- 1Department of Biology, Baylor University, Waco, TX, United States
- 2Biology Department, Sonoma State University, Rohnert Park, CA, United States
- 3Department of Biology, Colorado State University, Fort Hood, CO, United States
- 4Department of Earth and Environmental Sciences, Dalhousie University, Halifax, NS, Canada
Northern elephant seals (Mirounga angustirostris), like many marine mammals, rely on internal lipid stores, specifically fatty acids (FAs) stored in the blubber layer, to meet metabolic needs. The energetic demands of northern elephant seals vary with ontogeny, as each life-history stage experiences different metabolic requirements due to development, growth, and breeding. To date, no comprehensive study has reported on changes in blubber FA profiles across northern elephant seal age groups or sex. Therefore, our objective was to determine how blubber FAs differ across ontogeny and sex in northern elephant seals. As a sexually dimorphic mammal, northern elephant seals go through sex-specific ontogenetic changes in morphology and physiology; we hypothesized that these changes would be reflected in their FAs profiles. To determine this, FAs profiles were compared from full blubber cores collected from 79 northern elephant seals across four age groups. We provide the first evidence of blubber fatty acids differing across ontogeny as NES transition from young, developing seals to mature fully developed adults. However, we did not find differences in blubber FAs profiles between the sexes. Monounsaturated fatty acids (MUFAs) are found in the highest proportions across all NES age classes and sexes, followed by SFAs and PUFAs; this highlights the important role MUFAs play in maintaining fluidity of the blubber layer and in thermoregulation. The individual FAs with the highest concentrations (C16:1, C18:1n9 and C16:0) in northern elephant seal blubber are similar to those in other marine mammals. Weaned pup FAs profiles were significantly different from all other age classes; adults and juveniles also showed age-specific differences. Specifically, weaned pups had the highest proportions of SFAs and the lowest proportions of PUFA, suggesting use of PUFAs to aid development. Each life history stage of NES is interconnected to previous and future stages, making FA accumulation, mobilization, and storage an important process throughout an individual’s life. Further, any changes to this process can have cascading consequences throughout ontogeny in this species. Future monitoring of the FA composition of blubber across age classes could potentially indicate the costs of different environmental changes on blubber storage in NES.
Introduction
Mammals, like all living species, have evolved and adapted over time. For some, this has manifested in the form of a lipid-based metabolism and voluntarily fasting periods as a regular part of a natural life history (Castellini and Rea, 1992; Berg et al., 2002; Champagne et al., 2012; McCue et al., 2017). Capital breeders, like seals, sea lions, and walruses (pinnipeds), use previously-stored endogenous resources to meet metabolic demands during regular fasting periods as part of their natural life history (Castellini and Rea, 1992; Rea, 1995). More specifically, phocids (true seals) have evolved the ability to fast for extended periods (days to months) during breeding (Le Boeuf, 1974), post-weaning development (Reiter et al., 1978), lactating (Costa et al., 1986), or molting (Boyd et al., 1993). During these fasting periods, phocids rely on lipids to meet up to 98% of their metabolic requirements (Castellini and Rea, 1992; Crocker et al., 2001; Groscolas and Robin, 2001; Noren et al., 2003; Champagne et al., 2006).
Survival during fasting periods is dependent on phocids ability to store and mobilize fatty acids (FAs), their primary energy source, from adipocytes contained within blubber tissue (Strandberg et al., 2008a). Blubber is an adapted fat layer covering much of the body, with enriched collagen and elastic fibers to support structural aspects of the blubber and is ubiquitous across most marine mammals (Iverson, 2009). Blubber is the primary storage depot for FAs in marine mammals, and is, therefore, a critically important tissue for species employing a capital breeding strategy (Pond, 1978; Crocker et al., 2014). Previous research has shown the amount of lipids stored during at-sea foraging trips (which occur just prior to extended hauling out and fasting periods) directly impacts the fasting duration, reproductive effort, mating success, milk composition, immune function, and stress responses in phocids (Crocker et al., 2001; Crocker et al., 2014; Crocker et al., 2016; Sharick et al., 2015; Peck et al., 2016).
Beyond being the primary energy depot, blubber is a metabolically active tissue that has many key roles in maintaining homeostasis in phocids (Strandberg et al., 2008b). Phocids rely on blubber for thermoregulation to maintain their internal body temperature (Scholander et al., 1950; Strandberg et al., 2008a), which is more difficult while at-sea, since water has a much higher thermal conductivity (23-fold) compared to air (Davis, 2019). Blubber also helps streamline the body and helps seals achieve neutral buoyancy, thereby reducing diving costs and increasing foraging efficiency (Scholander et al., 1950; Kooyman, 1973; Ryg et al., 1988; Noren, 2002; Strandberg et al., 2008a).
Blubber is therefore an important component of both on-land (breeding, fasting, mating, molting) and at-sea (thermoregulation, diving) periods for phocids throughout their lives. While foraging, captured prey is digested, and prey FAs are deposited relatively unmodified into the blubber (Budge et al., 2004). The total sum of FAs deposited in the blubber during foraging is important when returning to land; the deposited FAs are used to meet metabolic needs during fasting periods. Furthermore, the FA composition of prey FAs have been shown to vary in response to environmental changes, such as those caused by anthropogenic activities (Noren, 2020; De Luca Peña et al., 2022). Thus, studying blubber FAs can provide important information related to diet changes and serve as an important signal of environmental stressors.
Blubber thickness varies among phocid taxa, while the chemical structure of FAs, and the classes of FA that are used by phocids all play specific, relatively constant roles across different phocid species (Fredheim et al., 1995; Champagne et al., 2012; Noren et al., 2013). Monounsaturated FAs (MUFAs) have one double carbon bond and help to maintain the fluidity of the blubber layer (Strandberg et al., 2008a; Liwanag et al., 2012; Davis, 2019). Saturated fatty acids (SFAs) in comparison, have no double carbon bonds, meaning no extra steps are required for oxidation (i.e. removing double bonds) resulting in a similar chain length SFA containing two more ATP per molecule compared to the monounsaturated counterpart (Strandberg et al., 2008b; Trumble and Kanatous, 2012). Thus, SFAs are more energy dense due to the lack of double bonds and a higher ATP yield. Polyunsaturated fatty acids (PUFAs) have two or more double carbon bonds, and are important for development, are precursors to eicosanoids, and are involved in neural tissue processes (Glandon et al., 2021). Due to the number of double bonds, PUFAs of the same carbon length will provide less ATP than both the MUFA and SFA counterparts.
Northern elephant seals (Mirounga angustirostris; NES) are sexually dimorphic deep-diving migratory phocids who undergo long fasting periods during two-yearly haul outs (breeding and molting; Boeuf et al., 1989; Champagne et al., 2012; Robinson et al., 2012). Throughout ontogeny, NES show distinct developmental behaviors resulting in fast lengths varying by life history stage (Boeuf et al., 1989; Champagne et al., 2012; Robinson et al., 2012). For instance, as pups, NES fast for up to three months post-weaning to develop their diving abilities, while relying on energy stores acquired during lactation (Reiter et al., 1978; Champagne et al., 2012). The transition from pups to juveniles is characterized by periods of somatic growth during one-month-long fasting haul outs, where seals rely on acquired energy stores acquired during their at-sea foraging trips (Field et al., 2005; Kelso et al., 2012). NES females reach sexual maturity around 3-5 years old; as adults they spend most of the year foraging at sea, interspersed by two annual fasts for breeding and molting (Reiter et al., 1981; Condit and Le Boeuf, 1984; Costa et al., 1986). Alternatively, NES males do not reach true adulthood (physical maturity) until 8-10 years old, spending an extended period of time classified as ‘subadults’ prior to reaching their adult body size and acquiring fully developed secondary sexual characteristics (Clinton and Boeuf, 1993; Boeuf and Laws, 1994). During this subadult period for males, the length of the fasts range from 37-71 days depending on their subadult stage (subadults are classified into one of four subadult groups based on morphology and size as described by Le Boeuf et al. (1996) (Boeuf and Laws, 1994; Casey et al., 2020). As adults, male NES will fast for up to four continuous months during the annual breeding season (Le Boeuf, 1974; Deutsch et al., 1990). Therefore, NES experience varying metabolic demands across ontogeny related to the life history stage and further within sex, and this likely impacts their energy storage and use during fasting periods.
Furthermore, sexual dimorphism in NES is related to future reproductive success, with NES pups already showing sex-specific differences in life history strategies. For example, male NES pups aided by delayed canine eruption, risk attempting to steal milk at the end of lactation, although the potential additional lipid accumulation is minimal (Reiter et al., 1978). As juveniles, NES exhibit sex differences in field metabolic rates and metabolic approaches to fasting; specifically, juvenile males preserve protein more efficiently than females (Kelso et al., 2012). Adult female NES experience the highest energetic demands during their 28 day lactation period, simultaneously providing pups with milk and maintaining their own metabolic needs to survive the fasting period (Fowler et al., 2018). As adults, males are up to 10 times larger than females and spend the breeding haul-out period competing for access to females and defending harems (Le Boeuf, 1974; Clinton and Boeuf, 1993; Casey et al., 2020). These sex-specific differences in behavior and physiology likely translate to differences in blubber composition.
Northern elephant seals exhibit significant differences in behavior, energetic requirements, and life history across ontogeny and sex. However, no studies to date have assessed how FAs, the primary energy source for NES, differ as a function of age and sex. Therefore, the objective of this study were to investigate how blubber FA profiles change as a result of ontogeny and sex in NES. We also identify the specific FAs important in driving age- and sex-specific differences. We hypothesized that blubber FA profiles would significantly differ across age classes (i.e., between weaned pups, juveniles, subadults and adults). We also predicted that the blubber FA profiles would differ between the sexes within each age class based on the sex-specific patterns in behavior, ecology, and physiology across ontogeny. Projected shifts in prey sources and prey FA compositions as a result of climate change may require NES to increase their foraging effort to remain in a positive energy balance (du Pontavice et al., 2020; Hückstädt et al., 2020; Mueter et al., 2021). However, NES are already foraging most of their time at sea, highlighting the importance of understanding drivers of intraspecific variation in FA profiles in NES and using these as a monitoring tool of energetic stressors across different life history stages (Adachi et al., 2021).
Methods
Sample collection
Blubber samples (N = 79) were collected from 79 NES at Año Nuevo State Reserve, CA, USA (37° 06’03"N, 122°20’10"W) between 2013-2016 (2013: n = 44, 2014: n = 15, 2015: n = 6, 2016: n = 14) under NMFS permits 14636 and 19108 (Table 1). Samples were taken across the breeding and molt haul-out periods as part of a long-term and ongoing demography studies. Life history and age class data were available for all sampled individuals (Table 1). Seals were categorized into one of four age groups based on age and sex: (1) weaned pups (1-12 months in age); juveniles (1-2 years); subadults (males only, SA4: 7 years old), and adults (females: ≥3 years old; males: >8 years old). Seals were chemically immobilized with an intramuscular injection of Telazol (tiletamine/zolazepam HCL) at a dose of 0.3 to 1.0mg/kg, and intravenous injections of ketamine (50-100 mg) were administered as needed to maintain immobilization following established protocols (Kelso et al., 2012). During chemical immobilization, a blubber sample was taken from the lateral side of the seal approximately one third of the body length rostral from the tip of the tail (Figure 1). A local anesthetic (lidocaine) was administered subcutaneously prior to tissue sample removal. A small incision was made with a disposable scalpel, and full depth blubber samples were taken using a 6 mm blubber punch. Blubber samples were immediately placed into cryovials, frozen in a liquid nitrogen shipper, and stored in a -80°C freezer until processing.
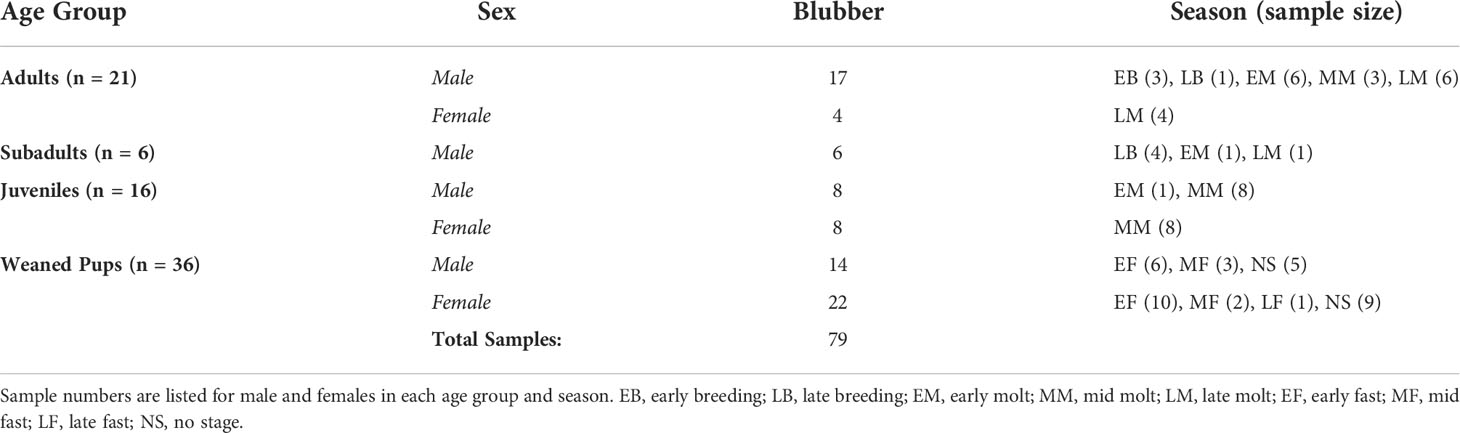
Table 1 Number of northern elephant seal blubber samples collected from 79 animals from different age classes between 2013-2016.
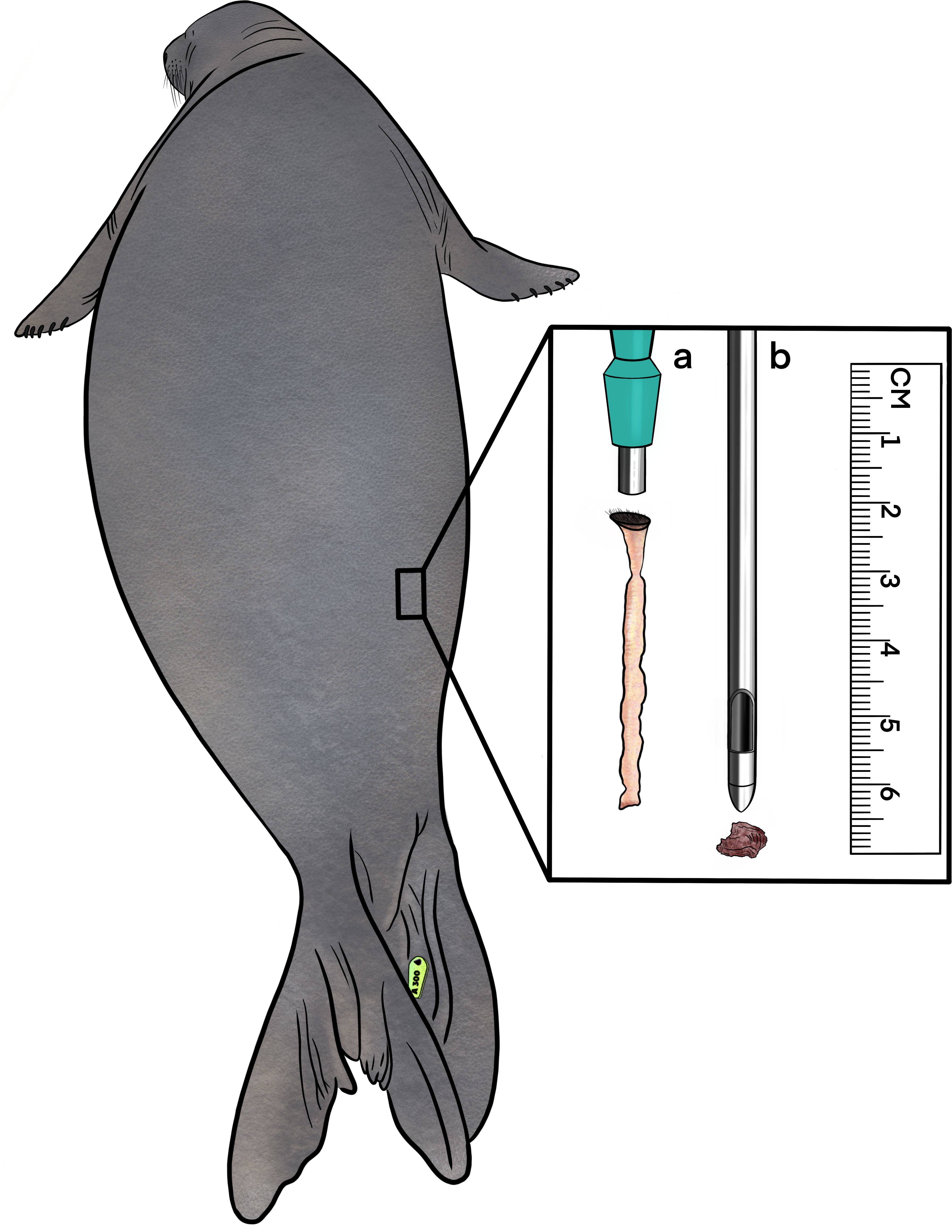
Figure 1 Location of sample collection for blubber and muscle tissue from northern elephant seals. A scalpel was used to make a small incision; (A) blubber cores were taken from the epidermis down to the muscle using a 6mm blubber punch, (B) while not used in this study, muscle samples were removed using a 6mm biopsy canula from the same incision point, extracting 30-60mg of muscle tissue from the main locomotor muscle, the longissimus dorsi.
Fatty acid analysis
Lipids were extracted from blubber samples using a modified 2:1 chloroform-methanol extraction method (Folch et al., 1957; Budge et al., 2006). In brief, lipids were extracted from the blubber using a Soxtec 2043 extraction system. Fatty acid methyl esters (FAMEs) preparation were then derivatized following established protocol using a Hilditch reagent (H2SO4 dissolved in: MeOH dried over anhydrous Na2SO4) (Budge et al., 2006). FAMEs were analyzed using a gas chromatograph (Thermo Trace 1300 and Thermo 1310 Autosampler) equipped with a flame ionization detector (GC-FID) and Zebron ZB-FAME column.
The injector port and detector were held at a constant 250°C. A 1 µl injection of sample with a split ratio of 100:1 was used with a split flow rate of helium of 100 mL/min. The carrier gas flow rate (helium) was set at 1 mL/min, with the hydrogen and air flow rates to the detector set at 30 mL/min and 300 mL/min, respectively. Initial oven temperature was held at 60°C for 2 minutes and then the temperature was ramped at a rate of 10°C/min to 220°C. The final temperature ramp was at 2°C/min to 250°C and held for 5 minutes until all FAME standards had eluted from the column. Total run time for the analysis was 38 minutes. Peak identification, peak area, and retention times were calculated with Chromeleon 7.2 using a set of 37 FAME standards (Supelco® 37 component FAME mix). Each FA identified from the 37 FAME commercial standard was calculated as a percentage of the cumulative of all FAs in each individual sample. Of the 37 FAs identified, only those found to be higher than trace amounts (<0.75%) were included in the statistical analyses (Grahl-Nielsen et al., 2011).
Post-FA analysis revealed that the cotton used during the Soxtec lipid extraction contained lipids, resulting in skewed FA values for a subset of individual FAs. All samples were processed with the same protocol and cotton; thus, we ran intra-laboratory experiments to create a correction factor. We ran blank lipid extractions on the original cotton (n = 10) as well as fat-free cotton (n = 10). We removed cotton lipids by running it three times through the Soxtec lipid extraction. The lipid extracts from cotton samples were processed into FAMEs and analyzed via GC-FID as described above. FA data were corrected using the percentage difference between pre- and post cotton-corrected samples. All statistical analysis was performed on the cotton-corrected FA data.
Statistical analysis
Initial analysis conducted identified no differences in season and therefore season was removed from further analysis (season information available in data repository file). Non-parametric multivariate analyses were used to determine blubber FA profile differences and interactions between two factors: age group and sex (Primer and PERMANOVA+ add on; PRIMER-E, Plymouth; Anderson, 2017). Data were normalized and transformed prior to analysis to improve normality using a log (x+1) transformation, and a Bray-Curtis dissimilarity matrix was created from the normalized data. A permutational multivariate analysis of variance (PERMANOVA; Anderson, 2017) was used to investigate each factor individually and all interactions (9999 permutations under a reduced model, type III sum of squares). P-values were set at 0.05 a priori.
All significant interactions were analyzed with the pairwise test function in PERMANOVA and visualized in multivariate space with NMDS plots. Similarity percentages (SIMPER) were used to determine the individual FAs contributing to significant differences determined from PERMANOVA tests. Permutational analysis of multivariate dispersions (PERMDISP) were used to test the homogeneity of multivariate dispersions using the null hypothesis of no difference in dispersion between multivariate groups. Individual FAs contributing to the highest contribution for significant PERMANOVA test results (identified using SIMPER routines) were analyzed using Mann-Whitney U tests (IBM SPSS Statistics for Mac OS, version 28; IBM Corp, Armonk, N.Y., USA). FAs are reported as percentage means ± standard error (SE.).
Results
Blubber profiles were analyzed using 18 FAs. These 18 FAs accounted for 96% of the overall FA contribution of all blubber profiles. When separated by FA classes, MUFAs (48.8 ± 1.6%) had the highest proportion across all blubber profiles, followed by SFAs (37.3 ± 1.5%) and polyunsaturated fatty acids (PUFAs; 13.9 ± 1.2%; Table 2). Of the 18 FAs, six individual FAs (C16:1, C18:1n-9, C16:0, C18:0, C22:6n-3, and C20:1) accounted for the majority (79%) of the blubber profiles (Table 3).
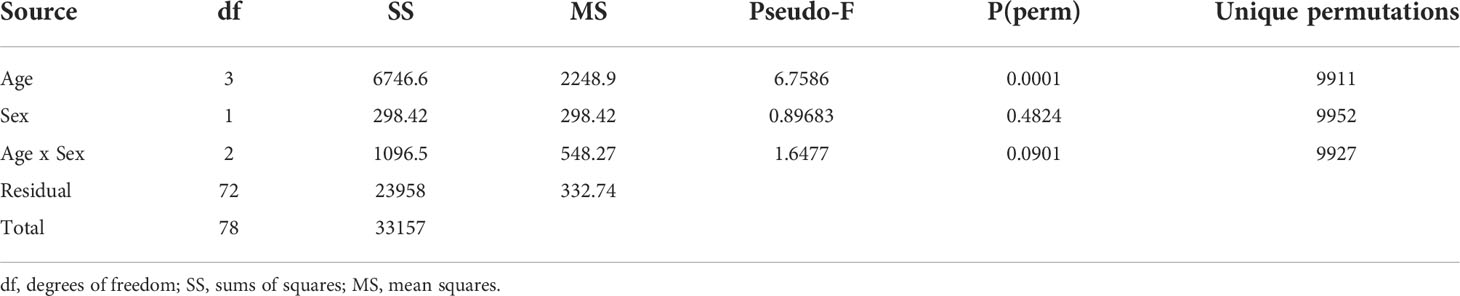
Table 3 PERMANOVA model to evaluate the effect of age group and sex on northern elephant seal blubber fatty acid compositions.
Fatty acid profiles significantly differed within age groups (Pseudo-F = 6.76, p<0.001). Specifically, age group differences occurred between: 1) adults and juveniles (t = 1.72, p<0.05, Table 4), 2) adults and weaned pups (t = 3.19, p <0.001), 3) subadults and weaned pups (t = 2.62, p <0.001), and 4) juveniles and weaned pups (t = 3.99, p <0.001, Figure 2). No sex differences were found in blubber FA profiles. Four FAs (C20:1, C22:1, C18:2n6t and C18:3n6) and the FA class of PUFAs increased in proportion with age from weaned pups to adults.
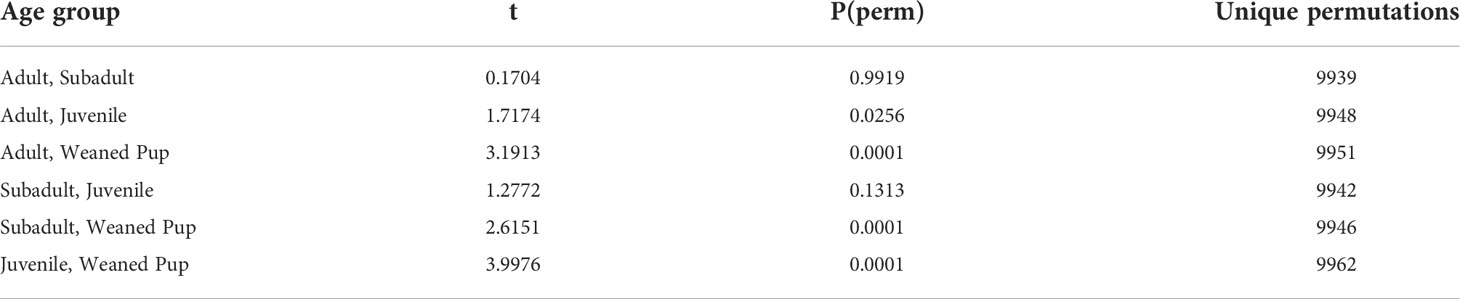
Table 4 PERMANOVA post hoc pairwise tests for the significant age group differences in northern elephant seal blubber samples.
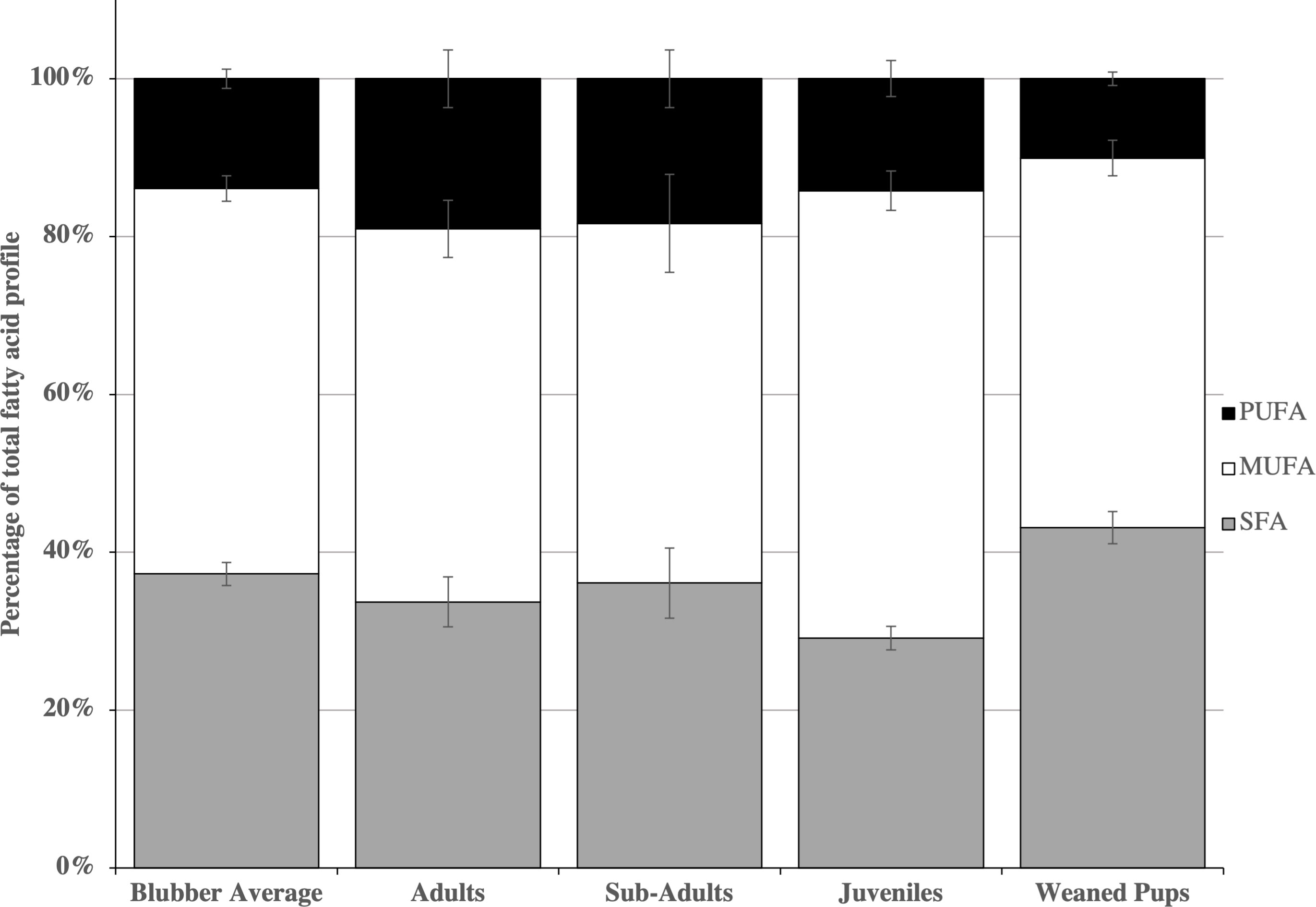
Figure 2 Mean percentages ± standard error of fatty acid classes in northern elephant seal age groups. SFAs, saturated fatty acids (grey bars); MUFAs, monounsaturated fatty acids (white bars) and PUFAs, polyunsaturated fatty acids (black bars).
The SFAs were highest in weaned pups (43.1 ± 2.0%, Figure 3), followed by subadults (36.1 ± 4.5%), adults (33.7 ± 3.2%) and juveniles (29.1 ± 1.5%). The MUFAs were found in the highest proportions in juveniles (56.7 ± 2.5%), followed by adults (47.3 ± 3.6%), weaned pups (46.8 ± 2.3%) and subadults (45.6 ± 6.2%). The PUFAs were found in the highest proportion in adults (19.0 ± 3.6%), followed by subadults (18.3 ± 3.6%), juveniles (14.2 ± 2.3%) and weaned pups (10.1 ± 0.9%).
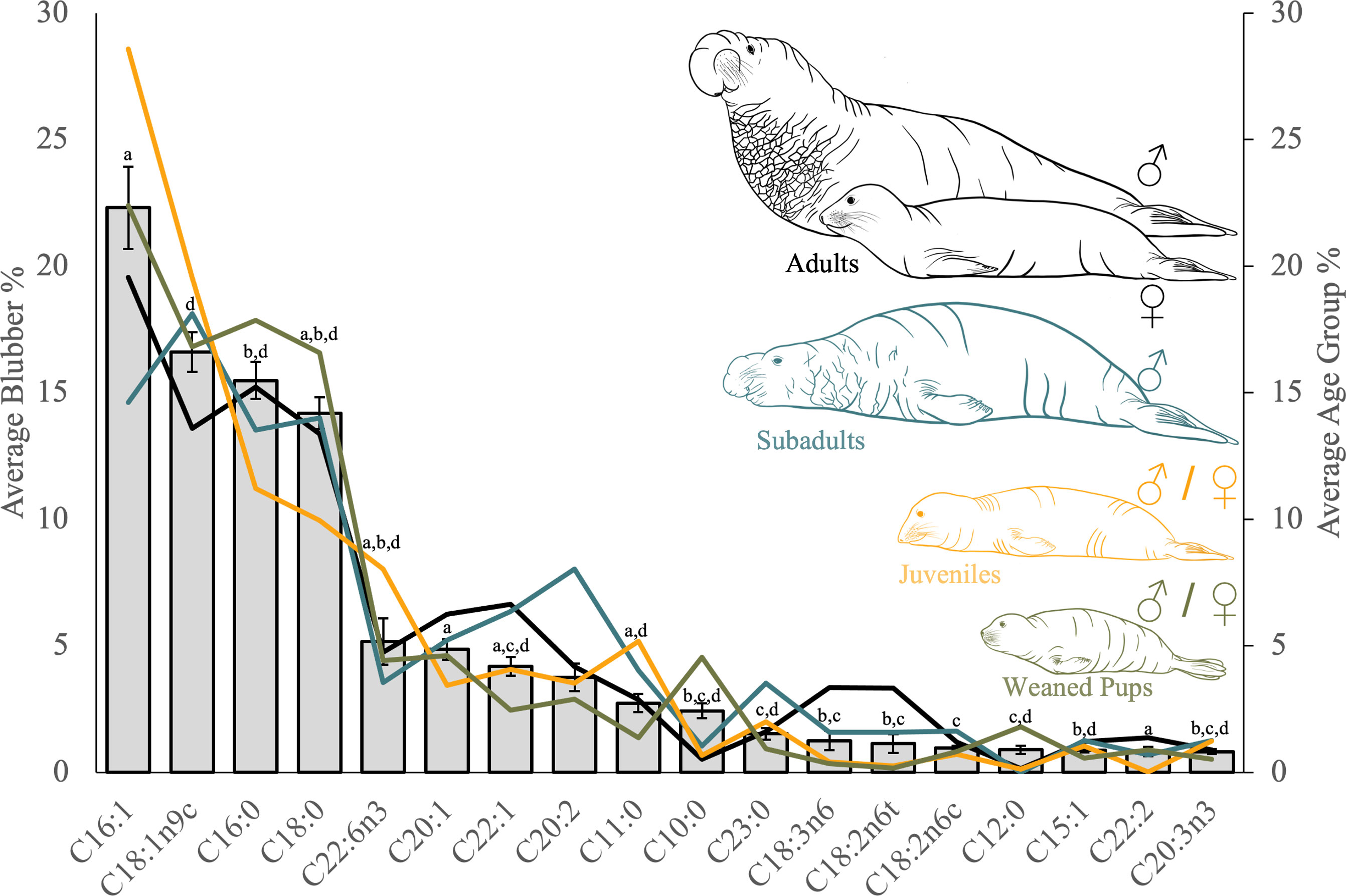
Figure 3 Blubber average (Bar plot ± S.E, primary axis) & age group averages (line plot, secondary axis; adults = black, subadult = blue, juvenile = orange, pup = green) for individual fatty acids ordered left to right by highest average proportion in blubber tissue. Statistical differences between age groups for individual fatty acids, are denoted by dissmilar superscript letters: a = adults and juveniles, b = adults and weaned pups, c = subadults and weaned pups, and d = juveniles and weaned pups.
The SIMPER analysis identified three FAs that contributed most to the difference between adults and juveniles FA profiles: C22:6n-3, C11:0 and C16:1 (Table S1). In comparison, the FAs contributing most to the difference between adults and weaned pups profiles were: C10:0, C16:1 and C22:1, although only C10:0 was significantly different (Table S2). Within subadults and weaned pups, C10:0, C20:2, C11:0 and C23:0 were the top contributing FAs, with only C10:0 and C23:0 reported as significantly different (Table S3). Finally, C10:0, C11:0 and C22:6n-3 were the top three individual FAs contributing to the difference between juveniles and weaned pups profiles, with all three reported as significantly different (Table S4).
Discussion
We provide the first evidence that the composition of blubber FA profiles differs across ontogenetic development as NES transition from young, developing seals to mature, fully developed adults. However, we found no sex-specific differences in blubber FAs at any life history stage. Our work adds to our understanding of intraspecific variation in blubber FA. Further, our findings are broadly concordant with FA patterns in the existing literature for NES blubber FAs in milk, weaned pups, and adult females (Noren et al., 2013; Fowler et al., 2014; Louis et al., 2015). Caution is required in the interpretations provided here as the opportunistic sampling did not allow for comparisons over the fast period, or within all the seasons required to definitively elucidate any sex differences.
General blubber trends
Here, we find that MUFAs are highest in proportion across all NES age classes and sexes, followed by SFAs and PUFAs. High proportions of MUFAs are typically found in the blubber of marine mammals, and the low melting point of MUFAs is thought to maintain fluidity in cold ocean habitats (Enser, 1984; Strandberg et al., 2008b; Knothe and Dunn, 2009; Guerrero and Rogers, 2017; Tverin et al., 2019). The second highest FA class, SFAs, provide more energy when oxidized, due to having fully saturated C-H bonds. For example, each molecule of palmitic acid (C16:0) provides two ATP more than its monounsaturated counterpart palmitoleic acid (C16:1; Coleman et al., 1993; Innis, 2005; Maillet and Weber, 2006; Trumble and Kanatous, 2012). Thus, on a bulk tissue basis, more SFA may lead to a meaningful physiological difference. Polyunsaturated fatty acids are essential fatty acids, as marine mammals cannot synthesize these, they are acquired and deposited from dietary FAs. The FAs in the blubber layer reflect diet and are deposited relatively unmodified into the blubber (Budge et al., 2004). The major FAs reported in NES prey were C18:1n-9, C22:1n-11, C20:1n-11, C16:0, C16:1n-9 & C22:6n3 (Goetsch et al., 2018). These same FAs contributed to the majority of the blubber FA composition in this study.
The individual MUFAs (C16:1, C18:1n-9c, C20:1), the SFAs (C16:0, C18:0), and the PUFA (C22:6n-3) were found in the highest proportions across all NES blubber FA profiles. In fact, these six individual FA accounted for nearly 80% of total blubber FAs regardless of age group. These FAs appear to be conserved across phocids, as these same individual FAs are commonly found in high proportions in many other pinnipeds, including Antarctic phocids (e.g., crabeater seals, Lobodon carcinophagus (Guerrero and Rogers, 2017); leopard seals, Hydrurga leptonyx (Guerrero et al., 2016); Weddell seals, Leptonychotes weddellii (Wheatley et al., 2008) and northern phocids (harbour seals, Phoca vitulina (Neises et al., 2021); ringed seals, Pusa hispida (Strandberg et al., 2008a); harp seals, Pagophilus groenlandicus (Haug et al., 2017); gray seals, Halichoerus grypus (Dannenberger et al., 2020).
Ontogenetic patterns in FA profiles
Weaned NES pups had similar levels of MUFAs to adults and subadults. However, weaned pups had the highest proportions of SFAs—and specifically, higher amounts of C16:0 and C18:0—compared to all the other age groups. Weaned pups also had the lowest proportions of PUFAs found in any life-history stage. Together, the high proportions of SFAs and low proportions of PUFAs indicate potential use of MUFAs and PUFAs in skeletal muscle to aid in diving development and to meet their daily metabolic energy requirements. Previously Louis et al. (2015) reported the major FAs mobilized from the inner blubber layer of NES pups during the post-weaning fast were made up of several PUFAs (C20:5n3, C18:3n6, C18:4n3, C20:4n6, & C20:4n3), SFAs (C10:0, and C16:0), and the MUFA C16:1n7. Several of these PUFAs were only found in trace amounts in this study (C20:5n3, C20:4n6, & C18:3n3); of these C20:5n3 and C20:4n6 are highly mobilized by pups, suggesting that these FAs were used to fuel pup development (Louis et al., 2015). PUFAs are important for post-weaning development as they act as precursors to bioactive molecules, structural growth, neurological development, and aid in physiological changes in skeletal muscle in preparation for diving (Castellini et al., 1994; Kanatous et al., 1999; Larque et al., 2002; Wheatley et al., 2008; Somo et al., 2015). Therefore, it is not surprising that weaned pups are mobilizing large amounts of PUFAs at this particular life history stage.
FA profiles of weaned pups were significantly different from those of all other age classes (e.g., adults, subadults, and juveniles). From birth, NES pups face significant challenges to survive, experiencing high energetic costs of developing from a terrestrial-born animal that must quickly become a deep-diving animal (Reiter et al., 1978; Noren, 2002; Noren et al., 2003; Gibson et al., 2020). Early on, due to their inability to forage, pups are fully dependent on the FAs transferred in the milk during lactation (Field et al., 2005; Noren et al., 2008; Fowler et al., 2014; Louis et al., 2015). The FA profiles of NES milk are primarily dominated by the MUFAs C18:1n9 and C20:1n11, and the SFA C16:0 (Fowler et al., 2014). Here ~57% of weaned pup blubber FA profiles were composed of the MUFA C18:1n9 and the SFAs C16:0 and C16:1; this reflects the direct transfer and deposition of these FA from the mother’s milk. Further, our findings match those from previous studies of NES pup blubber (Noren et al., 2013; Louis et al., 2015).
NES pups rely on blubber FAs to fuel their physiological development of diving while fasting, and to retain sufficient FAs to support thermoregulation once they go on their first at-sea foraging trip at two and a half months old (Noren, 2002). During the post-weaning fast, NES pups experience temperate air temperatures varying from 10°C to 27°C (Noren, 2002). In comparison phocid species, such as Weddell seals, inhabiting polar environments experience much colder temperatures. The warmer temperatures experienced by NES may potentially allow them to utilize more FAs for the physiological development when compared to a Weddell seal pup that must balance higher thermoregulation costs with development (Pearson et al., 2022).
During this study juvenile NES had the highest proportion of MUFAs in their blubber layer with the highest values of C16:1 and C18:1n-9 compared to all other age groups. Juveniles also had the lowest proportions of SFAs compared to all other age groups. As NES weaned pups transition to juveniles, their ability to catch prey and deposit FAs in the blubber is restricted by underdeveloped diving abilities, which, in turn, leads to reduced foraging efficiency during the first three trips to sea (Boeuf et al., 1996; Zeno et al., 2008). This period of life as a juvenile is also characterized by high mortality, as only half of all NES departing for the first foraging trip return to the colony the subsequent year (Thorson and Le Boeuf, 1994; Condit et al., 2014). The returning juveniles are a similar body mass as they were when they departed as pups, yet juveniles suffer a loss in total body lipids of up to 40% during their first 5-month trip to sea (Kretzmann et al., 1993). Thus, a thinner blubber layer and a higher surface area-to-volume ratio may increase heat conduction. As a result, juvenile NES may experience higher energetic demands to maintain thermoregulation compared to when they were pups (Brafield and Llewellyn, 1982), and likely retain MUFAs to maintain the fluidity of their blubber layer. Juveniles also experience high energetic demands as they need to continue to grow in size (Field et al., 2005; Kelso et al., 2012). Juveniles may offset the combined demands of thermoregulation and growth by the possible increased utilization of the relatively higher energy content of SFAs. This could aid in mitigating the effects of conflicting thermoregulation needs and the energetically costly physiological changes associated with growth in this life-history stage (Reiter et al., 1978; Kelso et al., 2012).
As juveniles transition to sexual maturity and adulthood, NES males and females have different life-history strategies that are associated with the development of secondary sexual characteristics and sexual size dimorphism (Kelso et al., 2012). NES females reach sexual maturity at four years old at which point they are classified as adult females as they begin breeding and giving birth to pups (Reiter et al., 1981). Breeding adult female NES experience the highest demands of any NES life-history stage, driven by the simultaneous production of milk during lactation and metabolic needs, with metabolic rates reaching 5.7x the predicted standard metabolic rate (SMR; Crocker et al., 2001). In our study, the samples collected from adult females came from the molt fast. In comparison to the breeding fast, the molt fast is not as energetically expensive; the SMR of adult females during the molt period is 2x the predicted SMR (Worthy et al., 1992; Wright et al., 2020). The lack of sex differences between adult northern elephant seals may be explained by the lack of adult female breeding samples.
Unlike females, male NES do not reach physical maturity until much later—around 8-10 years old (Condit et al., 2014). Male NESs are categorized into five groups (4 subadult groups plus adults) based on body size as well as the development of sexual characteristics like the proboscis and chest shield (Casey et al., 2020). The first four groups are considered as subadults differentiated by age, body size, and sexual characters. Subadult 1 males (SA1) are the smallest and most juvenile-like in appearance, and subadult 4 males (SA4s) are large, have a chest shield, and a large proboscis but have not reached full adult size yet. The final category of NES males are adults that are often 8 years and above (Boeuf and Laws, 1994). All the subadult males in this study were SA4s, the group closest in development and size to adult males (Casey et al., 2020). Here, we did not find any significant differences between the subadults and adult NES. This finding suggests that SA4 males are similar to adult males in terms of their development and metabolic requirements. Adult male NES fitness is related to body size; larger males are able to reduce their protein loss due to their large endogenous energy stores. In fact, the most dominant males (alphas) have the highest metabolic rates for NES males, up to 4x the predicted SMR (Crocker et al., 2012).
Adults and SA4s had the highest proportion of PUFAs in 6 of the 7 PUFAs analyzed (Table 2). Previously it has been hypothesized that PUFAs act as a potential oxygen conserving mechanism in diving phocids (Trumble et al., 2010; Trumble and Kanatous, 2012). However, while PUFAs increased across age groups here, NES sampled during this study were fasting on land. As such, more energy is needed to move their large mass in air and to support various costly behaviors associated with mating, defending harems during breeding or molting on land. We found adult NES of both sexes and male SA4s had the lowest proportions of short-chain SFAs (C10:0 & C12:0) and medium-chain SFAs (C16:0 & C18:0). As SFAs provide more energy when oxidized in comparison to their MUFA and PUFA counterparts, this suggests adults may rely on metabolizing short and medium-chain SFAs to obtain the higher energy provided (and required) during long fasting periods (Fowler et al., 2014). This may be supported by the lower proportions of SFAs reported here in adult and subadult blubber of NES and suggests NES burn SFAs during fasting periods while on land. Here our study is limited in interpretation due to the smaller sample sizes, and the inability to assess across each fasting period. Further studies of blubber FA changes across fasts in each age group are required to identify the definitive roles of blubber fatty acids in NES. For example, future studies using radiolabeled fatty acids may shed light on whether individual fatty acids are utilized by different sexes or age groups. Finally, investigating the energy content of each individual fatty acid and scaling to the appropriate mass of NES age groups and sex would help elucidate the energy use and differences in FA utilization across fasting periods.
In summary, age class, not sex, is the major driver of blubber FA profile differences in NES. We had hypothesized that there would be sex differences in FA profiles in this sexually dimorphic species but found no sex-specific changes in the blubber FA profiles of any NES age groups. This may not be reflected in blubber FA profiles, as blubber is the storage depot for FAs, thus, investigation of other tissues, such as skeletal muscle FA profiles (i.e., the site of FA oxidation), may reveal any potential sex differences in NES. However, the opportunistic sampling in this study may not have provided enough samples across comparable life-history stages to elucidate sex differences. Especially within adult northern elephant seals, here adult male samples covered both breeding and molting, while adult female samples were only available for the molt fast. Future studies should focus on acquiring larger sample sizes between sexes and detailed sampling across fasting seasons (e.g., breeding and molt), as this will provide more detailed information on the differences found in the FA profiles of NES.
Each ontogenetic stage in the NES life cycle shares a common, imperative need for lipid metabolism. From pups to fully grown mature adults, NES must constantly maintain sufficient stored FAs to survive and reproduce. Each life history stage is also interconnected to previous and future stages, making FA accumulation, mobilization, and storage an important process throughout an individual’s life. Further, any changes to this process can have cascading consequences throughout ontogeny in this species.
Pups, for example, are entirely dependent on the FAs provided by adult females during lactation (Noren et al., 2003). Thus, if females return to the breeding fast with inadequate energy stores, the lactation period may be cut short, as adult females leave to replenish their own total body energy stores (Crocker et al., 2001). Weaned pups with reduced or minimal energy stores may not be able to survive the entire post-weaning fast, instead leave early to begin foraging (Reiter et al., 1978). First-year mortality has been reported as high as upwards of 60%; therefore any further increase in juvenile mortality could reduce the success and overall numbers of weaned pups that transition to sexually mature adults (Reiter et al., 1978; Boeuf et al., 1996). Some adult NES, for example, spend 80 to 100% of their time continuously foraging during the post-breeding migration. In highly variable years, such as during extreme climatic events like El Nino, females have been shown to return from foraging periods in a negative energy balance (Adachi et al., 2021). In these years, adult females spent more time travelling between prey patches than other years, affecting mass gain, and resulting in the lowest mass gain measurements in 15 years (Crocker et al., 2006). More broadly, these patterns suggest that other broad-scale environmental changes—like those caused by anthropogenic climate change—may directly affect the ability of NES across multiple age classes to successfully acquire, maintain, and store energy stores needed for life.
Conclusions
Here we reported the major blubber fatty acids found across NES age groups are similar to other marine mammals. As NES develop from terrestrial-born pups to elite deep-diving adults, blubber fatty acid profiles change such that younger developing seal blubber FA profiles are different in comparison to older mature NES. Monounsaturated fatty acids were the most prevalent fatty acid class, followed by saturated and polyunsaturated fatty acids. During fasting, NES rely on the fatty acids stored in their blubber to meet most of their metabolic needs, subsequently, they may utilize the higher energy content of SFAs. Further detailed sampling, including radiolabeled fatty acids, may help to identify definitive changes across fasting periods.
Data availability statement
The datasets presented in this study can be found in online repositories. The names of the repository/repositories and accession number(s) can be found below: https://doi.org/10.6084/m9.figshare.19758541.v3.
Ethics statement
The animal study was reviewed and approved by Sonoma State University Institutional Animal Care and Use Committee under NMFS permits 14636 and 19108.
Author contributions
ST, SK, and DC contributed to the conception and design of the study. ST, SK, AK and DC all contributed to sample collection. AK, and DC organized the database ensuring all data was accompanied with the correct metadata for analysis. AK performed all laboratory work and data analysis at Baylor University. AK, KS, SK, SSK & ST contributed to the interpretation of the data. AK wrote the manuscript; all authors contributed to reading, revising, and approving the final manuscript.
Acknowledgments
We thank all from UCSC, Sonoma State, Colorado State, and Año Nuevo State Reserve who helped in collecting samples in the field. We would also like to thank Patrick Charapata for developing and helping run the cotton correction along with Emily Sperou. AK would like to thank Dr. José Vázquez-Medina for his valuable mentorship during this process.
Conflict of interest
The authors declare that the research was conducted in the absence of any commercial or financial relationships that could be construed as a potential conflict of interest.
Publisher’s note
All claims expressed in this article are solely those of the authors and do not necessarily represent those of their affiliated organizations, or those of the publisher, the editors and the reviewers. Any product that may be evaluated in this article, or claim that may be made by its manufacturer, is not guaranteed or endorsed by the publisher.
Supplementary material
The Supplementary Material for this article can be found online at: https://www.frontiersin.org/articles/10.3389/fmars.2022.942711/full#supplementary-material
References
Adachi T., Takahashi A., Costa D. P., Robinson P. W., Hückstädt L. A., Peterson S. H., et al. (2021). Forced into an ecological corner: Round-the-clock deep foraging on small prey by elephant seals. Sci. Adv. 7, eabg3628. doi: 10.1126/sciadv.abg3628
Anderson M. J. (2017). ““Permutational multivariate analysis of variance (PERMANOVA),” in Wiley StatsRef: Statistics reference online,”. Eds. Balakrishnan N., Colton T., Everitt B., Piegorsch W., Ruggeri F., Teugels J. L. (Chichester, UK: John Wiley & Sons, Ltd), 1–15. doi: 10.1002/9781118445112.stat07841
Berg J. M., Tymoczko J. L., Stryer L. (2002)Food intake and starvation induce metabolic changes. In: Biochemistry. 5th edition. Available at: https://www.ncbi.nlm.nih.gov/books/NBK22414/ (Accessed September 16, 2021).
Boeuf B. J. L., Laws R. M. (1994). Elephant seals: Population ecology, behavior, and physiology (University of California Press).
Boeuf B. J. L., Morris P. A., Blackwell S. B., Crocker D. E., Costa D. P. (1996). Diving behavior of juvenile northern elephant seals. Can. J. Zool. 74, 1632–1644. doi: 10.1139/z96-181
Boeuf B. J. L., Naito Y., Huntley A. C., Asaga T. (1989). Prolonged, continuous, deep diving by northern elephant seals. Can. J. Zool. 67, 2514–2519. doi: 10.1139/z89-355
Boyd I., Arnbom T., Fedak M. (1993). Water flux, body composition, and metabolic rate during molt in female southern elephant seals (Mirounga leonina). Physiol. Zool. 66, 43–60. doi: 10.1086/physzool.66.1.30158286
Budge S. M., Cooper M. H., Iverson S. J. (2004). Demonstration of the deposition and modification of dietary fatty acids in pinniped blubber using radiolabelled precursors. Physiol. Biochem. Zool. 77, 682–687. doi: 10.1086/420945
Budge S. M., Iverson S. J., Koopman H. N. (2006). Studying trophic ecology in marine ecosystems using fatty acids: A primer on analysis and interpretation. Mar. Mammal. Sci. 22, 759–801. doi: 10.1111/j.1748-7692.2006.00079.x
Casey C., Charrier I., Mathevon N., Nasr C., Forman P., Reichmuth C. (2020). The genesis of giants: behavioural ontogeny of male northern elephant seals. Anim. Behav. 166, 247–259. doi: 10.1016/j.anbehav.2020.06.014
Castellini M. A., Rea L. D. (1992). The biochemistry of natural fasting at its limits. Experientia 48, 575–582. doi: 10.1007/BF01920242
Castellini M. A., Rea L. D., Sanders J. L., Castellini J. M., Zenteno-Savin T. (1994). Developmental changes in cardiorespiratory patterns of sleep-associated apnea in northern elephant seals. Am. J. Physiol.-Regulatory Integr. Comp. Physiol. 267, R1294–R1301. doi: 10.1152/ajpregu.1994.267.5.R1294
Champagne C. D., Crocker D. E., Fowler M. A., Houser D. S. (2012). ““Fasting physiology of the pinnipeds: The challenges of fasting while maintaining high energy expenditure and nutrient delivery for lactation,”,” in Comparative physiology of fasting, starvation, and food limitation. Ed. McCue M. D. (Berlin, Heidelberg: Springer Berlin Heidelberg), 309–336. doi: 10.1007/978-3-642-29056-5_19
Champagne C. D., Houser D. S., Crocker D. E. (2006). Glucose metabolism during lactation in a fasting animal, the northern elephant seal. Am. J. Physiol.-Regulatory Integr. Comp. Physiol. 291, R1129–R1137. doi: 10.1152/ajpregu.00570.2005
Clinton W. L., Boeuf B. J. L. (1993). Sexual selection’s effects on Male life history and the pattern of Male mortality. Ecology 74, 1884–1892. doi: 10.2307/1939945
Coleman R. A., Florant G. L., Mostafa N., Xia T., Bhat B. C. (1993). ““Molecular mechanisms for selective retention of essential fatty acids during hibernation,”,” in Life in the cold (CRC Press).
Condit R., Le Boeuf B. J. (1984). Feeding habits and feeding grounds of the northern elephant seal. J. Mammal. 65, 281–290. doi: 10.2307/1381167
Condit R., Reiter J., Morris P. A., Berger R., Allen S. G., Le Boeuf B. J. (2014). Lifetime survival rates and senescence in northern elephant seals. Mar. Mammal. Sci. 30, 122–138. doi: 10.1111/mms.12025
Costa D. P., Boeuf B. J. L., Huntley A. C., Ortiz C. L. (1986). The energetics of lactation in the northern elephant seal, mirounga angustirostris. J. Zool. 209, 21–33. doi: 10.1111/j.1469-7998.1986.tb03563.x
Crocker D. E., Champagne C. D., Fowler M. A., Houser D. S. (2014). Adiposity and fat metabolism in lactating and fasting northern elephant seals. Adv. Nutr. 5, 57–64. doi: 10.3945/an.113.004663
Crocker D., Costa D., Le Boeuf B., Webb P., Houser D. (2006). Impact of El niño on the foraging behavior of female northern elephant seals. Mar. Ecol. Prog. Ser. 309, 1–10. doi: 10.3354/meps309001
Crocker D. E., Houser D. S., Webb P. M. (2012). Impact of body reserves on energy expenditure, water flux, and mating success in breeding Male northern elephant seals. Physiol. Biochem. Zool. 85, 11–20. doi: 10.1086/663634
Crocker D. E., Khudyakov J. I., Champagne C. D. (2016). Oxidative stress in northern elephant seals: Integration of omics approaches with ecological and experimental studies. Comp. Biochem. Physiol. Part A.: Mol. Integr. Physiol. 200, 94–103. doi: 10.1016/j.cbpa.2016.02.011
Crocker D. E., Williams J. D., Costa D. P., Boeuf B. J. L. (2001). Maternal traits and reproductive effort in northern elephant seals. Ecology 82, 15. doi: 10.1890/0012-9658(2001)082[3541:MTAREI]2.0.CO;2
Dannenberger D., Möller R., Westphal L., Moritz T., Dähne M., Grunow B. (2020). Fatty acid composition in blubber, liver, and muscle of marine mammals in the southern Baltic Sea. Animals 10, 1509. doi: 10.3390/ani10091509
Davis R. (2019). Marine mammals: Adaptations for an aquatic life. Springer Cham. doi: 10.1007/978-3-319-98280-9
De Luca Peña L. V., Taelman S. E., Préat N., Boone L., van der Biest K., Custódio M., et al. (2022). Towards a comprehensive sustainability methodology to assess anthropogenic impacts on ecosystems: Review of the integration of life cycle assessment, environmental risk assessment and ecosystem services assessment. Sci. Total Environ. 808, 152125. doi: 10.1016/j.scitotenv.2021.152125
Deutsch C. J., Haley M. P., Le Boeuf B. J. (1990). Reproductive effort of male northern elephant seals: estimates from mass loss. Can. J. Zool. 68, 2580–2593. doi: 10.1139/z90-360
du Pontavice H., Gascuel D., Reygondeau G., Maureaud A., Cheung W. W. L. (2020). Climate change undermines the global functioning of marine food webs. Global Change Biol. 26, 1306–1318. doi: 10.1111/gcb.14944
Enser M. (1984). ““2 - THE CHEMISTRY, BIOCHEMISTRY AND NUTRITIONAL IMPORTANCE OF ANIMAL FATS,”,” in Fats in animal nutrition. Ed. Wiseman J. (Butterworth-Heinemann), 23–51. doi: 10.1016/B978-0-408-10864-5.50008-5
Field I. C., Bradshaw C. J. A., Burton H. R., Hindell M. A. (2005). Juvenile southern elephant seals exhibit seasonal differences in energetic requirements and use of lipids and protein stores. Physiol. Biochem. Zool. 78, 491–504. doi: 10.1086/430227
Folch J., Lees M., Sloane Stanley G. H. (1957). A simple method for the isolation and purification of total lipides from animal tissues. J. Biol. Chem. 226, 497–509. doi: 10.1016/S0021-9258(18)64849-5
Fowler M., Champagne C., Crocker D. (2018). Adiposity and fat metabolism during combined fasting and lactation in elephant seals. J. Exp. Biol. 221, jeb161554. doi: 10.1242/jeb.161554
Fowler M. A., Debier C., Mignolet E., Linard C., Crocker D. E., Costa D. P. (2014). Fatty acid mobilization and comparison to milk fatty acid content in northern elephant seals. J. Comp. Physiol. B. 184, 125–135. doi: 10.1007/s00360-013-0787-7
Fredheim B., Holen S., Ugland K. I., Grahl-Nielsen O. (1995). “Fatty acid composition in blubber, heart and brain from phocid seals,” in Developments in marine biology whales, seals, fish and man. Eds. Blix A. S., Walløe L., Ulltang Ø. (Elsevier), 153–168. doi: 10.1016/S0163-6995(06)80019-3
Gibson E., Torres-Velarde J. M., Vazquez-Medina J. P., Crocker D. (2020). Prolonged fasting increases DNA methylation in northern elephant seal pups. FASEB J. 34, 1–1. doi: 10.1096/fasebj.2020.34.s1.04334
Glandon H. L., Loh A. N., McLellan W. A., Pabst D. A., Westgate A. J., Koopman H. N. (2021). Lipid signature of neural tissues of marine and terrestrial mammals: consistency across species and habitats. J. Comp. Physiol. B. 191, 815–829. doi: 10.1007/s00360-021-01373-x
Goetsch C., Conners M. G., Budge S. M., Mitani Y., Walker W. A., Bromaghin J. F., et al. (2018). Energy-rich mesopelagic fishes revealed as a critical prey resource for a deep-diving predator using quantitative fatty acid signature analysis. Front. Mar. Sci. 5. doi: 10.3389/fmars.2018.00430
Grahl-Nielsen O., Haug T., Lindstrøm U., Nilssen K. (2011). Fatty acids in harp seal blubber do not necessarily reflect their diet. Mar. Ecol. Prog. Ser. 426, 263–276. doi: 10.3354/meps09011
Groscolas R., Robin J.-P. (2001). Long-term fasting and re-feeding in penguins. Comp. Biochem. Physiol. Part A.: Mol. Integr. Physiol. 128, 643–653. doi: 10.1016/S1095-6433(00)00341-X
Guerrero A. I., Negrete J., Márquez M. E. I., Mennucci J., Zaman K., Rogers T. L. (2016). Vertical fatty acid composition in the blubber of leopard seals and the implications for dietary analysis. J. Exp. Mar. Biol. Ecol. 478, 54–61. doi: 10.1016/j.jembe.2016.02.004
Guerrero A. I., Rogers T. L. (2017). Blubber fatty acid composition and stratification in the crabeater seal, lobodon carcinophaga. J. Exp. Mar. Biol. Ecol. 491, 51–57. doi: 10.1016/j.jembe.2017.03.004
Haug T., Falk-Petersen S., Greenacre M., Hop H., Lindstrøm U., Meier S., et al. (2017). Trophic level and fatty acids in harp seals compared with common minke whales in the barents Sea. Mar. Biol. Res. 13, 919–932. doi: 10.1080/17451000.2017.1313988
Hückstädt L. A., Piñones A., Palacios D. M., McDonald B. I., Dinniman M. S., Hofmann E. E., et al. (2020). Projected shifts in the foraging habitat of crabeater seals along the Antarctic peninsula. Nat. Clim. Change 10, 472–477. doi: 10.1038/s41558-020-0745-9
Innis S. M. (2005). “Chapter 10 essential fatty acid metabolism during early development,” in Biology of growing animals biology of metabolism in growing animals. Eds. Burrin D. G., Mersmann H. J. (Elsevier), 235–274. doi: 10.1016/S1877-1823(09)70017-7
Iverson S. J. (2009). “Blubber,” in Encyclopedia of marine mammals (Second edition). Eds. Perrin W. F., Würsig B., Thewissen J. G. M. (London: Academic Press), 115–120. doi: 10.1016/B978-0-12-373553-9.00032-8
Kanatous S. B., DiMichele L. V., Cowan D. F., Davis R. W. (1999). High aerobic capacities in the skeletal muscles of pinnipeds: adaptations to diving hypoxia. J. Appl. Physiol. 86, 1247–1256. doi: 10.1152/jappl.1999.86.4.1247
Kelso E. J., Champagne C. D., Tift M. S., Houser D. S., Crocker D. E. (2012). Sex differences in fuel use and metabolism during development in fasting juvenile northern elephant seals. J. Exp. Biol. 215, 2637–2645. doi: 10.1242/jeb.068833
Knothe G., Dunn R. O. (2009). A comprehensive evaluation of the melting points of fatty acids and esters determined by differential scanning calorimetry. J. Am. Oil Chem. Soc. 86, 843–856. doi: 10.1007/s11746-009-1423-2
Kooyman G. L. (1973). Respiratory adaptations in marine mammals. Am. Zool. 13, 457–468. doi: 10.1093/icb/13.2.457
Kretzmann M. B., Costa D. P., Boeuf B. J. (1993). Maternal energy investment in elephant seal pups: evidence for sexual equality? Am. Nat. 141, 466–480. doi: 10.1086/285484
Larque E., Demmelmair H., Koletzko B. (2002). Perinatal supply and metabolism of long-chain polyunsaturated fatty acids. Ann. New York Acad. Sci. 967, 299–310. doi: 10.1111/j.1749-6632.2002.tb04285.x
Le Boeuf B. J. (1974). Male-Male competition and reproductive success in elephant seals. Am. Zool. 14, 163–176. doi: 10.1093/icb/14.1.163
Le Boeuf B. J., Crocker D. E. (1996). CHAPTER 17 - Diving Behavior of Elephant Seals: Implications for Predator Avoidance, Editor(s): Peter Klimley A., Ainley D. G., Great White Sharks, Academic Press, 193–205. doi: 10.1016/B978-012415031-7/50018-5
Liwanag H. E. M., Berta A., Costa D. P., Budge S. M., Williams T. M. (2012). Morphological and thermal properties of mammalian insulation: the evolutionary transition to blubber in pinnipeds. Biol. J. Linn Soc. 107, 774–787. doi: 10.1111/j.1095-8312.2012.01992.x
Louis C., Perdaens L., Suciu S., Tavoni S. K., Crocker D. E., Debier C. (2015). Mobilisation of blubber fatty acids of northern elephant seal pups (Mirounga angustirostris) during the post-weaning fast. Comp. Biochem. Physiol. Part A.: Mol. Integr. Physiol. 183, 78–86. doi: 10.1016/j.cbpa.2015.01.008
Maillet D., Weber J.-M. (2006). Performance-enhancing role of dietary fatty acids in a long-distance migrant shorebird: the semipalmated sandpiper. J. Exp. Biol. 209, 2686–2695. doi: 10.1242/jeb.02299
McCue M. D., Terblanche J. S., Benoit J. B. (2017). Learning to starve: impacts of food limitation beyond the stress period. J. Exp. Biol. 220, 4330–4338. doi: 10.1242/jeb.157867
Mueter F. J., Planque B., Hunt G. L., Alabia I. D., Hirawake T., Eisner L., et al. (2021). Possible future scenarios in the gateways to the Arctic for subarctic and Arctic marine systems: II. prey resources, food webs, fish, and fisheries. ICES J. Mar. Sci. 78, 3017–3045. doi: 10.1093/icesjms/fsab122
Neises V. M., Karpovich S. A., Keogh M. J., King R. S., Trumble S. J. (2021). Regional, seasonal and age class blubber fatty acid signature analysis of harbour seals in Alaska from 1997 to 2010. Conserv. Physiol. 9, coab036. doi: 10.1093/conphys/coab036
Noren D. P. (2002). Thermoregulation of weaned northern elephant seal ( Mirounga angustirostris ) pups in air and water. Physiol. Biochem. Zool. 75, 513–523. doi: 10.1086/342254
Noren S. R. (2020). Postnatal development of diving physiology: implications of anthropogenic disturbance for immature marine mammals. J. Exp. Biol. 223:1–11. doi: 10.1242/jeb.227736
Noren D. P., Budge S. M., Iverson S. J., Goebel M. E., Costa D. P., Williams T. M. (2013). Characterization of blubber fatty acid signatures in northern elephant seals (Mirounga angustirostris) over the postweaning fast. J. Comp. Physiol. B. 183, 1065–1074. doi: 10.1007/s00360-013-0773-0
Noren D. P., Crocker D. E., Williams T. M., Costa D. P. (2003). Energy reserve utilization in northern elephant seal (Mirounga angustirostris) pups during the postweaning fast: size does matter. J. Comp. Physiol. B. Biochem. Syst. Environ. Physiol. 173, 443–454. doi: 10.1007/s00360-003-0353-9
Noren S. R., Pearson L. E., Davis J., Trumble S. J., Kanatous S. B. (2008). Different thermoregulatory strategies in nearly weaned pup, yearling, and adult weddell seals ( Leptonychotes weddelli ). Physiol. Biochem. Zool. 81, 868–879. doi: 10.1086/588489
Pearson L. E., Weitzner E. L., Tomanek L., Liwanag H. E. M. (2022). Metabolic cost of thermoregulation decreases after the molt in developing weddell seal pups. J. Exp. Biol. 225 (6), jeb.242773. doi: 10.1242/jeb.242773
Peck H. E., Costa D. P., Crocker D. E. (2016). Body reserves influence allocation to immune responses in capital breeding female northern elephant seals. Funct. Ecol. 30, 389–397. doi: 10.1111/1365-2435.12504
Pond C. M. (1978). Morphological aspects and the ecological and mechanical consequences of fat deposition in wild vertebrates. Annu. Rev. Ecol. Systematics 9, 519–570. doi: 10.1146/annurev.es.09.110178.002511
Rea L. D. (1995) Prolonged fasting in pinnipeds. Available at: https://www.proquest.com/docview/304173237/abstract/5710DA85E4D94413PQ/1 (Accessed July 3, 2021).
Reiter J., Panken K. J., Le Boeuf B. J. (1981). Female competition and reproductive success in northern elephant seals. Anim. Behav. 29, 670–687. doi: 10.1016/S0003-3472(81)80002-4
Reiter J., Stinson N. L., Boeuf B. J. L. (1978). Northern elephant seal development: The transition from weaning to nutritional independence. Behav. Ecol. Sociobiol. 3, 337–367. doi: 10.1007/BF00303199
Robinson P. W., Costa D. P., Crocker D. E., Gallo-Reynoso J. P., Champagne C. D., Fowler M. A., et al. (2012). Foraging behavior and success of a mesopelagic predator in the northeast pacific ocean: Insights from a data-rich species, the northern elephant seal. PloS One 7, e36728. doi: 10.1371/journal.pone.0036728
Ryg M., Smith T. G., Øritsland N. A. (1988). Thermal significance of the topographical distribution of blubber in ringed seals ( Phoca hispida ). Can. J. Fish. Aquat. Sci. 45, 985–992. doi: 10.1139/f88-121
Scholander P. F., Walters V., Hock R., Irving L. (1950). BODY INSULATION OF SOME ARCTIC AND TROPICAL MAMMALS AND BIRDS. Biol. Bull. 99, 225–236. doi: 10.2307/1538740
Sharick J. T., Vazquez-Medina J. P., Ortiz R. M., Crocker D. E. (2015). Oxidative stress is a potential cost of breeding in male and female northern elephant seals. Funct. Ecol. 29, 367–376. doi: 10.1111/1365-2435.12330
Somo D. A., Ensminger D. C., Sharick J. T., Kanatous S. B., Crocker D. E. (2015). Development of dive capacity in northern elephant seals (Mirounga angustirostris): Reduced body reserves at weaning are associated with elevated body oxygen stores during the postweaning fast. Physiol. Biochem. Zool. 88, 471–482. doi: 10.1086/682386
Strandberg U., Käkelä A., Lydersen C., Kovacs K. M., Grahl-Nielsen O., Hyvärinen H., et al. (2008a). Stratification, composition, and function of marine mammal blubber: The ecology of fatty acids in marine mammals. Physiol. Biochem. Zool. 81, 473–485. doi: 10.1086/589108
Strandberg U., Käkelä A., Lydersen C., Kovacs K. M., Grahl-Nielsen O., Hyvärinen H., et al. (2008b). Stratification, composition, and function of marine mammal blubber: The ecology of fatty acids in marine mammals. Physiol. Biochem. Zool. 81, 473–485. doi: 10.1086/589108
Thorson P. H., Le Boeuf B. J. (1994). “Fifteen. developmental aspects of diving in northern elephant seal pups,” in Elephant seals. Eds. Le Boeuf B.J., Laws R. M. (University of California Press), 271–289. doi: 10.1525/9780520328150-017
Trumble S. J., Kanatous S. B. (2012). Fatty acid use in diving mammals: More than merely fuel. Front. Physiol. 3. doi: 10.3389/fphys.2012.00184
Trumble S. J., Noren S. R., Cornick L. A., Hawke T. J., Kanatous S. B. (2010). Age-related differences in skeletal muscle lipid profiles of weddell seals: clues to developmental changes. J. Exp. Biol. 213, 1676–1684. doi: 10.1242/jeb.040923
Tverin M., Westberg M., Kokkonen I., Tang P., Lehmann P., Lundström K., et al. (2019). Factors affecting the degree of vertical stratification of fatty acids in grey seal blubber. Mar. Biol. 166, 105. doi: 10.1007/s00227-019-3556-7
Wheatley K. E., Nichols P. D., Hindell M. A., Harcourt R. G., Bradshaw C. J. A. (2008). Differential mobilization of blubber fatty acids in lactating weddell seals: Evidence for selective use. Physiol. Biochem. Zool. 81, 651–662. doi: 10.1086/590397
Worthy G. A. J., Morris P. A., Costa D. P., Boeuf B. J. L. (1992). Moult energetics of the northern elephant seal (Mirounga angustirostris). J. Zool. 227, 257–265. doi: 10.1111/j.1469-7998.1992.tb04821.x
Wright T. J., Davis R. W., Holser R. R., Hückstädt L. A., Danesi C. P., Porter C., et al. (2020). Changes in northern elephant seal skeletal muscle following thirty days of fasting and reduced activity. Front. Physiol. 11. doi: 10.3389/fphys.2020.564555
Keywords: fasting, pinniped, adipose, lipid metabolism, phocid development, marine predator, sexual dimorphism
Citation: Kirkpatrick AW, Crocker DE, Kanatous SB, Smith KJ, Kienle SS and Trumble SJ (2022) Age group differences in blubber fatty acid profiles in northern elephant seals (Mirounga angustirostris). Front. Mar. Sci. 9:942711. doi: 10.3389/fmars.2022.942711
Received: 12 May 2022; Accepted: 09 August 2022;
Published: 24 August 2022.
Edited by:
Davina Derous, University of Aberdeen, United KingdomReviewed by:
Randall William Davis, Texas A&M University at Galveston, United StatesMelinda Fowler, Springfield College, United States
Copyright © 2022 Kirkpatrick, Crocker, Kanatous, Smith, Kienle and Trumble. This is an open-access article distributed under the terms of the Creative Commons Attribution License (CC BY). The use, distribution or reproduction in other forums is permitted, provided the original author(s) and the copyright owner(s) are credited and that the original publication in this journal is cited, in accordance with accepted academic practice. No use, distribution or reproduction is permitted which does not comply with these terms.
*Correspondence: Aaron W. Kirkpatrick, YWFyb24udy5raXJrcGF0cmlja0BnbWFpbC5jb20=; Stephen J. Trumble, c3RlcGhlbl90cnVtYmxlQGJheWxvci5lZHU=