds, is also supplied from river runoff, adjacent seas, thawed subsea permafrost, atmospheric deposition, groundwater discharge, and Arctic sediment efflux (Table 1). As bacteria and DOM have close interactions with each other, the increased DOM imports can affect the Arctic bacterial community in seawater. Before assessing potential DOM-induced alterations in bacteria caused by the impacts of a warming climate, fundamental information on the Arctic microbial population will be provided in Section 3.
3 Bacterial communities in the sea water of the Arctic Ocean
3.1 Bacterial abundance
The bacterial abundance of many Arctic Ocean sites has been studied; the majority of these studies were conducted in the summer and spring at varying depths (Table 2). In general, the number of bacteria in the Arctic Ocean has been reported to be in the 0.1–41.0 × 105 cells mL-1 range. Bacterial abundance generally peaks near the surface (euphotic zone: 30–80 m) and tends to decrease exponentially with increasing depth (Garneau et al., 2008). Bacterial distribution also varies spatially and seasonally. For example, the bacterial numbers are relatively high (~106 cells mL-1) in coastal seas (Børsheim, 2000; Howard-Jones et al., 2002; Benedikt and Rainer, 2004; Bezzubova et al., 2020) and Atlantic-influenced waters (Børsheim, 2000; Caruso et al., 2020; von Jackowski et al., 2020). Bacterial abundance tends to decrease from the coast to the central Arctic Ocean (Garneau et al., 2006; He et al., 2012; Bezzubova et al., 2020; Piontek et al., 2021); it is greater in ice-free water (106 cells mL-1) than in ice-covered sections (105 cells mL-1) (Cardozo-Mino et al., 2021). In addition, from winter to summer, the heterotrophic bacterial abundance in central Arctic sea waters (0–40 m) almost doubles, while it remains relatively constant at a depth of 80–120 m (Sherr et al., 2003).
3.2 Bacterial cell size and activity
Bacteria in the Arctic Ocean are often assumed to have small cell sizes and low levels of activity. Throughout the spring and summer, bacteria have an average cell volume of approximately 0.05–0.09 µm3 in the Chukchi Sea and the Canada Basin (Kirchman et al., 2007). The bacterial growth rates in the top 50 m of seawater were 0.004 ± 0.003 d−1 in winter and 0.019 ± 0.009 d−1 in summer, with an average doubling period of 300 and 46 days, respectively (Sherr and Sherr, 2003). The ratios of bacterial production (BP) to primary production (PP) vary from 0.03 to 0.32 (Sturluson et al., 2008; Uchimiya et al., 2016; Piontek et al., 2021); in several sites of the Arctic Ocean, these ratios were higher than 0.11, a common value in other oceans (Kirchman et al., 1993; Ducklow et al., 1995), which indicates that the fraction of PP that passes through DOM-bacteria coupling is not low under cold conditions, signifying an important role of bacteria in the carbon dynamics within the Arctic Ocean. Bacterial assemblages in marine oligotrophic settings, such as the Arctic Ocean, exhibit a high degree of metabolic adaptability to be able to access scarce carbon sources for growth (Sala et al., 2008). In addition, bacterial communities in the Arctic can adjust their metabolism to the high-latitude cycles of PP (Hop and Wiencke, 2019). It has been observed that organic matter breakdown by extracellular enzyme activity occurs in cold Arctic seas at rates comparable to those recorded in warmer lower-latitude seas (Huston and Deming, 2002). Furthermore, a previous study reported that microbial communities in (near-) Arctic climates functioning similarly to those in warmer climates, with algal carbon processed at similar rates and via similar paths (Oakes et al., 2016).
3.3 Bacterial composition
Bacteria in the Arctic Ocean mostly include newly identified bacterial taxa. Several recent studies have shown that the phylogenetic composition of Arctic bacteria is dominated by Alphaproteobacteria, Gammaproteobacteria, and Bacteroidetes (Table 3). At the sub-level, Pelagibacter (the SAR11 clade) accounts for the majority of Alphaproteobacteria (Bano and Hollibaugh James, 2002). The majority of Bacteroidetes sequences belong to Polaribacter (Comeau et al., 2011). Although the same predominant classes of bacteria are found in other ocean systems, including the Antarctic (Murray and Grzymski, 2007), the bacteria in the Arctic are evolutionarily distinct from all other isolates (Bano and Hollibaugh James, 2002). It was revealed that 441 of the 530 metagenome-assembled microbial genomes from 41 Arctic seawater samples belonged to previously unknown species, while 299 genomes exhibited an exclusively polar distribution (Royo-Llonch et al., 2021).
There are indications that bacterial populations in the Arctic are shifting as a consequence of global warming effects. Ramachandran et al. (2021) uncovered a previously unknown freshwater-originated Methylophilaceae clade (BS01) in the surface seawater of the Canada Basin. As freshwater bacteria transported by rivers rarely survive in the Arctic Ocean for long (Elifantz et al., 2007), this finding suggests the possibility of a bacterial evolutionary transition from freshwater to marine water in the Arctic Ocean, which receives increased water inputs from Arctic rivers as a result of climate warming effects (Ramachandran et al., 2021). In addition, Gammaproteobacteria and Flavobacteriia have been reported to be abundant in Atlantic-influenced waters (i.e., the Fram Strait, Svalbard archipelago, and the Norwegian Sea) (Fadeev et al., 2018; Carter-Gates et al., 2020; Thomas et al., 2020), which may reflect “Atlantification” in the Arctic Ocean. Notably, the Rhodobacteraceae and SAR11 clades (both members of the class Alphaproteobacteria) have been found in open ocean regions, such as Chukchi Borderland (Zeng et al., 2013; Lee et al., 2019) and the Fram Strait (Cardozo-Mino et al., 2021). Both Rhodobacteraceae and SAR11 abundances showed substantially positive correlations with water temperature (Pearson’s correlation coefficient > 0.5, p-value < 0.05) (Cardozo-Mino et al., 2021), suggesting a close linkage between cell prevalence and the warm Atlantic and Pacific input waters. Photoheterotrophic bacteria have recently been discovered in the Arctic Ocean (Cottrell Matthew and Kirchman David, 2009; Boeuf et al., 2013; Nguyen et al., 2015). This bacterial type can depart from completely heterotrophic living by receiving energy through nontraditional methods associated with increased sunlight penetration into surface waters caused by the melting of Arctic sea ice (Boeuf et al., 2014).
Overall, the bacteria in the Arctic are distinct from previously known taxa. According to the current understanding, they can be susceptible to environmental changes. This sensitivity raises more concerns about changes that may occur in bacterial populations as a result of increasing DOM influx into the Arctic Ocean.
4 Effects of DOM changes on bacterial communities in the Arctic Ocean
4.1 Bacterial abundance, cell size, and activity
Increased DOM can stimulate bacterial growth in the Arctic Ocean. The addition of glucose to western Arctic seawater resulted in the increase of leucine incorporation that was almost three times higher than that of the control at the same low temperature (Kirchman et al., 2005). Leucine is required to create new cellular proteins, and its incorporation rate can be applied to predict the produced amount of bacterial biomass (Piontek et al., 2021). Similarly, the addition of DOM produced from diatoms or permafrost results in an increase in the number and production of bacteria in the Arctic (Dadaglio et al., 2018; Müller et al., 2018). The yields of bacterial growth were found to range between 0.35 d-1 and 0.67 d-1 when the DOM obtained from sea ice was added naturally (Niemi et al., 2014). Based on these experiments, it was deduced that the addition of DOM may be more effective in stimulating the development of bacterial communities in cold waters than in warm seas (Wiebe et al., 1992; Wiebe et al., 1993). In addition, the lability of DOC is a critical factor in determining the quantity and activity of bacteria under polar conditions (Arnosti et al., 2011; Caruso et al., 2020; von Jackowski et al., 2020; Piontek et al., 2021). Numerous studies have shown a high degree of bacterial consumption in the Arctic Ocean, which is favorably correlated with amino acids, carbohydrates (i.e., glucose), and protein-like fluorescent DOM (Rich et al., 1997; Lønborg et al., 2010; Tisserand et al., 2020; Bruhn et al., 2021).
The addition of DOC to Arctic bacteria can also result in an increase in cell size and activation. For example, the release of DOM from Arctic sea ice (at 840 µmol L-1) resulted in a 2.1-3.2-fold increase in the apparent cell size of Arctic surface seawater bacteria (Niemi et al., 2014). Müller et al. (2018) noticed an increase in cell size in the coastal Arctic due to the input of tDOM. In addition, after sea-ice DOM enrichment, Niemi et al. (2014) found that the entire prokaryotic population was characterized by cells with a high nucleic acid (HNA) content (on average 97% of the total prokaryotic abundance). The quantity of HNA bacteria in the surface waters of Franklin Bay increased 5–6 weeks after phytoplankton development began in spring, growing from 1 × 105 to 3 × 105 cells mL− 1 during an 8-week period at a net growth rate of 0.018 d− 1 (Belzile et al., 2008). The presence of HNA suggests that prokaryotes develop metabolic activity and enter the exponential growth phase (Niemi et al., 2014). Müller et al. (2018) demonstrated that the addition of aged tDOM extracted from the active layer of permafrost soil increased the number of HNA cells. Consistently, bacteria of larger size (microbial size < 3.0 µm) are capable of metabolizing a broader spectrum of DOM, including aromatic terrestrial material from river discharge, than those with smaller cells (microbial size < 0.2 µm and < 0.7 µm) (Grunert et al., 2021). In summary, the reviewed literature reveals that the increase in the DOM fluxes to the Arctic Ocean can impact several features of bacterial community (Figure 3). Increased DOM can significantly increase the bacterial population, cell size, and metabolic activity, thereby releasing a substantial quantity of CO2 into the atmosphere.
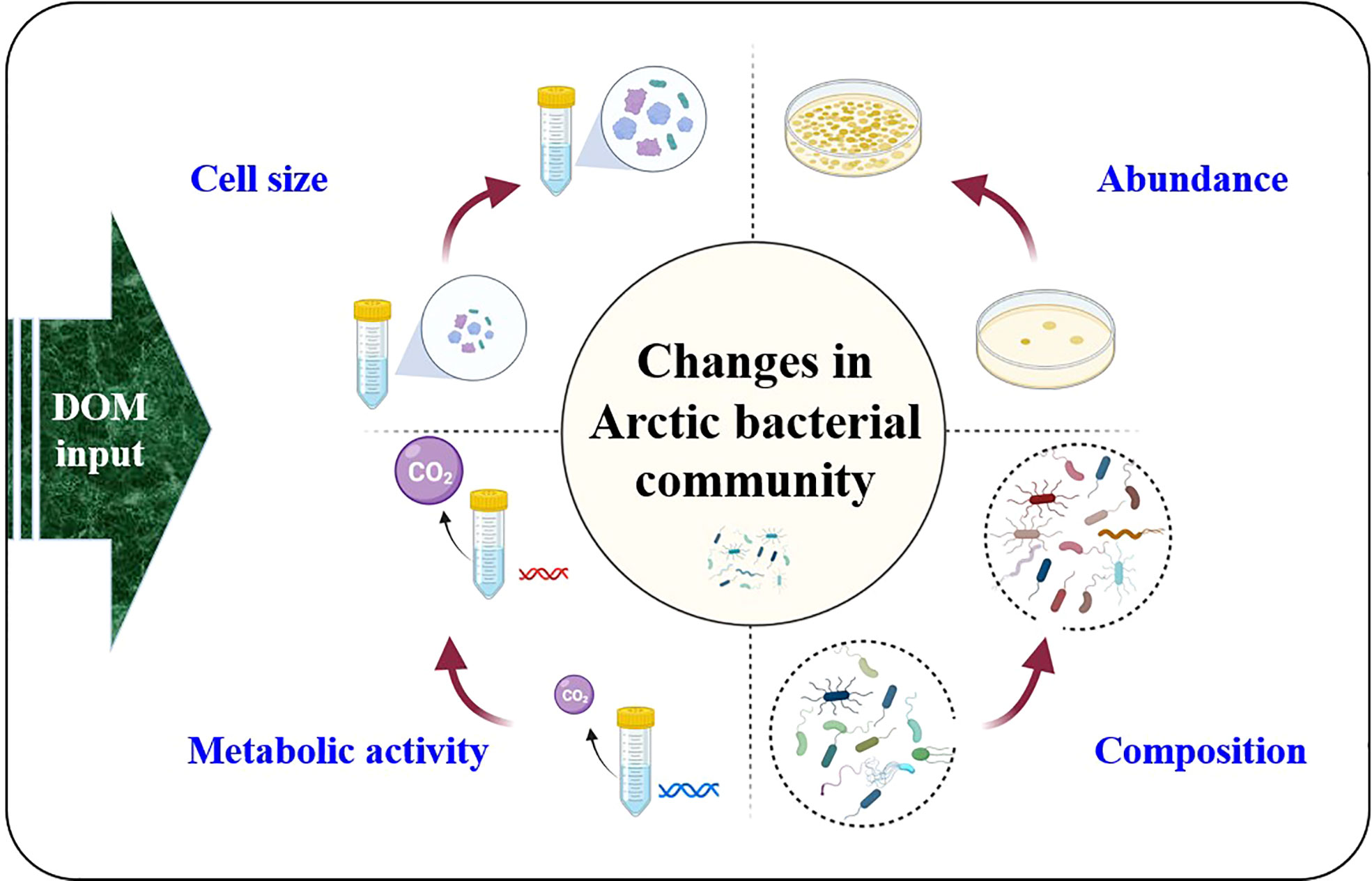
Figure 3 Elevation in population, size, metabolic activity, and structure of the bacterial communities in the Arctic Ocean as a result of the increased DOM in seawater.
4.2 Bacterial enzymatic system
The bacterioplankton populations in the Arctic are capable of enzymatic adaptations to DOM components. The genetic capability of bacterial strains to generate extracellular enzymes (e.g., leucine aminopeptidase peptidase, glucosidase, polysaccharide hydrolase, etc.) is related to the degradability of DOM (Arnosti, 2010; Balmonte et al., 2020; Tisserand et al., 2020). The majority of Arctic microbial communities have large genomes and the capacity for rapid generation rates, which may facilitate adaptation to a copiotrophic lifestyle in nutrient-dense environments, particularly those rich in carbon (Royo-Llonch et al., 2021). Several recent studies have documented alterations in the metabolism of bacterioplankton populations through enzymatic modifications in response to the diverse chemical composition of DOM in the Arctic Ocean. For example, bacterioplankton in the Fram Strait, Greenland Sea, and Kongsfjorden of the Arctic Ocean exhibited accelerated enzymatic hydrolysis of dissolved mixed carbohydrates that were produced in substantial amounts as a result of phytoplankton blooms and melted sea ice (Sala et al., 2010; Piontek et al., 2014; Jain and Krishnan, 2017). In addition, a metagenomic analysis of the Canada Basin of the western Arctic Ocean found that the pelagic phylum Chloroflexi was enriched with aromatic chemical degradation genes, which were acquired mostly by lateral exchange from terrestrial bacteria (Colatriano et al., 2018). Overall, changes in DOM have impacts on bacterial enzymatic activity in the Arctic seawater. Furthermore, alteration of the bacterial enzymatic system can eventually result in a shift in the taxonomic composition of the Arctic Ocean subpopulation community (Figure 3).
4.3 Structure of the bacterial community
Different types of DOM input can promote unique bacterial taxonomic groups. By choosing the most sensitive species with the proper genetic material to break down DOM at their disposal in their unique habitat, newly input DOM can change the bacterial community makeup in the Arctic Ocean (Tisserand et al., 2020). For example, Alphaproteobacteria have been shown to utilize DOM with an LMW (Nikrad et al., 2012; Amaral et al., 2016). Labile DOM from phytoplankton and sea ice is likely to be released more in open water areas than in other environments, thereby facilitating the growth of Alphaproteobacteria in open waters far from coastal areas in the Arctic Ocean (Bano and Hollibaugh James, 2002; Galand et al., 2008; Han et al., 2014). In contrast, Gammaproteobacteria are well-known for their ability to break down a diverse spectrum of carbon sources (Alonso-Sáez et al., 2008; Wilson et al., 2017; Lund Paulsen et al., 2019), which allows them to be widely distributed in coastal areas (Rex et al., 2007; Kellogg and Deming, 2009) that receive high tDOM from the river discharge.
The labile DOM input derived from phytoplankton blooms and melted sea ice facilitates the establishment of particular bacterial species. These are Roseobacter and operational taxonomic units close to Pseudoalteromonas of the Alphaproteobacteria class, Oceanospirillaceae and Alteromonadaceae of the Gammaproteobacteria class, and Flavobacteriaceae, Polaribacter, Formosa, and Tenacibaculum of the Flavobacteriia class in the Bacteroidetes phylum (Alonso-Sáez et al., 2008; Wilson et al., 2017; Dadaglio et al., 2018; Underwood et al., 2019; Piontek et al., 2021). The metabolic features of these bacteria allow them to respond rapidly to phytoplankton blooms linked with organic substances (Buchan et al., 2014). In particular, there are specific relationships among phytoplankton, roseobacteria, and flavobacteria in oceans (Buchan et al., 2014). Roseobacter possess a variety of metabolic characteristics that likely favor interactions with DOM derived from seawater phytoplankton (Buchan et al., 2014). Roseobacter isolates also have larger genomes (~4.5 Mb) and greater gene content (Newton et al., 2010) than other abundant marine lineages that are geographically restricted to marine planktonic settings (Rocap et al., 2003; Giovannoni Stephen et al., 2005). Both these features may be critical for the abundance succession of Roseobacter over phytoplankton blooms in the Arctic Ocean. Flavobacteriia genomes contain several physiological mechanisms that are likely involved in their interaction with phytoplankton-derived DOM (Buchan et al., 2014).
The addition of DOM from terrestrial sources may promote the formation of new bacterial groups in the Arctic Ocean. For example, Rhodobacteraceae (Sipler et al., 2017) and Sulfitobacter (Dyda et al., 2009) of the Alphaproteobacteria class, Colwellia (Sipler et al., 2017), Glaciecola, and Marinomonas (Müller et al., 2018), Balneatrix and SAR92 clade (Lund Paulsen et al., 2019) within Gammaproteobacteria, Arcobacter sp (Sipler et al., 2017). of the Epsilonproteobacteria class, and Polaribacter (Sipler et al., 2017) of Bacteroidetes increase their relative abundance with increasing tDOM concentration. These bacteria can decompose a wide range of complex organic substances. Marinomonas sp. includes several genes encoding enzymes involved in the breakdown of aromatic hydrocarbons (Liao et al., 2015). Arcobacter-affiliated sequences comprise genes encoding proteases, peptidases, and oligopeptidases, as well as enzymes required for the oxidative tricarboxylic acid cycle (He et al., 2020). In particular, Polaribacter and Colwellia can use a variety of organic substrates. Polaribacter are psychrophilic bacteria found in seawater and ice (Sipler et al., 2017; Yergeau et al., 2017), that are capable of decomposing polymeric organic molecules (Sipler et al., 2017). The genus Colwellia is a polar taxonomic species that produces well-adapted extracellular enzymes for the degradation of organic molecules (Methé et al., 2005). In general, future changes in the delivery of DOM to the Arctic Ocean may result in substantial alterations in the composition of the bacterial population.
Taken together, the critical changes in DOM influxes to the Arctic seas under climate warming effects have numerous implications on bacterial community, which was simplistic shown in Figure 4. Increased DOM input levels tend to modify the entire bacterial community in terms of quantity, cell size, metabolism, and composition.
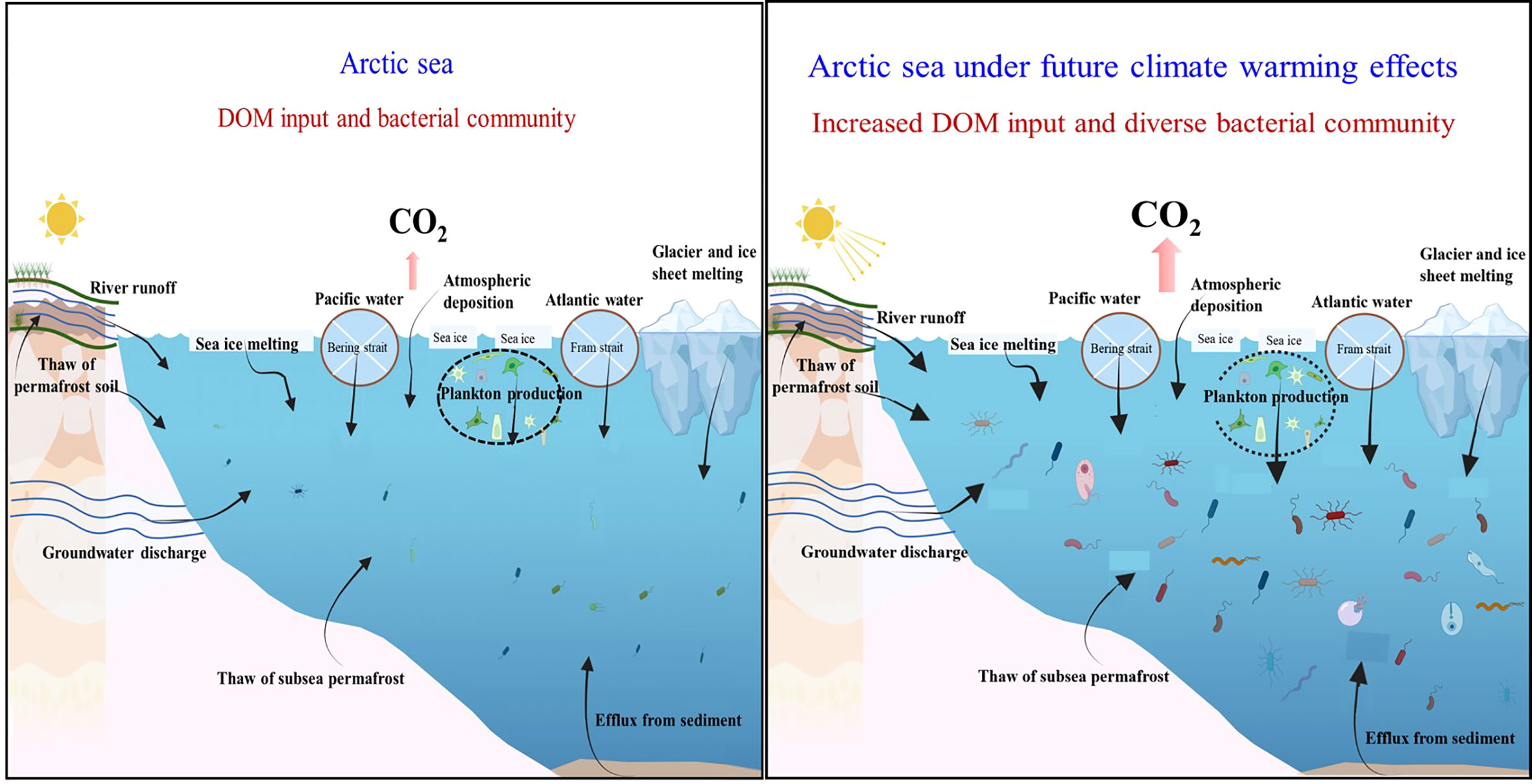
Figure 4 Changes in DOM input and its consequence on the bacterial community in the Arctic Ocean under the effects of climate warming. The arrows represent DOM input.
5 Changes of DOM quality in the Arctic Ocean and their consequences on bacteria under warming effects
5.1 Variations in DOM quality in the Arctic Ocean
It is well recognized that the Arctic Ocean is an oligotrophic marine ecosystem with insufficient carbon substrates (Sala et al., 2008; Sert et al., 2022). The chemical makeup of DOM in the Arctic sea water varies with changing dominant input sources (or different regions) with dissimilar compositions of different organic components (Table 1). In the central Arctic Ocean, carbohydrates (i.e., glucose) and five distinctive fluorescent components, including tryptophan-like, tyrosine-like, terrestrial humic-like and two humic-like components, were detected (Piontek et al., 2021; Williford et al., 2021), and intensity of in-situ DOM fluorescence (water depths <25 m) reached >0.030 RU (up to 0.089 RU) (Stedmon et al., 2021). The Canadian Basin surface waters were characterized by high percentage of autochthonous CDOM (a375 = 0.21 m-1), and the surface waters of the Eurasian Basin (100 m depth) showed a riverine feature (a375 = 0.41 m-1) (Rossel et al., 2020). Seawater samples taken along a transect over the Chukchi shelf and Arctic fjords exhibited the higher values of CDOM and FDOM intensities than those of the central Arctic Ocean (Chen et al., 2018; Retelletti Brogi et al., 2019; Chen et al., 2021; Cai et al., 2022). Temporal and vertical changes in DOM quality in the Arctic Ocean are also observed. In the central Canada Basin of the Arctic Ocean, a long-term research for DOM composition (from 2007 to 2017) found a substantial upward trend of protein-like component with the yearly increase from 0.0021 to 0.0046 RU (DeFrancesco and Guéguen, 2021). Furthermore, microbial humic-like and terrestrial humic-like components were all significantly higher in the lower polar mixed layer (LPML) at a depth of 31 m in the Canada Basin than in the upper water layer (average depth of 12 m) (p < 0.05) (DeFrancesco and Guéguen, 2021). The authors indicated that as sea ice cover diminished in the warming Arctic Ocean, photodegradation increased, leading to a more terrigenous DOM in the LPML. Overall, the current data suggested that carbohydrates (i.e., glucose), proteinaceous (i.e., amino acid and protein-like components), and terrigenous characteristics (i.e., humic-like components) in DOM are all expected to rise in their abundances in surface Arctic sea water as a result of increasing DOM input sources caused by warming.
5.2 Impacts of DOM quality on bacterial communities in the Arctic Ocean
Changes in DOM quality in Arctic waters (i.e., increased prevalence of various organic compounds) have impacts on the growth of microbial communities in Arctic waters. Many previous studies have reported that bacterial utilization positively correlates to the succession patterns of various organic components such as amino acids, carbohydrates, and proteinenous compounds (Rich et al., 1997; Jørgensen et al., 2014; Lund Paulsen et al., 2019; Tisserand et al., 2020; von Jackowski et al., 2020; Bruhn et al., 2021). Amino acids are a molecular indicator of DOM bioavailability (Benner, 2003; Lund Paulsen et al., 2019). Aromatic amino acids (tryptophan, tyrosine, and phenylalanine) are connected to protein-like fluorescent DOM, which has been proposed as a feasible tracer for bio-labile DOM (Yamashita and Tanoue, 2003; Lønborg et al., 2010). These labile organic components are subject to rapid (hours-weeks) microbial utilization and transformation (Hansell and Carlson, 2014). A previous study investigated utilization of the prokaryotic community in western Arctic Ocean surface water of different classes of labile organic compounds, including amino acids, diatom-derived extracellular polymers, protein, and glucose and reported that amino acids were the most readily assimilated by the bacteria (50–60%), followed by protein (20–30%), glucose (10–20%), and the extracellular polymeric substances (EPS) produced from diatoms (30–40%) (Elifantz et al., 2007). The study also found that Alphaproteobacteria contributed more to the degradation of amino acids and glucose, while Cytophaga-like bacteria (Bacteroides-Cytophaga-Flexibacter group) were more involved in the assimilation of proteins and EPS and Gammaproteobacteria contributed equally to the assimilation of both DOM subgroups (i.e., amino acids plus glucose and proteins plus EPS) (Elifantz et al., 2007).
Bacteria in Arctic sea water tend to degrade humic-like fluorescent components at slow rates. For example, bacterial communities from the Chukchi Sea water consumed ~7% of “humic-like” terrestrial-derived DOM within 4–6 d (Sipler et al., 2017). Another experimental study using microbial communities in Fram Strait seawater revealed only 11% consumption of humic substances over 400 h of incubation (Ingeborg, 1999). Humic-like fluorescent DOM is considered to be an indicator for recalcitrant DOM (Jørgensen et al., 2014). A very long period is usually required for the complete biodegradation of refractory compounds, up to 16,000 years or longer (Hansell, 2013). Lund Paulsen et al. (2019) observed that a reduction in visible FDOM, which is often associated with humic-like components in subsurface waters, corresponded with an increase in bacterial population and activity, suggesting an active bacterial uptake or modification of the DOM fraction in Arctic fjord water. The breakdown of visible FDOM seems to be linked to certain bacterial taxa in the class Gammaproteobacteria (Lund Paulsen et al., 2019). In general, different microbial groups are likley to be stimulated by various DOM inputs, all resulting in the enhanced diversity in the microbial community in the Arctic waters. In summary, enhanced DOM import fluxes into Arctic seas as a consequence of climate change are expected to result in increased CO2 emissions and heighten the likelihood that resident bacterial communities in the Arctic Ocean will eventually be replaced.
6 Conclusions and perspectives
The Arctic Ocean plays a critical role in global climate control, and is a particularly vulnerable system that has already undergone significant disturbances owing to climate change (Peterson Bruce et al., 2002; Camill, 2005). One of the most significant disturbances in Arctic waters is likely to be the increase in the concentration of DOM originating from multiple sources. Bacterial communities in the Arctic are highly specialized and highly susceptible to environmental stresses, thereby being able to respond rapidly to changes in DOM concentrations. As was detailed in this review, increased DOM levels may increase the population, production, and metabolic activity of bacteria in the seawater of the Arctic Ocean, thereby releasing a significant amount of CO2 into the atmosphere. In addition, the Arctic sea water is transitioning from a narrow range of organic substrates to a diverse spectrum of DOM constituents composed of carbohydrates (i.e., glucose), proteinaceous (i.e., amino acid and protein-like components), and terrigenous characteristics (i.e., humic-like components). By selecting the most sensitive species with the appropriate genetic material to degrade the extra DOM, the DOM input may change bacterial communities, perhaps resulting in the emergence of replacement resident populations. In summary, global warming-induced changes in the DOM input to the Arctic Ocean have massive consequences for entire bacterial communities in the Arctic (Figure 4). This finding emphasizes the importance of determining the rate of DOM entry into the Arctic and provides new aspects for estimating the influence of climate change on the Arctic Ocean from various perspectives.
An increase in DOM can further alter the dynamics of marine food webs and DOM cycling in the Arctic Ocean. As a higher DOM intake boosts bacterial development, the consumption of bacteria by protist grazers increases accordingly; then, the material and energy consumed by the grazers can be transferred to larger species in the Arctic Ocean (Kirchman et al., 2009). Grazers and larger creatures can also generate and mineralize DOM. A proportion of labile DOM can be rapidly utilized in microbial processes, while refractory DOM can persist for a long time in the ocean (Jiao et al., 2010; Hansell, 2013). In addition, approximately 2.9–10.3 Tg C yr-1 of tDOM discharged by rivers to the Arctic Ocean is exported to the North Atlantic through the East Greenland Current’s surface waters (Opsahl et al., 1999). This reveals a significant effect of increased DOM input in the Arctic on carbon fluxes in the oceans (Boeuf et al., 2014). Therefore, further research may focus on the impacts of DOM input to the Arctic on the carbon cycling of the whole Arctic Ocean. Investigations of changes in DOM flow patterns in oceans as a result of DOM sources entering Arctic waters are also a crucial topic for future studies.
Author contributions
HN was responsible for the conceptualization, investigation, visualization, and writing the original draft. YL made her contribution to reviewing the draft and validation. JKH made a contribution to funding acquisition. SH contributed to reviewing the original draft. MC made a contribution to the conceptualization and reviewing the original manuscript. JH was responsible for the project administration, conceptualization, and also writing the original manuscript. All authors contributed to the article and approved the submitted version.
Funding
This work was supported by the National Research Foundation of Korea (NRF) grant funded by the Korean government (MSIP) (No. 2020R1A4A2002823and 2021M3I6A1091270) and the Korean Ministry of Ocean and Fisheries (KIMST grant No. 1525011795).
Conflict of interest
The authors declare that the research was conducted in the absence of any commercial or financial relationships that could be construed as a potential conflict of interest.
Publisher’s note
All claims expressed in this article are solely those of the authors and do not necessarily represent those of their affiliated organizations, or those of the publisher, the editors and the reviewers. Any product that may be evaluated in this article, or claim that may be made by its manufacturer, is not guaranteed or endorsed by the publisher.
References
Alonso-Sáez L., Sánchez O., Gasol J. M., Balagué V., Pedrós-Alio C. (2008). Winter-to-summer changes in the composition and single-cell activity of near-surface Arctic prokaryotes. Environ. Microbiol. 10, 2444–2454. doi: 10.1111/j.1462-2920.2008.01674.x
Amaral V., Graeber D., Calliari D., Alonso C. (2016). Strong linkages between DOM optical properties and main clades of aquatic bacteria. Limnol. Oceanogr. 61, 906–918. doi: 10.1002/lno.10258
Ambrose W. G., Quillfeldt C. V., Clough L. M., Tilney P. V. R., Tucker T. (2005). The sub-ice algal community in the chukchi sea: large- and small-scale patterns of abundance based on images from a remotely operated vehicle. Polar Biol. 28, 784–795. doi: 10.1007/s00300-005-0002-8
Amon R. M. W., Benner R. (2003). Combined neutral sugars as indicators of the diagenetic state of dissolved organic matter in the Arctic ocean. Deep Sea Res. Part I Oceanogr. Res. Pap. 50, 151–169. doi: 10.1016/S0967-0637(02)00130-9
Anderson L. G., Amon R. M. W. (2015). Biogeochemistry of marine dissolved organic matter. 2nd ed. Eds. Hansell D. A., Carlson C. A. (Boston: Academic Press), 609–633.
Angelopoulos M., Westermann S., Overduin P., Faguet A., Olenchenko V., Grosse G., et al. (2019). Heat and salt flow in subsea permafrost modeled with CryoGRID2. J. Geophys. Res. Earth Surf. 124, 920–937. doi: 10.1029/2018JF004823
Anthony K. M. W., Zimov S. A., Grosse G., Jones M. C., Anthony P. M., Iii F. S. C., et al. (2014). A shift of thermokarst lakes from carbon sources to sinks during the Holocene epoch. Nature 511, 452–456. doi: 10.1038/nature13560
Arnosti C. (2010). Microbial extracellular enzymes and the marine carbon cycle. Ann. Rev. Mar. Sci. 3, 401–425. doi: 10.1146/annurev-marine-120709-142731
Arnosti C., Steen A. D., Ziervogel K., Ghobrial S., Jeffrey W. H. (2011). Latitudinal gradients in degradation of marine dissolved organic carbon. PloS One. 6, e28900. doi: 10.1371/journal.pone.0028900
Årthun M., Eldevik T., Smedsrud L. H., Skagseth Ø, Ingvaldsen R. B. (2012). Quantifying the influence of Atlantic heat on barents Sea ice variability and retreat. J. Clim 25, 4736–4743. doi: 10.1175/jcli-d-11-00466.1
Børsheim K. Y. (2000). Bacterial production rates and concentrations of organic carbon at the end of the growing season in the Greenland Sea. Aquat. Microb. Ecol. 21, 115–123. doi: 10.3354/ame021115
Backman J., Schmeisser L., Asmi E. (2021). Asian Emissions explain much of the Arctic black carbon events. Geophys. Res. Lett. 48, e2020GL091913. doi: 10.1029/2020GL091913
Balmonte J. P., Hasler-SheEtal H., Glud R. N., Andersen T. J., Sejr M. K., Middelboe M., et al. (2020). Sharp contrasts between freshwater and marine microbial enzymatic capabilities, community composition, and DOM pools in a NE Greenland fjord. Limnol. Oceanogr 65, 77–95. doi: 10.1002/lno.11253
Bano N., Hollibaugh James T. (2002). Phylogenetic composition of bacterioplankton assemblages from the Arctic ocean. Appl. Environ. Microbiol. 68, 505–518. doi: 10.1128/AEM.68.2.505-518.2002
Behnke M. I., McClelland J. W., Tank S. E., Kellerman A. M., Holmes R. M., Haghipour N., et al. (2021). Pan-Arctic riverine dissolved organic matter: Synchronous molecular stability, shifting sources and subsidies. Glob. Biogeochem. Cycles 35, e2020GB006871. doi: 10.1029/2020GB006871
Belicka L. L., Macdonald R. W., Yunker M. B., Harvey H. R. (2004). The role of depositional regime on carbon transport and preservation in Arctic ocean sediments. Mar. Chem. 86, 65–88. doi: 10.1016/j.marchem.2003.12.006
Belzile C., Brugel S., Nozais C., Gratton Y., Demers S. (2008). Variations of the abundance and nucleic acid content of heterotrophic bacteria in Beaufort shelf waters during winter and spring. J. Mar. Syst. 74, 946–956. doi: 10.1016/j.jmarsys.2007.12.010
Benedikt M., Rainer M. W. A. (2004). Heterotrophic bacterial activity and fluxes of dissolved free amino acids and glucose in the Arctic rivers ob, yenisei and the adjacent kara Sea. Aquat. Microb. Ecol. 37, 121–135. doi: 10.3354/ame037121
Benner R. (2003). Aquatic ecosystems. Eds. Findlay S. E. G., Sinsabaugh R. L. (Burlington: Academic Press), 121–137.
Benner R., Benitez-Nelson B., Kaiser K., Amon R. M. W. (2004). Export of young terrigenous dissolved organic carbon from rivers to the Arctic ocean. Geophys. Res. Lett. 31, L05305. doi: 10.1029/2003GL019251
Benner R., Louchouarn P., Amon R. M. W. (2005). Terrigenous dissolved organic matter in the Arctic ocean and its transport to surface and deep waters of the north Atlantic. Glob. Biogeochem. Cycles. 19, GB2025. doi: 10.1029/2004GB002398
Bezzubova E., Seliverstova A., Zamyatin I., Romanova N. (2020). Heterotrophic bacterioplankton of the laptev and East Siberian sea shelf affected by freshwater inflow areas. Oceanology. 60, 62–73. doi: 10.1134/S0001437020010026
Bhatia M. P., Das S. B., Longnecker K., Charette M. A., Kujawinski E. B. (2010). Molecular characterization of dissolved organic matter associated with the Greenland ice sheet. Geochim. Cosmochim. Acta 74, 3768–3784. doi: 10.1016/j.gca.2010.03.035
Bhatia M. P., Das S. B., Xu L., Charette M. A., Wadham J. L., Kujawinski E. B. (2013). Organic carbon export from the Greenland ice sheet. Geochim. Cosmochim. Acta 109, 329–344. doi: 10.1016/j.gca.2013.02.006
Bif M. B., Hansell D. A. (2019). Seasonality of dissolved organic carbon in the upper northeast pacific ocean. Glob. Biogeochem. Cycles. 33, 526–539. doi: 10.1029/2018GB006152
Bintanja R., Andry O. (2017). Towards a rain-dominated Arctic. Nat. Clim. Change 7, 263–267. doi: 10.1038/nclimate3240
Birdwell J. E., Engel A. S. (2010). Characterization of dissolved organic matter in cave and spring waters using UV–vis absorbance and fluorescence spectroscopy. Org. Geochem. 41, 270–280. doi: 10.1016/j.orggeochem.2009.11.002
Boeuf D., Cottrell M. T., Kirchman D. L., Lebaron P., Jeanthon C. (2013). Summer community structure of aerobic anoxygenic phototrophic bacteria in the western Arctic ocean. FEMS Microbiol. Ecol. 85, 417–432. doi: 10.1111/1574-6941.12130
Boeuf D., Humily F., Jeanthon C. (2014). Diversity of Arctic pelagic bacteria with an emphasis on photoheterotrophs: A review. Biogeosciences. 11, 3309–3322. doi: 10.5194/bg-11-3309-2014
Borsheim K. Y., Drinkwater K. F. (2014). Different temperature adaptation in Arctic and Atlantic heterotrophic bacteria in the barents Sea polar front region. J. Mar. Syst. 130, 160–166. doi: 10.1016/j.jmarsys.2012.09.007
Boucsein B., Stein R. (2000). Particulate organic matter in surface sediments of the laptev Sea (Arctic ocean): Application of maceral analysis as organic-carbon-source indicator. Mar. Geol. 162, 573–586. doi: 10.1016/S0025-3227(99)00066-3
Bruhn A. D., Stedmon C. A., Comte J., Matsuoka A., Speetjens N. J., Tanski G., et al. (2021). Terrestrial dissolved organic matter mobilized from eroding permafrost controls microbial community composition and growth in Arctic coastal zones. Front. Earth Sci. 9. doi: 10.3389/feart.2021.640580
Buchan A., LeCleir G. R., Gulvik C. A., González J. M. (2014). Master recyclers: features and functions of bacteria associated with phytoplankton blooms. Nat. Rev. Microbiol. 12, 686–698. doi: 10.1038/nrmicro3326
Burdige D. J., Komada T. (2015). Biogeochemistry of marine dissolved organic matter. 2nd ed. Eds. Hansell D. A., Carlson C. A. (Boston: Academic Press), 535–577.
Cai X., Zhuang Y., Li H., Xu J., Jin H., Chen J. (2022). Spatial distribution of colored dissolved organic matter in the Western Arctic ocean. J. Mar. Sci. Eng. 10, 352. doi: 10.3390/jmse10030352
Camill P. (2005). Permafrost thaw accelerates in Boreal peatlands during late-20th century climate warming. Clim. Change. 68, 135–152. doi: 10.1007/s10584-005-4785-y
Cardozo-Mino M. G., Fadeev E., Salman-Carvalho V., Boetius A. (2021). Spatial distribution of Arctic bacterioplankton abundance is linked to distinct water masses and summertime phytoplankton bloom dynamics (Fram strait, 79°N). Front. Microbiol. 12. doi: 10.3389/fmicb.2021.658803
Carlson C. (2014). “DOM sources, sinks, reactivity, and budgets,” in Biogeochemistry of marine dissolved organic matter. Eds. Carlson C. A., Hansell D. A. (San Diego, CA: Academic Press), 65–126.
Carter-Gates M., Balestreri C., Thorpe S. E., Cottier F., Baylay A., Bibby T. S., et al. (2020). Implications of increasing Atlantic influence for Arctic microbial community structure. Sci. Rep. 10, 19262. doi: 10.1038/s41598-020-76293-x
Caruso G., Madonia A., Bonamano S., Miserocchi S., Giglio F., Maimone G., et al. (2020). Microbial abundance and enzyme activity patterns: Response to changing environmental characteristics along a transect in kongsfjorden (Svalbard islands). J. Mar. Sci. Eng. 8 (10), 824. doi: 10.3390/jmse8100824
Chen M., Jung J., Lee Y. K., Hur J. (2018). Surface accumulation of low molecular weight dissolved organic matter in surface waters and horizontal off-shelf spreading of nutrients and humic-like fluorescence in the chukchi Sea of the Arctic ocean. Sci. Total Environ. 639, 624–632. doi: 10.1016/j.scitotenv.2018.05.205
Chen M., Kim J.-H., Lee Y. K., Lee D.-H., Jin Y. K., Hur J. (2021). Subsea permafrost as a potential major source of dissolved organic matter to the East Siberian Arctic shelf. Sci. Total Environ. 777, 146100. doi: 10.1016/j.scitotenv.2021.146100
Chen M., Kim J.-H., Nam S.-I., Niessen F., Hong W.-L., Kang M.-H., et al. (2016). Production of fluorescent dissolved organic matter in Arctic ocean sediments. Sci. Rep. 6, 39213. doi: 10.1038/srep39213
Chen M., Nam S. I., Kim J. H., Kwon Y. J., Hong S., Jung J., et al. (2017). High abundance of protein-like fluorescence in the amerasian basin of Arctic ocean: Potential implication of a fall phytoplankton bloom. Sci. Total Environ. 599, 355–363. doi: 10.1016/j.scitotenv.2017.04.233
Colatriano D., Tran P. Q., Guéguen C., Williams W. J., Lovejoy C., Walsh D. A. (2018). Genomic evidence for the degradation of terrestrial organic matter by pelagic Arctic ocean chloroflexi bacteria. Commun. Biol. 1, 1–9. doi: 10.1038/s42003-018-0086-7
Comeau A. M., Li W. K. W., Tremblay J-É, Carmack E. C., Lovejoy C. (2011). Arctic Ocean microbial community structure before and after the 2007 record Sea ice minimum. PloS One. 6, e27492. doi: 10.1371/journal.pone.0027492
Connolly C. T., Cardenas M. B., Burkart G. A., Spencer R. G. M., McClelland J. W. (2020). Groundwater as a major source of dissolved organic matter to Arctic coastal waters. Nat. Commun. 11, 1479. doi: 10.1038/s41467-020-15250-8
Cotner J. B., Biddanda B. A., Players S. (2002). Large Role: Microbial influence on biogeochemical processes in pelagic aquatic ecosystems. Ecosyst. 5, 105–121. doi: 10.1007/s10021-001-0059-3
Cottrell Matthew T., Kirchman David L. (2009). Photoheterotrophic microbes in the Arctic ocean in summer and winter. Appl. Environ. Microbiol. 75, 4958–4966. doi: 10.1128/AEM.00117-09
Dadaglio L., Dinasquet J., Obernosterer I., Joux F. (2018). Differential responses of bacteria to diatom-derived dissolved organic matter in the Arctic ocean. Aquat. Microb. Ecol. 82, 59–72. doi: 10.3354/ame01883
DeFrancesco C., Guéguen C. (2021). Long-term trends in dissolved organic matter composition and its relation to Sea ice in the Canada basin, Arctic ocean (2007–2017). J. Geophys. Res. Oceans. 126, e2020JC016578. doi: 10.1029/2020JC016578
Doherty S. J., Warren S. G., Grenfell T. C., Clarke A. D., Brandt R. E. (2010). Light-absorbing impurities in Arctic snow. Atmos. Chem. Phys. 10, 11647–11680. doi: 10.5194/acp-10-11647-2010
Ducklow H. W., Quinby H. L., Carlson C. A. (1995). Bacterioplankton dynamics in the equatorial pacific during the 1992 El niño. Deep Sea Res. Part II Top. Stud. Oceanogr. 42, 621–638. doi: 10.1016/0967-0645(95)00022-I
Dyda R. Y., Suzuki M. T., Yoshinaga M. Y., Rodger Harvey H. (2009). The response of microbial communities to diverse organic matter sources in the Arctic ocean. Deep Sea Res. Part II Top. Stud. Oceanogr. 56, 1249–1263. doi: 10.1016/j.dsr2.2008.10.019
Elifantz H., Dittell A. I., Cottrell M. T., Kirchman D. L. (2007). Dissolved organic matter assimilation by heterotrophic bacterial groups in the western Arctic ocean. Aquat. Microb. Ecol. 50, 39–49. doi: 10.3354/ame01145
Emerson S., Hedges J. (2008). Chemical oceanography and the marine carbon cycle (Cambridge, New York: Cambridge University Press).
Fadeev E., Salter I., Schourup-Kristensen V., Nöthig E.-M., Metfies K., Engel A., et al. (2018). Microbial communities in the East and West fram strait during Sea ice melting season. Front. Mar. Sci. 5. doi: 10.3389/fmars.2018.00429
Fang Z., Yang W., Chen M., Zheng M., Hu W. (2016). Abundance and sinking of particulate black carbon in the western Arctic and subarctic oceans. Sci. Rep. 6, 29959. doi: 10.1038/srep29959
Fang Z., Yang W., Stubbins A., Chen M., Li J., Jia R., et al. (2021). Spatial characteristics and removal of dissolved black carbon in the western Arctic ocean and Bering Sea. Geochim. Cosmochim. Acta 304, 178–190. doi: 10.1016/j.gca.2021.04.024
Fouché J., Christiansen C. T., Lafrenière M. J., Grogan P., Lamoureux S. F. (2020). Canadian Permafrost stores large pools of ammonium and optically distinct dissolved organic matter. Nat. Commun. 11, 4500. doi: 10.1038/s41467-020-18331-w
Fouilland E., Gosselin M., Rivkin R. B., Vasseur C., Mostajir B. (2007). Nitrogen uptake by heterotrophic bacteria and phytoplankton in Arctic surface waters. J. Plankton Res. 29, 369–376. doi: 10.1093/plankt/fbm022
Galand P. E., Lovejoy C., Pouliot J., M-è G., Vincent W. F. (2008). Microbial community diversity and heterotrophic production in a coastal Arctic ecosystem: A stamukhi lake and its source waters. Limnol. Oceanogr. 53, 813–823. doi: 10.4319/lo.2008.53.2.0813
Garneau M-È, Roy S., Lovejoy C., Gratton Y., Vincent W. F. (2008). Seasonal dynamics of bacterial biomass and production in a coastal arctic ecosystem: Franklin bay, western Canadian Arctic. J. Geophys. Res. Oceans. 113, C07S91. doi: 10.1029/2007JC004281
Garneau M-È, Vincent W. F., Alonso-Sáez L., Gratton Y., Lovejoy C. (2006). Prokaryotic community structure and heterotrophic production in a river-influenced coastal arctic ecosystem. Aquat. Microb. Ecol. 42, 27–40. doi: 10.3354/ame042027
Giannelli V., Thomas D. N., Haas C., Kattner G., Kennedy H., Dieckmann G. S. (2001). Behaviour of dissolved organic matter and inorganic nutrients during experimental sea-ice formation. Ann. Glaciol. 33, 317–321. doi: 10.3189/172756401781818572
Giovannoni Stephen J., Tripp H. J., Givan S., Podar M., Vergin Kevin L., Baptista D., et al. (2005). Genome streamlining in a cosmopolitan oceanic bacterium. Science 309, 1242–1245. doi: 10.1126/science.1114057
Gosselin M., Levasseur M., Wheeler P. A., Horner R. A., Booth B. C. (1997). New measurements of phytoplankton and ice algal production in the Arctic ocean. Deep Sea Res. Part II Top. Stud. Oceanogr. 44, 1623–1644. doi: 10.1016/S0967-0645(97)00054-4
Grosse G., Goetz S., McGuire A. D., Romanovsky V. E., Schuur E. A. G. (2016). Changing permafrost in a warming world and feedbacks to the earth system. Environ. Res. Lett. 11, 40201. doi: 10.1088/1748-9326/11/4/040201
Grunert B. K., Tzortziou M., Neale P., Menendez A., Hernes P. (2021). DOM degradation by light and microbes along the Yukon river-coastal ocean continuum. Sci. Rep. 11, 10236. doi: 10.1038/s41598-021-89327-9
Hall J. V., Loboda T. V. (2017). Quantifying the potential for low-level transport of black carbon emissions from cropland burning in Russia to the snow-covered Arctic. Front. Earth Sci. 5. doi: 10.3389/feart.2017.00109
Han D., Ha H. K., Hwang C. Y., Lee B. Y., Hur H.-G., Lee Y. K. (2015). Bacterial communities along stratified water columns at the chukchi borderland in the western Arctic ocean. Deep Sea Res. Part II Top. Stud. Oceanogr 120, 52–60. doi: 10.1016/j.dsr2.2015.01.018
Han D., Kang I., Ha H. K., Kim H. C., Kim O.-S., Lee B. Y., et al. (2014). Bacterial communities of surface mixed layer in the pacific sector of the Western Arctic ocean during Sea-ice melting. PloS One. 9, e86887. doi: 10.1371/journal.pone.0086887
Hansell D. A. (2013). Recalcitrant dissolved organic carbon fractions. Ann. Rev. Mar. Sci. 5, 421–445. doi: 10.1146/annurev-marine-120710-100757
Hansell D. A., Carlson C. A. (2014). Biogeochemistry of marine dissolved organic matter (London, UK: Academic Press).
Hernes P. J., Benner R. (2006). Terrigenous organic matter sources and reactivity in the north Atlantic ocean and a comparison to the Arctic and pacific oceans. Mar. Chem. 100, 66–79. doi: 10.1016/j.marchem.2005.11.003
Heslop J. K., Winkel M., Walter Anthony K. M., Spencer R. G. M., Podgorski D. C., Zito P., et al. (2019). Increasing organic carbon biolability with depth in yedoma permafrost: Ramifications for future climate change. J. Geophys. Res. Biogeosci 124, 2021–2038. doi: 10.1029/2018JG004712
He P., Xie L., Zhang X., Li J., Lin X., Pu X., et al. (2020). Microbial diversity and metabolic potential in the stratified sansha yongle blue hole in the south China Sea. Sci. Rep. 10, 5949. doi: 10.1038/s41598-020-62411-2
He J., Zhang F., Lin L., Ma Y., Chen J. (2012). Bacterioplankton and picophytoplankton abundance, biomass, and distribution in the Western Canada basin during summer 2008. Deep Sea Res. Part II Top. Stud. Oceanogr. 81-84, 36–45. doi: 10.1016/j.dsr2.2012.08.018
Holmes R. M., McClelland J. W., Raymond P. A., Frazer B. B., Peterson B. J., Stieglitz M. (2008). Lability of DOC transported by alaskan rivers to the Arctic ocean. Geophys. Res. Lett. 35, L03402. doi: 10.1029/2007GL032837
Hood E., Battin T. J., Fellman J., O'Neel S., Spencer R. G. M. (2015). Storage and release of organic carbon from glaciers and ice sheets, nat. Geosci 8, 91–96. doi: 10.1038/ngeo2331
Hood E., Fellman J., Spencer R. G. M., Hernes P. J., Edwards R., D’Amore D., et al. (2009). Glaciers as a source of ancient and labile organic matter to the marine environment. Nature 462, 1044–1047. doi: 10.1038/nature08580
Hop H., Wiencke C. (2019). The ecosystem of kongsfjorden, Svalbard. Eds. Hop H., Wiencke C. (Cham: Springer International Publishing), 1–20.
Howard-Jones M. H., Ballard V. D., Allen A. E., Frischer M. E., Verity P. G. (2002). Distribution of bacterial biomass and activity in the marginal ice zone of the central barents Sea during summer. J. Mar. Syst. 38, 77–91. doi: 10.1016/S0924-7963(02)00170-7
Huston A. L., Deming J. W. (2002). Relationships between microbial extracellular enzymatic activity and suspended and sinking particulate organic matter: seasonal transformations in the north water. Deep Sea Res. Part II Top. Stud. Oceanogr 49, 5211–5225. doi: 10.1016/S0967-0645(02)00186-8
Ingeborg B. (1999). Bacterial utilization of humic substances from the Arctic ocean. Aquat. Microb. Ecol. 19, 37–45. doi: 10.3354/ame019037
Jørgensen L., Lechtenfeld O. J., Benner R., Middelboe M., Stedmon C. A. (2014). Production and transformation of dissolved neutral sugars and amino acids by bacteria in seawater. Biogeosciences. 11, 5349–5363. doi: 10.5194/bg-11-5349-2014
Jørgensen L., Stedmon C. A., Granskog M. A., Middelboe M. (2014). Tracing the long-term microbial production of recalcitrant fluorescent dissolved organic matter in seawater. Geophys. Res. Lett. 41, 2481–2488. doi: 10.1002/2014GL059428
Jaffé R., Ding Y., Niggemann J., Vähätalo Anssi V., Stubbins A., Spencer Robert G. M., et al. (2013). Global charcoal mobilization from soils via dissolution and riverine transport to the oceans. Science 340, 345–347. doi: 10.1126/science.1231476
Jain A., Krishnan K. P. (2017). A glimpse of the diversity of complex polysaccharide-degrading culturable bacteria from kongsfjorden, Arctic ocean. Ann. Microbiol. 67, 203–214. doi: 10.1007/s13213-016-1252-0
Jiao N., Herndl G. J., Hansell D. A., Benner R., Kattner G., Wilhelm S. W., et al. (2010). Microbial production of recalcitrant dissolved organic matter: Long-term carbon storage in the global ocean. Nat. Rev. Microbiol. 8, 593–599. doi: 10.1038/nrmicro2386
Jones E. P., Anderson L. G., Jutterström S., Mintrop L., Swift J. H. (2008). Pacific freshwater, river water and sea ice meltwater across Arctic ocean basins: Results from the 2005 beringia expedition. J. Geophys. Res. Oceans. 113, C08012. doi: 10.1029/2007JC004124
Jones M. W., Coppola A. I., Santín C., Dittmar T., Jaffé R., Doerr S. H., et al. (2020). Fires prime terrestrial organic carbon for riverine export to the global oceans. Nat. Commun. 11, 2791. doi: 10.1038/s41467-020-16576-z
Jorgensen L., Stedmon C. A., Kaartokallio H., Middelboe M., Thomas D. N. (2015). Changes in the composition and bioavailability of dissolved organic matter during sea ice formation. Limnol. Oceanogr 60, 817–830. doi: 10.1002/lno.10058
Jung J., Son J. E., Lee Y. K., Cho K.-H., Lee Y., Yang E. J., et al. (2021). Tracing riverine dissolved organic carbon and its transport to the halocline layer in the chukchi Sea (western Arctic ocean) using humic-like fluorescence fingerprinting. Sci. Total Environ. 772, 145542. doi: 10.1016/j.scitotenv.2021.145542
Kaplan J. O., New M. (2006). Arctic Climate change with a 2°C global warming: Timing, climate patterns and vegetation change. Clim. Change 79, 213–241. doi: 10.1007/s10584-006-9113-7
Kellerman A. M., Guillemette F., Podgorski D. C., Aiken G. R., Butler K. D., Spencer R. G. M. (2018). Unifying concepts linking dissolved organic matter composition to persistence in aquatic ecosystems. Environ. Sci. Technol. 52, 2538–2548. doi: 10.1021/acs.est.7b05513
Kellerman A. M., Vonk J., McColaugh S., Podgorski D. C., van Winden E., Hawkings J. R., et al. (2021). Molecular signatures of glacial dissolved organic matter from Svalbard and Greenland. Glob. Biogeochem. Cycles 35, e2020GB006709. doi: 10.1029/2020GB006709
Kellogg C. T. E., Deming J. W. (2009). Comparison of free-living, suspended particle, and aggregate-associated bacterial and archaeal communities in the laptev Sea. Aquat. Microb. Ecol. 57, 1–18. doi: 10.3354/ame01317
Khan A. L., Wagner S., Jaffe R., Xian P., Williams M., Armstrong R., et al. (2017). Dissolved black carbon in the global cryosphere: Concentrations and chemical signatures. Geophys. Res. Lett. 44, 6226–6234. doi: 10.1002/2017GL073485
Kim S., Kaplan L. A., Benner R., Hatcher P. G. (2004). Hydrogen-deficient molecules in natural riverine water samples–evidence for the existence of black carbon in DOM. Mar. Chem. 92, 225–234. doi: 10.1016/j.marchem.2004.06.042
Kirchman D. L., Elifantz H., Dittel A. I., Malmstrom R. R., Cottrell M. T. (2007). Standing stocks and activity of archaea and bacteria in the western Arctic ocean. Limnol. Oceanogr 52, 495–507. doi: 10.4319/lo.2007.52.2.0495
Kirchman D. L., Hill V., Cottrell M. T., Gradinger R., Malmstrom R. R., Parker A. (2009). Standing stocks, production, and respiration of phytoplankton and heterotrophic bacteria in the western Arctic ocean. Deep Sea Res. Part II Top. Stud. Oceanogr 56, 1237–1248. doi: 10.1016/j.dsr2.2008.10.018
Kirchman D. L., Keel R. G., Simon M., Welschmeyer N. A. (1993). Biomass and production of heterotrophic bacterioplankton in the oceanic subarctic pacific. Deep Sea Res. Part I Oceanogr. Res. Pap 40, 967–988. doi: 10.1016/0967-0637(93)90084-G
Kirchman D. L., Malmstrom R. R., Cottrell M. T. (2005). Control of bacterial growth by temperature and organic matter in the Western Arctic. Deep Sea Res. Part II Top. Stud. Oceanogr 52, 3386–3395. doi: 10.1016/j.dsr2.2005.09.005
Kirchman D. L., Morán X. A. G., Ducklow H. (2009). Microbial growth in the polar oceans — role of temperature and potential impact of climate change. Nat. Rev. Microbiol. 7, 451–459. doi: 10.1038/nrmicro2115
Kopylov A. I., Kosolapov D. B., Zabotkina E. A., Romanenko A. V., Sazhin A. F. (2021). Distribution and relationship between heterotrophic organisms and viruses on the East Siberian Sea shelf. Oceanology 61, 220–232. doi: 10.1134/S0001437021020089
Kopylov A. I., Sazhin A. F., Zabotkina E. A., Romanova N. D. (2015). Virioplankton in the kara Sea: The impact of viruses on mortality of heterotrophic bacteria. Oceanology 55, 561–572. doi: 10.1134/S0001437015040104
Lønborg C., Álvarez-Salgado X. A., Davidson K., Martínez-García S., Teira E. (2010). Assessing the microbial bioavailability and degradation rate constants of dissolved organic matter by fluorescence spectroscopy in the coastal upwelling system of the ría de vigo. Mar. Chem. 119, 121–129. doi: 10.1016/j.marchem.2010.02.001
Lancelot C. (1984). Extracellular release of small and large molecules by phytoplankton in the southern bight of the north Sea. Estuar. Coast. Shelf Sci. 18, 65–77. doi: 10.1016/0272-7714(84)90007-6
Lawson E. C., Bhatia M. P., Wadham J. L., Kujawinski E. B. (2014). Continuous summer export of nitrogen-rich organic matter from the Greenland ice sheet inferred by ultrahigh resolution mass spectrometry. Environ. Sci. Technol. 48, 14248–14257. doi: 10.1021/es501732h
Lawson E. C., Wadham J. L., Tranter M., Stibal M., Lis G. P., Butler C. E. H., et al. (2014). Greenland Ice sheet exports labile organic carbon to the Arctic oceans. Biogeosciences. 11, 4015–4028. doi: 10.5194/bg-11-4015-2014
Lechtenfeld O. J., Kattner G., Flerus R., McCallister S. L., Schmitt-Kopplin P., Koch B. P. (2014). Molecular transformation and degradation of refractory dissolved organic matter in the Atlantic and southern ocean. Geochim. Cosmochim. Acta. 126, 321–337. doi: 10.1016/j.gca.2013.11.009
Lee J., Kang S.-H., Yang E. J., Macdonald A. M., Joo H. M., Park J., et al. (2019). Latitudinal distributions and controls of bacterial community composition during the summer of 2017 in Western Arctic surface waters (from the Bering strait to the chukchi borderland). Sci. Rep. 9, 16822. doi: 10.1038/s41598-019-53427-4
Legendre L., Ackley S. F., Dieckmann G. S., Gulliksen B., Horner R., Hoshiai T., et al. (1992). Ecology of sea ice biota. Polar Biol. 12, 429–444. doi: 10.1007/BF00243114
Lewis K. M., van Dijken G. L., Arrigo K. R. (2020). Changes in phytoplankton concentration now drive increased Arctic ocean primary production. Science. 369, 198–202. doi: 10.1126/science.aay8380
Liao L., Sun X., Yu Y., Chen B. (2015). Draft genome of marinomonas sp. BSi20584 from Arctic sea ice. Mar. Genomics. 23, 23–25. doi: 10.1016/j.margen.2015.03.013
Lind S., Ingvaldsen R. B., Furevik T. (2018). Arctic Warming hotspot in the northern barents Sea linked to declining sea-ice import. Nat. Clim. Change 8, 634–639. doi: 10.1038/s41558-018-0205-y
Lin Y.-S., Koch B. P., Feseker T., Ziervogel K., Goldhammer T., Schmidt F., et al. (2017). Near-surface heating of young rift sediment causes mass production and discharge of reactive dissolved organic matter. Sci. Rep. 7, 44864. doi: 10.1038/srep44864
Lund Paulsen M., Müller O., Larsen A., Møller E. F., Middelboe M., Sejr M. K., et al. (2019). Biological transformation of Arctic dissolved organic matter in a NE Greenland fjord. Limnol. Oceanogr 64, 1014–1033. doi: 10.1002/lno.11091
MacDonald E. N., Tank S. E., Kokelj S. V., Froese D. G., Hutchins R. H. S. (2021). Permafrost-derived dissolved organic matter composition varies across permafrost end-members in the western Canadian Arctic. Environ. Res. Lett. 16, 024036. doi: 10.1088/1748-9326/abd971
Mann P. J., Davydova A., Zimov N., Spencer R. G. M., Davydov S., Bulygina E., et al. (2012). Controls on the composition and lability of dissolved organic matter in siberia's kolyma river basin. J. Geophys. Res. Biogeosci. 117, G01028. doi: 10.1029/2011JG001798
Maranger R., Vaqué D., Nguyen D., Hébert M.-P., Lara E. (2015). Pan-Arctic patterns of planktonic heterotrophic microbial abundance and processes: Controlling factors and potential impacts of warming. Prog. Oceanogr. 139, 221–232. doi: 10.1016/j.pocean.2015.07.006
Methé B. A., Nelson K. E., Deming J. W., Momen B., Melamud E., Zhang X., et al. (2005). The psychrophilic lifestyle as revealed by the genome sequence of <em<Colwellia psychrerythraea</em< 34H through genomic and proteomic analyses. Proc. Natl. Acad. Sci. United States America. 102, 10913. doi: 10.1073/pnas.0504766102
Monteith D. T., Stoddard J. L., Evans C. D., de Wit H. A., Forsius M., Høgåsen T., et al. (2007). Dissolved organic carbon trends resulting from changes in atmospheric deposition chemistry. Nature 450, 537–540. doi: 10.1038/nature06316
Mori T., Kondo Y., Ohata S., Zhao Y., Sinha P. R., Oshima N., et al. (2020). Seasonal variation of wet deposition of black carbon in Arctic Alaska. J. Geophys. Res. Atmos. 125, e2019JD032240. doi: 10.1029/2019JD032240
Müller O., Seuthe L., Bratbak G., Paulsen M. L. (2018). Bacterial response to permafrost derived organic matter input in an Arctic fjord. Front. Mar. Sci. 5. doi: 10.3389/fmars.2018.00263
Muller S., Vahatalo A. V., Stedmon C. A., Granskog M. A., Norman L., Aslam S. N., et al. (2013). Selective incorporation of dissolved organic matter (DOM) during sea ice formation. Mar. Chem. 155, 148–157. doi: 10.1016/j.marchem.2013.06.008
Mundy C. J., Gosselin M., Ehn J., Gratton Y., Rossnagel A., Barber D. G., et al. (2009). Contribution of under-ice primary production to an ice-edge upwelling phytoplankton bloom in the Canadian Beaufort Sea. Geophys. Res. Lett. 36, L17601. doi: 10.1029/2009GL038837
Murray A. E., Grzymski J. J. (2007). Diversity and genomics of Antarctic marine micro-organisms. Philos. Trans. R. Soc Lond. B Biol. Sci. 362, 2259–2271. doi: 10.1098/rstb.2006.1944
Musilova M., Tranter M., Wadham J., Telling J., Tedstone A., Anesio Alexandre M. (2017). Microbially driven export of labile organic carbon from the Greenland ice sheet. Nat. Geosci 10, 360–365. doi: 10.1038/ngeo2920
Naganuma T., Kimura H., Karimoto R., Pimenov N. V. (2006). Abundance of planktonic thraustochytrids and bacteria and the concentration of particulate ATP in the Greenland and Norwegian seas. Polar Biosci. 20, 37–45 doi: 10.15094/00006257
Nakane M., Ajioka T., Yamashita Y. (2017). Distribution and sources of dissolved black carbon in surface waters of the chukchi Sea, Bering Sea, and the north pacific ocean. Front. Earth Sci. 5. doi: 10.3389/feart.2017.00034
Newton R. J., Griffin L. E., Bowles K. M., Meile C., Gifford S., Givens C. E., et al. (2010). Genome characteristics of a generalist marine bacterial lineage. ISME J. 4, 784–798. doi: 10.1038/ismej.2009.150
Nguyen D., Maranger R., Balagué V., Coll-Lladó M., Lovejoy C., Pedrós-Alió C. (2015). Winter diversity and expression of proteorhodopsin genes in a polar ocean. ISME J. 9, 1835–1845. doi: 10.1038/ismej.2015.1
Niemi A., Meisterhans G., Michel C. (2014). Response of under-ice prokaryotes to experimental sea-ice DOM enrichment. Aquat. Microb. Ecol. 73, 17–28. doi: 10.3354/ame01706
Nikrad M. P., Cottrell M. T., Kirchman D. L. (2012). Abundance and single-cell activity of heterotrophic bacterial groups in the Western Arctic ocean in summer and winter. Appl. Environ. Microbiol. 78, 2402–2409. doi: 10.1128/AEM.07130-11
O'Donnell J. A., Aiken G. R., Swanson D. K., Panda S., Butler K. D., Baltensperger A. P. (2016). Dissolved organic matter composition of Arctic rivers: Linking permafrost and parent material to riverine carbon. Glob. Biogeochem. Cycles 30, 1811–1826. doi: 10.1002/2016GB005482
O'Donnell J. A., Aiken G. R., Walvoord M. A., Butler K. D. (2012). Dissolved organic matter composition of winter flow in the Yukon river basin: Implications of permafrost thaw and increased groundwater discharge. Glob. Biogeochem. Cycles 26, GB0E06. doi: 10.1029/2012GB004341
Oakes J. M., Rysgaard S., Glud R. N., Eyre B. D. (2016). The transformation and fate of sub-Arctic microphytobenthos carbon revealed through 13C-labeling. Limnol. Oceanogr 61, 2296–2308. doi: 10.1002/lno.10377
Obu J., Westermann S., Bartsch A., Berdnikov N., Christiansen H. H., Dashtseren A., et al. (2019). Northern hemisphere permafrost map based on TTOP modelling for 2000–2016 at 1 km2 scale. Earth-Sci. Rev. 193, 299–316. doi: 10.1016/j.earscirev.2019.04.023
Opsahl S., Benner R., Amon R. M. W. (1999). Major flux of terrigenous dissolved organic matter through the Arctic ocean. Limnol. Oceanogr 44, 2017–2023. doi: 10.4319/lo.1999.44.8.2017
Osburn C. L., Kinsey J. D., Bianchi T. S., Shields M. R. (2019). Formation of planktonic chromophoric dissolved organic matter in the ocean. Mar. Chem. 209, 1–13. doi: 10.1016/j.marchem.2018.11.010
Osterholz H., Dittmar T., Niggemann J. (2014). Molecular evidence for rapid dissolved organic matter turnover in Arctic fjords. Mar. Chem. 160, 1–10. doi: 10.1016/j.marchem.2014.01.002
Overduin P. P., Liebner S., Knoblauch C., Günther F., Wetterich S., Schirrmeister L., et al. (2015). Methane oxidation following submarine permafrost degradation: Measurements from a central laptev Sea shelf borehole. J. Geophys. Res. Biogeosci 120, 965–978. doi: 10.1002/2014JG002862
Pain A. J., Martin J. B., Martin E. E., Rahman S., Ackermann P. (2020). Differences in the quantity and quality of organic matter exported from greenlandic glacial and deglaciated watersheds. Glob. Biogeochem. Cycles. 34, e2020GB006614. doi: 10.1029/2020GB006614
Paulsen M. L., Seuthe L., Reigstad M., Larsen A., Cape M. R., Vernet M. (2018). Asynchronous accumulation of organic carbon and nitrogen in the Atlantic gateway to the Arctic ocean. Front. Mar. Sci. 5, 416. doi: 10.3389/fmars.2018.00416
Peterson Bruce J., Holmes Robert M., McClelland James W., Vörösmarty Charles J., Lammers Richard B., Shiklomanov Alexander I., et al. (2002). Increasing river discharge to the Arctic ocean. Science 298, 2171–2173. doi: 10.1126/science.1077445
Petrich C., Eicken H. (2010). Growth, structure and properties of sea ice. Sea ice (Oxford, United Kingdom: Blackwell Publishing Ltd) 2, 23–77. doi: 10.1002/9781444317145.ch2
Piontek J., Galgani L., Nöthig E.-M., Peeken I., Engel A. (2021). Organic matter composition and heterotrophic bacterial activity at declining summer sea ice in the central Arctic ocean. Limnol. Oceanogr. 66, S343–SS62. doi: 10.1002/lno.11639
Piontek J., Sperling M., Nöthig E.-M., Engel A. (2014). Regulation of bacterioplankton activity in fram strait (Arctic ocean) during early summer: The role of organic matter supply and temperature. J. Mar. Syst. 132, 83–94. doi: 10.1016/j.jmarsys.2014.01.003
Pohlman J. W., Bauer J. E., Waite W. F., Osburn C. L., Chapman N. R. (2011). Methane hydrate-bearing seeps as a source of aged dissolved organic carbon to the oceans. Nat. Geosci. 4, 37–41. doi: 10.1038/ngeo1016
Poulton A. J., Daniels C. J., Esposito M., Humphreys M. P., Mitchell E., Ribas-Ribas M., et al. (2016). Production of dissolved organic carbon by Arctic plankton communities: Responses to elevated carbon dioxide and the availability of light and nutrients. Deep Sea Res. Part II: Topical Stud. Oceanography. 127, 60–74. doi: 10.1016/j.dsr2.2016.01.002
Pugach S. P., Pipko I. I., Shakhova N. E., Shirshin E. A., Perminova I. V., Gustafsson Ö, et al. (2018). Dissolved organic matter and its optical characteristics in the laptev and East Siberian seas: spatial distribution and interannual variability (2003–2011). Ocean Sci. 14, 87–103. doi: 10.5194/os-14-87-2018
Quadfasel D., Sy A., Wells D., Tunik A. (1991). Warming in the Arctic. Nature 350, 385. doi: 10.1038/350385a0
Ramachandran A., McLatchie S., Walsh D. A. (2021). A novel freshwater to marine evolutionary transition revealed within methylophilaceae bacteria from the Arctic ocean. MBio 12, e01306–e01321. doi: 10.1128/mBio.01306-21
Raymond P. A., McClelland J. W., Holmes R. M., Zhulidov A. V., Mull K., Peterson B. J., et al. (2007). Flux and age of dissolved organic carbon exported to the Arctic ocean: A carbon isotopic study of the five largest arctic rivers. Glob. Biogeochem. Cycles 21, GB4011. doi: 10.1029/2007GB002934
Retelletti Brogi S., Ha S.-Y., Kim K., Derrien M., Lee Y. K., Hur J. (2018). Optical and molecular characterization of dissolved organic matter (DOM) in the Arctic ice core and the underlying seawater (Cambridge bay, canada): Implication for increased autochthonous DOM during ice melting. Sci. Total Environ. 627, 802–811. doi: 10.1016/j.scitotenv.2018.01.251
Retelletti Brogi S., Jung J. Y., Ha S.-Y., Hur J. (2019). Seasonal differences in dissolved organic matter properties and sources in an Arctic fjord: Implications for future conditions. Sci. Total Environ. 694, 133740. doi: 10.1016/j.scitotenv.2019.133740
Rex R. M., Tiffany R. A. S., Matthew T. C., David L. K. (2007). Diversity, abundance, and biomass production of bacterial groups in the western Arctic ocean. Aquat. Microb. Ecol. 47, 45–55. doi: 10.3354/ame047045
Rich J., Gosselin M., Sherr E., Sherr B., Kirchman D. L. (1997). High bacterial production, uptake and concentrations of dissolved organic matter in the central Arctic ocean. Deep Sea Res. Part II Top. Stud. Oceanogr 44, 1645–1663. doi: 10.1016/S0967-0645(97)00058-1
Rocap G., Larimer F. W., Lamerdin J., Malfatti S., Chain P., Ahlgren N. A., et al. (2003). Genome divergence in two prochlorococcus ecotypes reflects oceanic niche differentiation. Nature 424, 1042–1047. doi: 10.1038/nature01947
Romanovskii N. N., Hubberten H. W., Gavrilov A. V., Eliseeva A. A., Tipenko G. S. (2005). Offshore permafrost and gas hydrate stability zone on the shelf of East Siberian seas. Geo-Mar. Lett. 25, 167–182. doi: 10.1007/s00367-004-0198-6
Rossel P. E., Bienhold C., Boetius A., Dittmar T. (2016). Dissolved organic matter in pore water of Arctic ocean sediments: Environmental influence on molecular composition. Org. Geochem 97, 41–52. doi: 10.1016/j.orggeochem.2016.04.003
Rossel P. E., Bienhold C., Hehemann L., Dittmar T., Boetius A. (2020). Molecular composition of dissolved organic matter in sediment porewater of the Arctic deep-Sea observatory HAUSGARTEN (Fram strait). Front. Mar. Sci. 7. doi: 10.3389/fmars.2020.00428
Royo-Llonch M., Sánchez P., Ruiz-González C., Salazar G., Pedrós-Alió C., Sebastián M., et al. (2021). Compendium of 530 metagenome-assembled bacterial and archaeal genomes from the polar Arctic ocean. Nat. Microbiol. 6, 1561–1574. doi: 10.1038/s41564-021-00979-9
Ruppel M. M., Gustafsson Ö, Rose N. L., Pesonen A., Yang H., Weckström J., et al. (2015). Spatial and temporal patterns in black carbon deposition to dated fennoscandian Arctic lake sediments from 1830 to 2010. Environ. Sci. Technol. 49, 13954–13963. doi: 10.1021/acs.est.5b01779
Saba G. K., Steinberg D. K., Bronk D. A. (2011). The relative importance of sloppy feeding, excretion, and fecal pellet leaching in the release of dissolved carbon and nitrogen by acartia tonsa copepods. J. Exp. Mar. Biol. Ecol. 404, 47–56. doi: 10.1016/j.jembe.2011.04.013
Sala M. M., Arrieta J. M., Boras J. A., Duarte C. M., Vaqué D. (2010). The impact of ice melting on bacterioplankton in the Arctic ocean. Polar Biol. 33, 1683–1694. doi: 10.1007/s00300-010-0808-x
Sala M. M., Terrado R., Lovejoy C., Unrein F., Pedrós-Alió C. (2008). Metabolic diversity of heterotrophic bacterioplankton over winter and spring in the coastal Arctic ocean. Environ. Microbiol. 10, 942–949. doi: 10.1111/j.1462-2920.2007.01513.x
Samylina O. S., Rusanov I. I., Tarnovetskii I. Y., Yakushev E. V., Grinko A. A., Zakharova E. E., et al. (2021). On the possibility of aerobic methane production by pelagic microbial communities of the laptev Sea. Microbiology 90, 145–157. doi: 10.1134/S0026261721020119
Sanz-Sáez I., Salazar G., Sánchez P., Lara E., Royo-Llonch M., Sà E. L., et al. (2020). Diversity and distribution of marine heterotrophic bacteria from a large culture collection. BMC Microbiol. 20, 207. doi: 10.1186/s12866-020-01884-7
Savvichev A. S., Kadnikov V. V., Kravchishina M. D., Galkin S. V., Novigatskii A. N., Sigalevich P. A., et al. (2018). Methane as an organic matter source and the trophic basis of a laptev Sea cold seep microbial community. Geomicrobiol. J. 35, 411–423. doi: 10.1080/01490451.2017.1382612
Schuur E. A. G., McGuire A. D., Schädel C., Grosse G., Harden J. W., Hayes D. J., et al. (2015). Climate change and the permafrost carbon feedback. Nature 520, 171–179. doi: 10.1038/nature14338
Semenov P., Portnov A., Krylov A., Egorov A., Vanshtein B. (2020). Geochemical evidence for seabed fluid flow linked to the subsea permafrost outer border in the south kara Sea. Geochemistry 80, 125509. doi: 10.1016/j.chemer.2019.04.005
Serreze M. C., Barry R. G. (2011). Processes and impacts of Arctic amplification: A research synthesis. Glob. Planet. Change 77, 85–96. doi: 10.1016/j.gloplacha.2011.03.004
Sert M. F., Niemann H., Reeves E. P., Granskog M. A., Hand K. P., Kekäläinen T., et al. (2022). Compositions of dissolved organic matter in the ice-covered waters above the aurora hydrothermal vent system, gakkel ridge, Arctic ocean. Biogeosci. Discuss 2022, 1–35. doi: 10.5194/bg-2021-350
Seuthe L., Töpper B., Reigstad M., Thyrhaug R., Vaquer-Sunyer R. (2011). Microbial communities and processes in ice-covered Arctic waters of the northwestern fram strait (75 to 80 n) during the vernal pre-bloom phase. Aquat. Microb. Ecol. 64, 253–266. doi: 10.3354/ame01525
Shakhova N., Semiletov I., Gustafsson O., Sergienko V., Lobkovsky L., Dudarev O., et al. (2017). Current rates and mechanisms of subsea permafrost degradation in the East Siberian Arctic shelf. Nat. Commun. 8, 15872. doi: 10.1038/ncomms15872
Shakhova N., Semiletov I., Salyuk A., Yusupov V., Kosmach D., Gustafsson Ö (2010). Extensive methane venting to the atmosphere from sediments of the East Siberian Arctic shelf. Science 327, 1246–1250. doi: 10.1126/science.1182221
Sherr B. F., Sherr E. B. (2003). Community respiration/production and bacterial activity in the upper water column of the central Arctic ocean. Deep Sea Res. Part I Oceanogr. Res. Pap 50, 529–542. doi: 10.1016/S0967-0637(03)00030-X
Sherr E. B., Sherr B. F., Wheeler P. A., Thompson K. (2003). Temporal and spatial variation in stocks of autotrophic and heterotrophic microbes in the upper water column of the central Arctic ocean. Deep Sea Res. Part I Oceanogr. Res. Pap 50, 557–571. doi: 10.1016/S0967-0637(03)00031-1
Shiklomanov A., Déry S., Tretiakov M., Yang D., Magritsky D., Georgiadi A., et al. (2021). Arctic Hydrology (Permafrost and Ecosystems: Springer), 703–738.
Shindell D. T., Chin M., Dentener F., Doherty R. M., Faluvegi G., Fiore A. M., et al. (2008). A multi-model assessment of pollution transport to the Arctic. Atmos. Chem. Phys. 8, 5353–5372. doi: 10.5194/acp-8-5353-2008
Sinha R. K., Krishnan K. P., Kerkar S., David T. D. (2017). Spatio-temporal monitoring and ecological significance of retrievable pelagic heterotrophic bacteria in kongsfjorden, an Arctic fjord. Indian J. OF Microbiol. 57, 116–120. doi: 10.1007/s12088-016-0621-5
Sipler R. E., Kellogg C. T. E., Connelly T. L., Roberts Q. N., Yager P. L., Bronk D. A. (2017). Microbial community response to terrestrially derived dissolved organic matter in the coastal Arctic. Front. Microbiol. 8. doi: 10.3389/fmicb.2017.01018
Slater A. G., Lawrence D. M. (2013). Diagnosing present and future permafrost from climate models. J. Clim 26, 5608–5623. doi: 10.1175/JCLI-D-12-00341.1
Smith L. C., Pavelsky T. M., MacDonald G. M., Shiklomanov A. I., Lammers R. B. (2007). Rising minimum daily flows in northern Eurasian rivers: A growing influence of groundwater in the high-latitude hydrologic cycle. J. Geophys. Res. Biogeosci 112, G04S47. doi: 10.1029/2006JG000327
Spencer R. G. M., Mann P. J., Dittmar T., Eglinton T. I., McIntyre C., Holmes R. M., et al. (2015). Detecting the signature of permafrost thaw in Arctic rivers. Geophys. Res. Lett. 42, 2830–2835. doi: 10.1002/2015GL063498
Stedmon C. A., Amon R. M. W., Bauch D., Bracher A., Gonçalves-Araujo R., Hoppmann M., et al. (2021). Insights into water mass origins in the central Arctic ocean from In-situ dissolved organic matter fluorescence. J. Geophys. Res. Oceans 126, e2021JC017407. doi: 10.1029/2021JC017407
Stedmon C. A., Amon R. M. W., Rinehart A. J., Walker S. A. (2011). The supply and characteristics of colored dissolved organic matter (CDOM) in the Arctic ocean: Pan Arctic trends and differences. Mar. Chem. 124, 108–118. doi: 10.1016/j.marchem.2010.12.007
Stedmon C. A., Thomas D. N., Granskog M., Kaartokallio H., Papadimitriou S., Kuosa H. (2007). Characteristics of dissolved organic matter in Baltic coastal Sea ice: Allochthonous or autochthonous origins? Environ. Sci. Technol. 41, 7273–7279. doi: 10.1021/es071210f
Steinbach J., Holmstrand H., Shcherbakova K., Kosmach D., Brüchert V., Shakhova N., et al. (2021). Source apportionment of methane escaping the subsea permafrost system in the outer Eurasian Arctic shelf. Proc. Natl. Acad. Sci. 118, e2019672118. doi: 10.1073/pnas.2019672118
Stein R., Macdonald R. W., Stein R., MacDonald R. W. (2004). The organic carbon cycle in the Arctic ocean (Berlin Heidelberg: Springer-Verlag).
Steward G. F., Smith D. C., Azam F. (1996). Abundance and production of bacteria and viruses in the Bering and chukchi seas. Mar. Ecol. Prog. Ser. 131, 287–300. doi: 10.3354/meps131287
Strauss J., Schirrmeister L., Grosse G., Fortier D., Hugelius G., Knoblauch C., et al. (2017). Deep yedoma permafrost: A synthesis of depositional characteristics and carbon vulnerability. Earth-Sci. Rev. 172, 75–86. doi: 10.1016/j.earscirev.2017.07.007
Sturluson M., Gissel Nielsen T., Wassmann P. (2008). Bacterial abundance, biomass and production during spring blooms in the northern barents Sea. Deep Sea Res. Part II Top. Stud. Oceanogr 55, 2186–2198. doi: 10.1016/j.dsr2.2008.05.001
Tanaka K., Takesue N., Nishioka J., Kondo Y., Ooki A., Kuma K., et al. (2016). The conservative behavior of dissolved organic carbon in surface waters of the southern chukchi Sea, Arctic ocean, during early summer. Sci. Rep. 6, 34123. doi: 10.1038/srep34123
Tarnocai C., Canadell J., Schuur E. A., Kuhry P., Mazhitova G., Zimov S. (2009). Soil organic carbon pools in the northern circumpolar permafrost region. Glob. Biogeochem. Cycles 23, GB2023. doi: 10.1029/2008GB003327
Thomas F. A., Sinha R. K., Krishnan K. P. (2020). Bacterial community structure of a glacio-marine system in the Arctic (Ny-Ålesund, Svalbard). Sci. Total Environ. 718, 135264. doi: 10.1016/j.scitotenv.2019.135264
Thornton D. C. O. (2014). Dissolved organic matter (DOM) release by phytoplankton in the contemporary and future ocean. Eur. J. Phycol. 49, 20–46. doi: 10.1080/09670262.2013.875596
Tisserand L., Dadaglio L., Intertaglia L., Catala P., Panagiotopoulos C., Obernosterer I., et al. (2020). Use of organic exudates from two polar diatoms by bacterial isolates from the Arctic ocean. Philos. Trans. R. Soc A 378, 20190356. doi: 10.1098/rsta.2019.0356
Turetsky M. R., Abbott B. W., Jones M. C., Anthony K. W., Olefeldt D., Schuur E. A. G., et al. (2020). Carbon release through abrupt permafrost thaw. Nat. Geosci 13, 138–143. doi: 10.1038/s41561-019-0526-0
Uchimiya M., Motegi C., Nishino S., Kawaguchi Y., Inoue J., Ogawa H., et al. (2016). Coupled response of bacterial production to a wind-induced fall phytoplankton bloom and sediment resuspension in the chukchi Sea shelf, Western Arctic ocean. Front. Mar. Sci. 3. doi: 10.3389/fmars.2016.00231
Underwood G. J. C., Aslam S. N., Michel C., Niemi A., Norman L., Meiners K. M., et al. (2013). Broad-scale predictability of carbohydrates and exopolymers in Antarctic and Arctic sea ice. Proc. Natl. Acad. Sci. 110, 15734. doi: 10.1073/pnas.1302870110
Underwood G. J. C., Michel C., Meisterhans G., Niemi A., Belzile C., Witt M., et al. (2019). Organic matter from Arctic sea-ice loss alters bacterial community structure and function. Nat. Clim. Change 9, 170–176. doi: 10.1038/s41558-018-0391-7
Urban-Rich J., McCarty J. T., Fernández D., Acuña J. L. (2006). Larvaceans and copepods excrete fluorescent dissolved organic matter (FDOM). J. Exp. Mar. Biol. Ecol. 332, 96–105. doi: 10.1016/j.jembe.2005.11.023
Vallières C., Retamal L., Ramlal P., Osburn C. L., Vincent W. F. (2008). Bacterial production and microbial food web structure in a large arctic river and the coastal Arctic ocean. J. Mar. Syst. 74, 756–773. doi: 10.1016/j.jmarsys.2007.12.002
Vaughan D., Comiso J., Allison I., Carrasco J., Kaser G., Kwok R., et al. (2013). 'Climate Change 20134 - The Physical Science Basis: Chapter 4 - Observations: Cryosphere. (Cambridge, New York: Cambridge University Press) 317–382. doi: 10.1017/CBO9781107415324
Vernet M., Matrai P. A., Andreassen I. (1998). Synthesis of particulate and extracellular carbon by phytoplankton at the marginal ice zone in the barents Sea. J. Geophys. Res. Oceans 103, 1023–1037. doi: 10.1029/97JC02288
Vetrov A., Romankevich E. (2019). Distribution and fluxes of dissolved organic carbon in the Arctic ocean. Polar Res. 38, 3500. doi: 10.33265/polar.v38.3500
von Jackowski A., Grosse J., Nöthig E.-M., Engel A. (2020). Dynamics of organic matter and bacterial activity in the fram strait during summer and autumn. Philos. Trans. R. Soc A 378, 20190366. doi: 10.1098/rsta.2019.0366
Vonk J. E., Mann P. J., Davydov S., Davydova A., Spencer R. G. M., Schade J., et al. (2013). High biolability of ancient permafrost carbon upon thaw. Geophys. Res. Lett. 40, 2689–2693. doi: 10.1002/grl.50348
Vonk J. E., Sánchez-García L., van Dongen B. E., Alling V., Kosmach D., Charkin A., et al. (2012). Activation of old carbon by erosion of coastal and subsea permafrost in Arctic Siberia. Nature 489, 137–140. doi: 10.1038/nature11392
Wadham J. L., Hawkings J. R., Tarasov L., Gregoire L. J., Spencer R. G. M., Gutjahr M., et al. (2019). Ice sheets matter for the global carbon cycle. Nat. Commun. 10, 3567. doi: 10.1038/s41467-019-11394-4
Walker S. A., Amon R. M. W., Stedmon C. A. (2013). Variations in high-latitude riverine fluorescent dissolved organic matter: A comparison of large Arctic rivers. J. Geophys. Res. Biogeosci 118, 1689–1702. doi: 10.1002/2013JG002320
Walvoord M. A., Striegl R. G. (2007). Increased groundwater to stream discharge from permafrost thawing in the Yukon river basin: Potential impacts on lateral export of carbon and nitrogen. Geophys. Res. Lett. 34, L12402. doi: 10.1029/2007GL030216
Wang Q., Jacob D. J., Fisher J. A., Mao J., Leibensperger E. M., Carouge C. C., et al. (2011). Sources of carbonaceous aerosols and deposited black carbon in the Arctic in winter-spring: implications for radiative forcing. Atmos. Chem. Phys. 11, 12453–12473. doi: 10.5194/acp-11-12453-2011
Wang Q., Wekerle C., Wang X., Danilov S., Koldunov N., Sein D., et al. (2020). Intensification of the Atlantic water supply to the Arctic ocean through fram strait induced by Arctic Sea ice decline. Geophys. Res. Lett. 47, e2019GL086682. doi: 10.1029/2019GL086682
Ward C. P., Cory R. M. (2015). Chemical composition of dissolved organic matter draining permafrost soils. Geochim. Cosmochim. Acta 167, 63–79. doi: 10.1016/j.gca.2015.07.001
Wheeler P. A., Watkins J. M., Hansing R. L. (1997). Nutrients, organic carbon and organic nitrogen in the upper water column of the Arctic ocean: implications for the sources of dissolved organic carbon. Deep Sea Res. Part II Top. Stud. Oceanogr 44, 1571–1592. doi: 10.1016/S0967-0645(97)00051-9
Wickland K. P., Aiken G. R., Butler K., Dornblaser M. M., Spencer R. G. M., Striegl R. G. (2012). Biodegradability of dissolved organic carbon in the Yukon river and its tributaries: Seasonality and importance of inorganic nitrogen. Glob. Biogeochem. Cycles 26, GB0E03. doi: 10.1029/2012GB004342
Wiebe W. J., Sheldon W. M., Pomeroy L. R. (1992). Bacterial growth in the cold: Evidence for an enhanced substrate requirement. Appl. Environ. Microbiol. 58, 359–364. doi: 10.1128/aem.58.1.359-364.1992
Wiebe W. J., Sheldon W. M., Pomeroy L. R. (1993). Evidence for an enhanced substrate requirement by marine mesophilic bacterial isolates at minimal growth temperatures. Microb. Ecol. 25, 151–159. doi: 10.1007/BF00177192
Williford T., Amon R. M. W., Benner R., Kaiser K., Bauch D., Stedmon C., et al. (2021). Insights into the origins, molecular characteristics and distribution of iron-binding ligands in the Arctic ocean. Mar. Chem. 231, 103936. doi: 10.1016/j.marchem.2021.103936
Wilson B., Müller O., Nordmann E.-L., Seuthe L., Bratbak G., Øvreås L. (2017). Changes in marine prokaryote composition with season and depth over an Arctic polar year. Front. Mar. Sci. 4. doi: 10.3389/fmars.2017.00095
Woodgate R. A. (2018). Increases in the pacific inflow to the Arctic from 1990 to 2015, and insights into seasonal trends and driving mechanisms from year-round Bering strait mooring data. Prog. Oceanogr 160, 124–154. doi: 10.1016/j.pocean.2017.12.007
Yamashita Y., Tanoue E. (2003). Chemical characterization of protein-like fluorophores in DOM in relation to aromatic amino acids. Mar. Chem. 82, 255–271. doi: 10.1016/S0304-4203(03)00073-2
Yergeau E., Michel C., Tremblay J., Niemi A., King T. L., Wyglinski J., et al. (2017). Metagenomic survey of the taxonomic and functional microbial communities of seawater and sea ice from the Canadian Arctic. Sci. Rep. 7, 42242. doi: 10.1038/srep42242
Zabłocka M., Kowalczuk P., Meler J., Peeken I., Dragańska-Deja K., Winogradow A. (2020). Compositional differences of fluorescent dissolved organic matter in Arctic ocean spring sea ice and surface waters north of Svalbard. Mar. Chem. 227, 103893. doi: 10.1016/j.marchem.2020.103893
Zeng Y., Yu Y., Li H., He J., Lee S. H., Sun K. (2013). Phylogenetic diversity of planktonic bacteria in the chukchi borderland region in summer. Acta Oceanol Sin. 32, 66–74. doi: 10.1007/s13131-013-0271-y
Keywords: Arctic Ocean, sea water, dissolved organic matter (DOM), bacterial communities, climate warming
Citation: Nguyen HT, Lee YM, Hong JK, Hong S, Chen M and Hur J (2022) Climate warming-driven changes in the flux of dissolved organic matter and its effects on bacterial communities in the Arctic Ocean: A review. Front. Mar. Sci. 9:968583. doi: 10.3389/fmars.2022.968583
Received: 14 June 2022; Accepted: 07 September 2022;
Published: 23 September 2022.
Edited by:
Ana Teresa Lima, Technical University of Denmark, DenmarkReviewed by:
Joanne Oakes, Southern Cross University, AustraliaGilberto Fonseca Barroso, Federal University of Espirito Santo, Brazil
Copyright © 2022 Nguyen, Lee, Hong, Hong, Chen and Hur. This is an open-access article distributed under the terms of the Creative Commons Attribution License (CC BY). The use, distribution or reproduction in other forums is permitted, provided the original author(s) and the copyright owner(s) are credited and that the original publication in this journal is cited, in accordance with accepted academic practice. No use, distribution or reproduction is permitted which does not comply with these terms.
*Correspondence: Jin Hur, amluaHVyQHNlam9uZy5hYy5rcg==