- 1State Key Laboratory of Tropical Oceanography, South China Sea Institute of Oceanology, Chinese Academy of Sciences, Guangzhou, China
- 2Key Laboratory of Tropical Marine Bioresources and Ecology, South China Sea Institute of Oceanology, Chinese Academy of Sciences, Guangzhou, China
- 3Southern Marine Science and Engineering Guangdong Laboratory (Guangzhou), Guangzhou, China
- 4Daya Bay Marine Biology Research Station, Chinese Academy of Sciences, Shenzhen, China
- 5School of Earth and Planetary, University of Chinese Academy of Sciences, Beijing, China
The importance of mangroves in blue carbon storage has been widely reported. However, the potential contribution of microbial extracellular polymeric substances (EPS) to the carbon pool is still poorly understood in mangrove ecosystems. Thus, a natural mangrove reserve located in Gaoqiao was chosen to investigate the linkage between microbial EPS and sediment organic carbon. Sequential resin extraction, Fourier transform infrared spectroscopy (FTIR), and 16S high-throughput sequencing were employed to determine the variations in EPS and bacterial taxa in different mangrove communities. Both EPS and EPS-C increased significantly with mangrove zonation from seaward to landward, irrespective of the EPS subfractions (colloidal and bound EPS) and components (extracellular protein and polysaccharide). Moreover, both EPS and EPS-C were found to be positively correlated with soil organic carbon. The present data further showed that EPS-C accounted for 1.84~10.69% of TOC in surface sediments. Multiple functional groups (e.g., O-H, N-H, and C=O), which may provide ligands for particle adsorption and complexation, were identified by FTIR. Consistent with the ascend of EPS with mangrove zonation from seaward to landward, the highest transmittance intensity for all functional groups was consistently exhibited in EPS isolated from landward Rhizophoraceae forest. In addition, the present data also indicated an interesting positive linkage between EPS and the abundance of some specific bacterial taxa, such as Rhizobiales, Corynebacteriales, and Gaiellales. In summary, this study claims the importance of EPS in the carbon pool in mangrove ecosystems. The present study may provide a better understanding of the functions of mangroves in carbon stocks.
Introduction
As special coastal guardians along tropical and subtropical shorelines, mangroves play a crucial role in windbreaks, siltation promotion and environmental purification (Atwood and Hammill, 2018). Mangrove ecosystems are also famous for their high productivity and carbon density, although the carbon stock in mangroves may vary significantly among different regions and communities (Komiyama et al., 2008; Alongi, 2014b; Wang, 2019; Wang and Gu, 2021). A previous study reported that approximately 75% of organic carbon in mangrove ecosystems was sequestered in sediments (Alongi, 2014a). It would be of interest to further explore the potential factors that may play crucial roles in carbon storage in mangrove sediments.
Extracellular polymeric substances (EPS), a kind of microbial secretion, have been widely found in aquatic habitats (Zhang et al., 2015b). Proteins and polysaccharides are the two dominant components of EPS (Frolund et al., 1995). As a buffer interface around microorganisms, EPS acts as an extra carbon and nutrition pool for microorganisms (Urrea et al., 2017; Tian et al., 2020). EPS-induced biofilms further facilitate the formation of microbial aggregates (Wingender et al., 1999). A large number of studies have clarified that EPS can protect cells and microorganisms from external adversities such as heavy metals (Hou et al., 2013; Kang et al., 2014) and persistent organic pollutants (Kang and Park, 2010; Yang et al., 2020). Moreover, EPS can promote the adsorption of macromolecular organic matter with the extracellular matrix due to its viscosity (Li and Ganczarczyk, 1990). A study of activated sludge treatment of sewage confirmed that EPS also contributed to flocculation settlement by aggregating suspended solids in water (Wilén et al., 2000). Zhu and Yan (2022) further indicated that the contribution of EPS to the carbon stock may far exceed than that of microbial biomass carbon (MBC) itself.
The distribution of mangroves in intertidal regions is highly correlated with geomorphological conditions (Fromard et al., 1998; Ellison et al., 2000; Chowdhury and Maiti, 2016). In southern China, the mangrove community usually possesses an interesting sequential zonation following the variation in environmental factors in the intertidal zones. Generally, pioneer mangrove species (e.g., Avicennia marina) often occur in seaward zones with low tidal gradients where the sediment pore water has relatively high salinity but low nutrients, while Rhizophoraceae mangroves are often located in the middle and/or landward areas with lower flooding stress (Cheng et al., 2020). Furthermore, carbon stock and sequestration potential of sediments may vary significantly among different mangrove communities along a continuous tidal gradient during mangrove zonation (Feng et al., 2019; Chen et al., 2021a). Moreover, both EPS contents and producers (e.g., bacteria, fungi, and benthic diatoms) may also vary significantly with the variation in aboveground mangroves (Xiao and Zheng, 2016; Wang et al., 2019b; Mai et al., 2022). Unfortunately, the dynamics of microbial EPS and their potential functions on carbon stock and sequestration in mangrove ecosystems are still poorly understood.
Therefore, a natural mangrove reserve with a three-zonation stage was chosen to (1) investigate the microbial community and distribution of EPS in different mangrove zonation gradients; (2) explore the potential effect of EPS on the carbon stock in mangrove ecosystems; and (3) investigate the relationships among mangrove zonation, microbial structure, EPS, and carbon stock. To the best of our knowledge, this is the first study to explore the carbon stock from the aspect of microbial EPS and provide a new perspective to realize variation in mangrove carbon storage in different mangrove communities.
Materials and methods
Sediment sampling
A natural mangrove reserve located in Gaoqiao, Zhanjiang City, Guangdong Province, China, was selected since it is one of the largest mangrove natural reserves in China. This region has a subtropical monsoon climate, with an average annual temperature of 23.4 °C and average annual precipitation of 1816 mm (mainly from April to September). Mangrove species colonization is partially controlled by terrain and tide.
In August 2021, surface sediment samples (0-5 cm) were collected from the Gaoqiao mangrove reserve. There were four sampling sites from seaward to landward (Figure 1), which represent the locations of bare mudflat (BM), Avicennia marina (AM, seaward pioneer mangrove), Aegiceras corniculatum (AC, medium transitional mangroves), and mixed Rhizophoraceae forest (MF, landward mixed rhizophoraceae forest, mainly occupied by Rhizophora stylosa and Bruguiera gymnorrhiza). At each sampling site, five surface sediment samples, with an interval of no less than 50 m, were collected. Thus, 20 packs of fresh sediment samples were acquired. Samples were transferred (stored with dry ice) to the laboratory in two days after field sampling.
Analysis of sediment physicochemical parameters
For total organic carbon (TOC) measurement approximately 0.05 g air-dried sediment was incubated in 0.5 mL 2 M HCl to remove carbonates (Cheng et al., 2020), then the samples were dried in the analyzer reactor with 500 mL/min air flow. The content of TOC was then determined using an elemental analyzer (TOC-V, CPH, Shimadzu, Japan) equipped with a solid sample module (SSM-5000A, Shimadzu, Japan).
For other physicochemical parameters, a pH meter (S210-KCN, Mettler Toledo, Germany) was employed to determine the pH of a 1:2.5 soil-to-water ratio turbid liquid, while salinity was measured in a 1:5 soil-to-water ratio turbid liquid using a salinity analyzer (PNT3000, STEPS, Nuremberg, Germany) (Wang et al., 2019a). Total nitrogen (TN) and total phosphorus (TP) analyses were performed using the modified Kjeldahl method and the molybdate reduction method according to the standard methods (HJ 717-2014 and HJ 632-2011), respectively.
EPS analysis and determination
EPS was extracted using cation exchange resin as described by previous investigators with minor modifications (Takahashi et al., 2009; Mai et al., 2022). In brief, artificial seawater (30‰ salinity) was added to tubes containing 30 g of fresh sediment and mixed in a shaker for 1 hour at 4°C in the dark. The supernatant was collected after centrifugation at 3500 rpm at 4°C for 10 min to obtain colloidal EPS. Then, 20 mL of artificial seawater and 2 g of activated cation exchange Dowex 50 WX8 resin (hydrogen form, 200-400 mesh, Sigma-Aldrich, MO, USA) were added to the remaining sediment, mixed for 1 hour at 4°C in the dark and then centrifuged at 3500 rpm at 4°C for 10 min to obtain bound EPS. Both the supernatants of the two EPS subfractions were purified using dialysis bags (3.5 kD, incubating at 4°C for 24 hours). The purified EPS samples were then freeze-dried.
The freeze-dried EPS powder was placed in an attenuated total reflection (ATR, Miracle, Pike, America) instrument after meticulously grinding. Analysis was conducted at 25 °C with Fourier transform infrared spectroscopy (FTIR, IR Affinity-1, Shimadzu, Japan) to record the functional group composition of the two EPS subfractions. A total of 25 scans with a resolution of 4 cm-1 and a scanning range of 500-4000 cm-1 were performed (Yang et al., 2018). The atmosphere was used as a blank, and the transmittance of air was subtracted for each testing sample. The inverted peaks thus appeared near 2400 cm-1 due to CO2 in the air.
For the measurements of extracellular protein (EPS-PT) and polysaccharide (EPS-PSC), the freeze-dried colloidal and bound EPS powder was redissolved in 10 mL of deionized water, respectively. The protein concentration was determined using a modified bicinchoninic acid protein assay kit (Sangon Biotech, Shanghai, China) with bovine serum albumin as the standard (Smith et al., 1985). The carbon ratio of EPS-PT was estimated to be an average of 40% according to the data reported by Bura et al. (1998). Polysaccharide content was determined using the phenol−sulfuric acid method with glucose as the standard (DuBois et al., 1956). Polysaccharides were assumed to be all converted to furfural (C5H4O2), so the carbon ratio of EPS-PSC was estimated to be 62.5% (DuBois et al., 1956). An elemental analyzer (TOC-V, CPH, Shimadzu, Japan), which was configured with an automatic sampler, was employed to measure dissolved organic carbon in EPS solution (Jiang et al., 2006).
16S illumina sequencing
Total DNA in 1 g of sediment was extracted by the Soil DNA Extraction Kit (Omega, United States). The 16S rRNA gene was amplified using 338F (5′ -ACTCCTACGGGAGGCAGCAG-3′) and 806R (5′-GGACTACHVGGGTWTCTAAT-3′) primers. Polymerase chain reaction (PCR) was performed in a 50 μL reaction system. The PCR program was as follows: pre-denaturation at 98°C for 2 min; 30 cycles of denaturation at 95°C for 1.5 min, annealing at 55°C for 30 s and extension at 72°C for 45 s; and final extension at 72°C for 10 min and preservation at 4°C. The PCR product was purified by agarose gel electrophoresis and recovered via a DNA Gel Extraction Kit (Omega, D2500-01, United States). The Illumina HiSeq platform (Illumina, San Diego, CA, United States) was employed for paired-end sequencing according to standard protocols. All sequences were submitted to the National Center for Biotechnology Information (NCBI) database.
Raw sequences were processed using Trimmomatic (Bolger et al., 2014), and merged using FLASH (version 1.2.11) (Magoč and Salzberg, 2011). The flashed reads were analyzed with the Quantitative Insights into Microbial Ecology (QIIME) (version 1.9.1) (Caporaso et al., 2010) and compared with a reference database (Gold database, http://drive5.com/uchime/uchime_download.html) (Haas et al., 2011) using the UCHIME algorithm to obtain effective tags (Edgar et al., 2011). Sequence analysis was processed using the UPARSE software (Uparse v7.0.1001), and 97% similarity was considered to indicate the same OTUs. For each representative sequence, taxonomic information with a confidence threshold of ≥ 0.5 was annotated using the Silva reference databases (Quast et al., 2013).
Data analysis
The data were tested for normality (Kolmogorov-Smirnov test, SPSS, version 22.0), and one-way analysis of variance (least significant difference post hoc test, LSD) was performed to evaluate significant differences. Kaiser-Meyer-Olkin (KMO) test was employed to analyze the correlation coefficient between all the variations before the principal component analysis (PCA) was performed. PCA and Pearson correlation matrix were performed using the software of Origin to assess the relationship between EPS-PT and EPS-PSC, EPS-C and sediment physicochemical properties (TOC, TN, TP, pH, salinity).
The raw bacterial reads were uploaded to NCBI (PRJNA839550). Data were analyzed base on the Majorbio cloud platform (www.majorbio.com). Analysis of similarities (ANOSIM) was performed to determine the statistical significance of differences of all OTUs between samples. Principal coordinate analysis (PCoA) was used to investigate the association with microbial communities among sites. Kruskal Wallis H test was applied in the analysis of the significant differences of species in four groups of sediment samples and their comparison between each pair (Wei et al., 2014). Correlation analysis among sediment physicochemical properties and bacterial community structure (using the relative abundance of microbial taxa) was demonstrated by a two-matrix correlation heatmap.
Results
Soil TOC and other physicochemical parameters
According to Table 1, TOC concentrations ranged from 3.22 to 39.57 g/kg, and the highest TOC concentration was found in MF, followed by AC, AM, and BM. Similar distribution patterns were also found in TN and TP, with the highest values in MF but the lowest in BM. In contrast, the maximum salinity was observed in BM, while the minimum salinity was observed in MF. The pH values ranged from 6.40 to 7.20, and the highest pH was found in BM, followed by AC, MF, and AM.

Table 1 Variation in sediment physicochemical properties at different sampling sites. Mean ± SE, small letters represent significant differences (p < 0.05) among the four sites.
EPS subfractions and components
The concentration of total EPS-PSC ranged from 18.04 to 158.64 mg/kg. As shown in Table 2, the maximum EPS-PSC is detected in MF, followed by AC, AM, and BM. Consistent with EPS-PSC, similar distribution patterns were also observed in colloidal and bound EPS-PSC.

Table 2 Variation in EPS at different sampling sites. Mean ± SE, small letters represent significant differences (p < 0.05) among the four sites.
The concentration of total EPS-PT ranged from 66.97 to 171.31 mg/kg (Table 2). Similarly, higher concentrations of bound EPS-PT were also detected in mangrove sediments than in bare mudflat. However, except for AM, the contents of colloidal EPS-PT were comparable between mangrove and mudflat sediments.
EPS-C
In total, EPS-C ranged from 0.33 to 0.73 g/kg, much higher EPS-C was detected in mangrove sediments than in mudflat, and the highest EPS-C was observed in MF. However, the bare mudflat exhibited a higher EPS-C/TOC ratio (10.69%) than the mangrove sediments (1.84 ~ 2.98%) (Figure 2).
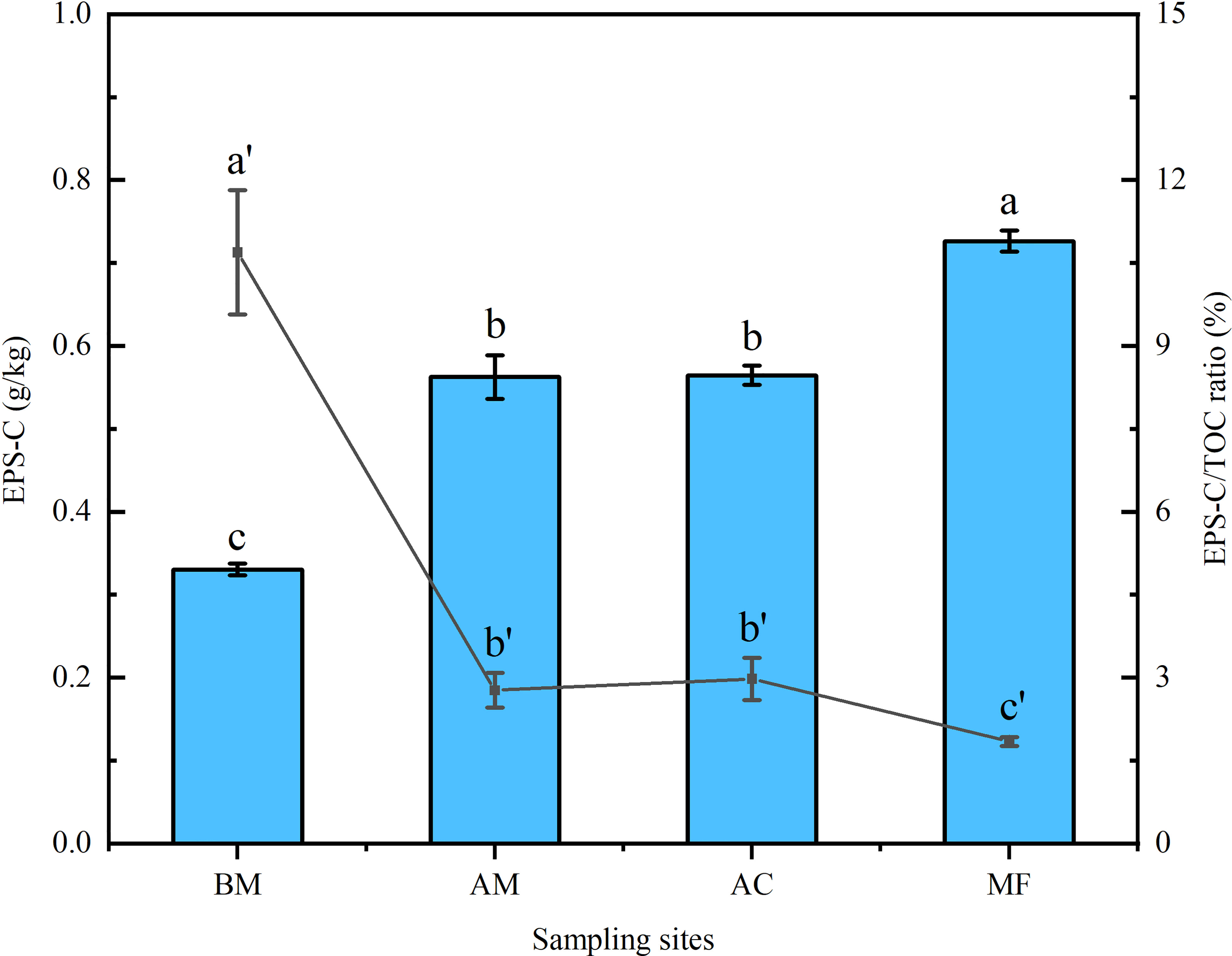
Figure 2 Variation in EPS-C content and EPS-C/TOC ratio in sediments associated with four sampling sites. The bar chart (relevant to the left Y-axis) and line graph (relevant to the right Y-axis) represent the values of EPS-C and the EPS-C/TOC ratio, respectively. Mean ± SE, different letters show significant differences (p < 0.05) among the four different sampling sites.
Detailed information for colloidal and bound EPS-C is shown in Figure S1. Both the contents of colloidal and bound EPS-C increased significantly (p < 0.05) with mangrove zonation from seaward to landward. The highest bound EPS-C ratio was also found in MF, followed by AC, AM, and BM. For the component of EPS-C, protein and polysaccharide occupied 8.10~11.77% and 3.41~13.78% of EPS-C, respectively, approximately equal to the ratio of these two components in total EPS (Table S1).
FTIR spectra of EPS
Similar infrared bands of EPS were observed, regardless of colloidal or bound subfraction. The results from Figure 3 show five dominant peak segments: 3520-3200 cm-1 in region I (O-H stretching associated with oxhydryl), 1800-1600 cm-1 in region II (C=C, C=O stretching, C=N, N-H vibrations from proteins), 1600-1400 cm-1 in region III (stretching of O-alkyl group, methyl and methylene which may be attributed to proteins), 1200-1000 cm-1 in region IV (ring vibrations of P=O, P-O, C-O-C, C-O-P partially attributed to polysaccharides) and 650-400 cm-1 in region V (region without functional groups, stretching of C-H). In terms of the difference in FTIR transmittance intensity among EPS extracted from the four sampling sites, the highest transmittance intensity for all infrared bands was consistently exhibited in MF, followed by AC, AM, and BM.
Microbial community composition
The ANOSIM results showed that significant differences were observed in the microbiome among BM, AM, AC, and MF (ANOSIM R = 0.7527, p = 0.001). The results of Figure 4A illustrate the differences in microbial composition among the four sampling sites at the class level. Compared with BM, higher abundances of Alphaproteobacteria, Actinobacteria, and Thermoleophilia were detected in mangrove sediments, especially in the sediment associated with MF.
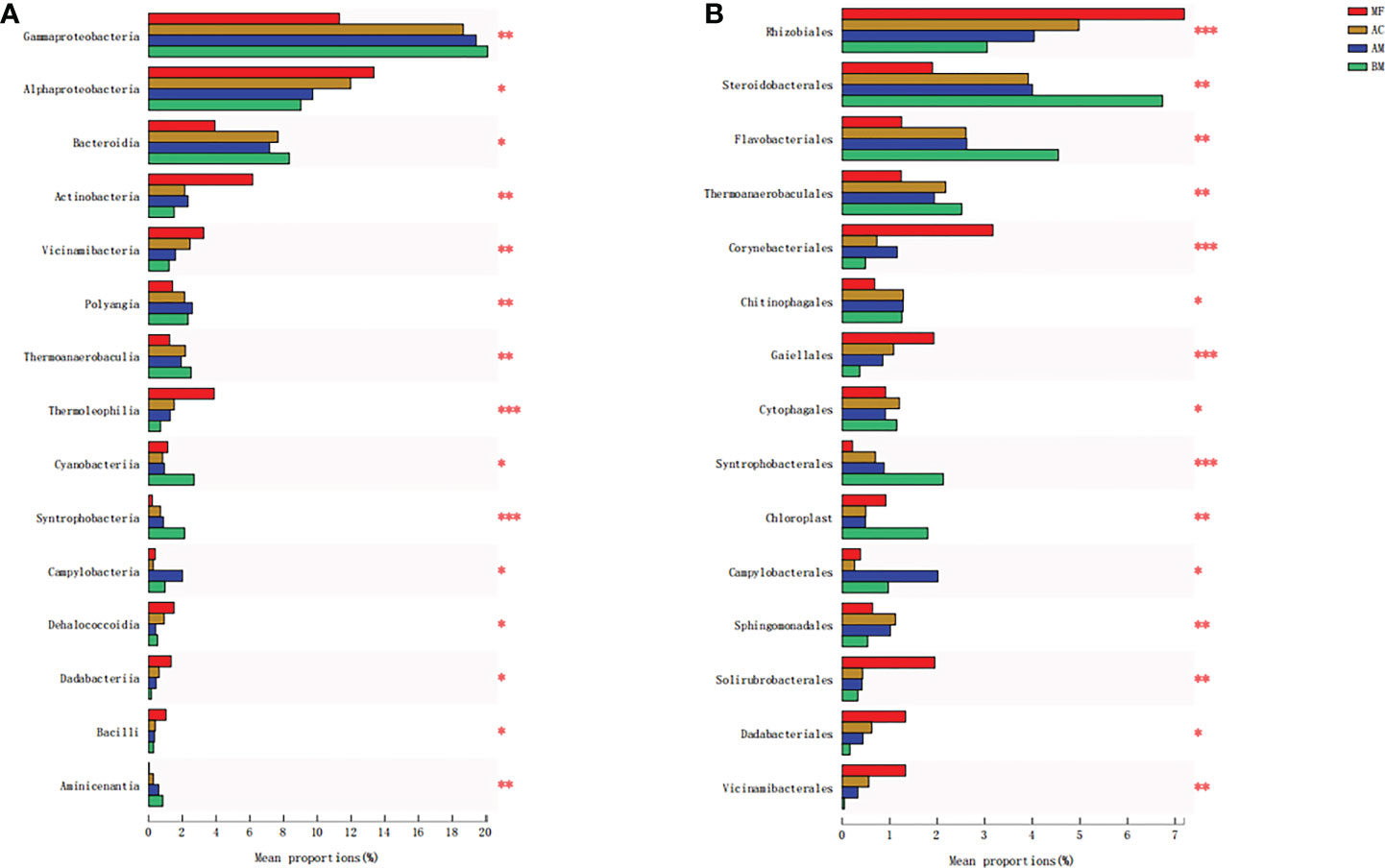
Figure 4 Significant difference analyses of the majority of bacterial taxa at the class level (A) and order level (B) from the four sampling sites. Meaning of asterisks, *p < 0.05; **p < 0.01; ***p < 0.001.
Figure 4B lists the discrepancy in microbial composition at the order level. Specifically, the sediment of MF exhibited the highest abundance of Rhizobiales and Solirubrobacterales in comparison with the other three sampling sites. Similarly, Corynebacteriales and Gaiellales also showed the highest abundance in MF sediment, whereas the lowest abundance was found in BM sediment. More detailed comparisons between each pair of bacteria are presented in Figures S2, S3.
Relationships between microbial composition, EPS, EPS-C, TOC, and other soil physicochemical properties
According to the PCoA results (Figure S4), microbial communities showed a similarity in AM and AC, while microbial communities of BM and MF were quite different. As shown in Figure 5, a significant positive relationship was observed between TOC and EPS-C, with a correlation coefficient of 0.92. Furthermore, both TOC and EPS-C were positively correlated with EPS-PT and EPS-PSC. In addition, TN and TP were also positively correlated with TOC and EPS-C.
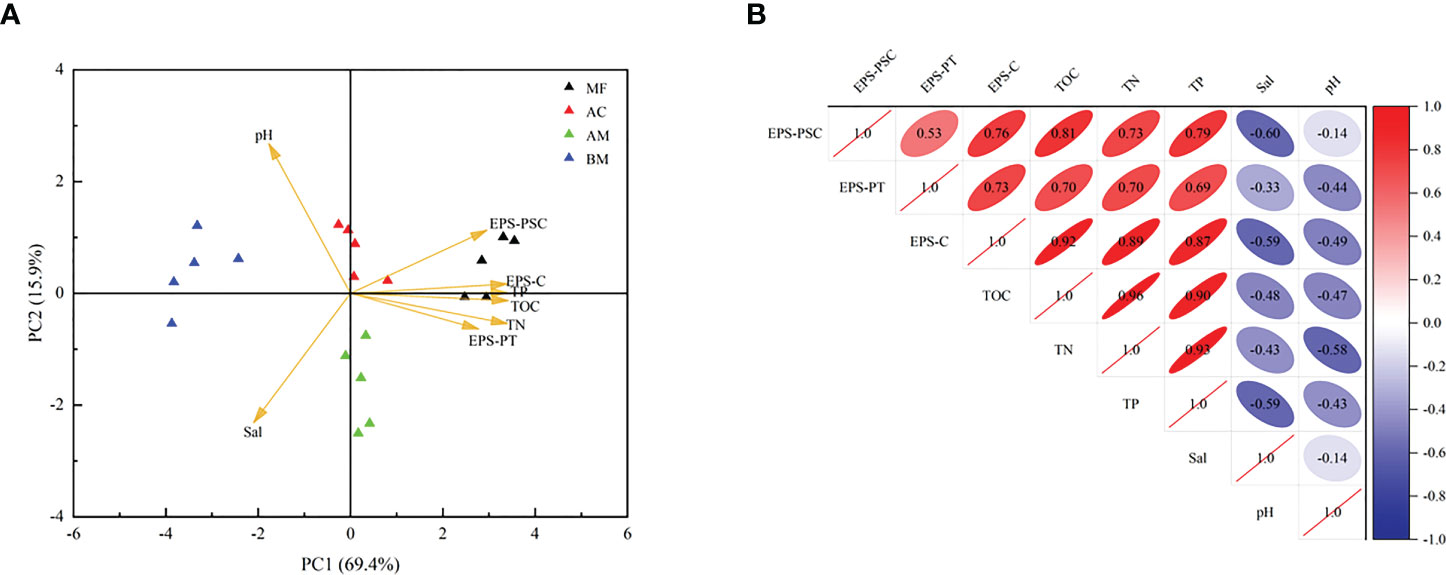
Figure 5 Principal component analysis and Pearson’s correlation analysis of sediment physicochemical properties (KMO value = 0.690; n = 20). (A): Triangle scatters in different colors represent the four sampling sites. (B): Positive correlations are identified in red, while negative correlations are identified in blue.
As shown in Figure 6A, at the class level, Alphaproteobacteria, Actinobacteria, Vicinamibacteria, and Thermoleophilia were positively correlated not only with TOC, TN and TP but also with EPS (e.g., EPS-PSC and EPS-PT) and EPS bound carbon (EPS-C). More detailed correlation analysis at the order level is presented in Figure 6B. Specifically, Rhizobiales, Microtrichales, Corynebacteriales, and Gaiellales were positively associated with EPS components and EPS-C. Positive correlations between those bacterial orders and sediment nutrient parameters (e.g., TN, TP, and TOC) were also observed. More information on the correlation analyses between EPS subfractions and the bacterial community at the class and/or order level is displayed in Figure S5.
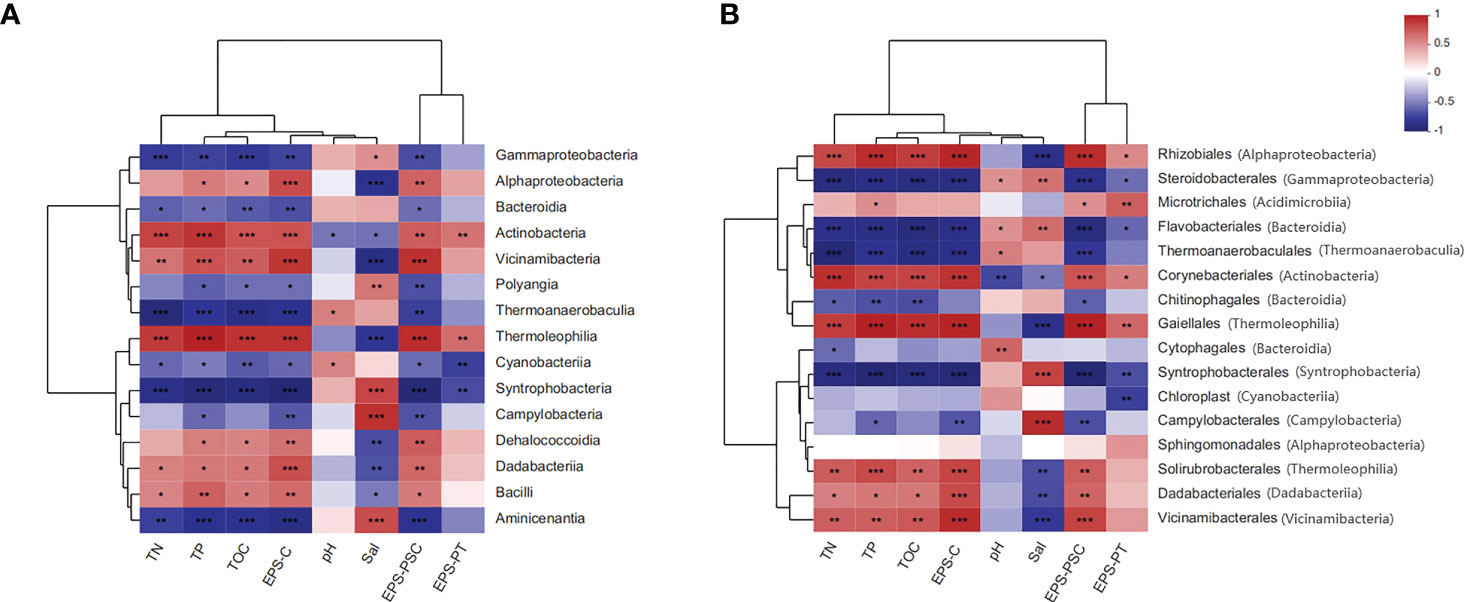
Figure 6 Two-matrix heatmap analyses for the relationships between sediment parameters and the bacterial community. (A): correlation analysis of the bacterial community at the class level; (B): correlation analysis of the bacterial community at the order level. Red indicates positive correlations, while blue indicates negative correlations. Meaning of asterisks, *p < 0.05; **p < 0.01; ***p < 0.001.
Discussion
Variation in carbon storage in sediments with mangrove zonation along a continuous tidal gradient
In this study, the content of TOC in the Gaoqiao mangrove reserve ranged from 19.96 to 39.57 g/kg, higher than that of broad-leaved forests in northeast Guangdong Province (ranging from 16.75 to 22.14 g/kg) (Lin and Gao, 2009). The contents of TOC, TN, and TP also increased significantly (p < 0.05) with the elevation of the tidal gradient during mangrove zonation (Table 1). Consistent with previous findings (Wang et al., 2013), the present data also found that landward mangrove sediment has a higher soil organic carbon concentration.
Organic carbon concentrations in sediments may vary significantly between different mangrove communities due to differences in tidal gradients, plant diversity, and deposition rates (Gleason and Ewel, 2002; Ceron et al., 2011). In this study, the seaward pioneer Avicennia marina and transition mangroves (Aegiceras corniculatum) exhibited very low plant diversity (almost pure forest). Previous studies have shown a positive correlation between mangrove diversity and sediment carbon storage (MacKenzie et al., 2016; Lamont et al., 2019). Rhizophoraceae plants, which occur in the middle and/or landward regions along the southern China coast, often constitute the climax mangrove community with the highest plant productivity and diversity (Bai et al., 2021). The enhancement of soil organic carbon in landward MF in this study may also be partly ascribed to its higher plant diversity. Therefore, the application of a multispecies planting strategy in mangrove restoration may improve biodiversity and blue carbon storage simultaneously (Alongi, 2011).
Variation in microbial community structure and EPS content with mangrove zonation
The data presented in Table 2 show that the EPS content increased significantly with mangrove zonation from seaward to landward. This increase may partly be ascribed to the improvement of sediment nutrient concentrations (such as TOC, TN, and TP) (Table 1) and some specific microbial taxa (Figures 4, S2, S3) during mangrove zonation in intertidal regions (Figure 5). In this study, the highest abundances of Alphaproteobacteria and Actinobacteria were found in the sediment of MF (Figure 4A).
A positive correlation was observed between these two classes of bacteria and EPS (Figure 6A). It was reported that Alphaproteobacteria and Actinobacteria could secrete EPS to ameliorate the rhizosheath microenvironment and increase water retaining capacity (Marasco et al., 2022). Previous studies also claimed that Alphaproteobacteria were the main EPS-producing bacteria in membrane bioreactors (Neoh et al., 2017). In addition, these two bacterial taxa were found to participate in the degradation of litterfall, rhizosphere metabolites, and recalcitrant organic carbon (Zhang et al., 2020). Increased TOC induced with the elevation of the tidal gradient along mangrove zonation may also promote the growth of these two bacterial taxa.
Correlation analysis at the order level (Figure 6B) suggested that increasing Rhizobiales, Corynebacteriales and Gaiellales abundances from seaward to landward may enhance EPS secretion. Rhizobiales are known as beneficial partners in interactions (Liu et al., 2022). Rhizobiales in carbon-rich substrates may accelerate the decomposition of amino acids and phenols due to their abundant extracellular carboxylase and oxidase enzymes (Mai et al., 2021; Yu et al., 2022). Moreover, Rhizobiales are regarded as one of the important producers of extracellular polymers in membrane bioreactors. EPS secreted by Rhizobiales are crucial for their stabilization and reproduction (Santaella et al., 2008; Cydzik-Kwiatkowska, 2015). Previous investigators also claimed that Solirubrobacterales promoted the formation of soil aggregates synergizing with Rhizobiales (Marcos et al., 2019). Guo et al. (2020) claimed the importance of Corynebacteriales in carbon metabolism and cellulose biodegradation. Chen et al. (2021b) also reported that Gaiellales could accelerate the formation process of soil aggregates through EPS secretion. The results showed that the abundance of some other bacteria (e.g., Steroidobacterales, Flavobacteriales, Syntrophobacterales, etc) was negatively correlated with sediment parameters (e.g., saline and pH) and EPS (Figure 6). The growth of these bacterial taxa may be limited by abiotic factors in mangrove sediment (e.g., high salinity, anaerobic and acidic conditions) (Wu et al., 2016), leading to a lower contribution to EPS content. In addition, according to the difference analysis of EPS in a diatom-dominated intertidal mudflat (Marennes–Oléron, France), the EPS content was higher in winter than that in summer (Pierre et al., 2014).
In summary, the dynamics of EPS are jointly regulated by the bacterial community. However, the enriched microbial taxa can either be EPS producing taxa or benefit from other organic substrates released by other organisms. The mechanisms of EPS secretion from microbes, especially for bacteria isolated from mangrove sediments, are still poorly understood. Investigation for screening EPS-producing bacterial taxa needs to be further conducted. In addition, similar studies on the relationships between EPS and other microorganisms, such as algae and fungi, should also be conducted.
Linkage between EPS and carbon storage and transportation in mangrove ecosystems
The present data showed that both EPS and EPS-C were positively correlated with TOC (Figure 5), and a similar positive relationship between glomalin-related soil protein and soil organic carbon was also reported (Zhang et al., 2015a; Wang et al., 2018). In this study, most of the EPS-C may come from the EPS itself because most organic particles attached to the EPS were removed during EPS extraction and purification, except for some soluble organic colloids (Yang et al., 2020). The present data further indicated a linkage between mangrove zonation and increased EPS-C. As shown in Figure 2, EPS-C contributed 1.84~10.69% of TOC, which is much higher than that of MBC in tropical (subtropical) forests (1.5%) and mangroves (0.45%) (Xu et al., 2013; Wang et al., 2018).
In this study, both colloidal and bound EPS-C increased significantly with mangrove zonation from seaward to landward (Figure S1). Soluble colloidal EPS may be easily degraded and utilized by bacteria and algae (McKew et al., 2013), while bound EPS is generally considered recalcitrant carbon and may be subjected to long-term retention and diagenesis in sediments (Evrard et al., 2008; Luo et al., 2020). Increased EPS (especially the increased bound EPS-C ratio, Figure S1B) favored by mangrove zonation may thus contribute to the burial of stable organic carbon in the sediment of landward MF. For EPS-C components, the present data showed that protein and polysaccharide only occupied a part of EPS-C, approximately equal to the ratio of these two components in total EPS (Table S1). The contributions of other EPS components (e.g., nucleic acids, lipids and humus) to EPS-C and the turnover rate of different EPS sub-fractions and components, as well as their potential contribution to the long-term storage of sediment carbon stock, are worth further investigation (Flemming and Wingender, 2001).
As shown in Figure 3, EPS isolated from mangrove sediments contained multiple functional groups (e.g., O-H, N-H, -COO-, and P-O). Similar spectroscopic characterization and functional traits were also observed in EPS isolated from single bacteria and/or activated sludge (Guibaud et al., 2005; Badireddy et al., 2008). Although the spectroscopic characterization and functional traits of EPS were similar, the spectral absorption peaks of these functional groups appeared to increase with the increase in tidal gradient along mangrove zonation, consistent with increased EPS content. Increased EPS and functional groups may facilitate bioflocculation and sedimentation through interparticle bridging and biosorption, leading to more organic particles precipitation during tidal flooding (Gerbersdorf and Wieprecht, 2015; Xu et al., 2017). Besides, increased EPS may also facilitate the formation process of soil aggregates and thus may partly protect soil organic carbon from mineralization (Degens, 1997; Deka et al., 2019). Moreover, interattraction between charged EPS functional groups and mineral particles may promote the formation of mineral-associated organic carbon (Lin et al., 2016; Liu et al., 2022). In addition, our previous study indicated that increased EPS favored by mangrove restoration can promote sediment anti-scouribility (Mai et al., 2022). Thus, higher EPS in landward MF sediment may not only benefit for TOC preservation in sediments, but also reduce soil TOC erosion simultaneously. More detailed mechanisms of EPS on soil carbon sequestration in mangroves are worthy of further investigation.
Nevertheless, it was interesting that bare mudflat sediments possessed a higher ratio of EPS-C/TOC than mangrove sediments, although a much lower content of EPS-C was detected in the sediment of the bare mudflat (Figure 2). The origin of sediment organic carbon is dominated by autochthonous carbon favored by mangrove litters (Xiong et al., 2018). The lower EPS-C/TOC ratio in mangrove sediments may be partly ascribed to the increment of vegetation carbon inputs driven by plant litters from aboveground mangroves (Feng et al., 2017). In the bare mudflat, almost 11% of sediment organic carbon was contributed by EPS-C. A previous study inferred that the majority of mangrove dissolved organic carbon will be exported into the estuary (up to 65%) (Romigh et al., 2006). Frequent tidal flooding may further enhance carbon exchange between mangrove sediments and their neighboring mudflats (Bouillon et al. (2007). Generally, the function of mudflats in carbon storage and sequestration is often ignored. Phang et al. (2015) estimated that mudflat carbon storage in the Chek Jawa intertidal zone in Singapore is equivalent to 29% of local mangrove carbon storage. Moreover, The amassment of nutrients in mudflats may benefit the invasion of pioneer mangrove species. Further studies focused on mangrove carbon export/import, such as the application of isotopic tracing and biomarker analyses, need to be conducted in the future.
Conclusion
This study provided evidence for a linkage between mangrove zonation and increased microbial EPS that may contribute to carbon storage in sediments. The data showed that EPS-C ranged from 0.33 to 0.73 g/kg and accounted for 1.84~10.69% of the total organic carbon in surface sediments. Moreover, the content of soil organic carbon was found to be positively related to EPS and EPS-C. Multiple functional groups (e.g., stretching of O-H, N-H, and C=O) in EPS that may facilitate the adsorption of organic materials were identified by FTIR. Consistent with the increasing EPS contents with mangrove zonation from seaward to landward, the highest transmittance intensity of all functional groups was also observed in the landward Rhizophoraceae forest. In addition, the results showed a positive correlation between the EPS content and the abundance of certain bacterial taxa, such as Rhizobiales, Corynebacteriales, and Gaiellales. This study indicated the importance of microbial EPS in the carbon stock in mangrove sediments.
Data availability statement
The data presented in the study are deposited in the NCBI (https://www.ncbi.nlm.nih.gov/) repository, accession number PRJNA839550.
Author contributions
D-XL contributed to the experiments, data analysis and the first draft of the manuscript; Z-MM and C-CS contributed to microbial analysis; Y-SW and HC contributed to conception and design of the study; H-HL and Y-WZ conducted field sample collection. All authors contributed to the article and approved the submitted version.
Funding
This research was supported by the National Key Research and Development Program of China (Science & Technology Basic Resources Investigation Program of China) (2017FY100707), Independent Research Project of State Key Laboratory of Tropical Oceanography (LTOZZ2202), Key Special Project for Introduced Talents Team of Southern Marine Science and Engineering Guangdong Laboratory (Guangzhou) (GML2019ZD0303 and GML2019ZD0305), National Natural Science Foundation of China (41676086, 41890853, 41906128, 42073078 and U1901211), Strategic Priority Research Program of the Chinese Academy of Sciences (XDA23050200), and Finance Science and Technology Project of Hainan Province (No. ZDKJ202018), Guangdong Basic and Applied Basic Research Foundation (No.2020A1515011137) .
Conflict of interest
The authors declare that the research was conducted in the absence of any commercial or financial relationships that could be construed as a potential conflict of interest.
Publisher’s note
All claims expressed in this article are solely those of the authors and do not necessarily represent those of their affiliated organizations, or those of the publisher, the editors and the reviewers. Any product that may be evaluated in this article, or claim that may be made by its manufacturer, is not guaranteed or endorsed by the publisher.
Supplementary material
The Supplementary Material for this article can be found online at: https://www.frontiersin.org/articles/10.3389/fmars.2022.967767/full#supplementary-material
Abbreviations
BM, bare mudflat; AM, Avicennia marina; AC, Aegiceras corniculatum; MF, mixed Rhizophoraceae forest; EPS, extracellular polymeric substances; EPS-PT, extracellular protein; EPS-PSC, extracellular polysaccharide; EPS-C, EPS carbon; FTIR, Fourier transform infrared spectroscopy; TOC, total organic carbon; Sal, salinity; TN, total nitrogen; TP, total phosphorus.
References
Alongi D. M. (2011). Carbon payments for mangrove conservation: ecosystem constraints and uncertainties of sequestration potential. Environ. Sci. Policy 14, 462–470. doi: 10.1016/j.envsci.2011.02.004
Alongi D. M. (2014a). Carbon cycling and storage in mangrove forests. Annu. Rev. 6 (1), 195–219. doi: 10.1146/annurev-marine-010213-135020
Alongi D. M. (2014b). Carbon sequestration in mangrove forests. Carbon Manage. 3, 313–322. doi: 10.4155/cmt.12.20
Atwood T. B., Hammill E. (2018). The importance of marine predators in the provisioning of ecosystem services by coastal plant communities. Front. Plant Sci. 9. doi: 10.3389/fpls.2018.01289
Badireddy A. R., Korpol B. R., Chellam S., Gassman P. L., Engelhard M. H., Lea A. S., et al. (2008). Spectroscopic characterization of extracellular polymeric substances from Escherichia coli and Serratia marcescens: suppression using sub-inhibitory concentrations of bismuth thiols. Biomacromolecules 9 (11), 3079–3089. doi: 10.1021/bm800600p
Bai J. K., Meng Y. C., Gou R. K., Lyu J. C., Dai Z., Diao X. P., et al. (2021). Mangrove diversity enhances plant biomass production and carbon storage in hainan island, China. Funct. Ecol. 35, 774–786. doi: 10.1111/1365-2435.13753
Bolger A. M., Lohse M., Usadel B. (2014). Trimmomatic: a flexible trimmer for illumina sequence data. Bioinformatics 30 (15), 2114–2120. doi: 10.1093/bioinformatics/btu170
Bouillon S., Dehairs F., Velimirov B., Abril G., Borges A. V. (2007). Dynamics of organic and inorganic carbon across contiguous mangrove and seagrass systems (Gazi bay, Kenya). J. Geophys. Res.: Biogeosci. 112, G02018. doi: 10.1029/2006JG000325
Bura R., Cheung M., Liao B., Finlayson J., Lee B. C., Droppo I. G., et al. (1998). Composition of extracellular polymeric substances in the activated sludge floc matrix. Water Sci. Technol. 37 (4-5), 325–333. doi: 10.2166/wst.1998.0657
Caporaso J. G., Kuczynski J., Stombaugh J., Bittinger K., Bushman F. D., Costello E. K., et al. (2010). QIIME allows analysis of high-throughput community sequencing data. Nat. Methods 7 (5), 335–336. doi: 10.1038/nmeth.f.303
Ceron R. M., Ceron J. G., Guerra J. J., Zavala J. C., Amador L. E., Endanu E., et al. (2011). “Determination of the amount of carbon stored in a disturbed mangrove forest in campeche, Mexico,” in Ecosystems and sustainable development VIII. (Univ Alicante: WIT Press).
Chen S., Chen B., Chen G., Ji J., Yu W., Liao J., et al. (2021a). Higher soil organic carbon sequestration potential at a rehabilitated mangrove comprised of Aegiceras corniculatum compared to Kandelia obovata. Sci. Total Environ. 752, 142279. doi: 10.1016/j.scitotenv.2020.142279
Cheng H., Liu Y., Jiang Z. Y., Wang Y. S. (2020). Radial oxygen loss is correlated with nitrogen nutrition in mangroves. Tree Physiol. 40, 1548–1560. doi: 10.1093/treephys/tpaa089
Chen Z., Maltz M. R., Zhang Y. G., O’Brien B. J., Neff M., Wang Y. H., et al. (2021b). Plantations of Cinnamomum camphora (Linn) presl with distinct soil sacterial communities mitigate soil acidity within polluted locations in southwest China. Forest 12, 657–671. doi: 10.3390/f12060657
Chowdhury A., Maiti S. K. (2016). Identification of metal tolerant plant species in mangrove ecosystem by using community study and multivariate analysis: a case study from Indian sunderban. Environ. Earth Sci. 75 (9), 21. doi: 10.1007/s12665-016-5391-1
Cydzik-Kwiatkowska A. (2015). Bacterial structure of aerobic granules is determined by aeration mode and nitrogen load in the reactor cycle. Bioresource Technol. 181, 312–320. doi: 10.1016/j.biortech.2015.01.101
Degens B. P. (1997). Macro-aggregation of soils by biological bonding and binding mechanisms and the factors affecting these: a review. Soil Res. 35 (3), 431–460. doi: 10.1071/S96016
Deka P., Goswami G., Das P., Gautom T., Chowdhury N., Boro R. C., et al. (2019). Bacterial exopolysaccharide promotes acid tolerance in Bacillus amyloliquefaciens and improves soil aggregation. Mol. Biol. Rep. 46, 1079–1091. doi: 10.1007/s11033-018-4566-0
DuBois M., Gilles K. A., Hamilton J. K., Rebers P. A., Smith F. (1956). Colorimetric method for determination of sugars and related substances. Anal. Chem. 28, 350–356. doi: 10.1021/ac60111a017
Edgar R. C., Haas B. J., Clemente J. C., Quince C., Knight R. (2011). UCHIME improves sensitivity and speed of chimera detection. Bioinformatics 27 (16), 2194–2200. doi: 10.1093/bioinformatics/btr381
Ellison A. M., Mukherjee B. B., Karim A. (2000). Testing patterns of zonation in mangroves: scale dependence and environmental correlates in the sundarbans of Bangladesh. J. Ecol. 88 (5), 813–824. doi: 10.1046/j.1365-2745.2000.00500.x
Evrard V., Cook P. L. M., Veuger B., Huettel M., Middelburg J. J. (2008). Tracing carbon and nitrogen incorporation and pathways in the microbial community of a photic subtidal sand. Aquat. Microb. Ecol. 53 (3), 257–269. doi: 10.3354/ame01248
Feng J. X., Wang S. G., Wang S. J., Ying R., Yin F. M., Jiang L., et al. (2019). Effects of invasive Spartina alterniflora loisel. and subsequent ecological replacement by Sonneratia apetala buch.-ham. on soil organic carbon fractions and stock. Forest 10, 171. doi: 10.3390/f10020171
Feng J. X., Zhou J., Wang L. M., Cui X. W., Ning C. X., Wu H., et al. (2017). Effects of short-term invasion of Spartina alterniflora and the subsequent restoration of native mangroves on the soil organic carbon, nitrogen and phosphorus stock. Chemosphere 184, 774–783. doi: 10.1016/j.chemosphere.2017.06.060
Flemming H. C., Wingender J. (2001). Relevance of microbial extracellular polymeric substances (EPSs) - part I: Structural and ecological aspects. Water Sci. Technol. 43 (6), 1–8. doi: 10.2166/wst.2001.0326
Frolund B., Griebe T., Nielsen P. H. (1995). Enzymatic-activity in the activated-sludge floc matrix. Appl. Microbiol. Biotechnol. 43, 755–761. doi: 10.1007/BF00164784
Fromard F., Puig H., Mougin E., Marty G., Betoulle J. L., Cadamuro L. (1998). Structure, above-ground biomass and dynamics of mangrove ecosystems: new data from French Guiana. Oecologia 115, 39–53. doi: 10.1007/s004420050489
Gerbersdorf S. U., Wieprecht S. (2015). Biostabilization of cohesive sediments: revisiting the role of abiotic conditions, physiology and diversity of microbes, polymeric secretion, and biofilm architecture. Geobiology 13, 68–97. doi: 10.1111/gbi.12115
Gleason S. M., Ewel K. C. (2002). Organic matter dynamics on the forest floor of a Micronesian mangrove forest: An investigation of species composition shifts. Biotropica 34 (2), 190–198. doi: 10.1111/j.1744-7429.2002.tb00530.x
Guibaud G., Comte S., Bordas F., Dupuy S., Baudu M. (2005). Comparison of the complexation potential of extracellular polymeric substances (EPS), extracted from activated sludges and produced by pure bacteria strains, for cadmium, lead and nickel. Chemosphere 59 (5), 629–638. doi: 10.1016/j.chemosphere.2004.10.028
Guo T. F., Zhang Q. M., Ai C., Liang G. Q., He P., Lei Q. L., et al. (2020). Analysis of microbial utilization of rice straw in paddy soil using a DNA-SIP approach. Soil Sci. Soc Am. J. 84, 99–114. doi: 10.1002/saj2.20019
Haas B. J., Gevers D., Earl A. M., Feldgarden M., Ward D. V., Giannoukos G., et al. (2011). Chimeric 16S rRNA sequence formation and detection in Sanger and 454-pyrosequenced PCR amplicons. Genome Res. 21 (3), 494–504. doi: 10.1101/gr.112730.110
Hou W. J., Ma Z. Q., Sun L. L., Han M. S., Lu J. J., Li Z. X., et al. (2013). Extracellular polymeric substances from copper-tolerance Sinorhizobium meliloti immobilize Cu2+. J. Hazard Mater. 261, 614–620. doi: 10.1016/j.jhazmat.2013.06.043
Jiang P. K., Xu Q. F., Xu Z. H., Cao Z. H. (2006). Seasonal changes in soil labile organic carbon pools within a Phyllostachys praecox stand under high rate fertilization and winter mulch in subtropical China. For. Ecol. Manage. 236, 30–36. doi: 10.1016/j.foreco.2006.06.010
Kang F. X., Alvarez P. J., Zhu D. Q. (2014). Microbial extracellular polymeric substances reduce ag+ to silver nanoparticles and antagonize bactericidal activity. Environ. Sci. Technol. 48, 316–322. doi: 10.1021/es403796x
Kang Y. S., Park W. (2010). Protection against diesel oil toxicity by sodium chloride-induced exopolysaccharides in acinetobacter sp. strain DR1. J. Biosci. Bioeng. 109, 118–123. doi: 10.1016/j.jbiosc.2009.08.001
Komiyama A., Ong J. E., Poungparn S. (2008). Allometry, biomass, and productivity of mangrove forests: A review. Aquat. Bot. 89, 128–137. doi: 10.1016/j.aquabot.2007.12.006
Lamont K., Saintilan N., Kelleway J. J., Mazumder D., Zawadzki A. (2019). Thirty-year repeat measures of mangrove above- and below-ground biomass reveals unexpectedly high carbon sequestration. Ecosystems 23, 370–382. doi: 10.1007/s10021-019-00408-3
Li D. H., Ganczarczyk J. J. (1990). Structure of activated sludge flocs. Biotechnol. Bioeng. 35, 57–65. doi: 10.1002/bit.260350109
Lin P. S., Gao Q. Z. (2009). Study on the soil organic carbon storage and vertical distribution of several forest types in mountain area of northeast guangdong. J. Soil Water Conserv. 23 (5), 5. doi: 10.13870/j.cnki.stbcxb.2009.05.035
Lin D., Ma W. T., Jin Z. X., Wang Y. X., Huang Q. Y., Cai P. (2016). Interactions of EPS with soil minerals: A combination study by ITC and CLSM. Colloids Surf. B 138, 10–16. doi: 10.1016/j.colsurfb.2015.11.026
Liu X., Lu X., Zhao W., Yang S., Wang J., Xia H., et al. (2022). The rhizosphere effect of native legume Albizzia julibrissin on coastal saline soil nutrient availability, microbial modulation, and aggregate formation. Sci. Total Environ. 806 (Pt 2), 150705. doi: 10.1016/j.scitotenv.2021.150705
Luo R. Y., Kuzyakov Y., Liu D. Y., Fan J. L., Luo J. F., Lindsey S., et al. (2020). Nutrient addition reduces carbon sequestration in a Tibetan grassland soil: Disentangling microbial and physical controls. Soil Biol. Biochem. 144, 107764. doi: 10.1016/j.soilbio.2020.107764
MacKenzie R. A., Foulk P. B., Klump J. V., Weckerly K., Purbospito J., Murdiyarso D., et al. (2016). Sedimentation and belowground carbon accumulation rates in mangrove forests that differ in diversity and land use: a tale of two mangroves. Wetlands Ecol. Manage. 24, 245–261. doi: 10.1007/s11273-016-9481-3
Magoč T., Salzberg S. L. (2011). FLASH: fast length adjustment of short reads to improve genome assemblies. Bioinformatics 27 (21), 2957–2963. doi: 10.1093/bioinformatics/btr507
Mai Z. M., Ye M., Wang Y., Foong S. Y., Wang L., Sun F., et al. (2021). Characteristics of microbial community and function with the succession of mangroves. Front. Microbiol. 12. doi: 10.3389/fmicb.2021.764974
Mai Z. M., Zeng X., Wei X., Sun C. C., Niu J. W., Yan W. W., et al. (2022). Mangrove restoration promotes the anti-scouribility of the sediments by modifying inherent microbial community and extracellular polymeric substance. Sci. Total Environ. 811, 152369. doi: 10.1016/j.scitotenv.2021.152369
Marasco R., Fusi M., Mosqueira M., Booth J. M., Rossi F., Cardinale M., et al. (2022). Rhizosheath-root system changes exopolysaccharide content but stabilizes bacterial community across contrasting seasons in a desert environment. Environ. Microbiome 17, 14. doi: 10.1186/s40793-022-00407-3
Marcos M. S., Bertiller M. B., Olivera N. L. (2019). Microbial community composition and network analyses in arid soils of the Patagonian Monte under grazing disturbance reveal an important response of the community to soil particle size. Appl. Soil Ecol. 138, 223–232. doi: 10.1016/j.apsoil.2019.03.001
McKew B. A., Dumbrell A. J., Taylor J. D., McGenity T. J., Underwood G. J. C. (2013). Differences between aerobic and anaerobic degradation of microphytobenthic biofilm-derived organic matter within intertidal sediments. FEMS Microbiol. Ecol. 84 (3), 495–509. doi: 10.1111/1574-6941.12077
Neoh C. H., Yung P. Y., Noor Z. Z., Razak M. H., Aris A., Md Din M. F., et al. (2017). Correlation between microbial community structure and performances of membrane bioreactor for treatment of palm oil mill effluent. Chem. Eng. J. 308, 656–663. doi: 10.1016/j.cej.2016.09.063
Phang V. X. H., Chou L. M., Friess D. A. (2015). Ecosystem carbon stocks across a tropical intertidal habitat mosaic of mangrove forest, seagrass meadow, mudflat and sandbar. Earth Surf. Processes Landforms 40, 1387–1400. doi: 10.1002/esp.3745
Pierre G., Zhao J. M., Orvain F., Dupuy C., Klein G. L., Graber M., et al. (2014). Seasonal dynamics of extracellular polymeric substances (EPS) in surface sediments of a diatom-dominated intertidal mudflat (Marennes–oléron, France). J. Sea Res. 92, 26–35. doi: 10.1016/j.seares.2013.07.018
Quast C., Pruesse E., Yilmaz P., Gerken J., Schweer T., Yarza P., et al. (2013). The SILVA ribosomal RNA gene database project: improved data processing and web-based tools. Nucleic Acids Res. 41 (D1), D590–D596. doi: 10.1093/nar/gks1219
Romigh M. M., Davis S. E., Rivera-Monroy V. H., Twilley R. R. (2006). Flux of organic carbon in a riverine mangrove wetland in the Florida coastal Everglades. Hydrobiologia 569, 505–516. doi: 10.1007/s10750-006-0152-x
Santaella C., Schue M., Berge O., Heulin T., Achouak W. (2008). The exopolysaccharide of Rhizobium sp. YAS34 is not necessary for biofilm formation on Arabidopsis thaliana and Brassica napus roots but contributes to root colonization. Environ. Microbiol. 10, 2150–2163. doi: 10.1111/j.1462-2920.2008.01650.x
Smith P. K., Krohn R. I., Hermanson G. T., Mallia A. K., Gartner F. H., Frovenzano M. D., et al. (1985). Measurement of protein using bicinchoninic acid. Anal. Biochem. 150, 76–85. doi: 10.1016/0003-2697(85)90442-7
Takahashi E., Ledauphin J., Goux D., Orvain F. (2009). Optimising extraction of extracellular polymeric substances (EPS) from benthic diatoms: comparison of the efficiency of six EPS extraction methods. Mar. Freshw. Res. 60, 1201–1210. doi: 10.1071/MF08258
Tian Y., Yan C. L., Wang Q., Ma W., Yang D., Liu J. C., et al. (2020). Glomalin-related soil protein enriched in δ13C and δ15N excels at storing blue carbon in mangrove wetlands. Sci. Total Environ. 732, 138327. doi: 10.1016/j.scitotenv.2020.138327
Urrea J. L., Collado S., Oulego P., Díaz M. (2017). Formation and degradation of soluble biopolymers during wet oxidation of sludge. ACS Sustain. Chem. Eng. 5, 3011–3018. doi: 10.1021/acssuschemeng.6b02664
Wang Y. S., Gu J. D. (2021). Ecological responses, adaptation and mechanisms of mangrove wetland ecosystem to global climate change and anthropogenic activities. Int. Biodeterior. Biodegrad. 162, 105248. doi: 10.1016/j.ibiod.2021.105248
Wang G., Guan D. S., Peart M. R., Chen Y. J., Peng Y. S. (2013). Ecosystem carbon stocks of mangrove forest in yingluo bay, guangdong province of south China. For. Ecol. Manage. 310, 539–546. doi: 10.1016/j.foreco.2013.08.045
Wang G., Guan D. S., Xiao L., Peart M. R., Zhang H., Singh M. (2019a). Changes in mangrove community structures affecting sediment carbon content in yingluo bay of south China. Mar. pollut. Bull. 149, 110581. doi: 10.1016/j.marpolbul.2019.110581
Wang Q., Lu H. L., Chen J. Y., Hong H. L., Liu J. C., Li J. W., et al. (2018). Spatial distribution of glomalin-related soil protein and its relationship with sediment carbon sequestration across a mangrove forest. Sci. Total Environ. 613-614, 548–556. doi: 10.1016/j.scitotenv.2017.09.140
Wang Q., Mei D., Chen J., Lin Y., Liu J., Lu H., et al. (2019b). Sequestration of heavy metal by glomalin-related soil protein: Implication for water quality improvement in mangrove wetlands. Water Res. 148, 142–152. doi: 10.1016/j.watres.2018.10.043
Wei Q., Zhang F., Richardson M. M., Roy N. H., Rodgers W., Liu Y. C., et al. (2014). CD82 restrains pathological angiogenesis by altering lipid raft clustering and CD44 trafficking in endothelial cells. Circulation 130 (17), 1493–1504. doi: 10.1161/CIRCULATIONAHA.114.011096
Wilén B. M., Keiding K., Nielsen P. H. (2000). Anaerobic deflocculation and aerobic reflocculation of activated sludge. Water Res. 34, 3933–3942. doi: 10.1016/S0043-1354(00)00274-8
Wingender J., Neu T. R., Flemming H. C. (1999). Microbial extracellular polymeric substances (Berlin, Heidelberg: Springer).
Wu P., Xiong X. F., Xu Z. Z., Lu C. Q., Cheng H., Lyu X. L., et al. (2016). Bacterial communities in the rhizospheres of three mangrove tree species from beilun estuary, China. PLos One 11, e0164082. doi: 10.1371/journal.pone.0164082
Xiao R., Zheng Y. (2016). Overview of microalgal extracellular polymeric substances (EPS) and their applications. Biotechnol. Adv. 34 (7), 1225–1244. doi: 10.1016/j.biotechadv.2016.08.004
Xiong Y. M., Liao B. W., Wang F. M. (2018). Mangrove vegetation enhances soil carbon storage primarily through in situ inputs rather than increasing allochthonous sediments. Mar. pollut. Bull. 131, 378–385. doi: 10.1016/j.marpolbul.2018.04.043
Xu L., Huo M. X., Sun C. Y., Cui X. C., Zhou D. D., Crittenden J. C., et al. (2017). Bioresources inner-recycling between bioflocculation of microcystis aeruginosa and its reutilization as a substrate for bioflocculant production. Sci. Rep. 7, 43784. doi: 10.1038/srep43784
Xu X. F., Thornton P. E., Post W. M. (2013). A global analysis of soil microbial biomass carbon, nitrogen and phosphorus in terrestrial ecosystems. Global Ecol. Biogeogr. 22, 737–749. doi: 10.1111/geb.12029
Yang L. H., Xiao S. R., Luan T. G., Tam N. F. Y. (2018). Overproduction of microbial extracellular polymeric substances in subtropical intertidal sediments in response to endocrine disrupting chemicals. Sci. Total Environ. 624, 673–682. doi: 10.1016/j.scitotenv.2017.12.160
Yang L. H., Xiao S. R., Yang Q., Luan T. G., Tam N. F. Y. (2020). Recovery of subtropical coastal intertidal system prokaryotes from a destruction event and the role of extracellular polymeric substances in the presence of endocrine disrupting chemicals. Environ. Int. 144, 106023. doi: 10.1016/j.envint.2020.106023
Yu L., Bai J., Huang L., Zhang G., Wang W., Wang X., et al. (2022). Carbon-rich substrates altered microbial communities with indication of carbon metabolism functional shifting in a degraded salt marsh of the yellow river delta, China. J. Cleaner Prod. 331, 129898. doi: 10.1016/j.jclepro.2021.129898
Zhang G., Bai J., Zhao Q., Jia J., Wang W., Wang X. (2020). Bacterial succession in salt marsh soils along a short-terminvasion chronosequence of Spartina alterniflora in the yellow river estuary, China. Micro. Eco. 79 (3), 644–661. doi: 10.1007/s00248-019-01430-7
Zhang M. J., Liao B. Q., Zhou X. L., He Y. M., Hong H. C., Lin H. J., et al. (2015b). Effects of hydrophilicity/hydrophobicity of membrane on membrane fouling in a submerged membrane bioreactor. Bioresour. Technol. 175, 59–67. doi: 10.1016/j.biortech.2014.10.058
Zhang J., Tang X. L., He X. H., Liu J. X. (2015a). Glomalin-related soil protein responses to elevated CO2 and nitrogen addition in a subtropical forest: Potential consequences for soil carbon accumulation. Soil Biol. Biochem. 83, 142–149. doi: 10.1016/j.soilbio.2015.01.023
Keywords: mangroves, mangrove zonation, extracellular polymeric substances, organic carbon, microbial community
Citation: Liu D-X, Mai Z-M, Sun C-C, Zhou Y-W, Liao H-H, Wang Y-S and Cheng H (2022) Dynamics of extracellular polymeric substances and soil organic carbon with mangrove zonation along a continuous tidal gradient. Front. Mar. Sci. 9:967767. doi: 10.3389/fmars.2022.967767
Received: 13 June 2022; Accepted: 25 October 2022;
Published: 17 November 2022.
Edited by:
Zhanfei Liu, University of Texas at Austin, United StatesReviewed by:
Shuting Liu, Kean University, United StatesAliya Naz, O. P. Jindal Global University, India
Hualong Hong, Xiamen University, China
Copyright © 2022 Liu, Mai, Sun, Zhou, Liao, Wang and Cheng. This is an open-access article distributed under the terms of the Creative Commons Attribution License (CC BY). The use, distribution or reproduction in other forums is permitted, provided the original author(s) and the copyright owner(s) are credited and that the original publication in this journal is cited, in accordance with accepted academic practice. No use, distribution or reproduction is permitted which does not comply with these terms.
*Correspondence: You-Shao Wang, eXN3YW5nQHNjc2lvLmFjLmNu; Hao Cheng, Y2hlbmdoYW9Ac2NzaW8uYWMuY24=
†These authors have contributed equally to this work