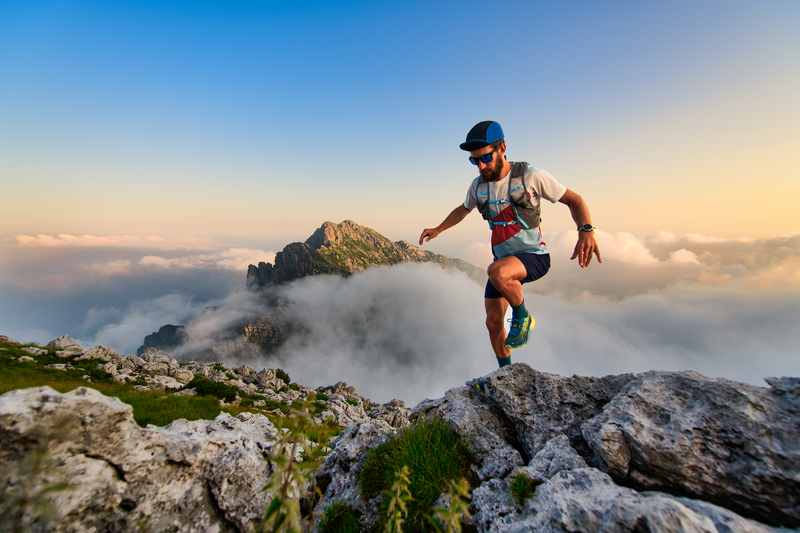
95% of researchers rate our articles as excellent or good
Learn more about the work of our research integrity team to safeguard the quality of each article we publish.
Find out more
REVIEW article
Front. Mar. Sci. , 07 October 2022
Sec. Aquatic Physiology
Volume 9 - 2022 | https://doi.org/10.3389/fmars.2022.939239
This article is part of the Research Topic Methods and Applications in Aquatic Physiology View all 7 articles
With regional hypoxic episodes in the world’s oceans becoming more severe and more frequent, a valuable management need will be a screening protocol to characterize the full diversity of hypoxic robustness among fishes (defined here as their tolerance and performance). Yet, high-throughput screening protocols to deal with the almost 33,000 fish species have the potential to trade off convenience with the information depth normally provided with comprehensive but more time-consuming phenotyping protocols. As a solution, we propose a two-tier testing approach, one that combines a simple, rapid, cost-effective, and well-established hypoxia challenge test (a measure of hypoxic tolerance) with an individual-based hypoxic performance test that defines maximum aerobic capacity over a wide range of ambient water oxygen levels (a hypoxic performance curve). The later test would be performed on selected indicator species identified from the tolerance screening test. Here we describe the two methodological approaches for this two-tier approach using some existing data to illustrate the potential for their applications in marine science.
Compared with the aerial environment, aquatic organisms live in an environment with more limited oxygen (O2) availability. Moreover, with rising water temperature, eutrophication, and coastal upwelling, ambient O2 availability in aquatic ecosystems is being further reduced (i.e., aquatic hypoxia). Indeed, regional hypoxic episodes in the world’s oceans are becoming more severe and more frequent (Schmidtko et al., 2017). Yet, fish are by far the most diverse vertebrate group, with around 33,000 species, and have evolved to inhabit nearly all types of aquatic habitats on our planet. In fact, the rich history of physiological studies of hypoxia tolerance and performance on a relatively small number of fish species has already informed us that fishes possess a broad repertoire of mechanisms to cope with hypoxic situations and sustain their cellular O2 supply. Many of these coping mechanisms can be formalized within the physiological concept of an O2 cascade system, which breaks down the O2 gradient from ambient water to mitochondria as a series of mechanistic and progressive steps (ventilation, gill diffusion, circulation, tissue diffusion, and cellular respiration).
When faced with short-term, moderate hypoxia (O2 level sustains 50% aerobic scope), a fish’s first line of defense to maintain the arterial blood O2 content as high as possible despite reduced O2 availability involves many physiological compensations that can be turned on within minutes. For example, hyperventilation of the gills and changes in blood perfusion within and among gill secondary lamellae (Holeton and Randall, 1967; Farrell et al., 1980; Sundin, 1995) can improve the rate of O2 diffusion into the blood. Hypoxic bradycardia (Randall and Shelton, 1963; Farrell, 2007) can increase the residence time of blood in lamellae, again favoring O2 diffusion. A leftward shift of the hemoglobin equilibrium curve (Wells et al., 1989) also favors blood O2 loading at the gills. A splenic release of red blood cell stores (Gallaugher et al., 1992; Soldatov, 1996) elevates arterial blood O2-carrying capacity, and an increased venous tone (Sandblom and Axelsson, 2006) improves ventricular filling to maintain cardiac output to the gills (see Fish Physiology: Hypoxia eds by Richards et al., 2009 for a full range of the known mechanisms in fishes). As a result, fish can not only tolerate moderately hypoxic aquatic environments but also successfully inhabit or exploit them, e.g., sculpins (Myoxocephalus scorpius) (Mandic et al., 2009) and European sea bass (Dicentrarchus labrax) (Pickett and Pawson, 1995).
With deeper hypoxic exposure, these physiological compensatory functions begin to fail in the ability to support the full aerobic scope of a fish in normoxia (here we define full aerobic scope as the maximum O2 uptake achievable in normoxia less the very basic O2 need of a fish, its standard metabolic rate, SMR). Thus, some activities must be curtailed in deep hypoxia (Farrell and Richards, 2009). Eventually, at some level of hypoxia, which is known to vary substantially among fishes, the O2 tension of the water (PO2) is so severe that the O2 cascade can no longer support a sufficient flux of O2 to mitochondria to support even SMR. The lowest water partial pressure of O2 when SMR can be maintained is called the critical O2 tension (Pcrit; also termed critical O2 concentration, Ccrit; critical O2 saturation, O2crit), a well-established physiological measure of hypoxia tolerance and one that can be experimentally standardized (Fry, 1947; Claireaux and Chabot, 2016). Implicit in this definition is that a fish at Pcrit has no aerobic scope, which means the fish has no access to spare O2 to perform the activities that are possible at a higher ambient PO2. Consequently, aerobic scope and a fish’s ability to perform activities beyond SMR fail progressively with a decreasing ambient PO2.
Below Pcrit, fishes can still recruit physiological mechanisms that can temporarily resist extreme hypoxia. Notably, substrate-level phosphorylation can be turned on (Hochachka et al., 1996). Also, the “pilot light of life” (i.e., SMR, the basic metabolic needs) can be suppressed to some degree through a variety of mechanisms, collectively called metabolic suppression (Hochachka et al., 1996; Vornanen et al., 2009; Jackson and Ultsch, 2010). Nevertheless, at such levels of extreme hypoxia, a fish is living on borrowed time because neither feeding nor reproduction is possible. Furthermore, end products of glycolytic metabolism (which can be noxious) are accumulating. All the same, a few fish species are genetically adapted for prolonging life in extremely hypoxic or even anoxic waters: crucian carp and goldfish metabolically convert the accumulating lactate and H+ to ethanol which can be excreted into the water via the gills (Shoubridge and Hochachka, 1980; Stecyk et al., 2004), or they enter a state of dormancy with a 90% suppression of SMR thereby reducing the production of harmful metabolic end products (see reviews by Vornanen et al., 2009; Stecyk, 2017). At the most extreme level of hypoxia, a fish’s righting reflex fails, and it can no longer maintain an upright posture in the water, termed a loss of equilibrium (LOE), another measure of hypoxia tolerance that can be experimentally standardized. Although a continuum from hypoxic performance (e.g., a percentage of aerobic scope in normoxia) to hypoxic tolerance (e.g., LOE) was measured separately in previous experiments (as shown in the case studies), they are on the same continuum of the limiting oxygenation level curve (Claireaux and Chabot, 2016).
While much is known about a fish’s general compensatory and coping mechanisms in hypoxia and their level of tolerance to extreme levels of hypoxia (Pcrit and LOE), relatively little quantitative information is available on how aerobic capacity becomes constrained by hypoxia other than at Pcrit. Yet, this is the very knowledge gap that we need to fill to better understand what activities might be possible under different severities of hypoxia exposures. Hence, there is a need for a hypoxic performance curve for aerobic scope (much like the thermal performance curve) coupled with measures of hypoxia tolerance. Very few of the almost 33,000 fish species have been studied to date. However, even this limited species representation suggests that hypoxia coping mechanisms can vary substantially and species-specifically (Dhillon et al., 2013; Mandic and Regan, 2018). Further complexity is that a fish acclimates to hypoxic water over time (typically weeks, but possibly shorter), allowing them to perform better than they might have with a similar acute hypoxic challenge. For example, hypoxic acclimation may induce gill remodeling (Sollid and Nilsson, 2006), cardiac remodeling (Gamperl et al., 2001), increased hemoglobin O2 affinity (Wells et al., 1989), and increased tissue capillarity (Egginton, 2011). Other environmental conditions, such as temperature and pollution, can modulate hypoxic tolerance and performance (Mauduit et al., 2016; Mauduit et al., 2019; Adams et al., 2022). As modeling fish distributions and responses are increasingly important, the use of tolerance traits in combination with performance traits can only increase the quality of such models. Therefore, a better appreciation of species diversity in hypoxic tolerance and a deeper knowledge of hypoxia performance (i.e., aerobic scope curve) will advance our understanding of the biological diversity of physiological responses to hypoxia and how environmental factors alter the responses. Unfortunately, a high-throughput protocol that screens the almost 33,000 fish species has the potential to trade off convenience with the comprehensive, time-consuming phenotyping protocols needed to generate the information required on hypoxic performance. We believe that there is a solution to this apparent dilemma.
We envision a two-tier, whole-organism phenotyping protocol that measures the hypoxic robustness of fishes (defined by a combination of hypoxia tolerance and hypoxic performance measurements) via quantification of the limiting oxygenation level curve. We propose screening most species of fish with a well-established hypoxic challenge test (HCT), which generates a tolerance trait for an extremely hypoxic condition that a fish might rarely experience. Information on tolerance would then be useful to select indicator species that are representative of a larger group of species or have special socioeconomic value (e.g., commercially harvested or keystone species in an ecosystem) for a test of a hypoxia performance curve (HPC). This provides representative and comprehensive information on a fish’s capacity to perform under less hypoxic, more ecologically relevant conditions.
An HCT represents a low-cost, high-throughput (testing 50–100 fish per trial), and rapid (~4 h for 50–100 fish) test for measuring hypoxia tolerance at LOE. Such high fish numbers allow for excellent and statistically robust characterization of individuals within a population. The simplest form of an HCT, potentially requiring the least amount of equipment, measures time-to-reach LOE at a fixed level of hypoxia. However, the information generated on hypoxia tolerance is less informative than knowing the ambient O2 level at LOE, which is reported by most O2 meters as either dissolved O2 content (DO, mg L-1) or percent air saturation (% air sat.). Thus, LOE during progressive hypoxic exposure would yield an incipient lethal O2 concentration (ILOC) or an incipient lethal O2 saturation (ILOS), respectively. Both ILOC and ILOS provide information on O2 availability but are dependent on water temperature and do not inform us about a key determinant for the diffusion of O2 from water into the lamellar blood, i.e., the partial pressure of O2 (PO2: mm Hg or kPa) in water which is independent of temperature (except for the effect on the water vapor pressure of atmospheric air). At LOE, the incipient lethal ambient PO2 (ILOP) is characterized and ILOP finds a better utility for comparisons across different temperatures. Our preference is to measure water PO2 during progressive hypoxia for an HCT (see Discussion for additional reasoning), although dissolved O2 content and air saturation are valid substitutes.
The HPC concept originates from Fry’s seminal work (Fry, 1947) and has already been incorporated into fisheries models (Neill et al., 1994). A requirement for an HPC is measurements of aerobic scope at different levels of hypoxia down to or close to Pcrit. This is possible by measuring the of a large group of fish, or individual fish. Both have advantages. Group fish measurements, as pioneered by Claireaux and Lagardère (1999), were the first to experimentally use the HPC concept by following of a large group (N = 64 and 80) of European sea bass (Dicentrarchus labrax, Linnaeus 1758) in a single, large, sealed aquarium, which was quite a technical and engineering feat of “mass respirometry.” A group-based HPC was generated by triggering the spontaneous activity of the group of fish en masse while progressively reducing the DO in the water (it is unwise to let ambient PO2 decrease through the fish’s collective because CO2 and possibly NH3 accumulate in the aquarium), assuming that this activity periodically generated a peak MO2 for the group. Since the measures of peak cannot theoretically exceed , it is then possible to fit an upper bounding curve for these peak data to describe the HPC. The limitations of this method are that there is no confidence limit around the group-based HPC and while the peak is known, the aerobic scope is not, unless separate experiments were performed to measure individual SMR (a surrogate using a group RMR measurement is possible, but further assumptions become necessary). A group-based HPC measured in this manner has the obvious benefit of high throughput and is better than relying on either hypoxia tolerance measurements for ecological applications, or repeatedly measuring SMR and individual peak in groups of fish exposed to different levels of hypoxia, which would be an incredibly time-consuming test to remove some of the assumptions of a group-based HPC.
Fortunately, a far less time-consuming, individual-based HPC has recently been validated (Zhang et al., 2022). Using static respirometry chambers for individual rainbow trout (Oncorhynchus mykiss, Walbaum 1792), each fish is measured in their own chamber where they were physically agitated inside their respirometer to directly measure their peak (; the highest attainable measured under all hypoxic conditions). By repeating this process while ambient PO2 was reduced in a controlled fashion, a reliable individual-based HPC was generated (see details in Methods below). Intermittent static respirometry also allows a reliable measurement of SMR and therefore a reliable calculation of individual values of AAS as a function of ambient PO2, to which an HPC can be statistically modeled with confidence limits. Furthermore, such a method allows normalization of individual values as a percentage AAS (%AAS, Chabot and Claireaux, 2008) from which a new comparative metric can be interpolated, the minimum PO2 to sustain 50% AAS (PAAS-50, Zhang et al., 2022; see Discussion). While measuring the individual-based HPC is equipment-intensive, ecologically relevant information becomes available for a range of ambient PO2 in an experiment that lasts no more than 2 days.
Thus, two-tier testing of hypoxic robustness includes the comprehension of the biological endpoint (e.g., LOE) and how hypoxic water constrains the aerobic capacity of individual fish. Such characterizations can be readily extended to explore individual variability and families (possibly extending to consider their genetic basis) as well as following acclimation (acclimatization) to a variety of abiotic and biotic factors that ultimately enable better modeling of fish distribution. We now describe the methodologies that we prescribe for this two-tier testing of hypoxic robustness and provide references on where to find the more detailed methodologies.
Testing conditions will always use water quality conditions that match the acclimation/acclimatization conditions of the fish population being considered, that is, unless there is an a priori reason to test the fish’s acute response to a new water quality condition. We use ambient PO2 as the desired metric for measuring hypoxia in this article and refer to other metrics if they were used in a cited study.
Careful control of the O2 content of the water in which the fish are tested is required both to perform an HCT and to generate an HPC. The static aquarium used for the HCT has no specific design requirements beyond ease of use and a reliable means of decreasing the ambient water PO2 in a controlled fashion (e.g., semiautomatic: a controller and solenoid valve, Oxy-REG; Loligo Systems, Tjele, Denmark, or manual options are also valid). Thus, HCT is eminently suitable for the field as well as laboratory studies. The aquarium volume is determined primarily by the number of fish being tested in a single trial (which may differ depending on local fish welfare requirements, but up to 100 fish have been tested). Nitrogen gas (N2) is delivered to fine-pore aerators strategically placed on the bottom of the aquarium for efficient deoxygenation. Compressed N2 cylinders typically supply the gas, but an N2 generator may be more useful in field situations and whenever a very large aquarium is being used. Importantly, submersible pumps are necessary to prevent heterogeneity of water PO2. O2 meters continuously monitor PO2 during the HCT, and they are strategically placed in the center and (or) corners of the aquarium. If batches of fish are being tested, aquarium water must be completely changed to prevent excessive and potentially toxic CO2 buildup. When testing air-breathing species, a transparent lid should be placed on the top of the tank and in contact with the water surface to prevent access to the O2 in the air.
The more sophisticated aquarium (a respirometer) used for the HPC test can be purchased or manufactured in-house. It needs to be water-tight (with numerous ports for water flow) and submersible. The respirometer can be operated either with a continuously flow-through of water or with an intermittent flow-through of water (our case studies below use the latter protocol). In either case, a vertical O2-stripping column is in place upstream of the main water inflow port. This stripping column introduces water at its top and N2 gas at its bottom so that water PO2 is reduced. The hypoxic water is then homogenized (using submersible pumps) in the outer water bath prior to servicing all respirometers via controlled water pumps. Water PO2 is regulated by adjusting the N2 flow rate relative to the water flow rate. An alternative field setting uses the fine-pore aerators to deliver a regulated N2 gas flow to the bottom of the water bath to strip water PO2, while strategically placed submersible pumps homogenize water PO2. The water PO2 in the bath can be monitored by an O2 meter. Furthermore, water homogeneity within the respirometer is ensured with a water circulation pump via a set of separate ports. The O2 meter is placed in this water circulation loop, providing a continuous recording of the PO2 within the respirometer to estimate . These O2 meters also monitor the deoxygenation process. Respirometers are submerged in a water bath which helps regulate water temperature during the hypoxic performance test. Up to 32, but more commonly four to eight individual respirometers, each with their own set of water flow controls and O2 probe, can be placed in the water bath.
Measurements of LOE date back many decades and have been performed on a wide range of fish species. In fact, in just the last 6 years, an HCT has been performed in at least 11 published studies using a range of test apparatuses (Joyce et al., 2016; Mauduit et al., 2016; Lund et al., 2017; Zhang et al., 2017; Zhang et al., 2018a; Mauduit et al., 2019; Zhang et al., 2019a; Polinski et al., 2021; Strowbridge et al., 2021; Adams et al., 2022). We recommend the following test protocol, which is based on procedures formalized by Claireaux et al. (2013) and expedites the high throughput reliably.
The initial phase of water deoxygenation is rapid (~1.5% min-1 over ∼40 min) to a level that is usually 25% higher than the estimated Pcrit value (Figure 1A). The ensuing slower rate of decrease (~0.15% min-1) is maintained until all fish reach LOE (Figure 1A). Each time a fish reaches its LOE, the ambient PO2 (or the time-to-LOE) is recorded as an index of an individual fish’s hypoxic tolerance (fish can be individually tagged to facilitate monitoring and follow-up). The fish are quickly recovered by rapidly transferring them from the experimental arena into a well-aerated aquarium at the same temperature. If researchers are interested in characterizing the responses of tissues and organs under hypoxic conditions, fish can be terminally sampled at or immediately after LOE following the 3Rs principle in animal welfare. The individuals with extreme hypoxic tolerance (e.g., top and bottom 10% of the population) can then be characterized by a hypoxic performance curve to gain more physiological insights. A greater description of the case study is available in Zhang et al. (2017). This study demonstrates a bimodal distribution of hypoxic tolerance traits with molecular underpinnings.
Figure 1 A demonstration of the results from a typical hypoxia challenge test (HCT) that quantifies the frequency distribution of the hypoxia tolerance trait in a population of fish. (A) The test reduces the ambient water oxygen (O2) level (% saturation (sat.) or kPa) in the testing arena at an initially fast and then slower rate (see details in the methods). The fast phase minimizes the possibility of a rapid acclimation response to hypoxia. The slow phase makes it easier to remove and identify the individual fish when they lose their upright equilibrium and provides for a more reliable resolution of the hypoxic tolerance trait, their incipient lethal oxygen saturation (ILOS). (B) The resultant frequency distribution of ILOS for a population of European sea bass (Dicentrarchus labrax) at 16.5°C, which revealed a bimodal distribution for hypoxia tolerance with no individual between 7.40% sat. and 8.25% sat. (1.5–1.7 kPa) (adapted from Zhang et al., 2017). Hence, this population of European sea bass (n = 96) had a near equal proportion of hypoxia tolerant individuals (HT; n = 42) and hypoxia-sensitive individuals (HS; n = 54). These two hypoxic tolerance traits reflect the important intraspecific variation. The insert (violin plot: dash line = median, dotted line = quantile) shows the modality (independent t-test) for ILOS between the HT and HS phenotypes.
The mass-respirometry system used for generating a group-based HPC needs a flow control pump, a sealable aquarium, and an O2 meter. Schematics of an ideal experimental apparatus are illustrated in Claireaux and Lagardère (1999). A group of European sea bass (Dicentrarchus labrax) (up to N = 80) is acclimated to the aquarium (natural photoperiod and temperature with filtered natural seawater, fed twice weekly on commercial dry pellets that is 2%–4% of their body mass) prior to the experiment. The activity , which includes spontaneous activity, is first measured in normoxia for a period of 3–4 days, and a resting is assigned to the lowest measurement, typically during the night. Deoxygenation is stepwise, pumping hypoxic water generated by a vertical O2-stripping column (described above) into the mass respirometer. At predetermined and strategic levels of hypoxia, the water supply is turned off to seal the respirometer and follow the decline of ambient PO2 inside the respirometer with an O2 measurement device (e.g., optical O2 probe). Fish can be agitated to elicit spontaneous activity (e.g., light stimulation; see Andersson et al., 2020) and increase (estimated by a sequential interval regression analysis; see Supplementary Material). Seven levels of hypoxia above Pcrit seem adequate to model a group-based HPC based on fitting an upper bounding curve (the original study eye-fitted an asymptotic model) for these data. Because the spontaneous activities are not controlled in the mass respirometry, is randomly distributed between and SMR at each PO2 level. A group-based HPC would take 8 h. Additional experimental groups of 16 fish could be used to populate the area under the HPC. A greater description of the case study is available in Claireaux and Lagardère (1999); the pioneer study illustrates the methodologies and modeling potentials of group-based HPC.
Multiplex, intermittent-flow respirometry systems that generate individual-based data are well established. In fact, in just the last 6 years, such measurements have been performed in at least 30 published studies using a range of test apparatuses to test anywhere for four to 32 individuals at a time. However, most previous measurements of exercise the fish to exhaustion outside of the respirometer and begin measurements within about 2 min once the fish is fully sealed within the respirometer. Deliberate and standardized air exposure is intended to delay the rapid initial fall in post-exhaustion (Scarabello et al., 1992; Zhang et al., 2018b) so that can be captured. Such assumptions are no longer necessary because a technique to measure with the fish already sealed within the respirometer has been validated (Zhang et al., 2020). Moreover, peaks in are more dynamic than previously thought, which requires careful off-line analysis of the decline in ambient PO2 to generate a reliable value (Zhang and Gilbert, 2017; Zhang et al., 2019b; see Supplementary Material) Details of an individual-based HPC are briefly described below and are based on Zhang et al. (2022), the first methodological study for individual-based HPC.
Each respirometer is immersed in the water bath and receives water from it, intermittently circulated by individual, computer-controlled (e.g., AquaResp v.3, Aquaresp.com, Denmark) flush pumps (e.g., EHEIM, Deizisau, Germany). An monitoring period (when the flushing pump is stopped) lasts 600 s. A flushing period and an equilibration period (durations adjusted to the size or species of the fish) precede each monitoring period, a fish can be maintained in the flushing mode if measurements are not needed. Following the decline in ambient PO2 during a monitoring period allows off-line analysis of . The optical O2 probe (e.g., optodes from various commercial suppliers provide PO2 data at a frequency of about 1 Hz) located in the recirculation loop (with a dedicated external pump) of each respirometer continuously records the O2 partial pressure (PO2, mm Hg) of ambient water inside the respirometer. O2 probes are pre-calibrated to 0 mm Hg (0 kPa, water saturated with sodium sulfite and bubbled with nitrogen gas) and fully aerated water (157 mm Hg = 20.9 kPa). Background of an empty respirometer must be measured too. It is applied to fish measurements to adjust for beyond that of the fish.
Peak is generated during a monitoring period by individually agitating each fish inside the respirometer using a chasing device, a small in-house modification to a standard respirometer (a piece of soft plastic attached to a metal stem inserted through a sealed port at the top of the respirometer; Zhang et al., 2020). By rapidly turning the chasing device for 5 min, the fish performs C-start turns until it becomes refractory to further agitation (fatigues), after which the fish is left undisturbed for the remaining 5 min of the measurement period, to capture the initial portion of the excess postexercise O2 consumption (EPOC). Off-line analysis assigned to the highest of the values recorded during the chase and the initial phase of EPOC (the duration of the chase and the initial measuring period needed for EPOC may vary with the species). Typically, peak is reliably captured (sometimes repeatedly) during the 5-min chase and occasionally early in the 5-min recovery period, when is observed to rapidly decrease. After this 10-min monitoring period, the respirometer flush pumps are turned on to introduce hypoxic water into the respirometer.
For an HPC testing protocol, determinations are made first in normoxia and then repeated on the same fish at strategic, predetermined levels of hypoxia as the water bath and the respirometers are progressively deoxygenated in a controlled fashion as described above. is remeasured during progressive hypoxia by again sealing the respirometer at the required PO2 level and re-agitating the fish in the same manner. Typically, each level of hypoxia takes 20 min to reach except for the final one which may require a slightly longer degassing period (~30 min). Thus, each 5-min period of chasing and initial recovery (PO2 trace used for calculating ) is followed by a further 20 min as a minimum recovery period (might vary with species) during which is followed online. Validation tests with individual rainbow trout (Oncorhynchus mykiss) show that five ambient O2 levels can model (see Supplementary Material. For asymptotic model) an HPC as reliably as seven levels (four levels of ambient O2 are less reliable though) (Zhang et al., 2022). The initial determination under a normoxic condition is assigned MO2max . At the end of the trial, the fish are removed from their respirometers and fully recovered in a well-aerated recovery bath. This protocol took 2.5 h to generate the data for individual HPCs for eight fish.
Also, individual variability of can be normalized by expressing an individual’s as a percentage of its measured at or extrapolated to normoxia (i.e. ). Thus, individual values can then be expressed as a percentage of an individual’s AAS (%AAS = ( −SMR)•(MO2max – SMR)-1•100%) and remodeled as a function of ambient water PO2 to generate a normalized HPC (see below for SMR protocol). This curve can be interpolated to determine the minimum PO2 level that can support different levels of AAS (e.g., PAAS-75 = 75% AAS, PAAS-50 = 50% AAS, PAAS-25 = 25% AAS).
Individual SMR can be determined with a well-established protocol with fish being left undisturbed in a normoxic respirometer for up to 2 diurnal cycles while continuously measuring routine . SMR is estimated from these data from sequential interval regression analysis (see Supplementary Mateial) by applying a 20th quantile algorithm (see Chabot et al., 2016 and Chabot et al., 2021 for a more detailed SMR method). This SMR determination could precede the HPC protocol, resulting in a 2-day protocol, or remeasuring the same individual in the same way after a full recovery period of say 10 days (Zhang et al., 2022). Knowing SMR allows for an estimate of Pcrit by extrapolating the normalized HPC to zero AAS, an approach that has been validated for rainbow trout (Zhang et al., 2022).
We synthesize three representative case studies that used an HCT (Zhang et al., 2017), a group HPC (Claireaux and Lagardère, 1999), and an individual HPC (Zhang et al., 2022). Because the case study of two-tier testing of hypoxic robustness does not exist to the best of our knowledge, our goal is that by stringing together the three case studies that used the different components of the two-tier testing we can inspire future studies to use the two-tier testing of hypoxic robustness. Thus, we highlight the merits of the two-tier testing by showcasing the discoveries of (1) bimodal distribution of the hypoxic tolerance traits with molecular underpinnings, (2) a curvilinear continuum of O2-limiting effect on aerobic scope, and (3) asymptotic modeling to quantify the biological safety margins based on the percentage of aerobic scope.
The recommended high-throughput HCT protocol not only reliably estimates the ambient O2 level at LOE for a species under a given set of test conditions but also has the power to resolve individual distributions for a population that is not necessarily normally distributed (of skewed) around a single mode. For example, when 96 European sea bass were tested simultaneously, their ILOS had a clear bimodal distribution with near equal distributions of hypoxia-tolerant (HT) and hypoxia-sensitive (HS) phenotypes within the same population (Zhang et al., 2017; Figure 1B). The average ILOS for the HT phenotype (6.5 ± 0.09% sat.; n = 42) was significantly 26% lower (unpaired t-test: t = 21.4, p < 0.0001) than the HS phenotype (8.8 ± 0.06% sat.; n = 54), with no fish having an ILOS between 7.40% sat. and 8.25% sat. (see the PO2 values in Figure 1B). Thus, a priori assumptions about normal distributions of hypoxia tolerance within a species or even a population are unwise.
This case study is particularly informative because blood samples were taken at multiple (4–5) hypoxic levels to measure plasma lactate. The two phenotypes (prescreened) had different plasma lactate accumulation rates as a function of ambient PO2, suggesting that an HCT screen can highlight a difference in underpinning physiological mechanisms. Specifically, the HT phenotype and HS phenotype accumulated the same amount of plasma lactate, ~15 mM, at ILOS (regarded by some as an upper lactate threshold; Jain et al., 1998). However, for the HT phenotype, this lactate level was reached ~1.5 h later in the HCT and at a deeper level of hypoxia (i.e., the time and PO2 differences for the phenotypes reaching ILOS). A slower accumulation of end products from substrate-level phosphorylation in hypoxia would have clear ecological benefits.
Group HPC describes that the of spontaneous activities of a group of fish (N = 64–80) declines as a function of ambient O2 level (Figure 2). The group HPC provides an approximation of under hypoxic conditions by placing an upper bounding hyperbolic curve. The HPC crosses the resting (; 87 mg O2 h-1 kg-1) at severe hypoxia and can extrapolate to (if necessary) the active ( ; 333 mg O2 h-1 kg-1) in normoxia (an approximation of ). Group values provide a single approximation of HPC for a population.
Figure 2 A hypoxia performance curve (HPC) for a group of fish which models a constraint on the oxygen uptake () of whole-organism activities () when the ambient oxygen (O2) level (oxygen concentration: mg L-1) is progressively reduced. In this example, a group of European sea bass (Dicentrarchus labrax) measured in the mass respirometry at 20°C (adapted from Claireaux and Lagardère, 1999), different values within the HPC are the results of spontaneous activity. The group-based HPC (group HPC) is mathematical modeling following an assumption that HPC encompasses all the (). Group HPC is an estimate of the responses of a population of fish to hypoxic water; hence, the model lacks a 95% CI. The in normoxia is referred to as active () when the full aerobic scope can accommodate the highest level of activities. Because multiple fish in the mass respirometry do not reach the standard metabolic rate at the same time and fish tend to interact when swimming in a school, the baseline is termed as resting ().
Inspired by group HPC, individual HPC measures the decline of of an individual fish as a function of ambient O2 level, a more statistically comparable characterization of the constraints on aerobic capacity by hypoxic water (Figures 3A, B). As a group mean and bounded by 95% CI, HPC is a hyperbolic shape and best described (R2 = 0.936 and AIC = 378) by an asymptotic equation (see Supplementary Material) which is similar to group HPC. The individual HPC protocol can then capture the individual variations of HPC for eight rainbow trout. The interpolation of an HPC to a PO2 level (using an individual HPC model) that supports 50% AAS shows the individual variations of PAAS-50 (dots in the violin plot in Figure 3B). Although PAAS-50 is derived from individual-based HPC, the individual-based HPC can be pooled to generate a population HPC, with a range of predictive power (i.e., 95% C.I.). The interpolation can also expand to other PO2 levels that have biological significance, either individual- or group-based, that support the 75% and 25% of AAS to derive the metrics such as PAAS-25 and PAAS-75. Such metrics are only examples, and more research is needed to understand the potential landmark PO2 on HPC.
Figure 3 A hypoxia performance curve (HPC) characterizes the quantitative constraint on whole-organism peak () when the ambient oxygen (O2) level (partial pressure of oxygen: % sat. or kPa) is progressively reduced (adapted from Zhang et al., 2022). (A) In this example for a group of eight individually tested rainbow trout (Oncorhynchus mykiss) at 11°C, their individual hypoxia performance curves are pooled as mean (and SEM), where data are plotted at five strategically selected ambient O2 levels that bracket a 50% reduction and are above the critical oxygen tension (Pcrit), when absolute aerobic scope (AAS) is zero. These data points are then reliably modeled (a hyperbolic curve in this case: R2 = 0.936, AIC = 378.0) to generate the HPC (solid blue line) and its 95% CI (blue shade). (B) These () data can be normalized to an absolute aerobic scope (AAS) of each individual and reliably modeled (a hyperbolic curve in this case: R2 = 0.968, AIC = 134.6) to generate the HPC (solid blue line). Extrapolation of this model curve should approximate Pcrit and the normoxic maximum , both of which can be tested independently for verification purposes. Most important is that this curve, which accounts for individual variation in (), can be interrogated through interpolations. For example, the minimum oxygen tension to support a portion of AAS (e.g., 75%, 50%, and 25%) can be quantified as standardized comparative metrics. We recommend the PAAS-50 (minimum PO2 that supports 50% of AAS) which are shown as individual data in the insert (violin plot: dash line = median, dotted line = quantile).
Despite the ever-expanding and ever-evolving knowledge of hypoxia tolerance and testing protocols, we strongly believe that LOE remains (five decades after its formal introduction; Holeton, 1973) a useful anchor point for hypoxia tolerance in fishes. Originally, hypoxia tolerance testing used 50% lethality of a population of fish exposed to constant severe hypoxia or a slowly decreasing ambient PO2, i.e., median lethal concentrations (LC50, Vargo and Sastry, 1977). The same protocol could be used to generate time-to-LOE (DiAngelo and Heath, 1987), although time-to-LOE is not necessarily comparable across studies because of the various PO2 levels of cross-species. Instead, a quantitative metric with comparative power should apply a common biological endpoint; measuring the ambient PO2 in a standardized HCT achieves exactly that (van Raaij et al., 1994; Claireaux et al., 2013).
An important operational consideration for interdisciplinary research is matching the sample size to the needs of others. Ecologists care about the frequency distribution of the traits within a species or a population. Thus, a single HCT testing 100 individuals more than meets this need because it can even reveal a bimodal distribution of hypoxia tolerance. Those interested in the genetic underpinnings of traits, such as genome-wide association studies (GWAS, an unbiased scanning), may need 1,000 individuals. Again, the HCT protocol can suffice this need with its high-throughput capability (see Strowbridge et al., 2021, where a strain difference in hypoxia tolerance was identified). In fact, since its formalization by Claireaux et al. (2013), the application of HCT has been far-reaching, testing the effects of toxicant exposure (Mauduit et al., 2016), viral infections (Lund et al., 2017), and warm temperature (Adams et al., 2022) and even making connections between whole-organism and cardiac hypoxia tolerance (Joyce et al., 2016) and linking thermal tolerance with hypoxic tolerance (Zhang et al., 2018a).
A group-based HPC can also operate to serve the needs of others by quantifying how hypoxic conditions constrain performance capabilities, and not just tolerance and resistance. However, while a group-based HPC offers reasonably rapid measurements on large numbers of fish, data on individual variability are lost to a group average which may be suitable for certain ecological questions. Comparisons become more challenging too without any confidence limits on the data (unless groups of fish are pooled to generate a mean and its variance). Group-based HPCs operate with two assumptions. The first assumption is that the group peak values measured simultaneously for 64–80 fish to generate the bounding HPC are a true reflection of the sum of the individual values at any given ambient PO2. However, it is unlikely that all individuals in the group expressed their peak at exactly the same given our new knowledge of the dynamic nature of (Zhang et al., 2019b). Therefore, a group HPC may somewhat underestimate the true performance capabilities under hypoxia. Future validation testing could test the extent of this concern. The group HPC also requires an assumption about oxyregulation (a fitted curve) or/and oxyconformation (a fitted straight line) even if the a priori relationship between and PO2 is more likely to be curvilinear (Farrell et al., 2021) given the multifaceted hypoxic coping mechanisms in fish. Not only can the assumptions required for and the shortcomings of a group-based HPC be obviated with an individual-based HPC with very little additional investment of time but provide additional and valuable comparative information can be generated by an individual HPC.
The individual-based HPC protocol described above may well be improved over time, but its principles provide for robust quantification of the constraint that hypoxia imposes on aerobic performance over a 2-day testing period, if SMR is also reliably determined. Most of all, values are accurately measured as a function of ambient PO2 and in individual fish, which allows confidence limits to be placed around a species-specific HPC for comparative purposes. Also, best-fit statistical modeling allows for regression analysis of oxyconformers. The HPC model can be extrapolated to Pcrit (and if necessary).
The crucial trade-off of the individual HPC is the limited number of fish that can be tested at one time. We show a validation example for eight fish, but there are respirometry systems that house and test 16–32 fish. Therefore, the needs of the ecologist are within reach given that each individual-HPC test lasts just 2 days. Still, individual-based HPC is not high-throughput like the HCT, which means results from an HCT should guide whether or not it is worth performing an individual HPC for a given fish species.
The individual-based HPC does benefit by only needing to measure at five levels of ambient PO2 from the same individual. Measuring individual at different levels of ambient PO2 with longer periods of recovery time would protract the testing time, something that could be obviated by testing new groups of fish at different levels of ambient PO2 (but then losing important information on individual variation). However, perhaps the greatest benefit of the proposed individual-based HPC is the possibility to normalize the of any individual to its AAS in normoxia (Figure 3). Hence, we propose that %AAS should be included along with AAS ( – SMR) and factorial aerobic scope (FAS = /SMR) as a powerful comparative metric. While AAS characterizes the amount of O2 available for aerobic activity above SMR and FAS better illustrates which ecological activity might be supported (Farrell, 2016), FAS does not have the comparative potential offered by %AAS (both the numerator and denominator used to determine FAS could vary within individuals and across species).
Knowing the PO2 of the ambient water will allow the calculation of biological safety factors when considering the partial O2 gradient of the O2 cascade system, which we recommend as a new comparative metric for hypoxic performance—PAAS-50. We envision that interpolation of PAAS-50 from an individual-based HPC is used as a powerful comparative metric in much the same way the P50 for the O2-hemoglobin curve (when hemoglobin is 50% saturated with O2) has been used for decades. As it turns out, European sea bass and rainbow trout need around 50% of AAS to fully accommodate the peak in during digestion (Zambonino-Infante et al., 2017; Adams et al., 2022). If hypoxia constrained AAS below 50% (i.e., an ambient PO2 below PAAS-50), either appetite would have to be suppressed to lower the or the duration of digestion extended to accommodate a reduced (Adams et al., 2022). Thus, PAAS-50 may prove to be a useful metric to ascertain any hypoxic constraint or digestion and hence growth. While sophisticated equipment ensures the highest precision, it is still possible to perform field studies with equipment of a lower cost and some sacrifice of precision. Such an approach would be warranted to examine species diversity for hypoxic performance with the caveat that type II statistical errors could be introduced when species with a very similar PAAS-50 were compared.
Other %AAS levels might be used to decide on other ambient PO2 thresholds for hypoxic performance because different essential activities require different levels of aerobic scope and species-specific variations do exist. For example, PAAS-75 and PAAS-25 might act as valuable comparative biological safety margins (Figure 3). At PAAS-75, peak performance capabilities would be modestly limited perhaps stunting growth or delaying development, but perhaps not hindering digestion or appetite. Conversely, PAAS-25 would reflect bare sustenance of activities, perhaps not even digestion but modest locomotion, and could be used to define extreme hypoxia in an ecological context and supplement Pcrit.
Fish biologists can gain more valuable insights by advancing from simply measuring what is convenient to measuring what is most informative for making predictions and providing explanations. We believe that two-tier testing of a fish’s hypoxic robustness goes a long way to achieving such goals. The two-tier testing of hypoxic robustness recognizes the technical merits and limitations of the two hypoxia testing approaches that are currently available: the broader application of hypoxic tolerance testing and the selected application of hypoxic performance testing. Hence, the two-tier testing of hypoxic robustness covers both the breadth and depth of the hypoxia coping responses among fishes, as well as potentially uncovering how hypoxia tolerance and hypoxic performance might covary, providing ecological relevance for the types of questions that conservation policymakers need to answer. We envision information on hypoxia tolerance (i.e., LOE) for a large number of fish species and fewer selected species for hypoxic performance (i.e., PAAS-50). These two hypoxic indices could then be used, for example, modeling fish worldwide distributions against a backdrop of relatively rapidly changing and oscillating water conditions. Therefore, the two-tier testing of hypoxic robustness is suitable to address an imperative challenge for those interested in conserving and managing the planet’s fish resources in the current era of global climate change.
YZ and APF conceived the manuscript. YZ wrote the manuscript and synthesized the data. APF helped write the manuscript and provided constructive feedback. Two authors have read and agreed to the published version of the manuscript.
APF holds a Canada Research Chair Tier I and held NSERC Canada research grants. YZ holds an NSERC postdoctoral fellowship.
The authors appreciate the numerous insightful discussions with Guy Claireaux and Denis Chabot over the years. YZ particularly appreciate the stellar mentorships from Guy Claireaux and Denis Chabot.
The authors declare that the research was conducted in the absence of any commercial or financial relationships that could be construed as a potential conflict of interest.
All claims expressed in this article are solely those of the authors and do not necessarily represent those of their affiliated organizations, or those of the publisher, the editors and the reviewers. Any product that may be evaluated in this article, or claim that may be made by its manufacturer, is not guaranteed or endorsed by the publisher.
The Supplementary Material for this article can be found online at: https://www.frontiersin.org/articles/10.3389/fmars.2022.939239/full#supplementary-material
Adams O. A., Zhang Y., Gilbert M. H., Lawrence C. S., Snow M., Farrell A. P. (2022). An unusually high upper thermal acclimation potential for rainbow trout. Conserv. Physiol. 10 (1), coab101. doi: 10.1093/conphys/coab101
Andersson M. L., Sundberg F., Eklöv P. (2020). Chasing away accurate results: Exhaustive chase protocols underestimate maximum metabolic rate estimates in European perch perca fluviatilis. J. Fish Biol. 97 (6), 1644–1650. doi: 10.1111/jfb.14519
Chabot D., Claireaux G. (2008). Environmental hypoxia as a metabolic constraint on fish: The case of Atlantic cod, Gadus morhua. Mar. pollut. Bull. 57 (6), 287–294. doi: 10.1016/j.marpolbul.2008.04.001
Chabot D., Steffensen J. F., Farrell A. P. (2016). The determination of standard metabolic rate in fishes. J. Fish Biol. 88 (1), 81–121. doi: 10.1111/jfb.12845
Chabot D., Zhang Y., Farrell A. P. (2021). Valid oxygen uptake measurements: Using high r2 values with good intentions can bias upward the determination of standard metabolic rate. J. Fish Biol. 98 (5), 1206–1216. doi: 10.1111/jfb.14650
Claireaux G., Chabot D. (2016). Responses by fishes to environmental hypoxia: Integration through fry’s concept of aerobic metabolic scope. J. Fish Biol. 88 (1), 232–251. doi: 10.1111/jfb.12833
Claireaux G., Lagardère J.-P. (1999). Influence of temperature, oxygen and salinity on the metabolism of the European sea bass. J. Sea Res. 42 (2), 157–168. doi: 10.1016/S1385-1101(99)00019-2
Claireaux G., Théron M., Prineau M., Dussauze M., Merlin F.-X., Le Floch S. (2013). Effects of oil exposure and dispersant use upon environmental adaptation performance and fitness in the European sea bass, Dicentrarchus labrax. Aquat. Toxicol. 130–131, 160–170. doi: 10.1016/j.aquatox.2013.01.004
Dhillon R. S., Yao L., Matey V., Chen B. J., Zhang A. J., Cao Z. D., et al(2013). Interspecific differences in hypoxia-induced gill remodeling in carp. Physiological and Biochemical Zoology, 86 (6), 727ߝ739. doi: 10.1086/673180
DiAngelo C. R., Heath A. G. (1987). Comparison of in vivo energy metabolism in the brain of rainbow trout, salmo gairdneri and bullhead catfish, ictalurus nebulosus during anoxia. Comp. Biochem. Physiol. Part B: Comp. Biochem. 88 (1), 297–303. doi: 10.1016/0305-0491(87)90118-0
Egginton S. (2011). Physiological factors influencing capillary growth. Acta Physiol. 202 (3), 225–239. doi: 10.1111/j.1748-1716.2010.02194.x
Farrell A. P. (2007). Tribute to p. l. Lutz: A message from the heart – why hypoxic bradycardia in fishes? J. Exp. Biol. 210 (10), 1715–1725. doi: 10.1242/jeb.02781
Farrell A. P. (2016). Pragmatic perspective on aerobic scope: Peaking, plummeting, pejus and apportioning. J. Fish Biol. 88 (1), 322–343. doi: 10.1111/jfb.12789
Farrell A. P., Mueller C. A., Seymour R. S. (2021). Coming up for air. J. Exp. Biol. 224 (17). doi: 10.1242/jeb.243101
Farrell A. P., Richards J. G. (2009). “Chapter 11 defining hypoxia: An integrative synthesis of the responses of fish to hypoxia,” in Fish physiology, vol. 27 . Eds. Richards J. G., Farrell A. P., Brauner &C.J. (Academic Press), 487–503. doi: 10.1016/S1546-5098(08)00011-3
Farrell A. P., Sobin S. S., Randall D. J., Crosby S. (1980). Intralamellar blood flow patterns in fish gills. Am. J. Physiol.-Regul. Integr. Comp. Physiol. 239 (5), R428–R436. doi: 10.1152/ajpregu.1980.239.5.R428
Gallaugher P., Axelsson M., Farrell A. P. (1992). Swimming performance and haematological variables in splenectomized rainbow trout, Oncorhynchus mykiss. J. Exp. Biol. 171 (1), 301–314. doi: 10.1242/jeb.171.1.301
Gamperl A. K., Todgham A. E., Parkhouse W. S., Dill R., Farrell A. P. (2001). Recovery of trout myocardial function following anoxia: Preconditioning in a non-mammalian model. Am. J. Physiol.-Regul. Integr. Comp. Physiol. 281 (6), R1755–R1763. doi: 10.1152/ajpregu.2001.281.6.R1755
Hochachka P. W., Buck L. T., Doll C. J., Land S. C. (1996). Unifying theory of hypoxia tolerance: Molecular/metabolic defense and rescue mechanisms for surviving oxygen lack. Proc. Natl. Acad. Sci. United States America 93 (18), 9493–9498. doi: 10.1073/pnas.93.18.9493
Holeton G. F. (1973). Respiration of Arctic char (Salvelinus alpinus) from a high Arctic lake. J. Fish. Res. Board Canada 30 (6), 717–723. doi: 10.1139/f73-128
Holeton G. F., Randall D. J. (1967). The effect of hypoxia upon the partial pressure of gases in the blood and water afferent and efferent to the gills of rainbow trout. J. Exp. Biol. 46 (2), 317–327. doi: 10.1242/jeb.46.2.317
Jackson D. C., Ultsch G. R. (2010). Physiology of hibernation under the ice by turtles and frogs. J. Exp. Zool. Part A Ecol. Genet. Physiol. 313 (6), 311–327. doi: 10.1002/jez.603
Jain K. E., Birtwell I. K., Farrell A. P. (1998). Repeat swimming performance of mature sockeye salmon following a brief recovery period: A proposed measure of fish health and water quality. Can. J. Zool. 76 (8), 1488–1496. doi: 10.1139/z98-079
Joyce W., Ozolina K., Mauduit F., Ollivier H., Claireaux G., Shiels H. A. (2016). Individual variation in whole-animal hypoxia tolerance is associated with cardiac hypoxia tolerance in a marine teleost. Biol. Lett. 12 (1), 20150708. doi: 10.1098/rsbl.2015.0708
Lund M., Dahle M. K., Timmerhaus G., Alarcon M., Powell M., Aspehaug V., et al. (2017). Hypoxia tolerance and responses to hypoxic stress during heart and skeletal muscle inflammation in Atlantic salmon (Salmo salar). PloS One 12 (7), e0181109. doi: 10.1371/journal.pone.0181109
Mandic M., Regan M. D. (2018). Can variation among hypoxic environments explain why different fish species use different hypoxic survival strategies? J. Exp. Biol. 221 (21), jeb161349. doi: 10.1242/jeb.161349
Mandic M., Todgham A. E., Richards J. G. (2009). Mechanisms and evolution of hypoxia tolerance in fish. Proc. R. Soc. B: Biol. Sci. 276 (1657), 735–744. doi: 10.1098/rspb.2008.1235
Mauduit F., Domenici P., Farrell A. P., Lacroix C., Le Floch S., Lemaire P., et al. (2016). Assessing chronic fish health: An application to a case of an acute exposure to chemically treated crude oil. Aquat. Toxicol. 178, 197–208. doi: 10.1016/j.aquatox.2016.07.019
Mauduit F., Farrell A. P., Domenici P., Lacroix C., Le Floch S., Lemaire P., et al. (2019). Assessing the long-term effect of exposure to dispersant-treated oil on fish health using hypoxia tolerance and temperature susceptibility as ecologically relevant biomarkers. Environ. Toxicol. Chem. 38 (1), 210–221. doi: 10.1002/etc.4271
Neill W. H., Miller J. M., van der Veer H. W., Winemiller K. O. (1994). Ecophysiology of marine fish recruitment: A conceptual framework for understanding interannual variability. Netherlands J. Sea Res. 32 (2), 135–152. doi: 10.1016/0077-7579(94)90037-X
Pickett G. D., Pawson M. G. (1995). Sea Bass: Biology, exploitation and conservation. Oceanog. Lit. Rev. 9 (42), 787–788. doi: 10.1002/aqc.3270050207
Polinski M. P., Zhang Y., Morrison P. R., Marty G. D., Brauner C. J., Farrell A. P., et al. (2021). Innate antiviral defense demonstrates high energetic efficiency in a bony fish. BMC Biol. 19 (1), 138. doi: 10.1186/s12915-021-01069-2
Randall D. J., Shelton G. (1963). The effects of changes in environmental gas concentrations on the breathing and heart rate of a teleost fish. Comp. Biochem. Physiol. 9 (3), 229–239. doi: 10.1016/0010-406X(63)90046-X
Sandblom E., Axelsson M. (2006). Adrenergic control of venous capacitance during moderate hypoxia in the rainbow trout (Oncorhynchus mykiss): Role of neural and circulating catecholamines. American journal of physiology. Regul. Integr. Comp. Physiol. 291 (3), R711–R718. doi: 10.1152/ajpregu.00893.2005
Scarabello M., Heigenhauser G. J., Wood C. M. (1992). Gas exchange, metabolite status and excess post-exercise oxygen consumption after repetitive bouts of exhaustive exercise in juvenile rainbow trout. J. Exp. Biol. 167 (1), 155–169. doi: 10.1242/jeb.167.1.155
Schmidtko S., Stramma L., Visbeck M. (2017). Decline in global oceanic oxygen content during the past five decades. Nature 542 (7641), 335–339. doi: 10.1038/nature21399
Shoubridge E. A., Hochachka P. W. (1980). Ethanol: Novel end product of vertebrate anaerobic metabolism. Science 209 (4453), 308–309. doi: 10.1126/science.7384807
Soldatov A. A. (1996). The effect of hypoxia on red blood cells of flounder: A morphologic and autoradiographic study. J. Fish Biol. 48 (3), 321–328. doi: 10.1111/j.1095-8649.1996.tb01430.x
Sollid J., Nilsson G. E. (2006). Plasticity of respiratory structures–adaptive remodeling of fish gills induced by ambient oxygen and temperature. Respir. Physiol. Neurobiol. 154 (1), 241–251. doi: 10.1016/j.resp.2006.02.006
Stecyk J. A. W. (2017). “5–cardiovascular responses to limiting oxygen levels,” in Fish physiology. Eds. Gamperl A. K., Gillis T. E., Farrell A. P., Brauner &C.J. (Academic Press) 36, 299–371. doi: 10.1016/bs.fp.2017.09.005
Stecyk J. A. W., Stensløkken K.-O., Farrell A. P., Nilsson G. E. (2004). Maintained cardiac pumping in anoxic crucian carp. Science 306 (5693), 77–77. doi: 10.1126/science.1100763
Strowbridge N., Northrup S. L., Earhart M. L., Blanchard T. S., Schulte P. M. (2021). Acute measures of upper thermal and hypoxia tolerance are not reliable predictors of mortality following environmental challenges in rainbow trout (Oncorhynchus mykiss). Conserv. Physiol. 9 (1), coab095. doi: 10.1093/conphys/coab095
Sundin L. I. (1995). Responses of the branchial circulation to hypoxia in the Atlantic cod, Gadus morhua. Am. J. Physiol. 268 (3 Pt 2), R771–R778. doi: 10.1152/ajpregu.1995.268.3.R771
van Raaij M. T. M., Bakker E., Nieveen M. C., Zirkzee H., van den Thillart G. E. E. J. M. (1994). Energy status and free fatty acid patterns in tissues of common carp (Cyprinus carpio, l.) and rainbow trout (Oncorhynchus mykiss, l.) during severe oxygen restriction. Comp. Biochem. Physiol. Part A: Physiol. 109 (3), 755–767. doi: 10.1016/0300-9629(94)90219-4
Vargo S. L., Sastry A. N. (1977). Acute temperature and low dissolved oxygen tolerances of brachyuran crab (Cancer irroratus) larvae. Mar. Biol. 40 (2), 165–171. doi: 10.1007/BF00396263
Vornanen M., Stecyk J. A. W., Nilsson G. E. (2009). “Chapter 9 the anoxia-tolerant crucian carp (Carassius carassius l.),” in Fish physiology. Eds. Richards J. G., Farrell A. P., Brauner &C.J. (Academic Press) 27, 397–441. doi: 10.1016/S1546-5098(08)00009-5
Wells R. M. G., Grigg G. C., Beard L. A., Summers G. (1989). Hypoxic responses in a fish from a stable environment: Blood oxygen transport in the Antarctic fish Pagothenia borchgrevinki. J. Exp. Biol. 141 (1), 97–111. doi: 10.1242/jeb.141.1.97
Zambonino-Infante J. L., Mazurais D., Dubuc A., Quéau P., Vanderplancke G., Servili A., et al. (2017). An early life hypoxia event has a long-term impact on protein digestion and growth in juvenile European sea bass. J. Exp. Biol. 220 (10), 1846–1851. doi: 10.1242/jeb.154922
Zhang Y., Claireaux G., Takle H., Jørgensen S. M., Farrell A. P. (2018b). A three-phase excess post-exercise oxygen consumption in Atlantic salmon Salmo salar and its response to exercise training. J. Fish Biol. 92 (5), 1385–1403. doi: 10.1111/jfb.13593
Zhang Y., Gilbert M. J. H. (2017). Comment on ‘Measurement and relevance of maximum metabolic rate in fishes by norin & clar).’. J. Fish Biol. 91 (2), 397–402. doi: 10.1111/jfb.13291
Zhang Y., Gilbert M. J. H., Farrell A. P. (2019b). Finding the peak of dynamic oxygen uptake during fatiguing exercise in fish. J. Exp. Biol. 222 (12). doi: 10.1242/jeb.196568
Zhang Y., Gilbert M. J. H., Farrell A. P. (2020). Measuring maximum oxygen uptake with an incremental swimming test and by chasing rainbow trout to exhaustion inside a respirometry chamber yields the same results. J. Fish Biol. 97 (1), 28–38. doi: 10.1111/jfb.14311
Zhang Y., Healy T. M., Vandersteen W., Schulte P. M., Farrell A. P. (2018a). A rainbow trout Oncorhynchus mykiss strain with higher aerobic scope in normoxia also has superior tolerance of hypoxia. J. Fish Biol. 92 (2), 487–503. doi: 10.1111/jfb.13530
Zhang Y., Mauduit F., Farrell A. P., Chabot D., Ollivier H., Rio-Cabello A., et al. (2017). Exposure of European sea bass (Dicentrarchus labrax) to chemically dispersed oil has a chronic residual effect on hypoxia tolerance but not aerobic scope. Aquat. Toxicol. (Amsterdam Netherlands) 191, 95–104. doi: 10.1016/j.aquatox.2017.07.020
Zhang Y., Montgomery D. W., White C. F., Richards J. G., Brauner C. J., Farrell A. P. (2022). Characterizing the hypoxic performance of a fish using a new metric: PAAS-50. J. Exp. Biol., jeb.244239. doi: 10.1242/jeb.244239
Zhang Y., Polinski M. P., Morrison P. R., Brauner C. J., Farrell A. P., Garver K. A. (2019a). High-load reovirus infections do not imply physiological impairment in salmon. Front. Physiol. doi: 10.3389/fphys.2019.00114
Keywords: aerobic scope, metabolic rate, exercise, maximum oxygen uptake, respirometry, high throughput, hypoxia performance curve
Citation: Zhang Y and Farrell AP (2022) Testing the hypoxia tolerance and hypoxic performance of fishes: A two-tier screening approach. Front. Mar. Sci. 9:939239. doi: 10.3389/fmars.2022.939239
Received: 08 May 2022; Accepted: 26 July 2022;
Published: 07 October 2022.
Edited by:
Menghong Hu, Shanghai Ocean University, ChinaReviewed by:
Amélie Crespel, University of Turku, FinlandCopyright © 2022 Zhang and Farrell. This is an open-access article distributed under the terms of the Creative Commons Attribution License (CC BY). The use, distribution or reproduction in other forums is permitted, provided the original author(s) and the copyright owner(s) are credited and that the original publication in this journal is cited, in accordance with accepted academic practice. No use, distribution or reproduction is permitted which does not comply with these terms.
*Correspondence: Yangfan Zhang, eWFuZ2Zhbl96aGFuZ0BmYXMuaGFydmFyZC5lZHU=
Disclaimer: All claims expressed in this article are solely those of the authors and do not necessarily represent those of their affiliated organizations, or those of the publisher, the editors and the reviewers. Any product that may be evaluated in this article or claim that may be made by its manufacturer is not guaranteed or endorsed by the publisher.
Research integrity at Frontiers
Learn more about the work of our research integrity team to safeguard the quality of each article we publish.