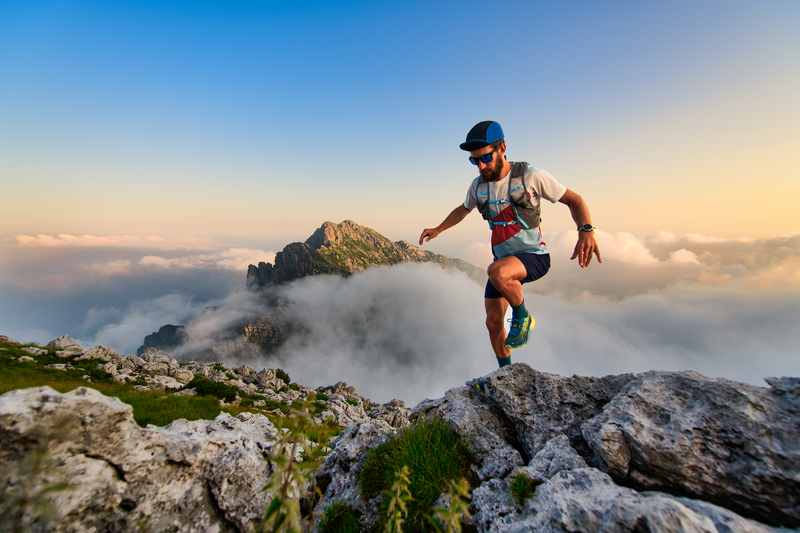
95% of researchers rate our articles as excellent or good
Learn more about the work of our research integrity team to safeguard the quality of each article we publish.
Find out more
ORIGINAL RESEARCH article
Front. Mar. Sci. , 16 August 2022
Sec. Marine Fisheries, Aquaculture and Living Resources
Volume 9 - 2022 | https://doi.org/10.3389/fmars.2022.947726
This article is part of the Research Topic Exploration and Utilization of Marine and Freshwater High-Value Biological Resources View all 12 articles
Fucoxanthin is a new dietary ingredient applied in healthy foods with specific benefits of body weight loss and liver fat reduction. The marine diatom Phaeodactylum tricornutum is a highly suitable species for fucoxanthin production. In the present study, aiming to promote fucoxanthin biosynthesis in mixotrophic P. tricornutum, NaNO3, tryptone, and urea were evaluated as nitrogen sources with 0.10 mol L−1 of glycerol as the organic carbon source for mixotrophic growth in shake flasks. Compared to NaNO3, the mixture of tryptone and urea (referred to as T+U, 1:1, mol N:mol N) as organic nitrogen sources could induce a higher biomass and fucoxanthin production. Through nitrogen utilization analysis, leucine, arginine, lysine, and phenylalanine in the T+U medium were identified as the amino acids that primarily support cell growth. Among those amino acids, arginine causes the highest rate of nitrogen utilization and cell growth promotion. After 12 days of cultivation, the highest biomass concentration (3.18 g L−1), fucoxanthin content (12.17 mg g−1), and productivity (2.68 mg L−1 day−1) were achieved using 25 mmol N L−1 of arginine and 5 mmol N L−1 of urea as nitrogen sources, indicating that arginine and urea performed synergistically on enhancing biomass and pigment production. This study provides new insights into the promotion of fucoxanthin biosynthesis by nitrogen utilization analysis and verifies the synergetic effect of arginine and urea on facilitating the development of a promising strategy for efficient enhancement of fucoxanthin production through mixotrophic cultivation of P. tricornutum.
Fucoxanthin is a carotenoid that is widely distributed in diatoms, brown algae, and golden algae (Yang et al., 2020). Given its antioxidant, anticancer, anti-obesity, anti-diabetes, and anti-Alzheimer’s disease properties (Lourenco-Lopes et al., 2021; Seth et al., 2021), fucoxanthin draws a growing attention and an increasing demand in the global market. Due to its health benefits and non-toxicity, fucoxanthin obtained the acknowledgement from the U.S. Food and Drug Administration (FDA) as a new dietary ingredient in 2017 for application in human health food (https://www.fda.gov/media/108748/download), especially for body weight management and liver health improvement (Yang et al., 2020; Sun et al., 2022). For example, fucoxanthin has been applied in commercial products of whole milk and skimmed milk as a weight loss drink (Mok et al., 2016; de Gonzalez et al., 2021). Currently, the commercial source of fucoxanthin is mainly brown seaweeds, but the very low content (<0.1% DW) of fucoxanthin and the difficulties of extraction result in the low quality and high cost of fucoxanthin-rich oil products (Rajauria et al., 2017).
Marine diatoms are microalgae that produce bioactive compounds like long-chain polyunsaturated fatty acids [eicosapentaenoic acid (EPA) and docosahexaenoic acid (DHA)], natural pigments (fucoxanthin and other high-value carotenoids, phycobiliproteins), and polysaccharides (chrysolaminarin, etc.), with broad applications in aquaculture, health foods, pharmaceuticals, and cosmetics (Yang et al., 2020). The marine diatom Phaeodactylum tricornutum is recognized as a suitable fucoxanthin producer due to its high content of fucoxanthin (Yang and Wei, 2020), and the highest level of fucoxanthin could reach 59 mg g−1 using flat panel photobioreactors (McClure et al., 2018). It can be cultivated at a large scale under autotrophic conditions for industrial purposes (Gao et al., 2017; Delbrut et al., 2018). The mixotrophic cultivation of P. tricornutum could achieve higher biomass productivity (1.01 g L−1 day−1) compared to autotrophic conditions, but the photosynthetic system was significantly inhibited, causing the low content of carotenoids (<0.7% DW) (Ceron-Garcia et al., 2013). As fucoxanthin is the main carotenoid in P. tricornutum, the content was even lower in the mixotrophic biomass. The results above suggested that it is hard to accumulate biomass and fucoxanthin simultaneously. Therefore, the development of a high-efficient manufacturing technology is an urgent demand for the commercial production of fucoxanthin by mixotrophic marine diatoms.
Since fucoxanthin exists in the form of a fucoxanthin–chlorophyll–protein complex (FCP) in the photosynthetic system of diatoms (Wang et al., 2019), nitrogen plays a vital role in FCP formation during cultivation. In diatoms, fucoxanthin biosynthesis requires nitrogen-rich conditions (Jauffrais et al., 2016; McClure et al., 2018; Wang et al., 2018). The fucoxanthin content was increased immediately after nitrate addition and then decreased with the consumption of nitrate (Pajot et al., 2022). The increase of nitrogen concentration from 40 to 400 μmol L−1 promoted a two-fold increase in pigment contents (including chlorophyll and fucoxanthin) (Jauffrais et al., 2016). The highest level of fucoxanthin content (5.92% DW) was reported under 10-fold NO3− addition (McClure et al., 2018). Biomass concentration and fucoxanthin content were also significantly enhanced around 50% and 70%, respectively, by extra nitrogen supply in the growth of P. tricornutum (Wang et al., 2018). Transcriptome analysis indicated that genes involved in the photosynthesis system and fucoxanthin biosynthesis pathway were upregulated under nitrogen-replete conditions (Alipanah et al., 2015; Remmers et al., 2018), and RNA-seq analysis demonstrated that the expression of light-harvesting complex genes (including FCP genes) was decreased with nitrogen depletion, resulting in the reduction of fucoxanthin content (Pajot et al., 2022). Thus, sufficient nitrogen is an essential nutrient for both cell growth and fucoxanthin biosynthesis in P. tricornutum.
Phaeodactylum tricornutum can utilize not only inorganic nitrogen sources like nitrate, nitrite, and ammonium but also organic nitrogen sources like urea, tryptone, and amino acids (Smith et al., 2019; Contreras and Gillard, 2021; Yang and Wei, 2020). Compared with nitrate and ammonium, P. tricornutum preferred to use urea, achieving a higher biomass concentration with no significant difference in fucoxanthin content (Zhang et al., 2016). Furthermore, the fucoxanthin production was 3.45-fold higher than that in the presence of urea when tryptone was used as a nitrogen source (Wang et al., 2021), and P. tricornutum could utilize most of the amino acids, in which the maximum uptake rate of nitrogen was reached by using arginine (Rees and Allison, 2006; Contreras and Gillard, 2021). Among 20 proteinogenic amino acids, arginine has four amino moieties, leading to the highest nitrogen to carbon ratio in the molecule. It is thus regarded as an effective storage of organic nitrogen in vivo (Winter et al., 2015). However, the impact of different types and concentrations of amino acids as well as the synergetic effect with other nitrogen sources on fucoxanthin biosynthesis is still not known clearly.
Phaeodactylum tricornutum cells assimilate nitrogen through glutamine synthetase (GS) and glutamine 2-oxoglutarate aminotransferase (GOGAT) cycle working with transport systems (Smith et al., 2019). Nitrate, nitrite, ammonium, and several amino acids that are metabolized extracellularly are assimilated in the chloroplast via GSII-GOGATFd and shuttle nitrogen to the mitochondria through the aspartate system (Smith et al., 2019; Contreras and Gillard, 2021). In contrast, urea, arginine, and lysine are assimilated by mitochondrial GSIII-GOGATa,b, and amino moieties are transported to the chloroplast through the alanine system (Flynn and Syrett, 1986; Smith et al., 2019). The aspartate and alanine systems both utilize the pyruvate carbon skeleton to transport nitrogen between organelles (Smith et al., 2019). In addition, pyruvate is an important precursor of photosynthetic pigment synthesis (Yang et al., 2020). To improve the efficiency of nitrogen assimilation, adequate carbon source and glycolysis capacity are required for the fast growth of cells. Previous studies indicated that mixotrophic conditions using glycerol as the organic carbon source could upregulate the pathway of glycolysis and provide more pyruvate in cells (Villanova et al., 2017), potentially supporting the biomass accumulation and fucoxanthin biosynthesis in P. tricornutum. For example, with glycerol and sufficient nitrogen addition, biomass productivity and fucoxanthin concentration were increased 43.5% and 97.5% in P. tricornutum, respectively (Wang et al., 2021). Therefore, it is feasible to improve nitrogen consumption rate and induce fucoxanthin biosynthesis by optimizing the types and concentrations of nitrogen sources with glycerol supply.
In this study, cell growth and fucoxanthin production were evaluated using inorganic (NaNO3) and organic nitrogen sources (the mixture of tryptone and urea) in mixotrophic P. tricornutum. Then, arginine was proven as the preferred amino acid by free amino acid consumption analysis in the medium using the mixture of tryptone and urea as nitrogen sources. Different concentrations of arginine, urea, and their mixture were investigated and optimized to develop a promising strategy for the efficient enhancement of fucoxanthin production. The proposed nitrogen assimilation pathway in P. tricornutum was fully discussed to explain the promotion of fucoxanthin biosynthesis.
The diatom P. tricornutum CCMP 1327 was kindly donated by Dr. Hanhua Hu from the Institute of Hydrobiology, Chinese Academy of Sciences (CAS), Wuhan, China. The seed culture was grown in 250-ml shake flasks containing 100 ml of modified f/2 medium with 9.20 g L−1 of glycerol and a mixture of 1.17 g L−1 of tryptone and 3 g L−1 of urea (1:1, N mol:N mol) in a shaking incubator with continuous illumination under 20 μmol m−2 s−1 by white LED light (OQ-PZP003050, 4,000 K, Guangdong Ocean Quantum Lighting Company, China) at 20°C and 160 rpm according to our previous work (Yang and Wei, 2020). The seed culture at the logarithmic phase was used as the inoculum for further experiments.
To investigate the effect of inorganic and organic nitrogen sources on cell growth and fucoxanthin accumulation, sufficient nitrogen concentrations (20 mmol L−1) of NaNO3 and the mixture of tryptone and urea (1:1, N mol:N mol, presented as T+U) selected from our previous study (Wang et al., 2021) were evaluated in mixotrophic growth with an initial cell density of 1 × 107 cells ml−1 (Yang and Wei, 2020) in shake flasks, respectively. Cell density, pH value, and nitrogen concentration were detected by sampling every 2 days during the cultivation. The biomass productivity and fucoxanthin content were detected at the end of cultivation according to our previous work (Yang and Wei, 2020).
During the cultivation above, the free amino acid concentrations in the T+U medium were analyzed by sampling every 2 days. According to the results of free amino acid consumption, the top 4 amino acids with high nitrogen consumption rate were identified as the preferred amino acids, in which the top 2 amino acids (lysine and arginine) at nitrogen contents of 0.3, 0.6, 0.9, and 1.2 mmol N L−1 based on the initial concentration in the T+U medium were then selected to compare their effects on cell density, biomass concentration, and chlorophyll fluorescence, as well as on the consumption rate of carbon and nitrogen. Nitrogen concentrations were selected according to the initial concentration of the top 2 amino acids identified in the T+U medium.
After the comparison of the top 2 preferred amino acids above, arginine, the dominant one, was investigated for its effect at the final nitrogen contents of 5, 10, 15, 20, 25, and 30 mmol N L−1 by comparing to urea at final nitrogen contents of 5, 10, 15, and 20 mmol N L−1. Moreover, their mixture at total nitrogen contents of 10, 15, 20, 25, and 30 mmol N L−1, in which urea was at 5 mmol N L−1, was carried out to investigate the synergetic effects by comparing with the solo nitrogen medium. Cell density, pH, and nitrogen concentration were monitored during the cultivation. Biomass concentration and productivity, pigment content, and fucoxanthin productivity were analyzed with glycerol consumption rate at the end of cultivation.
A 1-ml cell suspension was collected for cell density and chlorophyll fluorescence determination by CytoFLEX flow cytometry (Beckman Coulter, USA) according to the operation manual. The fluorescence intensity of chlorophyll was recorded in channel PC5.5 (excitation at 488 nm, emission at 690/50 nm through a BP filter).
The biomass concentration (g L−1) was determined by the gravimetric method. A 2-ml cell suspension was collected in a preweighed tube and biomass was measured after centrifuging, washing, and drying in a 60°C oven to a constant weight.
The specific growth rate (μ, day−1) and biomass productivity (mg L−1 day−1) were calculated using formulae (1) and (2):
where Nt and N0 are the cell densities (cells ml−1) at time t and time t0; Wt and W0 are the biomass concentrations (g L−1) at time t and time t0, respectively.
The glycerol concentration was determined by an M-100 biosensor analyzer (Siemens, China). NO3− and total nitrogen (TN) concentrations were determined using a water quality analyzer (HI83200, Hanna, Italy) and DR2700 spectrophotometer (HACH, USA), respectively (Luo et al., 2020; Yang and Wei, 2020).
The urea concentration was determined by the urease kit (C013-2-1, Jianyang, China). The reaction solution was prepared according to the kit’s instructions, and the absorbance was measured at 640 nm. The urea concentration was calculated using formula (3):
where Asample, Ablank, and Astandard are the absorbance of the sample, blank, and standard solution, while Cstandard is the concentration of the standard (mmol L−1).
The profile and concentration of free amino acids in the T+U medium were determined by an automatic amino acid analyzer (L8900, Hitachi, Japan) (Shim et al., 2013). A 2-ml cell-free culture broth of the T+U medium was collected every 48 h, and 0.5 ml of 15% sulfosalicylic acid was added for deproteinization. After mixing, the solution was stored at 2°C–4°C for 60 min. The supernatant was collected by centrifugation at 10,000×g for 15 min and then filtered through a 0.22-μm syringe membrane for further detection. The samples were detected by standard procedure with the MCI* buffer L-8500 pH kit, and then the free amino acids were identified and quantified by standard curves (Shen et al., 2021).
The arginine and lysine concentrations in the medium were determined by the ninhydrin colorimetric method (Tu, 2018). A 2× pH 5.6 buffer was prepared by 4.15 g of Na2HPO4·12H2O and 0.88 g of citric acid monohydrate. A 2% ninhydrin solution (w/v) was prepared using 1× pH 5.6 buffer. Two hundred microliters of 2× pH 5.6 buffer; a 200-μl supernatant of arginine, lysine standard, or sample; and a 400-μl ninhydrin solution were added in a glass tube and then put in a 100°C water bath for 15 min. Then, 2 ml of double-distilled H2O (ddH2O) was added to the tube after cooling at room temperature, and absorbance was measured at 570 nm. The arginine and lysine concentrations were calculated using arginine and lysine standard curves, respectively.
Natural pigments were extracted by organic solvents and measured by high-performance liquid chromatography (HPLC, DIONEX P680, Thermo Scientific, Waltham, USA) equipped with a PDA detector and a YMC™ Carotenoid column (150 mm × 4.6 mm, 3 μm) (Yang and Wei, 2020). The fucoxanthin productivity (mg L−1 day−1) was calculated by the following formula:
where Wt and W0 are the biomass concentrations (g L−1) at time t and time t0; Ct and C0 are the fucoxanthin contents (mg g−1) at time t and time t0, respectively.
The data were performed by triple biological replicates and presented as mean ± SD (standard deviation). The Origin V9.0 software was used for drawing the figures. The statistical analysis was carried out by one-way analysis of variance (ANOVA) and LDS t-test with SPSS V22.0. Different letters indicate significant differences (p< 0.05).
NaNO3 and the mixture of tryptone and urea (T+U) affected the cell growth of P. tricornutum. Higher cell density (10.86 × 107 cells ml−1) and specific growth rate (0.17 day−1) were observed in the T+U medium compared to the NaNO3 medium (p< 0.05) (Figure 1A; Table 1). It is coincident with the previous studies that P. tricornutum performed better in terms of cell growth with tryptone or urea addition (Wang et al., 2021). Compared with nitrate, it was reported that organic nitrogen sources, including leucine, isoleucine, and valine, could induce higher cell density in P. tricornutum (Hu et al., 2019). The pH value in the NaNO3 medium remained around 8.20~8.40 during the cultivation (Figure 1A), like in previous reports (Yongmanitchai and Ward, 1991; Eustance et al., 2013). In the T+U medium, pH was increased sharply to peak at 9.18 and then gradually decreased to 7.3 with faster cell growth in the logarithmic phase (Figure 1A). This pH fluctuation might relate to the priority utilization of nitrogen in the mixture of urea and free amino acids in the T+U medium. Moreover, the decline in nitrogen concentration was positively correlated with the increase of cell growth (Figures 1A, B). The consumption rate of total nitrogen (11.50 mg L−1 day−1) in the T+U medium was 15% higher than that in the NaNO3 medium (p< 0.05) (Table 1).
Figure 1 Cell density and pH value (A), total nitrogen concentration in the medium (B), pigment (fucoxanthin and chlorophyll a) contents, and biomass productivity (C) in the mixotrophic growth of Phaeodactylum tricornutum using inorganic (NaNO3) and organic nitrogen (tryptone:urea = 1:1, mol N:mol N) sources at 20 mmol N L−1. Different letters indicate a significant difference (p< 0.05).
Table 1 The specific growth rate, carbon and nitrogen consumption rate, and pigment content in the mixotrophic growth of P. tricornutum using NaNO3 and the mixture of tryptone and urea (1:1, mol N:mol N) as nitrogen source at 20 mmol N L−1 in shaking flasks.
In the T+U medium, the biomass productivity, glycerol consumption rate, fucoxanthin content, and chlorophyll a content were 245.2 mg L−1 day−1, 233.3 mg L−1 day−1, 16.11 mg g−1, and 34.33 mg g−1 (Figure 1C; Table 1), which were 40%, 27%, 157%, and 229% higher those in the NaNO3 medium, respectively (p< 0.05). Notably, the increase of biomass productivity was higher than the increase of glycerol consumption rate, which indicated that the higher biomass accumulation in the T+U medium might be caused by the additional carbon supply from the organic nitrogen sources. A previous study demonstrated that carbon and nitrogen tend to flow toward carbohydrate and protein synthesis rather than the photosynthetic system under glycerol and NaNO3 supply, which might explain the low content of photosynthetic pigments (fucoxanthin and chlorophyll a) in the NaNO3 medium (Villanova et al., 2017). It is reported that urea induced higher biomass productivity than nitrate in P. tricornutum (Zhang et al., 2016), and higher biomass was obtained using tryptone compared with both nitrate and urea in the diatom Nitzschia laevis (Wen and Chen, 2001). Earlier research also demonstrated that a 36% increase of biomass and a 28% increase of fucoxanthin were obtained using the mixture of tryptone and urea compared to tryptone (Wang et al., 2021). It is noteworthy that cellular chlorophyll a (1.10 pg cell−1) in the T+U medium was twice that in the nitrate medium with a 59% increase of cellular fucoxanthin (p< 0.05) (Table 1), indicating that the organic nitrogen source significantly promoted cellular pigment biosynthesis. Ammonium induced higher intracellular chlorophyll a concentrations in P. tricornutum compared with nitrate (Frada et al., 2013), and Entomoneis paludosa also reached the highest level of fucoxanthin content under ammonium compared to nitrate (Jauffrais et al., 2016) due to the high activities of the GS and GOGAT systems (Rees, 2003). Most of the available nitrogen (free amino acids and urea) in the T+U medium was finally utilized intracellularly as ammonium (Smith et al., 2019; Contreras and Gillard, 2021), which might promote intracellular nitrogen metabolism and contribute to the increased biosynthesis of photosynthetic pigments including FCP.
Therefore, the mixture of tryptone and urea was the optimal nitrogen source for enhancing biomass and fucoxanthin production, in which tryptone provided a complex nutrition (free amino acids, peptides, vitamins, and growth factors). However, high cost and lower bioavailability limit its industrial-scale application. Thus, the following experiments were carried out to determine the dominant nitrogen components for utilization and simplify of the medium.
To explore the amino acid utilization in the T+U medium, the concentration of free amino acids was monitored during the cultivation. As shown in Figure 2, leucine, lysine, arginine, and phenylalanine were the most abundant amino acids in the T+U medium (Figure 2A). When preferentially used up in 4~6 days, their nitrogen consumption rates reached 0.83, 2.09, 2.09, and 0.44 mg L−1 day−1, respectively (Figure 2C). Methionine, tyrosine, histidine, glutamate, and aspartic acid could be utilized to support cell growth as well (Figure 2A). A similar phenomenon was observed in previous studies, in which arginine, glutamate, leucine, and isoleucine could be used as sole nitrogen sources in P. tricornutum, and cells performed best by using arginine (Contreras and Gillard, 2021). A higher cell density was achieved by using arginine in E. paludosa compared with that using glutamine and glycine (Jauffrais et al., 2016). Interestingly, the rapid consumption of leucine, lysine, arginine, and phenylalanine was observed as the preferred amino acids (Figure 2A). Valine, serine, threonine, and alanine levels remained stable during the first 2 days and then increased sharply within the following 2 days (Figure 2B). Though the isoleucine content dropped slightly in the first 2 days, it reached the highest level on the fourth day. When the preferred amino acids were used up in the T+U medium after 6 days (Figure 2A), valine, serine, threonine, alanine, and isoleucine started to be consumed (Figure 2B). However, proline and glycine were secreted into the medium on the 4th and 10th day, respectively, without consumption during the cultivation (Figure 2B). The results were coincident with a previous report on E. paludosa where glycine was the worst nitrogen source for cell growth and pigment production (Jauffrais et al., 2016). A similar phenomenon was observed in P. tricornutum since proline and serine were hardly utilized after nitrogen deprivation (Rees and Allison, 2006). The intracellular glycine was reported as the only amino acid that increased in P. tricornutum after 4 days of cultivation (Ge et al., 2014), which was consistent with the trend of glycine in this study. Therefore, leucine, lysine, arginine, and phenylalanine were identified as the preferred amino acids for P. tricornutum utilization.
Figure 2 Concentrations of preferred free amino acids (A) and other free amino acids (B) in the medium and nitrogen consumption rates of four preferred amino acids (C) in the mixotrophic growth of Phaeodactylum tricornutum using organic nitrogen source (tryptone:urea = 1:1, mol N:mol N) at 20 mmol N L−1. Different letters represent significant difference (p<0.05).
To deeply understand nitrogen assimilation in P. tricornutum, the proposed assimilation pathway of amino acids and other nitrogen sources is summarized in Figure 3 according to the literature. In P. tricornutum, amino acids can be utilized mainly in two ways (Rees and Allison, 2006). On the one hand, amino acids are transported into cells by amino acid-specific transporters on the membrane systems and metabolized intracellularly (Flynn and Syrett, 1986). On the other hand, amino acids are oxidized to NH4+, α-keto acid, and hydrogen peroxide by extracellular L-amino acid oxidase (LAAO), then taken up by NH4+ transporters (AMT) on the membrane systems (Rees and Allison, 2006; Contreras and Gillard, 2021). Arginine and lysine can be assimilated by transporters directly and metabolized in vivo (Flynn and Wright, 1986; Rees and Allison, 2006). After entering the cells, arginine is converted to urea and ornithine by arginase (ARG) in the urea cycle in the mitochondrial membrane, and then urea is metabolized to NH4+ participating in the GS III-GOGATa,b cycle in the mitochondria (Smith et al., 2019), contributing to cell growth and protein synthesis (Zhang et al., 2015). Meanwhile, lysine is transported into cells directly and catabolized by the α-amino adipic acid pathway to produce glutamate joining in the GS III-GOGATa,b cycle (Arruda and Barreto, 2020), as well as acetyl-CoA, which finally enters the tricarboxylic acid (TCA) cycle contributing to carbohydrate accumulation (Zhang et al., 2015; Pan et al., 2020). In contrast, alanine, methionine, leucine, glutamate, valine, and asparagine are oxidized exclusively by LAAO extracellularly, and histidine is partially oxidized by LAAO in P. tricornutum (Rees and Allison, 2006; Contreras and Gillard, 2021). Phenylalanine, tyrosine, isoleucine, serine, threonine, aspartic acid, and glycine are also catalyzed by LAAO in other microalgae (Calatrava et al., 2019). It was reported that hydrogen peroxide produced by LAAO could not be utilized by cells and remained in the medium (Palenik and Morel, 1990), in which the accumulation of hydrogen peroxide triggered a massive cell death in P. tricornutum (Contreras and Gillard, 2021). Therefore, amino acids that are metabolized through LAAO are not considered favorable nitrogen sources for cell growth due to their indirect cell-damaging effect. All information above suggested that arginine and lysine are taken up by membrane transporters without hydrogen peroxide generation and have a great potential to be used as exclusive nitrogen sources for cell culturing and fucoxanthin production in P. tricornutum.
Figure 3 Proposed pathway of nitrogen assimilation in Phaeodactylum tricornutum and other microalgae. Solid arrow lines represent the direct reactions between the metabolites, and dash arrow lines represent the multistep reactions between those metabolites. Red boxes represent the amino acids consumed via LAAO by P. tricornutum; green boxes represent the amino acids consumed via LAAO by other microalgae. NRT, nitrate transporter; NAR, nitrite transporter; NR, nitrate reductase; NIR, nitrite reductase; GS, glutamine synthetase; GOGAT, glutamine 2-oxoglutarate aminotransferase; AMT, ammonium transporter; UT, urea transporter; ART, arginine transporter; ARG, arginase; HIT, histidine transporter; LYT, lysine transporter; LAAO, L-amino acid oxidase; FCP, fucoxanthin–chlorophyll–protein complex.
As the top 2 preferred amino acids in the T+U medium, arginine and lysine triggered the same nitrogen consumption rate (2.09 mg L−1 day−1) (Figure 2C). By taking into account the original nitrogen concentrations of arginine (0.61 mmol N L−1) and lysine (0.65 mmol N L−1) in the T+U medium (calculated from Figure 2A), the initial concentrations of arginine and lysine were set as 0.30, 0.60, 0.90, and 1.20 mmol N L−1 for the comparative study. As shown in Figure 4A, the cell density was higher under arginine supply, and the higher concentration of nitrogen led to a higher biomass concentration, glycerol, and nitrogen consumption rate (Figures 4A–D). Compared with the lysine medium, the biomass concentration increased 6%~30% in the arginine medium with a 12%~42% increase of glycerol consumption rate (Figures 4B–D). After inoculation in the medium, both arginine and lysine were consumed immediately, and the maximum nitrogen consumption rate (7.93 mg L−1 day−1) on the second day was achieved in the 1.20 mmol N L−1 arginine medium, which was 1.92-fold higher than the highest level in the 1.20 mmol N L−1 lysine medium (p< 0.05) (Figure 4D). In the literature, cells also performed better in the arginine medium than in the lysine medium, and the uptake rate of arginine was 64% higher than that of lysine in P. tricornutum (Flynn and Syrett, 1986; Rees and Allison, 2006), indicating that cells preferred arginine to lysine. In P. tricornutum, arginine has four amino moieties and three of them finally participated in nitrogen metabolism through the urea cycle and the GS-GOGAT cycle (Smith et al., 2019), while lysine only has two amino moieties and one was transported to the nitrogen metabolism pathway via the saccharopine pathway to produce glutamate (Arruda and Barreto, 2020) (Figure 3), suggesting that arginine has a higher nitrogen conversion rate in vivo. According to the nitrogen consumption curves, arginine was consumed entirely, while lysine remained in trace amounts in the medium. Though cells reached a nitrogen-deficient state in later cultivation, the final chlorophyll fluorescence using arginine was still 36%~79% higher than lysine (Figure 4B). Contreras and Gillard reported that P. tricornutum obtained the highest chlorophyll a fluorescence under arginine supply compared to other amino acids. The photosynthetic capacity was positively correlated to fluorescence (Contreras and Gillard, 2021), indicating that the photosynthetic system was more active in the arginine medium. Nitrogen starvation could significantly inhibit both growth and photosynthetic systems (including FCP) (Pajot et al., 2022); thus, the arginine supply was selected for an in-depth survey in the following experiments with the aim to obtain higher cell density, biomass, and photosynthetic capacity.
Figure 4 Cell density (A), mean fluorescence intensity (MFI) of chlorophyll and biomass concentration (B), nitrogen concentration (C), and average (ave.) glycerol consumption rate and maximum (max.) nitrogen consumption rate (D) using arginine (Arg) and lysine (Lys) at 0.3, 0.6, 0.9, and 1.2 mmol N L−1 in the mixotrophic medium. Different letters indicate a significant difference (p< 0.05).
To clarify the role of mixtures of organic nitrogen sources, urea was chosen to compare algal growth with or without the addition of arginine. The performance of cell growth under arginine, urea, and the mixture of arginine and urea (Arg + urea) in various concentrations is shown in Figure 5. Algal cultures showed a deeper brown color in the Arg + urea medium than in those media containing arginine or urea only. With the increase in nitrogen concentration, cells grew better in arginine and in the Arg + urea medium, but it was the opposite in the urea medium (Figure 5A–C). As shown in Figure 5D, a principal component analysis (PCA) was carried out according to the cell growth, nutrient consumption, pH change, and pigment production to understand the differences among various nitrogen sources. Principal component 1 (PC1) explained 77% of the total variation and showed a separated arginine cluster on the left, Arg + urea cluster on the right, and urea cluster in the middle. With the increase in nitrogen concentration, the dots in arginine and Arg + urea clusters exhibited a rightward shift, but the urea cluster showed the opposite trend. The separation in PC1 and PC2 suggested a significant difference between the three clusters with nitrogen types and concentrations.
Figure 5 The images of the mixotrophic Phaeodactylum tricornutum at the end of cultivation using (A) Arg (5, 10, 15, 20, 25, 30 mmol N L−1), (B) urea (5, 10, 15, 20 mmol N L−1), and (C) the mixture of Arg and urea (10, 15, 20, 25, 30 mmol TN L−1, in which urea was at 5 mmol N L−1) as nitrogen source and (D) score plot of the principal component analysis (PCA) for cell growth performance and pigment production.
The cell density increased rapidly after inoculating in both arginine and Arg + urea media. The specific growth rate was increased with the rise of nitrogen concentration, but it was the opposite in the urea medium (Figures 6A–C; Table 2) on the first 4 days, which were coincident with the color of algal cultures (Figure 5) and a previous report (Jauffrais et al., 2016). In the diatom E. paludosa, the cells obtained higher cell density under a higher concentration of arginine and achieved the maximum specific growth rate under a lower concentration of urea (Jauffrais et al., 2016). However, the cell growth became very slow after 4 days due to the sharp drop of pH in the arginine medium (Figure 6A). Though P. tricornutum continued to grow on the 10th day when adapted to low pH around 4.5, the cell densities (4.12~6.05 × 107 cells ml−1) on the 12th day were insignificantly different than those in the urea media (3.62~6.22 × 107 cells ml−1) but lower than those in the Arg + urea media. Obviously, the maximum cell density (9.21 × 107 cells ml−1) was obtained in the Arg + urea medium at 25 mmol N L−1 even though the pH finally dropped to 5.23, which was 52% and 48% higher than those in the 30 mmol N L−1 arginine and 5 mmol N L−1 urea media, respectively (Figures 6A–C).
Figure 6 Cell density and pH value (A–C), TN concentration in the medium (D–F), and pigment contents (G–I) using Arg (5,10, 15, 20, 25, 30 mmol N L−1) (A, D, G), urea (5, 10, 15, 20 mmol N L−1) (B, E, H), and the mixture of Arg and urea (10, 15, 20, 25, 30 mmol TN L−1, in which urea was at 5 mmol N L−1) (C, F, I) as nitrogen sources. The solid and dotted lines represent cell density and pH value (A–C), respectively. Total NH4+-N concentration (con.) represents NH4+ from the decomposed urea and secreted NH4+ from cells (E). Different letters indicate a significant difference (p< 0.05).
Table 2 The specific growth rate, biomass concentration, glycerol consumption rate (CR), and productivities of biomass and fucoxanthin in the mixotrophic growth of Phaeodactylum tricornutum using different types and concentrations of nitrogen sources in shaking flasks.
Arginine is a basic amino acid with a positive charge in the side chain group, and the uptake of arginine results in the release of hydrogen ions resulting in the decrease of pH value (Allen et al., 2011). Under an acidic pH environment, the function of FCPs switched from light-harvesting to energy-quenching via regulation of energy transfer pathways (Nagao et al., 2020), which might explain why the cells stopped growing after 4 days at pH below 4.5 (Figures 6A, C). As shown in Figure 6D, arginine was fully consumed on the 4th day at 5 mmol N L−1, and the Arg-N concentration curves in the other concentrations indicated that the consumption rate of arginine also slowed down due to the decrease of pH, which was coincident with the cell growth curve (Figure 6A). pH showed an increase after inoculation of seed cultures in the urea medium within 48 h and then decreased slightly except in 5 mmol N L−1 of urea, in which pH decreased to 7.28 after the 8th day (Figure 6B). Similar results were observed that the utilization of urea would not change the pH in the medium at the beginning (Eustance et al., 2013) and decreased to around 7.0 at the end of the culture period (Wen and Chen, 2001). It was reported that urea was metabolized intracellularly to form NH4+ and secreted it into the medium when urea was used as the only nitrogen source, causing an elevation of pH (Dhup et al., 2016). The medium with 5 mmol N L−1 of urea showed a rise in total NH4+-N concentration during the first 4 days and complete consumption on the 12th day (Figure 6E). Because extracellular urea-N concentration in the medium was determined by the chromogenic reaction of NH4+ generated from urea via the urease kit, the fluctuating curve of total nitrogen demonstrated the secretion of extra NH4+ in the medium (Figure 6E). The secretion and consumption of extra NH4+ caused the rise and decline of pH in the medium with 5 mmol N L−1 of urea (Wen and Chen, 2001; Eustance et al., 2013). Compared with the solo arginine media, the addition of 5 mmol N L−1 of urea in the arginine medium increased cell density and also maintained the pH in the range of 7.96~9.30 during the first 6 days as expected (Figure 6C). As shown in Figure 6F, arginine was barely consumed on the first 4 days with an increase of pH (Figure 6C), indicating that cells preferred to uptake urea at the beginning in the Arg + urea medium. After the fourth day, cells entered the logarithmic phase in the Arg +urea medium and entered the stationary phase on the eighth day when the pH decreased to 6.60 (Figure 6C).
As shown in Figures 6G–I; Table 2, biomass productivity, glycerol consumption rate, and pigment content were increased with the rise of nitrogen concentrations in arginine and Arg + urea media. The maximum biomass concentration (3.18 g L−1) and productivity (217.9 mg L−1 day−1) with a glycerol consumption rate of 247.22 mg L−1 day−1 were achieved under 30 mmol TN L−1 of Arg + urea medium, significantly higher than the solo arginine or urea medium (p< 0.05) (Table 2). In contrast, the biomass productivity in the urea media reached the highest level (142.93 mg L−1 day−1) at 5 mmol N L−1, 47% higher than that at 30 mmol N L−1 of arginine (Table 2). However, fucoxanthin content (7.79 mg g−1) and productivity (0.31 mg L−1 day−1) were decreased by 24% and 69%, respectively, suggesting that urea addition could significantly promote biomass production but reduce the biosynthesis of fucoxanthin. A previous study reported that more arginine–carbon was respired with the same uptake rate when ammonium and arginine were both present in the medium (Flynn and Wright, 1986). In this study, the addition of urea in the arginine medium provided sufficient ammonium in vivo. It could induce the assimilation of arginine, which might explain the higher biomass accumulation in the Arg + urea media. Furthermore, the maximum contents of fucoxanthin (12.17 mg g−1) and chlorophyll a (29.45 mg g−1) were both obtained at the highest concentration (30 mmol TN L−1) in the Arg + urea medium (p< 0.05), significantly increased by 18%~64% and 68%~133% compared with the solo arginine and urea media, respectively (Figures 6G–I). Fucoxanthin and chlorophyll a share the geranylgeranyl pyrophosphate building block that forms from pyruvate as a precursor. The biosynthesis of chlorophyll additionally requires the participation of glutamate (Bertrand, 2010; Meier et al., 2011). The synergetic effect of arginine and urea might promote the activity of the urea cycle, GS-GOGAT cycle, and TCA cycle (Figure 3), providing more substrate for the biosynthesis of photosynthetic pigments and proteins, leading to the higher contents of fucoxanthin and chlorophyll a. After 12 days of cultivation, the highest fucoxanthin productivity (2.68 mg L−1 day−1) was observed in the Arg + urea medium with 30 mmol TN L−1, 2.71-fold and 8.64-fold higher than that in the arginine medium at 30 mmol N L−1 and in the urea medium at 5 mmol N L−1, respectively (Table 2), but had no significant difference with the Arg + urea medium at 25 mmol TN L−1. In the literature, P. tricornutum was cultivated under complex organic carbon or nitrogen sources, but the bottleneck still remained for the simultaneous accumulation of biomass and fucoxanthin. For example, the fucoxanthin content achieved 17.55 mg g−1 with the supplementation of 1.5 ml L−1 of Laminaria japonica hydrolysate in P. tricornutum, while the biomass was only 1.59 g L−1 (Wang et al., 2022). When the spruce hydrolysate and yeast extract (C/N = 60) were used as carbon and nitrogen sources, biomass concentration reached 3.31 g L−1, but carotenoid concentration only achieved 16.92 mg L−1, in which the fucoxanthin concentration was lower (Patel et al., 2019). In this study, the maximum biomass productivity (217.9 mg L−1 day−1) and fucoxanthin content (12.17 mg g−1) in the Arg + urea medium at 30 mmol TN L−1 were 24% and 94% higher than those in the nitrate medium (Figure 1). We could thus realize the simultaneous accumulation of biomass and fucoxanthin and provide an efficient strategy for fucoxanthin production by mixotrophic P. tricornutum. In future studies, a higher biomass and fucoxanthin productivity could be expected with pH control in synergetic systems of arginine and urea by mixotrophic cultivation of P. tricornutum.
In this study, various inorganic and organic nitrogen sources were evaluated for improving cell growth and fucoxanthin production by mixotrophic P. tricornutum. Arginine was proven as the dominant amino acid for promoting cell growth compared to other free amino acids in tryptone and urea media. The synergetic effect of arginine and urea in the mixotrophic medium with 0.1 mol L−1 glycerol addition could promote biomass production and fucoxanthin biosynthesis simultaneously, achieving the highest productivity of biomass (217.9 mg L−1 day−1) and fucoxanthin (2.68 mg L−1 day−1). The present study provided new insights into fucoxanthin biosynthesis promoted by nitrogen metabolism, facilitating the development of an efficient process for enhancing fucoxanthin production by mixotrophic P. tricornutum.
RY: investigation, data curation, formal analysis, methodology, conceptualization, and writing—original draft. DW: conceptualization, supervision, and writing—review and editing. GP: supervision and writing—review and editing. All authors contributed to the article and approved the submitted version.
This work was supported by Guangdong Basic and Applied Basic Research Foundation (2019B1515120002). This work was partly supported by the 111 Project (B17018).
The authors declare that the research was conducted in the absence of any commercial or financial relationships that could be construed as a potential conflict of interest.
All claims expressed in this article are solely those of the authors and do not necessarily represent those of their affiliated organizations, or those of the publisher, the editors and the reviewers. Any product that may be evaluated in this article, or claim that may be made by its manufacturer, is not guaranteed or endorsed by the publisher.
Alipanah L., Rohloff J., Winge P., Bones A. M., Brembu T. (2015). ). whole-cell response to nitrogen deprivation in the diatom phaeodactylum tricornutum. J. Exp. Bot. 66 (20), 6281–6296. doi: 10.1093/jxb/erv340
Allen A. E., Dupont C. L., Obornik M., Horak A., Nunes-Nesi A., McCrow J. P., et al. (2011). Evolution and metabolic significance of the urea cycle in photosynthetic diatoms. Nature 473 (7346), 203–209. doi: 10.1038/nature10074
Arruda P., Barreto P. (2020). Lysine catabolism through the saccharopine pathway: Enzymes and intermediates involved in plant responses to abiotic and biotic stress. Front. Plant Sci. 11. doi: 10.3389/fpls.2020.00587
Bertrand M. (2010). Carotenoid biosynthesis in diatoms. Photosynthesis Res. 106 (1-2), 89–102. doi: 10.1007/s11120-010-9589-x
Calatrava V., Hom E. F. Y., Llamas A., Fernandez E., Galvan A. (2019). Nitrogen scavenging from amino acids and peptides in the model alga chlamydomonas reinhardtii. The role of extracellular l-amino oxidase. Algal Research-Biomass Biofuels Bioproducts 38, 11. doi: 10.1016/j.algal.2018.101395
Ceron-Garcia M. C., Fernandez-Sevilla J. M., Sanchez-Miron A., Garcia-Camacho F., Contreras-Gomez A., Molina-Grima E. (2013). Mixotrophic growth of phaeodactylum tricornutum on fructose and glycerol in fed-batch and semi-continuous modes. Bioresource Technol. 147, 569–576. doi: 10.1016/j.biortech.2013.08.092
Contreras J. A., Gillard J. T. F. (2021). Asparagine-based production of hydrogen peroxide triggers cell death in the diatom phaeodactylum tricornutum. Bot. Lett. 168(1), 6–17 doi: 10.1080/23818107.2020.1754289
de Gonzalez M. T. N., Attaie R., Mora-Gutierrez A., Woldesenbet S., Jung Y. (2021). Stability of fucoxanthin in pasteurized skim and whole goat milk. Foods 10 (7), 10. doi: 10.3390/foods10071647
Delbrut A., Albina P., Lapierre T., Pradelles R., Dubreucq E. (2018). Fucoxanthin and polyunsaturated fatty acids Co-extraction by a green process. Molecules 23 (4), 874. doi: 10.3390/molecules23040874
Dhup S., Kannan D. C., Dhawan V. (2016). Understanding urea assimilation and its effect on lipid production and fatty acid composition of scenedesmus sp. SOJ Biochem. 2 (1), 7. doi: 10.15226/2376-4589/2/1/00112
Eustance E., Gardner R. D., Moll K. M., Menicucci J., Gerlach R., Peyton B. M. (2013). Growth, nitrogen utilization and biodiesel potential for two chlorophytes grown on ammonium, nitrate or urea. J. Appl. Phycol 25 (6), 1663–1677. doi: 10.1007/s10811-013-0008-5
Flynn K. J., Syrett P. J. (1986). Utilization of l-lysine and l-arginine by the diatom phaeodactylum-tricornutum. Mar. Biol. 90 (2), 159–163. doi: 10.1007/bf00569122
Flynn K. J., Wright C. R. N. (1986). The simultaneous assimilation of ammonium and l-arginine by the marine diatom phaeodactylum-tricornutum bohlin. J. Exp. Mar. Biol. Ecol. 95 (3), 257–269. doi: 10.1016/0022-0981(86)90258-3
Frada M. J., Burrows E. H., Wyman K. D., Falkowski P. G. (2013). Quantum requirements for growth and fatty acid biosynthesis in the marine diatom phaeodactylum tricornutum (Bacillariophyceae) in nitrogen replete and limited conditions. J. Phycol 49 (2), 381–388. doi: 10.1111/jpy.12046
Gao B. Y., Chen A. L., Zhang W. Y., Li A. F., Zhang C. W. (2017). Co-Production of lipids, eicosapentaenoic acid, fucoxanthin, and chrysolaminarin by phaeodactylum tricornutum cultured in a flat-plate photobioreactor under varying nitrogen conditions. J. Ocean Univ. China 16 (5), 916–924. doi: 10.1007/s11802-017-3174-2
Ge F., Huang W. C., Chen Z., Zhang C. Y., Xiong Q., Bowler C., et al. (2014). Methylcrotonyl-CoA carboxylase regulates triacylglycerol accumulation in the model diatom phaeodactylum tricornutum. Plant Cell 26 (4), 1681–1697. doi: 10.1105/tpc.114.124982
Hu H., Pan Y., Yu C. (2019). Use of branched chain amino acids for culturing phaeodactylum tricornutum having high neutral lipid content (in Chinese). Hubei, China: Chinese Acad. Sci. Inst, Hydrobiology. CN110819536.
Jauffrais T., Jesus B., Meleder V., Turpin V., Russo A., Raimbault P., et al. (2016). Physiological and photophysiological responses of the benthic diatom entomoneis paludosa (Bacillariophyceae) to dissolved inorganic and organic nitrogen in culture. Mar. Biol. 163 (5), 14. doi: 10.1007/s00227-016-2888-9
Lourenco-Lopes C., Fraga-Corral M., Jimenez-Lopez C., Carpena M., Pereira A. G., Garcia-Oliveira P., et al. (2021). Biological action mechanisms of fucoxanthin extracted from algae for application in food and cosmetic industries. Trends Food Sci. Technol. 117, 163–181. doi: 10.1016/j.tifs.2021.03.012
Luo X., Chen J., Wei D. (2020). High efficient assimilation of NO3- -n with coproduction of microalgal proteins by chlorella pyrenoidosa (in Chinese). Chin. J. Biotechnol. 36 (06), 1150–1161. doi: 10.13345/j.cjb.190358
McClure D. D., Luiz A., Gerber B., Barton G. W., Kavanagh J. M. (2018). An investigation into the effect of culture conditions on fucoxanthin production using the marine microalgae phaeodactylum tricornutum. Algal Research-Biomass Biofuels Bioproducts 29, 41–48. doi: 10.1016/j.algal.2017.11.015
Meier S., Tzfadia O., Vallabhaneni R., Gehring C., Wurtzel E. T. (2011). A transcriptional analysis of carotenoid, chlorophyll and plastidial isoprenoid biosynthesis genes during development and osmotic stress responses in arabidopsis thaliana. BMC Syst. Biol. 5, 19. doi: 10.1186/1752-0509-5-77
Mok I. K., Yoon J. R., Pan C. H., Kim S. M. (2016). Development, quantification, method validation, and stability study of a novel fucoxanthin-fortified milk. J. Agric. Food Chem. 64 (31), 6196–6202. doi: 10.1021/acs.jafc.6b02206
Nagao R., Yokono M., Ueno Y., Shen J.-R., Akimoto S. (2020). Acidic pH-induced modification of energy transfer in diatom fucoxanthin chlorophyll a/c-binding proteins. J. Phys. Chem. B 124 (24), 4919–4923. doi: 10.1021/acs.jpcb.0c04231
Pajot A., Lavaud J., Carrier G., Garnier M., Saint-Jean B., Rabilloud N., et al. (2022). The fucoxanthin chlorophyll a/c-binding protein in tisochrysis lutea: influence of nitrogen and light on fucoxanthin and chlorophyll a/c-binding protein gene expression and fucoxanthin synthesis. Front. Plant Sci. 13. doi: 10.3389/fpls.2022.830069
Palenik B., Morel F. M. M. (1990). Amino-acid utilization by marine-phytoplankton - a novel mechanism. Limnol Oceanogr 35 (2), 260–269. doi: 10.4319/lo.1990.35.2.0260
Pan Y., Hu F., Yu C., Li C., Huang T., Hu H. (2020). Amino acid catabolism during nitrogen limitation in phaeodactylum tricornutum. Front. Plant Sci. 11. doi: 10.3389/fpls.2020.589026
Patel A., Matsakas L., Hruzova K., Rova U., Christakopoulos P. (2019). Biosynthesis of nutraceutical fatty acids by the oleaginous marine microalgae phaeodactylum tricornutum utilizing hydrolysates from organosolv-pretreated birch and spruce biomass. Mar. Drugs 17 (2), 119. doi: 10.3390/md17020119
Rajauria G., Foley B., Abu-Ghannam N. (2017). Characterization of dietary fucoxanthin from himanthalia elongata brown seaweed. Food Res. Int. 99 (3), 995–1001. doi: 10.1016/j.foodres.2016.09.023
Rees T. A. V. (2003). Mitochondrial oxidative phosphorylation is required for ammonium assimilation in light in a marine diatom. Physiol Plantarum 117 (4), 558–563. doi: 10.1034/j.1399-3054.2003.00074.x
Rees T. A. V., Allison V. J. (2006). Evidence for an extracellular l-amino acid oxidase in nitrogen-deprived phaeodactylum tricornutum (Bacillariophyceae) and inhibition of enzyme activity by dissolved inorganic carbon. Phycologia 45 (3), 337–342. doi: 10.2216/04-92.1
Remmers I. M., D’Adamo S., Martens D. E., de Vos R. C. H., Mumm R., America A. H. P., et al. (2018). Orchestration of transcriptome, proteome and metabolome in the diatom phaeodactylum tricornutum during nitrogen limitation. Algal Research-Biomass Biofuels Bioproducts 35, 33–49. doi: 10.1016/j.algal.2018.08.012
Seth K., Kumar A., Rastogi R. P., Meena M., Vinayak V., Harish (2021). Bioprospecting of fucoxanthin from diatoms-challenges and perspectives. Algal Research-Biomass Biofuels Bioproducts 60, 10. doi: 10.1016/j.algal.2021.102475
Shen Y., Hu L. T., Xia B., Ni Z. J., Elam E., Thakur K., et al. (2021). Effects of different sulfur-containing substances on the structural and flavor properties of defatted sesame seed meal derived maillard reaction products. Food Chem. 365, 11. doi: 10.1016/j.foodchem.2021.130463
Shim Y. S., Yoon W. J., Ha J., Seo D., Lee K. W., Lee W. Y., et al. (2013). Method validation of 16 types of structural amino acids using an automated amino acid analyzer. Food Sci. Biotechnol. 22 (6), 1567–1571. doi: 10.1007/s10068-013-0252-0
Smith S. R., Dupont C. L., McCarthy J. K., Broddrick J. T., Obornik M., Horak A., et al. (2019). Evolution and regulation of nitrogen flux through compartmentalized metabolic networks in a marine diatom. Nat. Commun. 10, 14. doi: 10.1038/s41467-019-12407-y
Sun H., Yang S. F., Zhao W. Y., Kong Q., Zhu C. L., Fu X. D., et al. (2022). Fucoxanthin from marine microalgae: A promising bioactive compound for industrial production and food application. Crit. Rev. Food Sci. Nutr., 1–18. doi: 10.1080/10408398.2022.2054932
Tu Y. (2018). Determination of total free amino acid in tea by ninhydrin (in Chinese). Modern Agric. Sci. Technol. 14, 238. Available at: https://kns.cnki.net/kcms/detail/34.1278.S.20180724.1139.290.html
Villanova V., Fortunato A. E., Singh D., Dal Bo D., Conte M., Obata T., et al. (2017). Investigating mixotrophic metabolism in the model diatom phaeodactylum tricornutum. Philos. Trans. R. Soc. B-Biol Sci. 372 (1728), 1–14. doi: 10.1098/rstb.2016.0404
Wang Z. P., Wang P. K., Ma Y., Lin J. X., Wang C. L., Zhao Y. X., et al. (2022). Laminaria japonica hydrolysate promotes fucoxanthin accumulation in phaeodactylum tricornutum. Bioresource Technol. 344, 4. doi: 10.1016/j.biortech.2021.126117
Wang S., Yang R., Song P., Wei D. (2021). Improving production of biomass and fucoxanthin in mixotrophic phaeodactylum tricornutum by optimization of carbon and nitrogen sources (in Chinese). J. Food Sci. Biotechnol. 40 (10), 82–90. doi: 10.3969/j.issn
Wang W. D., Yu L. J., Xu C. Z., Tomizaki T., Zhao S. H., Umena Y., et al. (2019). Structural basis for blue-green light harvesting and energy dissipation in diatoms. Science 363 (6427), 598. doi: 10.1126/science.aav0365
Wang H., Zhang Y., Chen L., Cheng W., Liu T. (2018). Combined production of fucoxanthin and EPA from two diatom strains phaeodactylum tricornutum and cylindrotheca fusiformis cultures. Bioprocess Biosyst. Eng. 41 (7), 1061–1071. doi: 10.1007/s00449-018-1935-y
Wen Z. Y., Chen F. (2001). Optimization of nitrogen sources for heterotrophic production of eicosapentaenoic acid by the diatom nitzschia laevis. Enzyme Microb Technol. 29 (6-7), 341–347. doi: 10.1016/s0141-0229(01)00385-4
Winter G., Todd C. D., Trovato M., Forlani G., Funck D. (2015). Physiological implications of arginine metabolism in plants. Front. Plant Sci. 6. doi: 10.3389/fpls.2015.00534
Yang R., Wei D. (2020). Improving fucoxanthin production in mixotrophic culture of marine DiatomPhaeodactylum tricornutumby LED light shift and nitrogen supplementation. Front. Bioengineering Biotechnol. 8. doi: 10.3389/fbioe.2020.00820
Yang R. Q., Wei D., Xie J. (2020). Diatoms as cell factories for high-value products: chrysolaminarin, eicosapentaenoic acid, and fucoxanthin. Crit. Rev. Biotechnol. 40 (7), 993–1009. doi: 10.1080/07388551.2020.1805402
Yongmanitchai W., Ward O. P. (1991). Growth of and omega-3-fatty-acid production by phaeodactylum-tricornutum under different culture conditions. Appl. Environ. Microbiol. 57 (2), 419–425. doi: 10.1128/aem.57.2.419-425.1991
Zhang W., Gao B., Li A., Zhang C. (2016). Effects of different culture conditions on growth and accumulation of bioactive compounds by phaeodactylum tricornutum. Mar. Sci. 40 (5), 57–65. doi: 10.11759/hykx20150706002
Keywords: fucoxanthin, Phaeodactylum tricornutum, amino acids, arginine, urea
Citation: Yang R, Wei D and Pohnert G (2022) Nitrogen utilization analysis reveals the synergetic effect of arginine and urea in promoting fucoxanthin biosynthesis in the mixotrophic marine diatom Phaeodactylum tricornutum. Front. Mar. Sci. 9:947726. doi: 10.3389/fmars.2022.947726
Received: 19 May 2022; Accepted: 20 July 2022;
Published: 16 August 2022.
Edited by:
Shuhao Huo, Jiangsu University, ChinaReviewed by:
Yi Cui, Jiangsu University, ChinaCopyright © 2022 Yang, Wei and Pohnert. This is an open-access article distributed under the terms of the Creative Commons Attribution License (CC BY). The use, distribution or reproduction in other forums is permitted, provided the original author(s) and the copyright owner(s) are credited and that the original publication in this journal is cited, in accordance with accepted academic practice. No use, distribution or reproduction is permitted which does not comply with these terms.
*Correspondence: Dong Wei, ZmV3ZDMwNEBzY3V0LmVkdS5jbg==
Disclaimer: All claims expressed in this article are solely those of the authors and do not necessarily represent those of their affiliated organizations, or those of the publisher, the editors and the reviewers. Any product that may be evaluated in this article or claim that may be made by its manufacturer is not guaranteed or endorsed by the publisher.
Research integrity at Frontiers
Learn more about the work of our research integrity team to safeguard the quality of each article we publish.