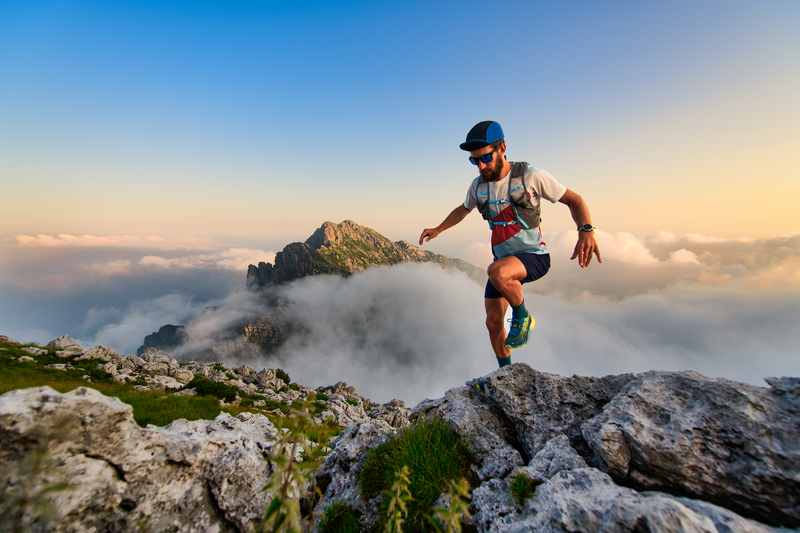
95% of researchers rate our articles as excellent or good
Learn more about the work of our research integrity team to safeguard the quality of each article we publish.
Find out more
ORIGINAL RESEARCH article
Front. Mar. Sci. , 28 July 2022
Sec. Marine Biotechnology and Bioproducts
Volume 9 - 2022 | https://doi.org/10.3389/fmars.2022.957217
This article is part of the Research Topic Boosting the Potential of Algae for Biomass Production, Valorisation, and Bioremediation View all 9 articles
This study raises the question of the potential long-term effects of a feeding pulse with diets containing algal biomass on the quality of frozen turbot (Scophthalmus maximus) fillets. A feeding trial was carried out with this aim, in which juvenile turbot were fed during 60 d with six different experimental diets, two of which were enriched with the macroalgae Ulva rigida (U diets), the other two with a microalgae blend (M diets), plus a non-supplemented control diet (CT), and finally, a commercial aquafeed (COM). Two inclusion levels were considered in algae-enriched diets (50 and 100 g kg-1) so that they were designed as U5 and U10 for U. rigida, and M5 and M10 for microalgae-enriched diets. The duration of the feeding pulse with the functional diets was 60 days, and then, all specimens were fed with a COM diet for a further 150-d period until reaching a body size close to the commercial standard (approximately 750 g). Subsequently, fish were sacrificed; fillets were removed from the pigmented flank and kept at -20 °C for 5 months. Then, fillets were thawed and the muscle proximal composition and fatty acid profile were analyzed, and fillet quality parameters were assessed during a 10-d co\ld storage period at 4°C. The possible deferred effects of algae-enriched diets on fillet quality were compared with a control diet without algae (CT) and with a commercial diet (COM). Roughly, the results obtained indicated that a 60-d administration pulse of algae-enriched diets induced some long-term changes in both the nutritional and organoleptic quality of turbot fillets. In this regard, the algae-enriched aquafeeds improved the muscle antioxidant response throughout the cold storage period, prolonging the shelf life of thawed turbot fillets. In addition, in terms of consumers’ acceptability, this feeding pulse on pre-growing stage of the productive cycle was able to modify the skin and muscle pigmentation of fish of commercial size. Namely, the U10 diet increased the protein content, improved muscle texture, reduced fillet total lipid content, and favored muscle selective retention of n-3 polyunsaturated fatty acids. On the other hand, the strategy based on the early administration of a diet rich in fishmeal (CT) for 60 d, followed by feeding with commercial feed, also affected positively the quality of fillets, with values comparable to U10 specimens. Thus, the results obtained indicate that a two-month feeding pulse with algae-supplemented diets during pre-growing phase may represent a promising strategy to improve the nutritional and organoleptic quality of frozen turbot fillet, as well as to extend their shelf life during the post-thawing cold storage.
Fillet quality attributes and commercial shelf life not only rely on fish species-specific factors but also on the utilization of commercial additives aimed at delaying fish deterioration throughout cold storage (Kumar et al., 2015). Both microbiological and biochemical phenomena jeopardize the quality of the final product, and therefore, they represent the target for most food additives (Nakazawa and Okazaki, 2020). However, the utilization of chemically synthesized substances is restricted not only by legal regulations but also by the low acceptability of additives by consumers. The consequence is that a scarce array of such preservatives is available for the seafood industry, whereas novel processed fish formats are increasingly present in the market, not least those involving extended storage periods. In this regard, frozen fish involve long-term storage, in which biochemical deterioration is still possible. Namely, lipid peroxidation and protein degradation are among the most known phenomena that can alter the quality of frozen fish (Erkan and Yeşiltaş, 2014). Scarce research has been conducted aimed at controlling oxidative deterioration in frozen fish based on dietary strategies, and therefore, there is plenty of room for innovation in this field. The utilization of functional aquafeeds enriched with natural bioactive additives included at a low percentage (less than 10%) represents a promising strategy in this context.
Among the potential additives with this purpose, plant extracts, prebiotics, and algae stand out, all of them with potentially valuable physiological effects on fillet pigmentation or lipid oxidation (Bharathi et al., 2019). In this context, algae (macro- and microalgae) are rich in a wide variety of bioactive compounds, such as carotenoids and polyphenols among others, which provide remarkable antioxidant and antimicrobial activities (Ghosh et al., 2022). Thus, several studies have pointed out the potential of algae as natural preservatives for fish products (Halldorsdottir et al., 2014; Sáez et al., 2021).
On the other hand, turbot (Scophthalmus maximus) is emerging as a suitable candidate when it comes to diversifying fish species in Southern European aquaculture. Owing to their leanness, this flatfish species can be marketed both as fresh or frozen, since a high lipid content is regarded as a major constraint for frozen storage. To date, the potential effects of functional aquafeeds on objective quality parameters in turbot remains virtually unexplored.
The so-called “nutraceutical” aquafeeds would be indicated to be administered at the end of the production cycle (finishing feeds), given that dietary changes are rapidly reflected in fillet quality parameters (Grigorakis et al., 2002; Suárez et al., 2010). However, the inclusion of such supplements in finishing diets (a period in which huge amounts of feeds are consumed on farm), might be questionable so that increased feeding costs could outweigh any potential improvement in the quality attributes of fish. Therefore, an alternative strategy virtually unexplored might involve the utilization of feeds supplemented with additives just at earlier stages of the production cycle (namely, juvenile fish), with the aim of further obtaining certain valuable characteristics in fish once the commercial size is reached. This is, a sort of “deferred effect” of functional feeds after discontinuing the administration, which is expected to persist at later stages of the production cycle, would reduce feeding costs since a less amount of feed is required at early periods of the production cycle. This kind of effect has been suggested recently (Sáez et al., 2020).
In this context, this study explores whether a dietary pulse with aquafeeds enriched with an Ulva rigida meal or a blend of microalgae at a low inclusion level during a 60-d period in the pre-fattening stage (60 d) could influence the quality parameters of frozen fillets obtained from the turbots of commercial size, as well as modify the extension of their shelf life after thawing.
A blend of freshwater and marine microalgae provided by the company LifeBioencapsulation S.L. (Spin-off; Universidad de Almerıá, Almería, Spain) was used. U. rigida was cultivated at the facilities of Planta de Cultivos Marinos El Bocal (Instituto Español de Oceanografía-CSIC, Santander, Spain), in an outdoor closed system equipped with 1500 L-horizontal semicylindrical tanks with aeration. Ulva biomass was grown in natural seawater enriched with an f/2 medium and 1.8-mM nitrate and 0.1-mM phosphate, under a natural photoperiod. Seaweed was harvested, rinsed with tap water, dried at 30°C, and stored in a cold chamber.
Five isonitrogenous (50.5% crude protein on a dry-weight basis, DW) and isolipidic (16.5% crude lipid DW) experimental feeds were elaborated; two of them contained 50 and 100 g kg-1 of a microalgae mixture (designated as M5 and M10, respectively); the other two included 50 and 100 g kg-1 of a U. rigida meal (U5 and U10, respectively), and a fifth diet, algae-free, was used as a control batch (CT). In addition, a commercial feed (COM), specific for this species, was also included in the feeding trial. The proximal composition and fatty acid profiles of all diets are shown in Tables 1, 2, respectively.
Experimental diets were manufactured at Ceimar-Universidad de Almería facilities (Servicio de Piensos Experimentales, http://www.ual.es/stecnicos_spe) (Almeria, Spain) using standard aquafeed processing procedures. Briefly, feed ingredients and algal biomass were mixed together, and then, water was added to the mixture (up to 300 g kg-1) to make up homogeneous dough in a vertical helix ribbon mixer (Sammic BM-10, Sammic, Azpeitia, Spain). The dough was passed through a single-screw laboratory extruder (Miltenz 51SP; JSConwell Ltd, Palmerston North, New Zealand) to obtain 5-mm-diameter pellets. The extruder barrel consisted of four sections and the temperature profile. In each segment (from the inlet to outlet) was 90°C, 92°C, 95°C, and 105°C, respectively. The feeds were dried (30°C, 24 h) in a 12-m3 drying chamber with forced-air circulation (Airfrio, Almería, Spain), and stored at -20°C until use.
The feeding trial was carried out at the aquaculture facilities of Planta de Cultivos Marinos El Bocal (Santander, Spain), Instituto Español de Oceanografía (IEO-CSIC). All experimental procedures complied with the Guidelines of the European Union (Directive 2010/63/EU) and the Spanish regulations (Real Decreto 53/2013, as amended by RD 118/2021) on the protection of laboratory animals. A total of 306 turbot juveniles (26.38±7.55 g initial body weight) were randomly distributed in 500-L tanks (triplicate tanks per dietary treatment), but only 180 specimens were withdrawn for this study (6 dietary treatments × 3 tanks per treatment × 10 fish per tank).
Fish were fed with a commercial feed (COM diet) during a 30-d acclimation period prior to the beginning of the feeding trial. Afterwards, the experimental diets were offered at a 4% feeding rate twice per day until triplicating the initial body weight. The feeding trial was carried out in an open-flow circuit, keeping the seawater renewal rate (35‰ salinity) at 500 L h−1 and ammonia and nitrite values (<0.1 mg L-1) suitable for turbot culture. Animals were kept under a 16:8 (light:dark) photoperiod, and the water temperature was kept at 18.4±1.2°C. Light intensity ranged from 100 to 150 lux. Tanks were equipped with aerators to maintain an adequate level of oxygenation (above 6 mg L-1).
After the acclimation period, experimental diets were administrated for 60 days using the same feeding protocol. Afterwards all specimens were fed with COM diet for a further 150-d period, until reaching a size close to the commercial standard (approximately 750-g average body weight). Subsequently, 10 fish per tank (30 animals per dietary treatment) were withdrawn, individually weighed and measured, and killed by anesthetic overdose (200 mg L-1 clove oil; isoeugenol) followed by spine severing. Animals were photographed, and fillets were removed immediately from the pigmented flank (the skin was kept), packed in transparent sterile polyethylene bags, and stored at -20°C for 5 months. After that period, fillets were thawed and the muscle proximal composition and fatty acid profile were analyzed. With the aim of assessing changes in quality parameters throughout the shelf life in cold storage (4°C), samples were taken at each of the following sampling times: 1, 3, 6, 8, and 10 days (six fillets per dietary treatment and sampling time). Lipid oxidation, texture profile analysis (TPA), pH, water- holding capacity (WHC), and skin and flesh pigmentation were determined.
The proximate analysis (dry matter, ash, and crude protein, Kjeldahl N × 6.25) of aquafeeds and muscle samples were determined according to AOAC (2000) protocols. Lipids were extracted following Folch (1957) methodology using chloroform/methanol (2:1 v/v) as a solvent, and the total lipid content was calculated gravimetrically. The fatty acid profiles of diets and muscle samples were determined by gas chromatography (Hewlett Packard, 4890 Series II, Hewlett Packard Company, Avondale, PA, USA) according to Rodríguez-Ruiz et al. (1998), using a modification of the direct transesterification method described by Lepage and Roy (1984) that requires no prior separation of the lipid fraction. From the data on the FA profile, the atherogenicity index (AI) and the thrombogenicity index (TI) were calculated according to Senso et al. (2007). The value of flesh lipid quality (FLQ), which indicates the ratio of EPA and DHA to total FAs, expressed as percentage, was determined as indicated in Suárez et al. (2015).
Fillet lipid oxidation was assessed by thiobarbituric acid–reactive substances (TBARS) analysis, according to Buege and Aust (1978). Briefly, muscle samples (5 g each) were homogenized in 4 ml of 50 mM NaH2PO4, 0.1% (v/v) Triton X-100 solution. The mixture was centrifuged (10,000 g, 20 min, 4°C) and supernatants were mixed in a 1:5 (v/v) ratio with a 2-thiobarbituric acid (TBA) reagent [0.375% w/v TBA, 15% w/v trichloroacetic acid (TCA), 0.01% w/v 2,6-dibutyl hydroxytoluene, BHT, and 0.25 N HCl]. The mixture was heated (100°C) for 15 min, then centrifuged (3,600 g, 10 min, 4°C), and the absorbance of supernatants was measured at 535 nm. The amount of TBARS was expressed as milligrams of malonyl dialdehyde (MDA) per kilogram of muscle after comparing with the MDA standard.
The texture of fillets obtained from the pigmented flank was measured by the compression of the anterior area to the dorsal fin, above the lateral line of fillets using a Texture Analyzer (TXT2 plus “Stable Micro System”), equipped with a load cell of 5 kN, controlled with Texture Expert Exceed 2.52 software (Stable Micro Systems, Surrey, England). Fillet samples were subjected to two consecutive cycles of 25% compression, with 5 s between cycles, in which a 20-mm cylindrical probe was used for pressing downwards into the fillet at a constant speed of 1 mm/s. The textural parameter hardness (maximum force required to compress the sample), springiness (ability of the sample to recover its original form after removing the deforming force), cohesiveness (extent to which the sample could be deformed prior to rupture), gumminess (force needed to disintegrate a semisolid sample to a steady state of swallowing), chewiness (the work needed to chew a solid sample to reach a steady state of swallowing), and resilience (how well a product fights to regain its original position) were calculated as described in Bourne (1978).
Flesh pH was determined in the dorsal muscle by means of a penetration electrode (Crison, model GLP 21; sensitivity 0.01 pH units) as described in Suárez et al. (2010). The water-holding capacity (WHC; expressed in percentage) was calculated from the small cubes (1 cm3) of the anterior part of the dorsal muscle as the difference between the initial percentage of water and the percentage of water released after centrifugation, as detailed in Suárez et al. (2010).
Color was measured on the skin and muscle sides of fillets (three independent determinations on each side and sampling time) by the L*, a*, and b* system (CIE, 1986), using a Minolta Chroma meter CR400 device (Minolta, Osaka, Japan). The parameter brightness (L*, on a 0–100-point scale from black to white), redness–greenness (a*, estimates the position between red, positive values, and green, negative values), and yellowness (b*, estimates the position between yellow, positive values, and blue, negative values) were determined.
The effect of the categorical variables “diet,” “storage time,” “additive,” and “dose” as well as their interactions were determined for each numeric parameter studied by fitting a generalized lineal statistical model (GLM analysis) that relates measured parameters to predictive factors, using specific software (SPSS 22, IBM Corporation Inc.). Least squares means were tested for differences using Fisher’s least significant difference (LSD) procedure. Unless otherwise is specified, a significance level of 95% was considered to indicate statistical difference (P < 0.05). When measurements were expressed as a percentage (e.g., fatty acids profile), the arcsine transformation of their square root was carried out in order to normalize data prior to the statistical analysis.
Results obtained indicated that a 60-d discontinued administration with algae-enriched diets in prefattening phase induced some long-term changes in the fillet chemical composition (Table 3). Although considering data as a whole, the variables “additive” (microalgae or U. rigida) and “dose” (5% and 10%) did not affect this parameter; nevertheless, specimens fed on CT and U10 diets showed increased muscle protein content, while the lowest values for protein were found in the M10 treatment (P=0.004). Significantly higher lipid values were found in M10 and U5 batches compared to CT and U10 lots (P < 0.001). In this case, the algae source influenced muscle lipid contents, with higher values in specimens fed with microalgae-enriched diets (P=0.021), regardless of the dose administered (M5 or M10). Ash and moisture contents were not influenced by dietary treatments.
Table 3 Effects of the dietary inclusion of algae-enriched additives on the muscle proximal composition of Scophthalmus maximus thawed fillets (% fresh weight).
With regard to the fatty acid (FA) profile (Table 4), roughly, certain effects were observed, owing to the early-state algae supplementation. Specifically, CT and U10 fillets showed increased saturated (ΣSFA) and polyunsaturated (ΣPUFA) contents, whereas the lowest values for both categories were found in the M10 batch. Conversely, M10 fillets yielded higher values of monounsaturated (ΣMUFA) fatty acids, particularly of 16:1n7 and 18:1n9. SFA and MUFA contents were also influenced by the source of algal biomass, and thus Ulva-enriched diets increased the relative values of SFAs (P=0.009), whereas microalgae-containing diets caused higher values of MUFAs (P= 0.047).
Table 4 Effects of the dietary treatments on the fatty acid (FA) profile of turbot thawed fillets (% of total FAs).
Regarding PUFAs, and in particular the n3-PUFA series, the highest values were obtained in U10 fillets, whereas in the case of n6-PUFA, no significant differences were observed among dietary treatments. Roughly, the feeding pulse with U10 and CT diets caused a long-term effect on the fillet n3/n6 ratio (P < 0.001). EPA and DHA contents were significantly higher in CT and U10 samples (P < 0.001), both experimental batches also yielding a higher lipid quality index (FLQ) than the rest of groups. On the contrary, the lowest values for this parameter were found in the M10 lot. The rest of lipid-related indices (AI and TI) were not affected by algae-enriched diets at the end of the turbot production cycle.
Changes in TBARS contents observed in defrosted turbot fillets during cold storage at 4°C are shown in Figure 1. Roughly, muscle lipid oxidation indicated differences attributable to the factors dietary treatment, storage time, and their interactions. However, neither additive source (P = 0.356) nor dosage (P = 0.587) influenced the oxidative status of muscle lipids. TBARS content increased markedly over time in all the experimental lots (P < 0.01); however, from the beginning of the post-thawing period, the highest values for this parameter were found in fish fed with a COM diet (P < 0.01) consistently throughout the complete period. On the other hand, all the algae-enriched diets, irrespectively of the inclusion level, were responsible for fillets with a significantly higher antioxidant response than CT from day 6 onwards (P < 0.01).
Figure 1 Time course of muscle lipid oxidation [estimated as the thiobarbituric acid-reactive substances (TBARS) content] of defrosted turbot fillets throughout a 10-d cold (4°C) storage period. CT: algae-free control diet; M5 and M10 stand for a microalgae blend included at 5% and 10%, respectively. U5 and U10: diets containing 5% and 10% Ulva rigida, respectively; COM: commercial aquafeed. Values are expressed as average±standard deviation (SD) (n = 6).
The effects of the dietary treatments on fillet textural parameters are summarized in Figure 2 and Table 5. Overall, diets and the storage time contributed to changes in hardness, cohesiveness, gumminess, chewiness, and resilience parameters. The additive source (microalgae vs. U. rigida) caused changes in cohesiveness, gumminess, chewiness, and resilience parameters, while cohesiveness and resilience attributes showed a dose-dependent effect. Concerning fillet hardness (Figure 2), COM fillets yielded lower values for this parameter during the complete post-thawing storage (although differences were not significant at 10 days post-thawing) compared with the rest of dietary treatments, especially with CT, U10, and M5 batches (P < 0.01). Fillet hardness decreased in all the experimental lots throughout the post-thawing cold storage (P<0.01), although differences with respect to initial values did not reach statistical significance in M5, M10, and U5 lots (P = 0.324, 0.657, and 0.521, respectively). Roughly, gumminess and chewiness displayed a tendency similar to that observed for hardness, whereas figures for cohesiveness and resilience were generally higher (with some exceptions) in specimens fed with U5 diet feeding-pulse. Finally, neither diet nor fillet storage time caused a long-term impact on fillet springiness.
Figure 2 Time course of the hardness of defrosted turbot fillets throughout a 10-d cold (4°C) storage period. CT: algae-free control diet; M5 and M10 stand for a microalgae blend included at 5% and 10%, respectively. U5 and U10: diets containing 5% and 10% U. rigida, respectively; COM: commercial aquafeed. Values are expressed as average±SD (n=6).
Table 5 Changes in texture profile analysis parameters in turbot thawed fillets during a 10-d cold storage (4°C) period.
With regard to muscle pH, dietary treatments and the storage time were responsible for significant differences in this parameter (P < 0.01 and P < 0.01, respectively, Figure 3). On the other hand, neither the algal source nor the dosage had an impact on fillet pH values (P = 0.709 and 0.240, respectively). The administration of M10 and COM diets during the pre-fattening stage resulted in significantly higher muscle pH values up to 6 days post-thawing, whereas CT fish yielded the lowest pH values up to day 6 of the storage period. On the other hand, WHC measured in fillets during the post-thawing period (Figure 2B) showed no differences attributable to any of the variables studied (diet, storage time, additive source, dose, and their interactions).
Figure 3 Time course of changes in pH (A) and WHC (B) of defrosted turbot fillets during a 10-d cold storage period. CT: algae-free control diet; M5 and M10 stand for a microalgae blend included at 5% and 10%, respectively. U5 and U10: diets containing 5% and 10% U. rigida, respectively; COM: commercial aquafeed. Values are expressed as average±SD (n = 6).
With regard to instrumental color determinations (Figure 4), a certain long-term influence of the dietary treatments was observed for the different color parameters measured on both sides (skin and flesh) of turbot fillets. Thus, fillets from the ocular (pigmented) side showed significant differences during the cold storage period for the parameters a* and b* attributable to the factors dietary treatment, dose, and additive source. Roughly, specimens temporarily fed with algae-containing diets showed higher greenness (lower a*) and higher yellowness (higher b*) than animals fed with CT and COM fillets. No changes in lightness (L*) could be attributed to diet, storage time, or additive dosage. Fillet brightness was influenced only by the algal source, yielding U5 and U10 lots overall higher values (P = 0.045) than the rest of batches.
Figure 4 Time course of changes in L* (A), a* (B), and b* (C) skin color parameters of defrosted turbot fillets during a 10-d cold storage period. CT: algae-free control diet; M5 and M10 stand for a microalgae blend included at 5% and 10%, respectively. U5 and U10: diets containing 5% and 10% U. rigida, respectively; COM: commercial aquafeed. Representative images of turbot specimens (D) subjected to the different experimental diets are shown. Values are expressed as average±SD (n = 6).
With regard to the a* parameter, M10 and U10 fillets showed higher greenness skin pigmentation after 6 days post-thawing, this measurement being influenced by the additive dosage (the higher the dose, the lower the a* values, P = 0.02) and additive source (lower values observed for fish fed with U. rigida-enriched diet, P <0.01). On the other hand, the post-thawing storage time was responsible for the loss of the greenish skin tone in all batches, this decrease being significant in those specimens fed with CT, M5, and COM diets. The parameter b* was also affected by all the variables studied (storage period, additive source, and dose). Overall, the dietary pulse with algae-enriched diets increased b* values in turbot skin, this rise being dose-dependent (P = 0.031) and higher for U5 and U10 diets (P = 0.002). In addition, the storage time reduced b* values in all the batches, but differences were significant only in CT, M5, and COM batches.
On the other hand, the flesh (muscle) side of turbot fillets was also influenced by the experimental factors considered (Figure 5). In this context, changes in L* values did not follow a clear tendency attributable to the long-term effects of the dietary treatments, although some significant differences were observed among the different groups. Roughly, flesh brightness tended to increase over the storage time, but no solid effects could be observed among groups. The parameter a* showed negative values for all batches, indicating a slight greenish coloration in the turbot muscle, which increased significantly during the post-thawing period. Among dietary treatments, roughly, the higher the dose tested, the higher the muscle color (P < 0.001). Comparing microalgae vs. U. rigida (Figure 5B), no impact was observed on a* values. Finally, negative values (slightly bluish tones) were observed for the parameter b* (Figure 5C) at the initial sampling point, which turned to positive values (slightly yellowish) after 6 days post-thawing in all the experimental groups. With some exceptions, no clear differences attributable to diet were observed, although b* values tended to be lower for fish fed with algae-enriched diets compared to the COM lot. On the other hand, neither the variables additive nor dose influenced this color parameter (P = 0.548 and 0.457, respectively).
Figure 5 Time course of changes in L* (A), a* (B), and b* (C) flesh color parameters of defrosted turbot fillets during a 10-d cold storage period. CT: algae-free control diet; M5 and M10 stand for microalgae blend included at 5% and 10%, respectively. U5 and U10: diets containing 5% and 10% U. rigida, respectively; COM: commercial aquafeed. Values are expressed as average±SD (n = 6).
Turbot (S. maximus) is a marine flatfish from European coastal waters with a high commercial value (Cai et al., 2015), appreciated for the lean, white, and firm flesh, with an excellent aptitude for industrial processing and freezing. The demand of turbot has been increasing in the last decade, and the market is supplied by both fishery and fish farming. Traditionally, turbot was sold as whole fish, but currently, turbot fillets, either fresh or frozen, have become a more convenient product, this also enhancing the added value of the species. Frozen storage, compared to other long-term preservation strat-gies, yields an excellent balance between the prolongation of the storage time (this also enables long haul transportation), and the persistence of the physicochemical and organoleptic characteristics of fish, in such a manner that quality differences between fresh and frozen fish are nowadays becoming less and less pronounced.
However, during frozen storage, some chemical deterioration remains uninterrupted, not least that related to fish lipid and protein fractions. Among the strategies available aimed at preventing this chemical degradation, the most widespread are represented by the utilization of food additives, not least, antioxidants. However, consumers’ aptitude against chemically synthesized additives is encouraging the search for natural alternatives. In this regard, algae (both macro and microalgae) emerge as valuable, but yet almost unexplored, sources of bioactive compounds with theoretical potential to reduce the quality losses of fish when added as food additives (Sáez et al., 2021). In this scenario, a novel strategy consists of the inclusion of algae bioactive compounds with potential effects on fish quality as feed additives, instead of using them as food additives. In other words, the aim is the use of algae-enriched aquafeeds with the purpose of influencing the quality of fish “from within the animals.” Most of the studies performed in this regard have dealt with the short-term effects of finishing diets at the end of the fattening period on the characteristics of fish (Teimouri et al., 2013; Moroney et al., 2015; Moroney et al., 2016; Valente et al., 2016). However, it has recently been reported (Sáez et al., 2020) that a feeding pulse at earlier stages of the production cycle (namely, pre-fattening) can also influence fillet quality, at least in some flatfish species. Interestingly, even if the administration had been discontinued, certain effects remained at latter stages of the production cycle in that study.
It is well known that feeding plays a key role in the quality of fillets (Matos et al., 2017), owing to the fact that variations in diet are very quickly reflected in the proximal composition of farmed fish (Suárez et al., 2010). In agreement, the inclusion of algae as a feed additive (less than 10% inclusion level) in aquafeeds affects muscle proximal composition (Wan et al., 2017). Short-term supplementation with Ulva sp. increased the muscle protein content in tilapia (Oreochromis niloticus; Azaza et al., 2008; Ergun et al., 2008) and rainbow trout (Oncorhynchus mykiss; Yildirin et al., 2009). The results found in this study (Table 3) also point to such effects, especially for the U10 diet, but with the caveat that in our case, the supplementation did not take place close to slaughtering but at an earlier stage of rearing (for just 60 d during the pre-fattening stage). Since this effect was not observed for COM or U5 specimens, it also suggests a certain dose-dependent effect.
With regard to microalgae, the results obtained showed a higher protein content in M5 fillets compared to the M10 batch, which indicate, on the contrary, a lack of dose-dependent effect difficult to explain. Nevertheless, the literature is inconclusive in this respect, and thus, a 5% dietary inclusion of Nannochloropsis gaditana did not modify the muscle composition in turbot (Qiao et al., 2019), Senegalese sole (Vizcaíno et al., 2018), and gilthead seabream (Sales et al., 2021; Sáez et al., 2022) juveniles, but others have reported an increased muscle protein content attributable to microalgae supplementation (Galafat et al., 2020; Perera et al., 2020).
On the other hand, it is worth mentioning that CT fillets (fed with an algae-free diet designed in our laboratory) showed the highest protein content with respect to those fed during the entire feeding trial with COM feed, this fact suggesting that there is still room for improvement in the design of specific diets for this species. A possible explanation for these differences between both algae-free diets would be related to the digestive bioavailability of dietary protein (both diets were isonitrogenous), which is higher for fishmeal (abundant in the CT diet) than for plant protein sources (although not completely known, it is assumed that COM outweighs CT in this regard). Not only in this case, but likely in others involving differences in muscle composition, a dissimilar bioavailability of nutrients among macro- and microalgae sources might account for such dissimilarities, a fact that introduces the need to evaluate at a species-specific level their digestibility and bioavailability (Shah et al., 2018).
Concerning the lipid content, all batches showed values less than 3.5% (fresh matter), within the range for lean fish species such as turbot (Grigorakis, 2017). However, some long-term effects on lipids were observed due to the discontinued feeding with the experimental diets (Table 3). In this sense, lipid deposition in muscle was higher in fish fed with the M10 diet, in agreement with the results described by Abdel-Tawwab and Ahmad (2009) and Perera et al. (2020) for microalgae-containing diets. However, a contrasting effect was observed in U10-thawed fillets, which, together with CT group, yielded a low muscle lipid content. Results in this line have been reported in other studies assessing diets enriched with less than 10% of Ulva sp. biomass (Ergun et al., 2008; Yildirin et al., 2009; Nisha et al., 2014). Although not completely ascertained, decreased lipid deposition in muscle has been attributed to the high vitamin C content of Ulva sp. meal (Garcia Casal et al., 2007). However, the low inclusion level considered in our diets cast doubts on the role of this specific substance, and it is likely that further bioactive compounds from algae might have influenced fish lipid metabolism and, therefore, muscle lipid deposition.
Regarding the deferred effects of diets on muscle lipid metabolism, this phenomenon was observed with a 5% U. ohnoi feeding pulse on Senegalese sole (Sáez et al., 2020) but not in the present study at this inclusion level (U5 lot). Several variables might well have influenced the differences observed between both studies: i) a longer duration of the feeding pulse (90 d vs. 60 d) and ii) a species-specific effect, since both fish and algae species were different.
In terms of lipid quality, the muscle fatty acid profile is directly influenced by the characteristics of feedstuffs (Turchini et al., 2009; Tocher et al., 2019). The progressive replacement of fishmeal by plant ingredients may lead to an overuse of n6-FA in certain feeding formulae, this yielding increased n6-PUFA content in fish muscle (mainly LA, 18:2n-6) and, therefore, a lower n-3/n-6 ratio compared to wild specimens, a fact that implies a certain decrease in the nutritional quality of the lipid fraction (Turchini et al., 2009). In agreement, continuous administration of the COM diet in our study confirmed a significant modification of the fatty acid profile, decreasing the n3-PUFA content, in agreement with the results of Xu et al. (2021) for turbot continuously fed with plant-based diets. On the other hand, several advantages have been described for the utilization of aquafeeds enriched with algae biomass at a low inclusion level (less than 10%), and thus, Sprague et al. (2015) and Kousoulaki et al. (2016) reported that Schizochytrium sp. biomass (at 5.5 and 1.5%, respectively) increased DHA and EPA contents in Atlantic salmon. Seabream (Sparus aurata) juveniles fed for 90 d with a low dietary level of Tisochrysis lutea also showed increased muscle n3-PUFA (Vizcaíno et al., 2016). Not only microalgae but also macroalgae can increase n3-PUFA, as found in previous studies with Ulva-containing diets (Valente et al., 2016; Vizcaíno et al., 2019). This sort of n3-PUFA-selective retention for algae-enriched diets has been attributed to both the structural function of these FA and to certain effects (not yet fully ascertained) of algal bioactive compounds on fish lipid metabolism (Perera et al., 2020).
With regard to long-term effects in this study, roughly speaking, microalgae-enriched diets (M5 and M10) did not cause changes in fillet n3-PUFAs at the end of the assay (Table 4). In contrast, U. rigida–enriched diet at the highest dose assayed (U10) resulted in a long-term increase of SFA, n3-PUFA, EPA, and DHA in turbot fillets. These results are in line with those observed after a discontinued feeding pattern during 90 d in Senegalese sole) with a diet enriched with 5% U. ohnoi (Sáez et al., 2020). The increase in EPA and DHA contents is also responsible for the higher lipid quality index (FLQ) found for CT and U10 batches in this study, despite the lower total lipid content in fillets (Table 4). Not only was discontinued feeding with U10 diet able to increase n3-PUFA in fillets, but an algae-free diet (CT) also caused a similar effect. However, it should be borne in mind that, even if not enriched with macro- or microalgae biomass, a CT diet is rich in fishmeal and fish oil, which obviously have plenty of n3-PUFA.
Lipid peroxidation is one of the main concerns during fish frozen storage, especially with regard to long-chain PUFA (Karlsdottir et al., 2014; Ojagh et al., 2014). TBARS values in all the experimental groups at day 1 post-thawing after a 5-month frozen storage (Figure 1) were very low (between 1.2 and 1.4 mg MDA kg-1). After 10 days post-thawing at 4°C, even if the values increased significantly in all groups, they persisted below 3 mg kg-1 in all cases, which are considered of perfect quality for human consumption (Ozden and Erkan, 2006). Undoubtedly, the leanness of turbot has accounted for these values, and they also confirm the aptitude for the frozen storage of flatfish in general. It is remarkable that COM fillets, obtained from specimens fed continuously for 7 months with a COM, showed the highest level of lipid oxidation during the 10 days of post-thawing cold storage, compared to the rest of the groups. The effects of dietary changes on this specific quality parameter in turbot fillets is virtually unexplored, although the results reported by Xu et al. (2021) pointed out that MDA measured in the serum of turbot juveniles fed with a diet rich in ingredients of plant origin was significantly higher than that measured in fish fed with fishmeal and fish oil, a fact that would likely be reflected in muscle as well.
Although the inclusion of algae biomass in diets did not determine differences at the time of thawing, an evident effect was attributable to algae enriched-diets (M5, M10, U5, and U10) during the post-thawing cold storage (Figure 1). There is plenty of evidence in the literature pointing to the antioxidant effects of algae, attributed to compounds such as pigments, vitamins, and polyphenols (Halldorsdottir et al., 2014; Wells et al., 2017; Sáez et al., 2021). Similar results were obtained following a comparable administration schedule of a diet supplemented with 5% U. ohnoi in Senegalese sole (Sáez et al., 2020). In addition to the COM group, in the rest of experimental batches, differences become significant from day 6 post-thawing onwards between CT and algae-containing diets, this fact suggesting a remarkable and persistent protective effect of all the algae-enriched experimental aquafeeds against lipid oxidation.
Fillet texture is an important attribute that determines the consumers’ acceptance of farmed fish, so that fillet softness leads to commercial depreciation (Nagappan et al., 2021). Fish hardness decreases after long-term frozen storage, owing to both the freezing process itself and to the fact that some proteolytic processes continue even at low temperatures (Sáez et al., 2015; Nakazawa and Okazaki, 2020). The results obtained in this study (Figure 2) are in agreement with this idea, given that hardness immediately after thawing, even if high compared to other lean fish species, was still lower than that described for fresh turbot (Wijerah et al., 2020). As expected, fillet hardness decreased during post-thawing cold storage, irrespectively of the dietary treatment, as a result of the biochemical changes leading to the relaxation of the muscle structure (Delbarre-Ladrat et al., 2006). However, clear differences among the experimental groups could be observed, and thus, the inclusion of 10% U. rigida (U10) for 60 d resulted in higher fillet hardness compared to the rest of algae-enriched diets (U5, M5, and M10) immediately after thawing. CT fillets yielded similarly high firmness than U10, whereas the COM batch showed the lowest values for this parameter.
The improvement observed for U10 and CT for this textural parameter might well be explained by the already-mentioned lower fillet lipid content in both batches (Table 3), given that it is known that the higher the lipid content in muscle, the lower the firmness (Thakur et al., 2003). In this line, the higher lipid content in M10 and U5 batches (Table 3) could also explain their lower firmness (Table 5). There is not extensive literature available on the effects of dietary changes on the textural parameters of turbot fillets, but the results found, roughly, are similar to those reported previously (Sáez et al., 2020). In other fish species (Regost et al., 2003; Mørkøre, 2006; Xu et al., 2016) the substitution of fish oil by plant oils can negatively affect fillet hardness, although a recent study (Xu et al., 2021) did not find significant differences in turbot fillet texture among the control group and groups alternating plant and marine sources. The alteration of lipids during the freezing process leads to the formation of free fatty acids (de Koning and Mol, 1991), especially in lean species, which, together with already oxidized lipids, can enable protein denaturation processes (Mackie, 1993; Sotelo et al., 1995). This phenomenon might well explain the poor values for textural parameters in COM fillets (Figure 2), since this experimental batch also showed the highest values for lipid oxidation (Figure 1).
Fillet pH is a valuable index that provides information on the muscle condition. During post-thawing cold storage, values ranged from 6.0 to 6.8, thus indicating an optimum condition of thawed fillets even after 10 days of post-thawing cold conservation (Figure 3A). In addition, the initial pH values of CT, M5, and U10 batches were lower than the rest, with no significant differences among the lots from 8-d post-thawing onwards. It is usual that pH values increase during storage as a result of the formation of alkaline compounds from the metabolism of bacteria (Goulas and Kontominas, 2007). Bacterial proliferation also leads to lower hardness since the proteolytic enzymes of these microorganisms can affect the structure of the muscle. Again, the high values of hardness observed in CT, U10, and M5 groups (Figure 2) could be related to the low pH values measured in these fillets (Figure 3A).
With regard to WHC, no clear differences attributable to dietary treatments or post-thawing storage period could be observed. This parameter decreases during fish cold storage since it reflects both the mechanical damage in muscle tissue and protein denaturation taking place during muscle deterioration (Duun and Rustad, 2007). The values in this study were felt within the range between 60% and 66% (Figure 3B), which are considerably high taking into account that these fillets were kept frozen for 5 months. This fact, together with the stability of values throughout the post-thawing cold storage period irrespectively of the dietary treatment, reflects again the excellent aptitude for the freezing of this species.
The literature has confirmed that feeding fish with algae-enriched diets can modify the skin pigmentation of farmed fish (Nagappan et al., 2021). In this respect, as mentioned above, both macro- and microalgae are natural sources of pigments with a valuable potential to provide farmed fish with more attractive tonality, thus increasing their marketability (Posten and Schaub, 2009). As also mentioned above, commercial aquafeeds are rich in ingredients from plant origin that are responsible for the poor coloration of fish, compared to wild individuals, which include a wide variety of marine natural ingredients in their diet. A feasible strategy to recover attractive pigmentation in farmed fish might well be the inclusion in the feeding formulae of the most important primary source of natural pigments in the marine trophic chain, that is, algae. Particularly, the high content of chlorophylls, xanthophylls, and phycocyanins reported for Ulva sp. (Stengel et al., 2011; Moroney et al., 2016) might have accounted for changes in some color attributes found in this study. Not only macroalgae but also microalgae have been responsible for changes in color attributes in numerous fish species (Teimouri et al., 2013; Abdulrahman and Ameen, 2014; Cardinaletti et al., 2018). However, the results in the present study have been modest in comparison with many of them. Undoubtedly, the discontinued feeding carried out in this work, compared to finishing or long-term trials performed in previous studies, have accounted for the limited effects observed. Even so, algae-enriched diets improved green and yellow tonalities (Figure 4), especially in U10 fillets, compared to CT and COM batches. Similar long-term results were also described previously (Sáez et al., 2020), suggesting that pigments from algae are selectively retained in flatfish skin chromatophores for a prolonged period of time.
With regard to the flesh side of fillets, turbot muscle is recognized for its whiteness (Roth et al., 2009), with a clear tendency to evolve to a pale yellowish color as storage progresses. A certain impact has also been observed in this side as a result of the dietary pulse considered in this study, which can be summarized as the emergence of a very slightly teal tonality in muscle as a result of the inclusion of any of the algal sources. On the contrary, COM-fed animals yielded fillets that tended to be yellowish, a fact likely associated with greater lipid oxidation (Thanonkaew et al., 2006; Sáez et al., 2014).
However, as mentioned above, strictly from the point of view of profitability, it is unlikely that increased cost linked to the utilization of finishing diets enriched with a low-to-moderate level of algae would be worthy in terms of color improvement. On the contrary, considering that a feeding pulse with algae-containing diets at early stages of the production cycle is relatively affordable, even if the impact observed on color is modest, nevertheless, the beneficial effects observed in terms of the fillet texture, lipid profile, and oxidative status might well justify their inclusion in commercial diets.
It is also worth mentioning that the commercial aquafeed used as control in this study, roughly speaking, yielded the poorer values for most of the fillet quality parameters assessed. It is likely that these results reflect the need to further optimize the commercial aquafeed formula, which ideally should fulfill not only fish growth and nutritional requirements but also other aspects involved in quality requirements that consumers demand for farmed fish. There is therefore a long way to go before all these aspects are achieved in farmed fish, but, undoubtedly, algae biomass will play a key role in this regard.
The raw data supporting the conclusions of this article will be made available by the authors, without undue reservation.
The animal study was reviewed and approved by C.N. Instituto Español de Oceanografía - CSIC. C.O. de Santander.
MS, FA, AHR and CR conceived and designed the experiments. FA prepared the aquafeeds. AG, MS, AV and TM performed fish sampling. AHR and CR participated in sampling, fish care, and maintenance. AG, performed and interpreted fatty acid analysis. MS, TM, and FA performed analytical analysis and discussed the data. MS, TM, and FA drafted the manuscript. AHR obtained the necessary funds for conducting the research. All authors critically revised and approved the manuscript. All authors contributed to the article and approved the submitted version.
This research was founded by the project ALGADIET I and II (Pleamar program of Biodiversity Foundation from Ministry for the Ecological Transition and the Demographic challenge, cofunded by European Maritime and Fisheries Fund). The authors thank grants UNAM15-CE-3510, EQC2018-004984-P, and EQC2019-006380-P to Service of Experimental Diets. AG has been supported by a post-doc contract from AquaTech4Feed project (grant # PCI2020-112204 by AEI within the ERA-NET BioBlue COFUND).
The authors declare that the research was conducted in the absence of any commercial or financial relationships that could be construed as a potential conflict of interest.
All claims expressed in this article are solely those of the authors and do not necessarily represent those of their affiliated organizations, or those of the publisher, the editors and the reviewers. Any product that may be evaluated in this article, or claim that may be made by its manufacturer, is not guaranteed or endorsed by the publisher.
Abdel-Tawwab M., Ahmad M. H. (2009). Live spirulina (Arthrospira platensis) as a growth and immunity promoter for Nile tilapia, Oreochromis niloticus (L.), challenged with pathogenic aeromonas hydrophila. Aquac. Res. 40, 1037–1046. doi: 10.1111/j.1365-2109.2009.02195.x
Abdulrahman N. M., Ameen J. H. (2014). Replacement of fishmeal with microalgae spirulina on common carp weight gain, meat and sensitive composition and survival. pak. J. Nutt. 13, 93–98. doi: 10.3923/pjn.2014.93.98
AOAC: The Association of Official Analytical Chemists (2000). “Official methods of analysis,” in The association of official analytical chemists, 17th Edition(Gaithersburg, MD, USA).
Azaza M. S., Dhraïef M. N., Kraïem M. M. (2008). Effects of water temperature on growth and sex ratio of juvenile Nile tilapia Oreochromis niloticus (Linnaeus) reared in geothermal waters in southern Tunisia. J. Therm. Bio. 33, 98–105. doi: 10.1016/j.jtherbio.2007.05.007
Bharathi S., Cheryl A., Rajagopalasamy C. B. T., Uma A., Ahilan B., Aanand S. (2019). Functional feed additives used in fish feeds. Int. J. Fish Aquat. Stud. 7 (3), 44–52.
Buege J. A., Aust S. D. (1978). “Microsomal lipid peroxidation,” in Methods in enzymology, vol. Vol. 52. (Academic Press), 302–310. doi: 10.1016/s0076-6879(78)52032-6
Cai L., Cao A., Li T., Wu X., Xu Y., Li J. (2015). Effect of the fumigating with essential oils on the microbiological characteristics and quality changes of refrigerated turbot (Scophthalmus maximus) fillets. Food Bioprocess Tech. 8, 844–853. doi: 10.1007/s11947-014-1453-0
Cardinaletti G., Messina M., Bruno M., Tulli F., Poli B. M., Giorgi G., et al. (2018). Effects of graded levels of a blend of Tisochrysis lutea and Tetraselmis suecica dried biomass on growth and muscle tissue composition of European sea bass (Dicentrarchus labrax) fed diets low in fish meal and oil. Aquaculture 485, 173–182. doi: 10.1016/j.aquaculture.2017.11.049
CIE (1986). Recommendations on uniform color spaces, color difference equations, psychometric color terms, supplement 2 to CIE publication 15 (E1.3.1) 1971/ (TC1.3) (Vienna, Austria: Central Bureau of the Commission Internationale de l'Éclairage).
de Koning A., Mol T. (1991). Quantitative quality tests for frozen fish: Soluble protein and free fatty acid content as quality criteria for hake (Merluccius capensis) stored at –18°C. J. Sci. Food Agric. 58, 449–458. doi: 10.1002/jsfa.2740540316
Delbarre-Ladrat C., Cheret R., Taylor R., Verrez-Bagnis V. (2006). Trends in post mortem aging in fish: Understanding of proteolysis and disorganization of the myofibrillar structure. Crit. Rev. Food Sci. Nutr. 46, 409–421. doi: 10.1080/10408390591000929
Duun A. S., Rustad T. (2007). Quality changes during superchilled storage of cod (Gadus morhua) fillets. Food Chem. 105, 1067–1075. doi: 10.1016/j.foodchem.2007.05.020
Ergun S., Soyuturk M., Guroy B., Guroy D., Merrifield D. (2008). Influence of Ulva meal on growth, feed utilization and body composition of juvenile Nile tilapia (Oreochromis niloticus) at two levels of dietary lipid. Aquac. Int. 17, 355–361. doi: 10.1007/s10499-008-9207-5
Erkan N., Yeşiltaş M. (2014). Effects of sodium alginate coating and vacuum packaging on the extension of the shelf life of hot smoked rainbow trout fillets. Fleischwirtschaft Int. 6, 52–57. doi: 10.1016/j.foodcont.2010.10.012
Folch J. (1957). A simple method for the isolation and purification of total lipids form animal tissues. J. Biol. Chem. 226, 497–509. doi: 10.1016/S0021-9258(18)64849-5
Galafat A., Vizcaíno A. J., Sáez M. I., Martínez T. F., Jerez-Cepa I., Mancera J. M., et al. (2020). Evaluation of arthrospira sp. enzyme hydrolysate as dietary additive in gilthead seabream (Sparus aurata) juveniles. J. Appl. Phycol. 32, 3089–3100. doi: 10.1007/s10811-020-02141-0
Garcia Casal M. N., Pereira A. C., Leets I., Ramırez J., Quiroga M. F. (2007). High iron content and bioavailability in humans from four species of marine algae. J. Nutt. 137, 2691–2695. doi: 10.1093/jn/137.12.2691
Ghosh S., Sarkar T., Pati S., Kari Z. A., Edinur H. A., Chakraborty R. (2022). Novel bioactive compounds from marine sources as a tool for functional food development. Front. Mar. Sci. 10. doi: 10.3389/fmars.2022.832957
Goulas A. E., Kontominas M. G. (2007). Combined effect of light salting, modified atmosphere packaging and oregano essential oil on the shelf-life of sea bream (Sparus aurata): Biochemical and sensory attributes. Food Chem. 100, 287–296. doi: 10.1016/j.foodchem.2005.09.045
Grigorakis K. (2017). Fillet proximate composition, lipid quality, yields and organoleptic quality of Mediterranean farmed marine fish: A review with emphasis on new species. Crit. Rev. Food Sci. Nutr. 57, 2956–2969. doi: 10.1080/10408398.2015.1081145
Grigorakis K., Alexis M. N., Taylor K. D. A., Hole M. (2002). Comparison of wild and cultured gilthead sea bream (Sparus aurata); composition, appearance and seasonal variations. Int. J. Food Sci. Techn. 37 (5), 477–484. doi: 10.1046/j.1365-2621.2002.00604.x
Halldorsdottir S., Sveinsdottir H., Gudmundsdottir A., Thorkelsson G., Kristinsson H. (2014). High quality fish protein hydrolysates prepared from byproduct material with Fucus vesiculosus extract. J. Funct. Foods. 9, 10–17. doi: 10.1016/j.jff.2014.04.009
Karlsdottir G. M., Sveinsdottir K., Kristinsson G. H., Dominique V., Craft B., Arason S. (2014). Effects of temperature during frozen storage on lipid deterioration of saithe (Pollachius virens) and hoki (Macruronus novaezelandiae). Food Chem. 156, 234–242. doi: 10.1016/j.foodchem.2014.01.113
Kousoulaki K., Mørkøre T., Nengas I., Berge R. K., Sweetman J. (2016). Microalgae and organic minerals enhance lipid retention efficiency and fillet quality in Atlantic salmon (Salmo salar l.). Aquaculture 451, 47–57. doi: 10.1016/j.aquaculture.2015.08.027
Kumar Y., Yadav D. N., Ahmad T., Narsaiah K. (2015). Recent trends in the use of natural antioxidants for meat and meat products. Compr. Rev. Food Sci. Food Saf. 14, 796–812. doi: 10.1111/1541-4337.12156
Lepage G., Roy C. C. (1984). Improved recovery of fatty acid through direct transesterification without prior extraction or purification. J. Lipid Res. 25, 1391–1396. doi: 10.1016/S0022-2275(20)34457-6
Mørkøre T. (2006). Relevance of dietary oil source for contraction and quality of pre-rigor filleted Atlantic cod, Gadus morhua. Aquaculture 251, 56–65. doi: 10.1016/j.aquaculture.2005.05.016
Mackie I. (1993). The effect of freezing on flesh proteins. Food Rev. Int. 9, 575–610. doi: 10.1080/87559129309540979
Matos E., Dias J., Dinis M. T., Silva T. S. (2017). Sustainability vs. quality in gilthead seabream (Sparus aurata l.) farming: are trade-offs inevitable? Rev. Aquac. 9, 388–409. doi: 10.1111/raq.12144
Moroney N. C., Wan A. H., Soler-Vila A., FitzGerald R. D., Johnson M. P., Kerry J. P. (2015). Inclusion of Palmaria palmata (red seaweed) in Atlantic salmon diets: effects on the quality, shelf-life parameters and sensory properties of fresh and cooked salmon fillets. J. Sci. Food Agric. 95, 897–905. doi: 10.1002/jsfa.6753
Moroney N. C., Wan A. H., Soler-Vila A., O'Grady M. N., FitzGerald R. D., Johnson M. P., et al. (2016). Influence of green seaweed (Ulva rigida) supplementation on the quality and shelf life of Atlantic salmon fillets. J. Aquat. Food Prod. Technol. 26, 1175–1188. doi: 10.1080/10498850.2015.1046099
Nagappan S., Das P., AbdulQuadir M., Thaher M., Khan S., Mahata C., et al. (2021). Potential of microalgae as a sustainable feed ingredient for aquaculture. J. Biotechnol. 341, 1–20. doi: 10.1016/j.jbiotec.2021.09.003
Nakazawa N., Okazaki E. (2020). Recent research on factors infuencing the quality of frozen seafood. Fish Sci. 86, 231–244. doi: 10.1007/s12562-020-01402-8
Nisha P. R., Mary A. E., Uthayasiva M., Arularasan S. (2014). Seaweed Ulva reticulata a potential feed supplement for growth, colouration and disease resistance in fresh water ornamental gold fish, Carassius auratus. J. Aquac. Res. Dev. 5, 1000254. doi: 10.4172/2155-9546.1000254
Ojagh S. M., Rezaei M., Razavi S. H. (2014). Improvement of the storage quality of frozen rainbow trout by chitosan coating incorporated with cinnamon oil. J. Aquat. Food Prod. Technol. 23, 146–154. doi: 10.1080/10498850.2012.701710
Ozden Ö., Erkan N. (2006). Effect of different packaging methods on the shelf-life of marinated rainbow trout. Arch. Lebensm. Hyg. 57, 69–75.
Perera E., Sánchez-Ruiz D., Sáez M. I., Galafat A., Barany A., Fernández-Castro M., et al. (2020). Low dietary inclusion of nutraceuticals from microalgae improves feed efficiency and modifies intermediary metabolisms in gilthead sea bream (Sparus aurata). Sci. Rep. 10, 18676. doi: 10.1038/s41598-020-75693-3
Posten C., Schaub G. (2009). Microalgae and terrestrial biomass as source for fuels-a process view. J. Biotechnol. 142, 64–69. doi: 10.1016/j.jbiotec.2009.03.015
Qiao H., Hu D., Ma J., Wang X., Wu H., Wang J. (2019). Feeding effects of the microalga Nannochloropsis sp. on juvenile turbot (Scophthalmus maximus l.). Algal Res. 41, 101540. doi: 10.1016/j.algal.2019.101540
Regost C., Arzel J., Robin J., Rosenlund G., Kaushik S. J. (2003). Total replacement of fish oil by soybean or linseed oil with a return to fish oil in turbot (Psetta maxima): I. growth performance, flesh fatty acid profile, and lipid metabolism. Aquaculture 217, 465–482. doi: 10.1016/S0044-8486(02)00259-4
Rodríguez-Ruiz J., Belarbi E. H., García J. L., López D. (1998). Rapid simultaneous lipid extraction and transesterification for fatty acid analyses. Biotechnol. Tech. 12, 689–691. doi: 10.1023/A:1008812904017
Roth B., Imsland A. K., Foss A. (2009). Live chilling of turbot and subsequent effect on behaviour, muscle stiffnes, muscle quality, blood gases and chemistry. Anim. Welfare 18, 33–41.
Sáez M. I., Galafat A., Vizcaíno A., Chaves-Pozo E., Ayala M. D., Arizcum M., et al. (2022). Evaluation of Nannochloropsis gaditana raw and hydrolysed biomass at low inclusion level as dietary functional additive for gilthead seabream (Sparus aurata) juveniles. Aquaculture 556, 738288. doi: 10.1016/j.aquaculture.2022.738288
Sáez M. I., Martínez T. F., Cárdenas S., Suárez M. D. (2014). Effect of different preservation strategies on microbiological counts, lipid oxidation and colour of cultured meagre (Argyrosomus regius, l) fillets. J. Food Process. Preserv. 39, 768–775. doi: 10.1111/jfpp.12286
Sáez M. I., Suárez M. D., Alarcón F. J., Martínez T. F. (2021). Assessing the potential of algae extracts for extending the shelf life of rainbow trout (Oncorhynchus mykiss) fillets. Foods 10, 910. doi: 10.3390/foods10050910
Sáez M. I., Suarez M. D., Cárdenas S., Martínez T. F. (2015). Influence of freezing and freezing-thawing cycles on textural and biochemical changes of meagre (Argyrosomus regius, l) fillets during further cold storage. Int. J. Food Prop. 18, 1635–1647. doi: 10.1080/10942912.2014.919319
Sáez M. I., Vizcaíno A., Galafat A., Anguís V., Férnandez-Díaz C., Balebona M. C., et al. (2020). Assessment of long-term effects of the macroalgae Ulva ohnoi included in diets on Senegalese sole (Solea senegalensis) fillet quality. Algal Res. 47, 101885. doi: 10.1016/j.algal.2020.101885
Sales R., Galafat A., Vizcaíno A. J., Sáez M. I., Martínez T. F., Cerón-García M. C., et al. (2021). Effects of dietary use of two lipid extracts from the microalgae Nannochloropsis gaditana (Lubián 1982) alone and in combination on growth and muscle composition in juvenile gilthead seabream, Sparus aurata. Algal Res. 10, 2270. doi: 10.1016/j.algal.2020.102162
Senso L., Suarez M. D., Ruiz-Cara T., Garcıa-Gallego M. (2007). The possible effects of harvesting season and chilled storage on the fatty acid profile of the fillet of farmed gilthead sea bream (Sparus aurata). Food Chem. 101, 298–307. doi: 10.1016/j.foodchem.2006.01.036
Shah M. R., Lutzu G. A., Alam A., Sarker P., Chowdhury M. K., Parsaeimehr A., et al. (2018). Microalgae in aquafeeds for a sustainable aquaculture industry. J. Appl. Phycol. 30 (1), 197–213. doi: 10.1007/s10811-017-1234-z
Sotelo C. G., Piñeiro C., Pérez-Martín R. I. (1995). Denaturation of fish proteins during frozen storage: role of formaldehyde. Z. Lebensm. Unters. Forsch. 200, 14–23. doi: 10.1007/BF01192902
Sprague M., Walton J., Campbell P. J., Strachan F., Dick J. R., Bell J. G. (2015). Replacement of fish oil with a DHA-rich algal meat derived from Schizochytrium sp. on the fatty acid and persistent organic pollutant levels in diets and flesh of Atlantic salmon (Salmo salar, l.) post-smolts. Food Chem. 185, 413–421. doi: 10.1016/j.foodchem.2015.03.150
Stengel D. B., Connan S., Popper Z. A. (2011). Algal chemodiversity and bioactivity: Sources of natural variability and implications for commercial application. Biotech. Adv. 29, 483–501. doi: 10.1016/j.biotechadv.2011.05.016
Suárez M. D., Martinez T. F., Sáez M. I., Morales A. E., García-Gallego M. (2010). Effects of dietary restriction on post-mortem changes in white muscle of sea bream (Sparus aurata). Aquaculture 307, 49–55. doi: 10.1016/j.aquaculture.2010.07.006
Suárez M. D., Gonzalez M. J., Sáez M. I., Martinez T. F., Guil-Guerrero J. L.. (2015). Seasonal changes of proximate composition and fatty acids of farmed dusky grouper (Epinephelus marginatus Lowe, 1834). Int. J. Food Sci. Tech. 50, 1823–30. doi: 10.1111/ijfs.12838
Teimouri M., Amirkolaie A. K., Yeganeh S. (2013). The effects of Spirulina platensis meal as a feed supplement on growth performance and pigmentation of rainbow trout (Oncorhynchus mykiss). Aquaculture 396, 14–19. doi: 10.1016/j.aquaculture.2013.02.009
Thakur D. P., Morioka K., Itoh Y., Obatake A. (2003). Lipid composition and deposition of cultured yellowtail Seriola quinqueradiata muscle at different anatomical locations in relation to meat texture. Fish. Sci. 69, 487–494. doi: 10.1046/j.1444-2906.2003.00649.x
Thanonkaew A., Benjakul S., Visessanguan W., Decker E. A. (2006). Development of yellow pigmentation in squid (Loligo peali) as a result of lipid oxidation. J. Agric. Food Chem. 54 (3), 956–962. doi: 10.1021/jf052107h
Tocher D. R., Betancor M. B., Sprague M., Olsen R. E., Napier J. A. (2019). Omega-3 long-chain polyunsaturated fatty acids, EPA and DHA: bridging the gap between supply and demand. Nutrients 11, 89. doi: 10.3390/nu11010089
Turchini G. M., Torstensen B. E., Ng W. K. (2009). Fish oil replacement in finfish nutrition. Rev. Aquac. 1, 10–57. doi: 10.1111/j.1753-5131.2008.01001.x
Valente L. M. P., Araújo M., Batista S., Peixoto M. J., Sousa-Pinto I., Brotas V., et al. (2016). Carotenoid deposition, flesh quality and immunological response of Nile tilapia fed increasing levels of IMTA-cultivated ulva spp. J. Appl. Phycol. 28, 691–701. doi: 10.1007/s10811-015-0590-9
Vizcaíno A. J., Fumanal M., Sáez M. I., Martinez T. F., Moringo M. A., Fernández-Diaz C., et al. (2019). Evaluation of Ulva ohnoi as funtional dietary ingredient in juvenile Senegalese solea (Solea senegalensis): Effects on the structure and functionality of the intestinal mucosa. Algal Res. 42, 1608. doi: 10.1016/j.algal.2019.101608
Vizcaíno A. J., Rodiles A., López G., Saáez M. I., Herrera M., Hachero I., et al. (2018). Growth performance, body composition and digestive functionality of Senegalese sole (Solea senegalensis kaup 1858) juveniles fed diets including microalgae freeze-dried biomass. Fish Physiol. Biochem. 44, 1–17. doi: 10.1007/s10695-018-0462-8
Vizcaíno A. J., Saéz M. I., López G., Arizcun M., Abellán E., Martínez T. F., et al. (2016). Tetraselmis suecia and Tisochrysis lutea meal as dietary ingredients for gilthead seabream (Sparus aurata l.) fry. J. Appl. Phycol. 28 (5), 2843–2855. doi: 10.1007/s10811-016-0845-0
Wan A. H. L., Davies S. J., Soler-Vila A., Filtzgerald R., Johnson M. P. (2017). Macroalgae as a sustainable aquafeee ingredient. Rev. Aquac. 11, 458–492. doi: 10.1111/raq.12241
Wells M. L., Potin P., Craigie J. S., Raven J. A., Merchant S. S., Helliwell K. E., et al. (2017). Algae as nutritional and functional food sources: revisiting our understanding. J. Appl. Phycol. 29, 949–982. doi: 10.1007/s10811-016-0974-5
Wijerah W. H. A. S., Yue Z., Jiahuan L., Mengxi Y., Wenbing Z., Kangsen M. (2020). Dietary taurine improves muscle growth and texture characteristics in juvenile turbot (Scophthalmus maximus). Aquac. Rep. 17, 100305. doi: 10.1016/j.aqrep.2020.100305
Xu H., Bi Q., Liao Z., Sun B., Jia L., Wei Y., et al. (2021). Long-term alternate feeding between fish oil-and terrestrially sourced oil-based diets mitigated the adverse effects of terrestrially source oils on turbot fillet quality. Aquaculture 531, 735974. doi: 10.1016/j.aquaculture.2020.735974
Xu H., Dong X., Zuo R., Mai K., Ai Q. (2016). Response of juvenile Japanese seabass (Lateolabrax japonicus) to different dietary fatty acid profiles: growth performance, tissue lipid accumulation, liver histology and flesh texture. Aquaculture 461, 40–47. doi: 10.1016/j.aquaculture.2016.04.023
Keywords: dietary deferred effects, fillet quality, frozen storage, functional additive, microalgae, Ulva rigida
Citation: Sáez MI, Galafat A, Vizcaíno AJ, Rodríguez C, Hernández de Rojas A, Alarcón FJ and Martínez TF (2022) Long-term effect of a short pulse of dietary supplementation with algae on the quality of turbot (Scophthalmus maximus) frozen fillets. Front. Mar. Sci. 9:957217. doi: 10.3389/fmars.2022.957217
Received: 30 May 2022; Accepted: 04 July 2022;
Published: 28 July 2022.
Edited by:
Erik-Jan Malta, CTAQUA Aquaculture Technological Centre, SpainReviewed by:
Chuanqi Yu, Jiangxi Agricultural University, ChinaCopyright © 2022 Sáez, Galafat, Vizcaíno, Rodríguez, Hernández de Rojas, Alarcón and Martínez. This is an open-access article distributed under the terms of the Creative Commons Attribution License (CC BY). The use, distribution or reproduction in other forums is permitted, provided the original author(s) and the copyright owner(s) are credited and that the original publication in this journal is cited, in accordance with accepted academic practice. No use, distribution or reproduction is permitted which does not comply with these terms.
*Correspondence: F. Javier Alarcón, ZmFsYXJjb25AdWFsLmVz
Disclaimer: All claims expressed in this article are solely those of the authors and do not necessarily represent those of their affiliated organizations, or those of the publisher, the editors and the reviewers. Any product that may be evaluated in this article or claim that may be made by its manufacturer is not guaranteed or endorsed by the publisher.
Research integrity at Frontiers
Learn more about the work of our research integrity team to safeguard the quality of each article we publish.