- 1Estonian Marine Institute, University of Tartu, Tallinn, Estonia
- 2Department of Marine Science, University of Otago, Dunedin, New Zealand
- 3Department of Functional and Evolutionary Ecology, University of Vienna, Vienna, Austria
- 4Coastal People Southern Skies Centre of Research Excellence, University of Otago, Dunedin, New Zealand
Through the fixation of large quantities of dissolved inorganic carbon (DIC), macroalgae facilitate the energetic foundation of highly productive coastal ecosystems. While the processes controlling photosynthesis and carbon fixation by macroalgae are well known, the fate of organic matter fixed by macroalgae is less well understood. This study quantified release rates of DOC by three ecologically significant Baltic macroalgae species: the perennial habitat forming Fucus vesiculosus and Furcellaria lumbricalis, and the seasonal fast-growing Ulva intestinalis, under both light and dark conditions. The released products were assessed using bacterial incubations whereby radiolabeled leucine was used to evaluate the uptake and lability of these products by marine heterotrophic bacteria. DOC was found to be released by both F. vesiculosus and U. intestinalis at rates of 0.27 mg C·h−1 under light and 0.13 mg C·h−1 per unit of dry mass under dark treatments, respectively, whereas F. lumbricalis DOC release was observed to be negligible under both light and dark. Our findings further validate previous hypotheses that factors such as photosynthetic activity are a primary driver behind DOC release and that DOC release is not an entirely passive process. Additionally, we reaffirm the need to relate a given species life characteristics and habitat in order to understand why DOC products are released. The consumption of macroalgae-derived DOC by heterotrophic bacteria reveals that released DOC is variable in its lability. After a period of 12 h and under maximum photosynthetic conditions, the release of DOC by F. vesiculosus and U. intestinalis achieved a peak rate of 219 µg C·L−1·day−1 and 214 µg C·L−1·day−1 for each gram of dry weight material, respectively, directly into the microbial loop via heterotrophic bacterial consumption. In contrast, F. lumbricalis’ low rate of DOC release and the subsequent low bacterial consumption indicate that habitats dominated by this species have a reduced importance in the transfer energy via the microbial loop. These findings have implications for how we view carbon transfer within coastal food webs and highlight how changes in species composition and coverage may dramatically affect coastal ecosystem productivity through the microbial loop.
Introduction
Macroalgae constitute the foundation of coastal ecosystems through the formation of a complex three-dimensional habitat that acts to fix large quantities of inorganic carbon. While the importance of carbon uptake is well established, we know comparatively little about its subsequent release or the role this plays within the broader ecology of coastal marine systems. From the latter half of the mid-20th century, the works of Pomeroy (1974) and Azam et al. (1983) demonstrated that a substantial proportion of algal photosynthetic products is released back into the water as dissolved organic carbon (DOC). This carbon source is crucial in supplying energy for microbial communities and reintegrating this carbon into higher-order trophic levels through the microbial loop.
Our current understanding of the underlying mechanisms that control for the release of DOC are incomplete. While DOC release is inherently related to macroalgae productivity as a function of photosynthetic activity and limited nutrient availability, the physiological processes that result in DOC release on a cellular level are not readily apparent. Enigmatically, DOC release would appear to have no distinct biological advantage in that it is an energetically wasteful process whereby there is no mechanism for algae to re-assimilate extracellular products. Two primary contending hypotheses attempt to reconcile this inconsistency. The stochiometric overflow hypothesis as described in Fogg (1983) asserts that DOC release is related to surplus photosynthate production under high photosynthetic conditions. Under normal circumstances, excess photosynthate may be synthesized into reserve energy stores or cell material. However, it may be more energetically efficient to release excess products for a short period rather than invest in the required structures and enzymes for storage or synthesis respectively (Hatcher et al., 1977; Fogg 1983; Thornton, 2014; Livanou et al., 2019). In contrast, the photosynthate diffusion hypothesis posits that DOC release is passive in nature and is related to low weight low-molecular-weight compounds diffusing through cell membranes during cellular growth or lysis (Bjørrisen, 1988; Møller et al., 2003; Saba et al., 2011; Agustí and Duarte, 2013). It should be noted, however, that these two hypotheses are not mutually exclusive, and that DOC release may in fact be a combination of both phenomena.
Irrespective of the underlying release mechanism, it is the consumption of DOC by other organisms that is perhaps most important in terms of understanding energy transfer and coastal ecology. As microorganisms constitute the dominant fraction of biomass in the marine environment, when active, their high metabolic rates dominate the flux of energy and biologically important elements in the ocean (Pomeroy et al, 2007). Duarte and Cebrian (1996) compiled data from the literature on the production pathways for a number of macrophytes including macroalgal communities. Their work determined that decomposition within the system, herbivore grazing pressure, and export outside the system are significant vectors for production, and also indicated that storage within sediment is negligible. However, only a small proportion of macroalgae (~10%) is directly consumed by higher-order organisms (Miller et al., 1971; Gerard, 1976; Newell et al., 1982). As such, a significant proportion of fixed carbon derived from macroalgae must first be acted upon by bacteria before it can be utilized by higher trophic levels (Duggins et al., 1989). Because estimates of macrophyte production and consumption typically ignore the release of DOC, the degree to which it contributes to marine food web function is unknown.
The Baltic Sea is a unique aquatic system in that it is a shallow, semi-enclosed brackish water sea. The species that reside there typically operate at their osmotic limits and represent a mixture of both marine and freshwater species (Snoeijs-Leijonmalm and Andrén, 2017). As a consequence, the Baltic Sea ecosystem displays a trend of low diversity and high biomass, and exhibits higher pelagic and benthic productivity relative to most oceanic marine systems (Kautsky et al., 2017; Snoeijs-Leijonmalm, 2017; (Snoeijs-Leijonmalm and Andrén, 2017). Additionally, the Baltic Sea is readily impacted by anthropogenic forces, particularly high nutrient loads due to its semi-enclosed nature and high residence time leading to eutrophication (Snoeijs-Leijonmalm, 2017). The effect eutrophication has on macroalgae is multifaceted but in the Baltic context primarily affects communities through increased water turbidity reducing water clarity and limiting photosynthesis (Snoeijs-Leijonmalm, 2017; Snoeijs-Leijonmalm and Andrén, 2017). For these reasons, when evaluating the function of Baltic Sea ecosystems, it is useful to examine species specific to the Baltic system.
This study investigated possible release mechanisms (passive cell leak hypothesis vs. stochiometric overflow) of DOC from three important Baltic Sea macroalgae species: the perennial habitat forming Fucus vesiculosus (Brown) and Furcellaria lumbricalis (Red), and the seasonal fast-growing Ulva intestinalis (Green), by quantifying DOC released by individuals in incubation chambers under simulated night and day conditions (light vs. dark). Additionally, we examined the consumption of released DOC by heterotrophic marine bacteria by quantifying bacterial biomass production in terms of carbon uptake/growth and reported as micrograms of carbon as a means to evaluate the potential energy transfer from microbes to higher-order trophic levels. As coastal ecosystems are increasingly viewed as vehicles for carbon transfer, we aim to better understand the fate of the substantial amounts of released hidden carbon that moves through these systems.
Materials and methods
Macroalgae collection and pre-treatment
The collection of macroalgal specimens for experimentation occurred during the summer vegetative season. Representative macrophytes were chosen on the basis of their dominance within the coastal system of the Northern Baltic Sea. Three species were selected for study. In particular, sites containing complex three-dimensional rock and boulder habitat consisting of shallow degrees of relief and moderate wave exposure typical of Northern Baltic temperate macroalgae dominated habitats were utilized. Individual macroalgae specimens were collected for incubation in each experiment by means of snorkeling and SCUBA. F. vesiculosus and U. intestinalis were collected via snorkeling from Kukumäe Bay (59°27’33.5”N 24°34’03.0”E) and Tallinn Bay (59°27’33.2”N 24°34’57.5”E) on the southern coast of the Gulf of Finland, Estonia respectively. F. lumbricalis was collected via SCUBA from Kõiguste Bay (58°21’39.3”N 22°59’25.2”E) on the southern coast of Saaremaa Island, Estonia (Figure 1). Ten individuals were collected for each species.
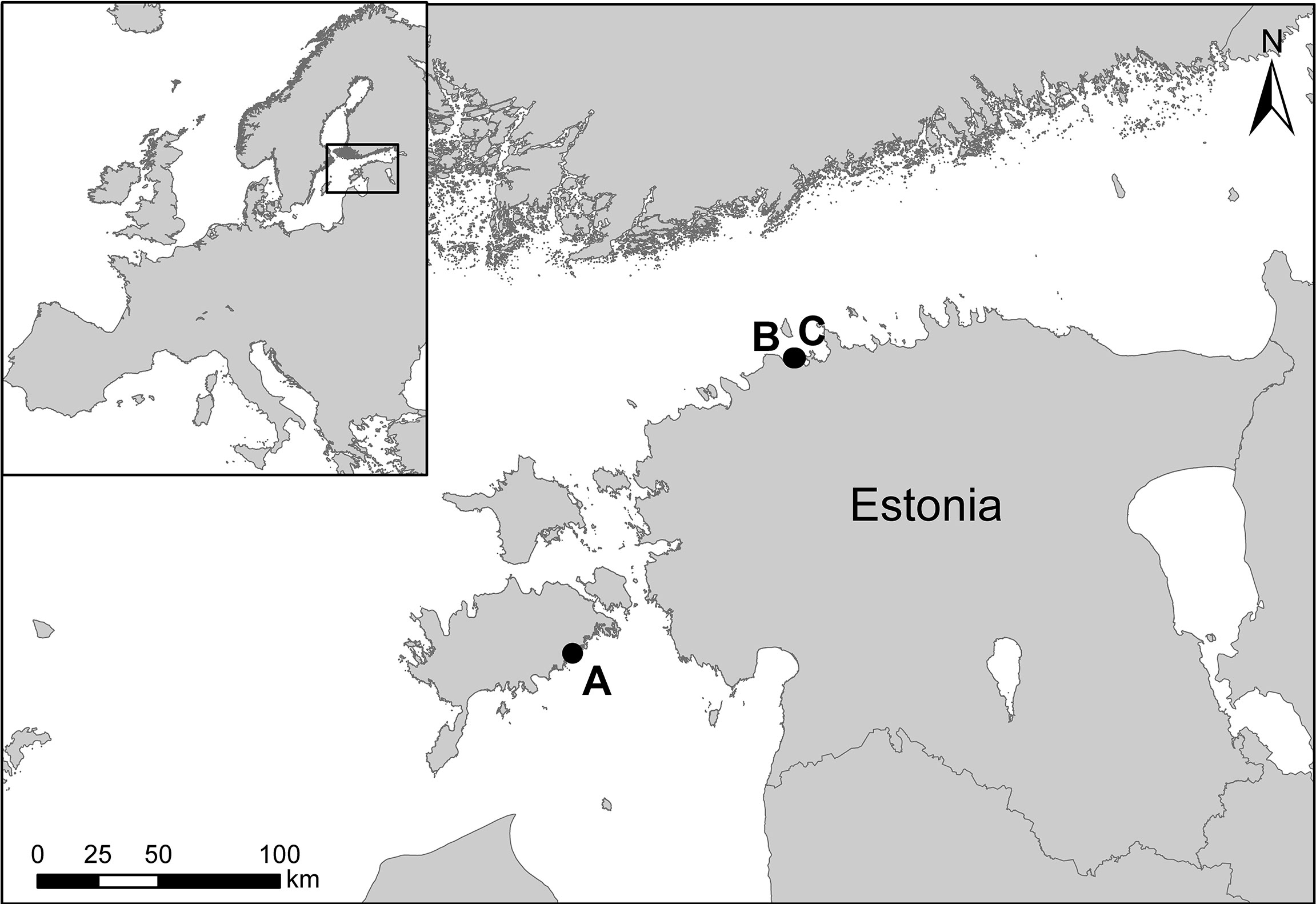
Figure 1 Map of the Estonian coastline. Sampled specimens were retrieved from (A) Kõiguste Bay, (B) Kukumäe Bay, and (C) Tallinn Bay.
Upon collection, individuals were stored in 20-L clear plastic bins containing site water with free-flowing air providing aeration and water motion. Additionally, site water was collected for use in the forthcoming experiment. The collected individuals were transported to the Estonian Marine Institute laboratory and stored in a climate-controlled room set to the ambient seawater temperature of the sample site for a period of 24 h in order for the macroalgae individuals to acclimatize. Prior to experimentation, individuals were gently cleaned in order to remove any epiphyte algae or animals. Experimentation consisted of two phases: (1) the measurement of DOC production and (2) the assimilation of DOC by heterotrophic marine bacteria.
Macroalgae DOC production
The release of macroalgae-derived DOC was quantified through the use of 4-L glass incubation chambers over a period of 12 h. In total, four experiments were conducted, one for each of the three selected species as well as one seawater control. Each experiment consisted of 10 biological replicates of distinct individuals in the case of F. vesiculosus and ~20 g of wet weight material for U. intestinalis and F. lumbricalis, which were incubated in separate chambers. Each experiment consisted of two treatments with five replicates under saturating light conditions (PAR measured using Li-Cor light meter) and five in total darkness for a total of 10 simultaneous incubations. The incubations were conducted in a temperature-controlled room reflective of ambient seawater temperature at the time of sample collection. Free-flowing bubblers supplied aeration and water motion to each incubation chamber.
The incubation medium consisted of 4 L of filtered seawater collected from the associated sample site. Filtered seawater was used as a means to remove the bulk of heterotrophic bacteria that may consume released DOC over the incubation period. This was achieved firstly by passing seawater through a 0.8-mm-size screen to remove zooplankton and any large detritus followed by filtering the water using a pump system with a 0.8-micron filter (nylon membrane, Whatman) to remove phytoplankton followed by a 0.22-micron filter (nylon membrane, Whatman) to remove the final bacterioplankton fraction. Water samples (50 ml) were taken every 0, 3, 6, 9, and 12 h from each of the incubation chambers using a sterile plastic lure lock tipped syringe fitted with 0.45-micron G/F sterile syringe filters (Minisart) with the sample being dispensed into individual 50-ml acid-washed glass bottles. Water samples were immediately frozen for analysis at a later time.
At the conclusion of the 12-h incubation period, 200 ml from each of the incubation chambers under the light treatment was re-filtered using the same pump and filter system described prior and dispensed into sterile glass sample bottles. This process was repeated for the dark treatment. The now “DOC enriched” seawater was retained for bacterial consumption experiments. Any algal material was weighed and dried to obtain both wet weight and dry weights. The concentration of DOC present in the seawater samples was quantified through high-temperature catalytic oxidation methodology as described by Sugimura and Suzuki (1988) using a total organic carbon analyser (Shimadzu Corp.) equipped with a platinum catalyst and reported as micrograms per liter.
Heterotrophic bacterial consumption of released DOC products
The consumption of macroalgae-derived DOC by heterotrophic bacteria was examined through the use microcosm experiments utilizing the retained seawater from the previous experiments and quantified using radiolabeled leucine. One hundred eighty microliters of the pooled macroalgae seawater from each replicate of both the light and dark treatments was separated into 10 separate acid-washed glass vessels (labeled A–E for light and F–J for dark) for a total of 10 microcosm incubations. Twenty milliliters of filtered seawater (0.45 μm) was used to inoculate the microcosm with ambient bacteria associated with the sample site seawater. The microcosms were buffed with 16.43 μl of NaNO3 (1.06 μmol L−1), 10.53 μl of KH2PO4 (0.077 μmol L−1), and 0.78 μl of NH4Cl (0.172 μmol L−1) to prevent nutrient limitation hindering bacterial growth. The microcosms were set on top of shaker tables in order to generate turbulent motion and mixing and were kept covered from any light as well as kept in a temperature-controlled room reflective of sample site temperature. The incubation was carried out for a period of 120 h. In order to evaluate total DOC consumption at the conclusion of the incubation period, DOC samples were taken for each of the microcosm chambers.
Heterotrophic bacterial biomass production was measured every 24 h (including initial) and quantified as the incorporation of [3H]-labeled leucine (Perkin–Elmer, specific activity = 169 Ci mmol−1) into bacterial cells for each microcosm chamber (Smith and Azam, 1992). Four 1.2-ml samples were taken from each microcosm and dispensed into separate 1.7-ml Eppendorf’s tubes. Three of the Eppendorf’s tubes were designated the technical replicates and the fourth was designated the blank (control). One hundred twenty microliters of trichloroacetic acid (TCA) 50% was dispensed to “kill off” the controls. Forty-eight microliters (40 nM of 1 μM solution) of radiolabeled leucine was dispensed into the technical replicates and controls. The technical replicates and controls were incubated for 90 min in the dark at 10°C. This temperature is reflective of ocean temperature at the time of sample collection. Post incubation, 120 μl of TCA 50% was dispensed to terminate growth in the technical replicates. The technical replicates and controls were centrifuged at 12,000 g before aspiration of the Eppendorf’s fluid was used to drain the technical replicates and controls of liquid, leaving the precipitated protein. A final solution of 5% TCA was dispensed into the technical replicates and controls, with the centrifugation and aspiration processes then being repeated. The technical replicates and controls were then immersed in 1 ml of liquid scintillation cocktail (Ultima Gold AB, Perkin-Elmer) in preparation for radio assay. The technical replicates and controls were allowed to sit for a period of several days as a means to maximize the dispersion of radioactivity into the scintillation cocktail before a count was performed. Heterotrophic bacterial uptake of [3H]-leucine was radio assayed and measured as decompositions per minute (DPM) in a Tri-Carb® Liquid Scintillation Counters scintillation counter (Perkin-Elmer) with quenching correction. Quenching was corrected by the external standard ratio method before the technical replicates and their associated controls were put to count. A conversion factor (DPM per microliter of leucine solution) was used to convert the reported DPM to moles of leucine. Bacterial biomass production was measured as pmol (leucine) L−1 h−1 and converted to μg (carbon) L−1 day-1.
Statistics
Statistical analyses were carried out using JMP software Version 14.1 (14.0) and R (Version 3.6.0). Changes in species (F. vesiculosus, U. intestinalis, and F. lumbricalis) DOC concentration and BBP rates were assessed separately using repeated measures analysis of variance (ANOVA) for the factor of treatment (light/dark) with the repeated measure being time. In both instances, the assumed sphericity was tested using Mauchly’s sphericity test of which both sets failed to meet the assumption. As such, the Greenhouse–Geisser correction was applied to correct for the departure from sphericity in the data (DOC: Table 1; BBP: Table 2). Post-hoc testing was conducted using pairwise t-tests with Bonferroni correction. Changes in DOC concentration produced under light and dark conditions by each macroalgal species after the 120-h incubation period were tested using Student’s t-test analysis with Bonferroni correction. The significance level for all statistical tests was set at 5% (a = 0.05).
Results
Mean macroalgae DOC production rates varied between both macroalgae species and the treatment they were exposed to (Figure 2). F. vesiculosus incubated under the light treatment was the most productive in terms of DOC release with a significantly different DOC concentration detected after a period of 9 h when compared to the starting concentration. In total, F. vesiculosus released a mean value of 3.26 mg of carbon per gram of dry weight material in the 12-h incubation period, achieving a maximum average production rate of 0.27 mg C·g DW−1·h−1 (Figure 2). In contrast, F. vesiculosus incubated under the dark treatment displayed no significant change in DOC concentration throughout the time course (Figure 2). The second most productive species in terms of DOC release was U. intestinalis, which displayed a significant increase in DOC concentration after a period of 3 h for the light treatment as well as the dark treatment after a period of 6 h, relative to the starting concentration (Figure 2). Overall, U. intestinalis released a mean value of 2.64 and 1.01 mg of carbon per gram of dry weight material for the light and dark treatments over the course of the 12-h incubation period, respectively (Figure 2). U. intestinalis achieved a maximum DOC release rate of 0.22 mg C·g DW−1·h−1 for light and 0.13 mg C·g DW−1·h−1 for dark (Figure 2). In terms of F. lumbricalis, no change in DOC concentration was observed over the course of the 12-h incubation for the light treatment; however, a small but statistically significant decrease was observed relative to the starting concentration for the dark treatment after a 9-h period with a decrease of 0.19 mg of carbon per gram of dry weight occurring at the conclusion of the incubation (Figure 2). DOC production rates appear relatively consistent between both F. vesiculosus and U. intestinalis, implying that DOC production occurs at a consistent rate without large pulses or drop offs (Figure 2).
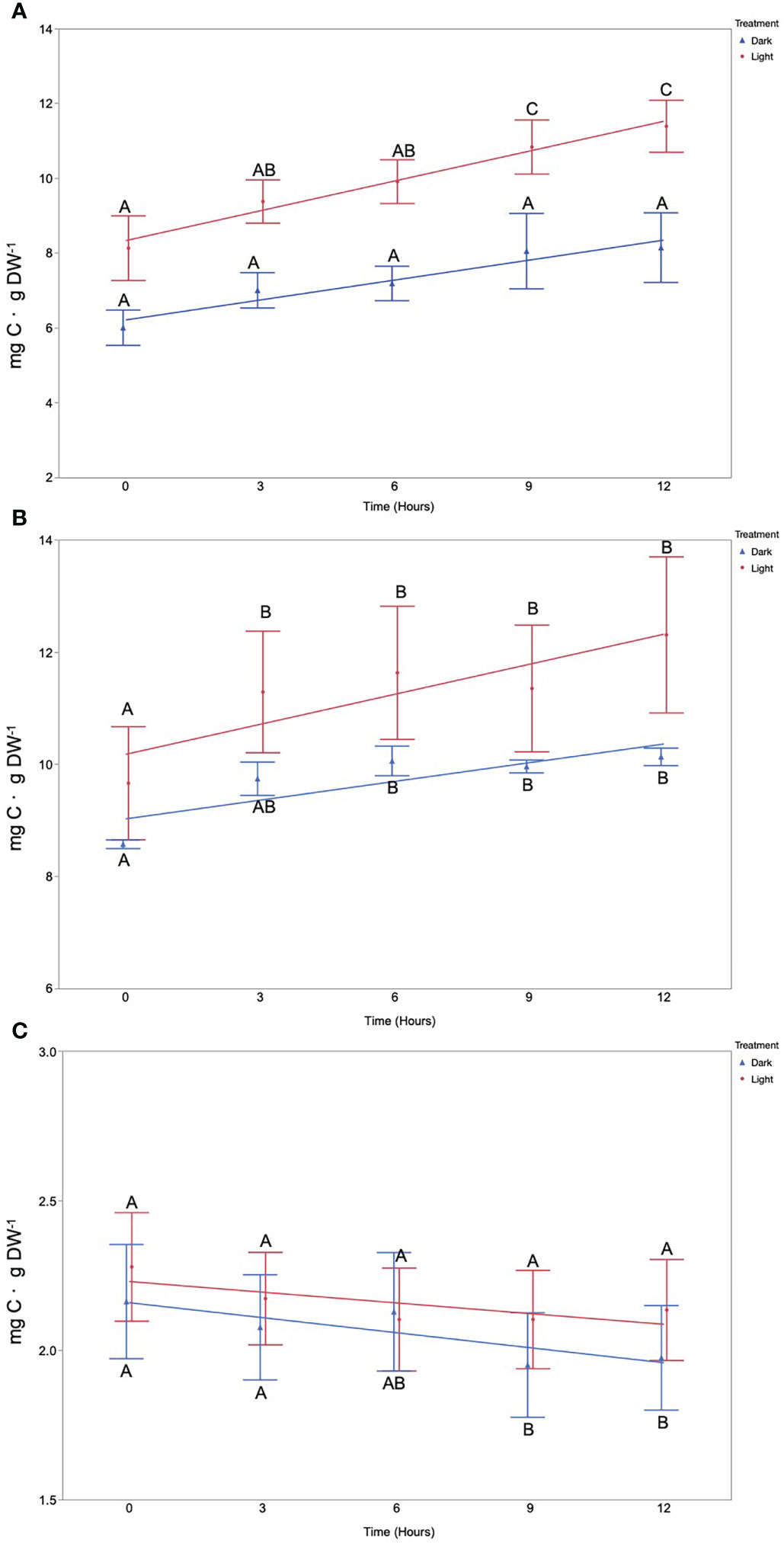
Figure 2 Total dissolved organic carbon concentration per milligram of dry weight of algal material over a 12-h period under light and dark treatments for the species (A) (F) vesiculosus, (B) U. intestinalis, and (C) (F) lumbricalis. Different letters indicate significantly different DOC concentrations (mean ± 1 SE, n = 5) (Tukey’s HSD, a = 0.05).
The degree to which released DOC products were consumed by heterotrophic bacteria varied between the species and the treatment it was produced under. Intial mean DOC concentration produced by F. vesiculosus under the light treatment decreased significantly after 120 h from 8.07 mg C·L−1 to 6.40 mg C·L−1, representing a decrease of 20.7% (Figure 3). No change in mean DOC concentration under the dark treatment was observed for the F. vesiculosus dark treatment (Figure 3). The initial mean DOC concentration produced by U. intestinalis under the light and dark treaments significantly decreased after a period of 120 h with a change in concentration of 7.93 to 6.89 mg C·L−1 for light and 7.34 to 6.67 mg C·L−1 for dark, representing a decrerase of 13.1% and 9.1% respectively (Figure 3). No significant difference was detected between the intial and final DOC concentrations for F. lumbricalis light and dark treaments (Figure 3). In regard to the seawater controls, the light treamtent displayed a decrease in DOC concetration from 6.36 to 5.8 mg C·L−1, representing a decrease of 8.8%; no change in the dark treatment was observed (Figure 3).
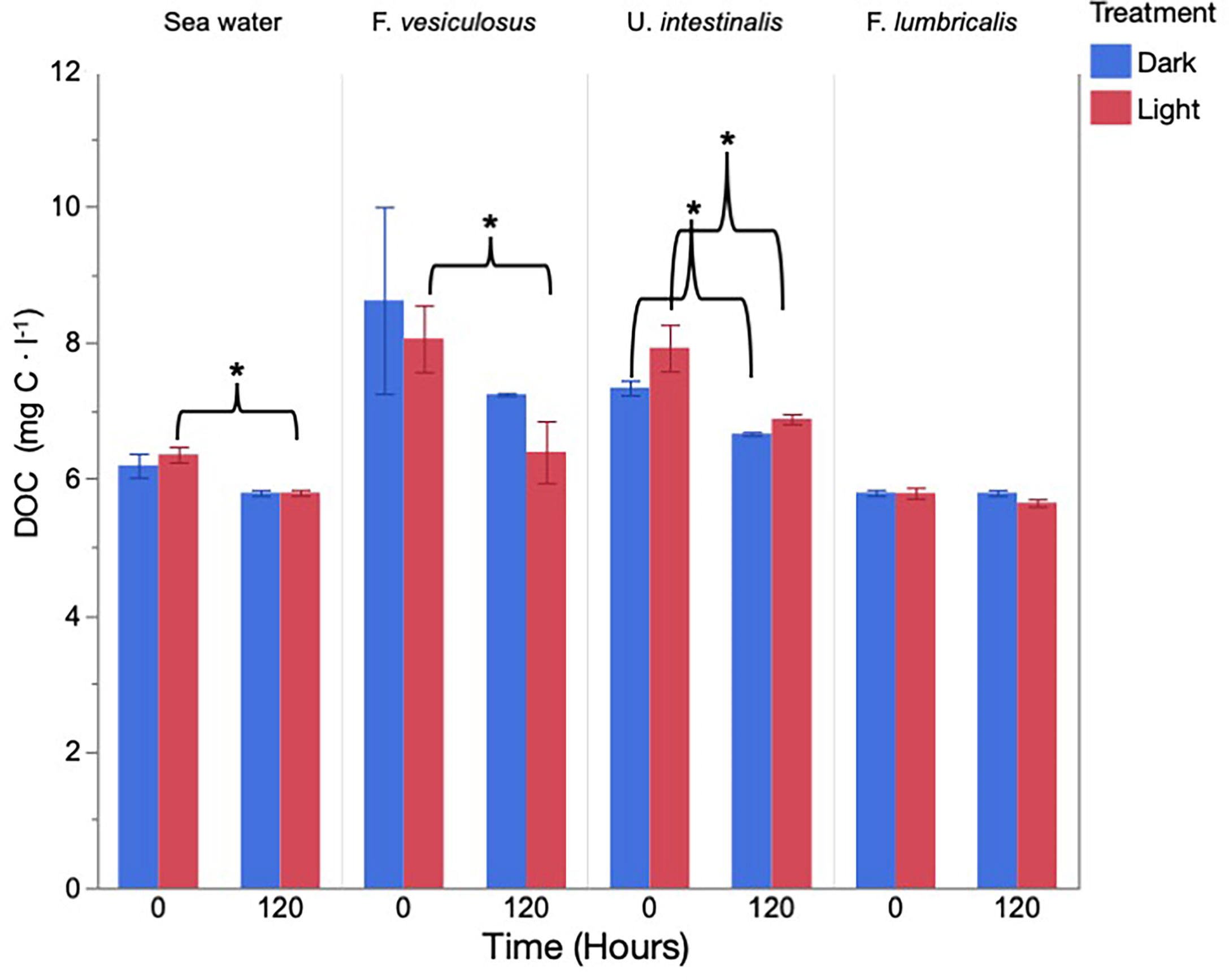
Figure 3 Consumption of total dissolved organic carbon released by macroalgae under light and dark treatments after a period of 120 h measured as milligrams of carbon per liter. * = significant difference (<0.05) after time for the given species.
DOC produced by both F. vesiculosus and U. intestinalis under the light treatment was assimilated more readily as a metric of bacterial biomass production (BBP) than that produced under the dark treatment achieving higher total BBP rates. Mean BBP peaked at 219 µg C·L−1·D−1 at 24 h for DOC released by F. vesiculosus under the light treatment representing a 47.18% increase over F. vesiculosus DOC produced in the dark which peaked at 135.4 µg C·L−1·D−1 at 48 h (Figure 4). U. intestinalis displayed a similar pattern of BBP production with maximum mean BBP production achieved for DOC released under light conditions occurring on day 2 at 214 µg C·L−1·D−1 with maximum BBP for DOC produced under dark reaching 152.8 µg C ·L−1·D−1 after 24 h representing a 28.6% decrease (Figure 4). F. lumbricalis displayed a pattern of rapid decline through time in BBP for both the light and dark treatments.
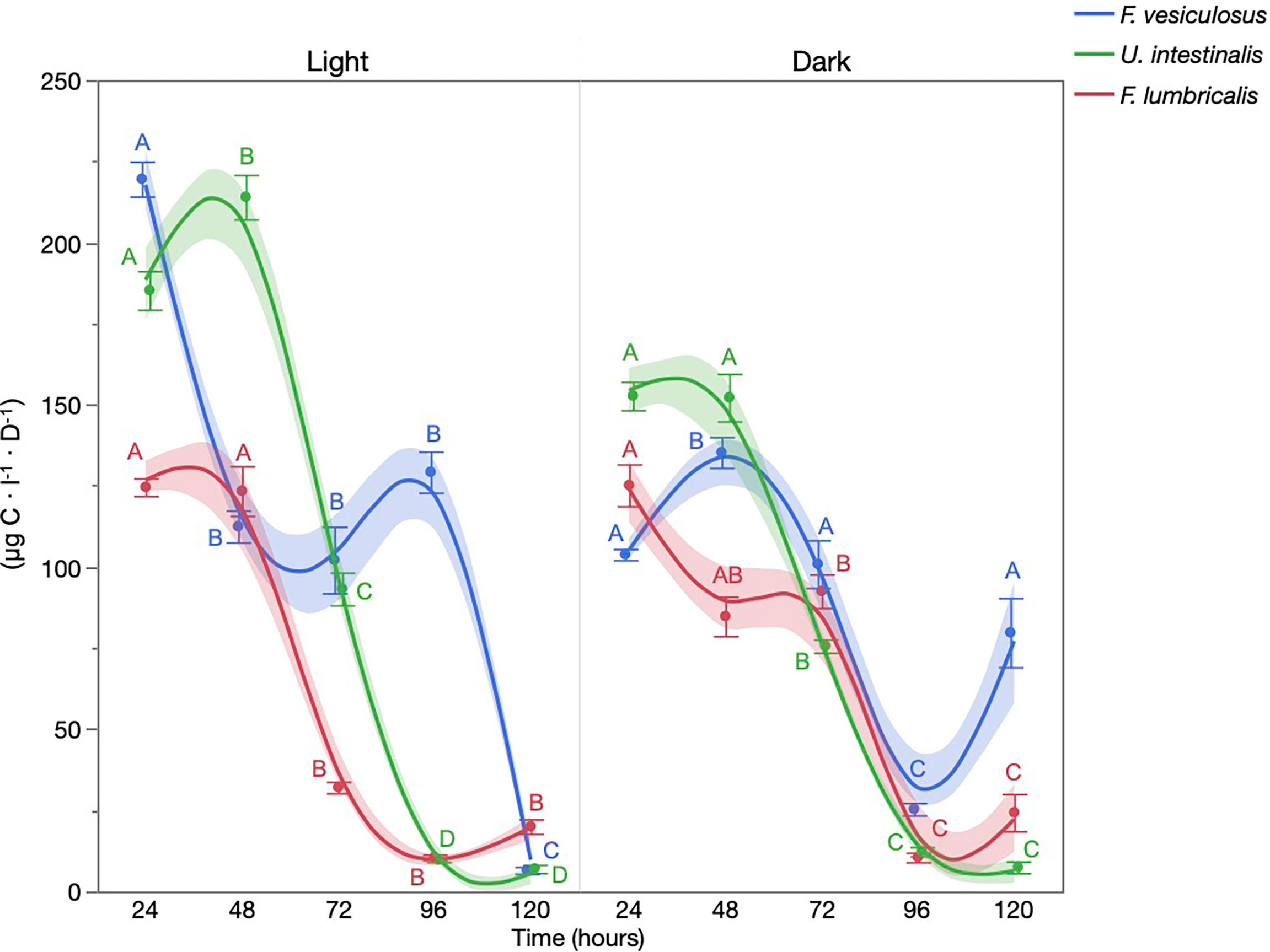
Figure 4 Heterotrophic bacterial biomass production (BBP) or carbon flux estimated (µg C·L−1·D−1) based on tritiated leucine incorporation rates for macroalgae DOC released under light and dark treatments. Different letters indicate significant difference within light and dark treatments (mean ± 1 SE, n = 5) (Tukey’s HSD, a = 0.05) (Smooth line = cubic spline with a lambda of 0.05).
Discussion
In this study, we investigated both the production/release of DOC by key Baltic Sea macrophytes under both light and dark conditions and related this production to its consumption by marine heterotrophic bacteria. We demonstrate that DOC production varies between species with photosynthetic activity induced by light having a positive effect on DOC release for F. vesiculosus and dark conditions having a positive effect on DOC release by U. intestinalis (Figure 2). In the second component of this experiment, DOC released by the prior examined macroalgal species was assessed in terms of its ability to be assimilated by heterotrophic bacteria, and its overall lability was evaluated. The results show that the lability of the released products is specific to the species and is affected by the conditions in which it was released, i.e., light vs. dark (Figure 3). A proportion of the measured DOC released by F. vesiculosus under the light treatment as well as by U. intestinalis under the light and dark treatments was found to be labile with the products released acted upon by heterotrophic bacteria. In contrast, no consumption of DOC was observed for the F. lumbricalis incubation supporting the previous measurements depicting little to no DOC released (Figure 3). With regard to the seawater control only a small decrease of 0.54 mg C·L−1 was observed for the light treamtent and no change was observed for the dark after 120 h, indicating the background seawater as being largely refractory (Figure 3).
In addition to direct measurements of DOC concentration, bacterial biomass production by heterotrophic bacteria was used to evaluate DOC assimilation and carbon incorporation into the microbial loop. The patterns of consumption reported here largely mirror those discussed earlier with DOC uptake being predicted by a combination of the species and whether photosynthesis was occurring. In particular, DOC produced under photosynthetic conditions was acted upon more readily than that produced in the dark, indicating that either (a) photosynthesis increases the lability of the released DOC or (b) low concentrations of DOC restricted the bacterial response (Figure 4). After a period of 12 h under maximum photosynthetic conditions, F. vesiculosus and U. intestinalis DOC supported BBP rates of 219 µg C·L−1·D−1 and 214 µg C·L−1·D−1 for each gram of dry weight material, respectively. It should be noted that depending on the given microbial community’s growth efficiency, a portion of this carbon would be respired away rather than entering the microbial loop and higher trophic levels under natural conditions.
Our results demonstrate that macroalgal DOC production is linked not only to the underlying macroalgal species and their unique physiology, but also to external environmental factors. As described previously, two main hypotheses attempt to explain the biological mechanisms for macroalgal DOC release. The stochiometric overflow hypothesis as described in Fogg (1983) asserts that DOC release is related to surplus photosynthate production under high photosynthetic conditions. In contrast, the photosynthate diffusion hypothesis posits that DOC release is passive in nature and is related to low-molecular-weight compounds diffusing through cell membranes during cellular growth or lysis. The data presented in this study would imply that both hypotheses occur in some capacity and would act to explain the observed trends, but fall short of providing a unifying explanation for DOC. Primarily, we can see that DOC is produced consistently by F. vesiculosus under saturating light conditions demonstrating the loss of photosynthetic products and that U. intestinalis released products under both light and dark conditions, implying that, at least to some degree, the passive loss of products occurs for this species. Interestingly, F. lumbricalis’ lack of DOC production under both light and dark conditions indicates that DOC release is not a universal trait of marine macroalgae. As a whole, a single unifying explanation for why macroalgae release DOC is not possible here, with the results suggesting that macroalgae release DOC for different reasons and likely through differing mechanisms.
Establishing the function and magnitude of the microbial loop in the ocean remains a substantial challenge of modern marine ecology. The task is inherently challenged by the methodological difficulties imposed in part by the small size and dilute concentrations of microbes in complex solutions of contaminating organisms and dead particles (Kemp et al., 1993). Understanding the role macroalgae-derived DOC has in supporting bacterial biomass production and the level to which this production introduces fixed carbon into coastal food webs is drastically limited by a lack of primary measurements. To determine the ecological importance of macroalgae DOC release rates measured in this study, it is important to contextualize them with regard to a macroalgae’s specific life characteristics.
F. vesiculosus is a highly productive, slow-growing, perennial canopy-forming brown macroalgae. While it has long been established that macroalgae canopies are some of the most productive habitats on Earth (Mann, 1973), numerous works since have focused on better quantifying this productivity at a species-specific level. (Attard et al., 2019) evaluated the daily net ecosystem (NEM) metabolism of sublittoral F. vesiculosus in the Baltic Sea, determining that NEM was primarily positive, predicting a net autotrophic canopy for two-thirds of the year (due to elevated light levels) with annual canopy NEM amounting to 25 mol O2 m−2 year−1 or approximately six times that of net phytoplankton production. As such, the measured positive DOC production rates could reflect a majority of F. vesiculosus life history, particularly that of the vegetative growth season (summer) which, as this experiment has demonstrated, would release DOC at an elevated rate. Our findings would therefore be consistent with other works investigating the release of DOC by phaeophyceae. In particular, Macrocystis pyrifera (Ochrophyta, CA, USA), Fucus vesiculosus (Ochrophyta, RI, USA), and Nereocystis luetkeana (Ochrophyta, WA, USA) all demonstrated marked increases of DOC release under daytime conditions relative to that of night, with Sieburth (1969) suggesting that as much as 30%–40% of F. vesiculosus total fixed carbon is released back as dissolved organic matter (Sieburth, 1969; Reed et al., 2015; Weigel and Pfister, 2021). Furthermore, as the DOC released under light conditions was shown to be readily assimilated into bacterial biomass, it can be stated that F. vesiculosus actively supports heterotrophic bacterial growth within the water column. We can therefore assert that F. vesiculosus represents a potentially important source of labile carbon for the microbial loop in coastal systems.
Similar to F. vesiculosus, U. intestinalis is notable for its high productivity, particularly as a species that blooms (Martins and Marques, 2002). Similar to F. vesiculosus, U. intestinalis is notable for its high productivity, particularly as a species that blooms (Martins and Marques, 2002). Within the context of this study, direct measurements of DOC released by U. intestinalis were shown to increase through time for both the light and dark treatments with the light treatment achieving a peak hourly release rate 69.2% higher than that of the dark treatment (Figure 2). The release of DOC in the dark treatment implies that, to some degree, passive leakage does occur. In addition, the DOC concentration of both the light and dark treatments was observed to decrease after 120 h with a natural bacterial community present indicating released products were at least partially consumed (Figure 3). Interestingly, when comparing the peak BBP rate for both treatments, it can be observed that the DOC released under the light treatment stimulated bacterial production to a much greater degree than that of the dark treatment, achieving a peak BBP rate that is 40.1% higher (Figure 4). This finding demonstrates that U. intestinalis DOC release supports the microbial production primarily during photosynthesis. Several factors may help explain this observed trend. Firstly, and perhaps most likely, is that the elevated levels of DOC released under photosynthetic conditions enabled heterotrophic bacteria to be more readily acted upon, elevating the BBP rate. An additional factor, and one that does not exclude the previous one, is that the products released by the light treatment are inherently more labile than those that are passively released and as such are more readily assimilated into bacterial biomass. Lastly and perhaps the least likely in this instance is the strong propensity of Ulva sp. for the production of secondary metabolites that display antimicrobial, antiherbivory, and antifouling properties, particularly as a by-product of photosynthesis (Nelson et al., 2003; Dobretsov et al., 2006; Lane and Kubanek, 2008; Twigg et al., 2020). In particular, Ulva sp. is known to release hydrogen peroxide in high concentrations (upwards of five times greater than other species) with in situ measurements showing that it inhibits bacterial production and carbon flow through the microbial loop by up to 75% (Twigg et al., 2020). As such, released hydrogen peroxide may have prevented DOC incorporation by bacteria via stunting bacterial growth. If this is the case, then released U. intestinalis DOC may represent a source of latent production once either the hydrogen peroxide has decayed (Petasne and Zika, 1997), or the DOC has been exported away from the system where it can be incorporated into the microbial loop. However, this would suggest that U. intestinalis released hydrogen peroxide to a greater affect under the dark treatment even though hydrogen peroxide production is typically viewed as a photosynthetic process derived from the Mehler reaction (Mehler, 1951; Collén et al., 1995). Even so, non-photosynthetic pathways do exist, primarily through that of incomplete reduction of oxygen in the mitochondria as well as through superoxide dismutase, an enzyme that catalyzes the dismutation of superoxide radicals into oxygen and hydrogen peroxide. (Collén et al., 1995; Rezayian et al., 2019). By any means, a more detailed examination of the effect of hydrogen peroxide on consumption is needed in order to fully evaluate its role in DOC uptake.
F. lumbricalis is widely distributed in the sublittoral zone of the Baltic. While typically found growing on hard rocky substrate, it is somewhat unique in that it forms large unattached “meadows” in deeper low light waters (Martin et al., 2006a; Martin et al., 2006b). For this reason, F. lumbricalis may represent a large source of both coastal and “deep” water DOC. The mesocosm data presented in this study show that F. lumbricalis does not readily release DOC under light or dark conditions (Figure 2). This is supported by the both lack of DOC consumption after a period of 120 h and reduced BBP measurements (Figures 3, 4). Therefore, this study would indicate that F. lumbricalis does not meaningfully support deeper water microbial loop function. As a whole, few studies (10) have examined the release of DOC by marine rhodophyta under ambient conditions and until now none have attempted to directly connect the release of dissolved products to that of heterotrophic bacterial production (Paine et al., 2021). A review by Paine et al. (2021) examined published values of DOC release by the three major algal divisions, reporting mean release rate to be lowest among Rhodophyta at 10.25 μmol C·g DW−1·h−1 when compared to 36.39 μmol C·g DW−1·h−1 for Chlorophyta and 20.25 μmol C·g DW−1·h−1 for Ochrophyta. However, large ranges in the reported rates and lack of published values highlight the need for the further examination of DOC rates and in particular for those of Rhodophytes.
Patterns in primary production as related to a given macroalgal species life strategy could help explain observed trends in DOC release rates. In particular, the regulation of cell homeostasis conceptualized under the “stochiometric overflow hypothesis” describes a physiological mechanism in which the exudation of DOC acts as a “release valve” for photosynthetic products that cannot readily be stored (Fogg, 1983, Wood and Van Valen, 1990; Borchard and Engel, 2015). More specifically, the release of DOC protects the cell from detrimental levels of photosynthate buildup under high light conditions and allows for RuBisCO to maintain maximum photosynthetic capacity. The overflow hypothesis would therefore adequality explain the observed DOC production trends reported here for all three of the investigated species when considerations are made for the habitat in which these species are found in and the growth strategies in which they employ. For instance, the deeper water F. lumbricalis typically photosynthesizes in low- or poor-quality light conditions. The need for a photosynthate release mechanism would not be needed as high growth and metabolic rates would rarely, if ever, be achieved. In contrast, F. vesiculosus is a slow-growing perennial species that inhabits in the high light, shallow sublittoral zone. As such, employing a mechanism to actively regulate internal photosynthate concentrations would be crucial as the species would be restricted in its ability to reduce storage pressures by assimilating photosynthetic products into biomass through the formation of new tissue structures. Like F. vesiculosus, U. intestinalis also grows in shallow, high light environments; however, unlike F. vesiculosus, U. intestinalis displays a high growth rate. As such, U. intestinalis can likely more readily utilize and incorporate photosynthate into new tissue structures and would reduce the need for DOC release. Direct measurements of net primary production (NPP) further support this conclusion. Net primary production rates for the three species investigated here were measured by Pajusalu et al. (2013). Their work reports U. intestinalis as having a markedly higher NPP than both F. vesiculosus and F. lumbricalis, with F. vesiculosus having the lowest NPP of the measured species and displaying the slowest growth rate. Overall, it should be recognized that the factors and mechanisms behind both DOC production and consumption are as complex as they are multifaceted. In this regard, attempts to provide a singular unifying explanation for all groups may fall short.
Salinity represents a major abiotic stress for aquatic organisms and one that is particularly relevant within the context of the Baltic Sea. In terms of DOC production, salinity is believed to affect DOC rates of release by altering the osmotic balance within macroalgal cells, which is compensated for by modifying cellular solute concentrations (Kirst, 1996). To date, only two studies have expressly examined the impact salinity has on DOC release rates (Sieburth, 1969; Pregnall, 1983). Sieburth (1969) examined fully marine specimens of F. vesiculosus (Rhode Island, USA) under differing salinity concentrations. DOC release rates were observed to decrease with salinity from 0.429 mg C·g DW−1·h−1 (reported as 35.72 μmol) at 30.46‰ salinity and 0.13 mg C·g DW−1·h−1 (reported as 11.18 μmol) at a 10.15‰ salinity (Sieburth, 1969). The reported results would therefore match those observed here with brackish water Baltic Sea F. vesiculosus specimens releasing DOC at a reduced rate compared to their marine counterparts. Furthermore, Enteromorpha prolifera was examined in a similar fashion by Pregnall (1983), who reported an inverse trend whereby DOC release rates increased with decreasing salinity with 0.13–0.57 mg C·g DW−1·h−1 released at a salinity of 30‰ and 1.02 mg C·g DW−1·h−1 at 5‰ salinity. Interestingly, we observe that U. intestinalis, a similar species to that of E. prolifera, released DOC equivalent to E. prolifera in a marine setting, indicating that this trend does not necessarily carry across between species. This discrepancy could be explained by U. intestinalis successfully adapting to the lower salinity of the Baltic Sea and as such better able to regulate internal osmotic balance without the need for elevated DOC release. As it stands, studies examining the effect salinity has on DOC for species in the phylum Rhodophyta are entirely lacking.
Understanding how the world’s changing seas will affect coastal ecosystems represents a major challenge for the field of marine science. Numerous physical, chemical, and biological factors acting in conjunction are predicted to influence macroalgae physiology in a variety of ways (Diaz-Pulido et al., 2007; Harley et al., 2012). Such factors include ocean acidification (Koch et al., 2013; Pajusalu et al., 2013; Fernández et al., 2015; Cornwall and Hurd, 2019); increased surface seawater temperatures and associated heat waves (Wernberg et al., 2016; Britton et al., 2020); eutrophication as a consequence of increased nitrogen loading due to agriculture and poor land use management (Voss et al., 2011; Harley et al., 2012; Roleda and Hurd, 2019); changes in light regimes due to factors such as turbidity, sedimentation, and/or canopy structure (Desmond et al., 2015); and changes in salinity due to ice melt or alterations in precipitation patterns (Vuorinen et al., 2015; Meredith et al., 2019). These environmental factors are predicted to substantially alter coastal ecosystem function including through changes in carbon flow and DOC cycling (Lønborg et al., 2020). For example, Pajusalu et al. (2013) evaluated the effect decreasing water pH would have on the net primary production for both U. intestinalis and F. vesiculosus. Their study found that increased CO2 levels in seawater favored the photosynthetic activity of the macroalgae U. intestinalis but not F. vesiculosus and concluded that elevated CO2 levels will favor the production of fast-growing filamentous species. From this, we can speculate that elevated CO2 could enhance the viability of U. intestinalis through increased photosynthesis and decrease the contribution of DOC from F. vesiculosus as it is displaced by faster-growing, more opportunistic species. Such changes may act to disrupt the flow of carbon either through altering rates of production, the lability of the released products, or limiting the ability for heterotrophic bacteria to assimilate it. In reality, evaluating the true extent of these changes is highly difficult with our current limited understanding of both DOC production and consumption. Such difficulties are only exacerbated by the complexity of the systems in which they operate in. It is therefore important that we establish baselines of production now so that we can better evaluate potential changes in the future.
Summary statement
Measurements of primary production as an isolated metric are insufficient in developing a cohesive understanding of the importance primary producers, particularly that of macrophytes, have on the movement of carbon in marine systems. As it stands, the literature remains sparse in terms of published DOC values for macroalgae and even more so for the lability of a given species’ DOC. This work highlights the importance macroalgae play in supporting coastal ecosystem function through the release of DOC. In particular, we report key rates of production for important Baltic Sea macroalgal species and relate this release directly to its consumption by heterotrophic bacteria. Additionally, we examine how these rates vary between species and how factors such as light influence the rate of release. Future studies should prioritize understanding the mechanisms of release (passive vs. active release) and energy balance associated with this, the composition and lability of released products, and finally the environmental factors that regulate release particularly in relation to how these factors may alter with our changing seas. By further developing our understanding of the pathways macroalgae-derived carbon takes, a more cohesive understanding of marine food web function can be formed.
Data availability statement
The raw data supporting the conclusions of this article will be made available by the authors, without undue reservation.
Author contributions
JH, FB, CH, and GM designed the experiments. JH and GA conducted the field work and experiments. JH and IT conducted the statistical analysis of the data. JH wrote the manuscript with FB, CH, GA, and GM, and provided editorial advice. All authors contributed to the article and approved the submitted version.
Funding
Funding for this study was provided through basic research and infrastructure fund of Estonian Marine Institute.
Acknowledgments
The authors would like to acknowledge those who have aided us in the completion of this work. We would like to thank Irma Lubiene, Klaipeda University, Marine Research Institute, for assistance in DOC sample analysis and Maris-Johanna Tahk, University of Tartu, Institute of Chemistry, for aide with scintillation counting.
Conflict of interest
The authors declare that the research was conducted in the absence of any commercial or financial relationships that could be construed as a potential conflict of interest.
Publisher’s note
All claims expressed in this article are solely those of the authors and do not necessarily represent those of their affiliated organizations, or those of the publisher, the editors and the reviewers. Any product that may be evaluated in this article, or claim that may be made by its manufacturer, is not guaranteed or endorsed by the publisher.
References
Agusti S., Duarte C. M. (2013). Phytoplankton lysis predicts dissolved organic carbon release in marine plankton communities. Biogeosciences 10 (3), 1259–1264. doi: 10.5194/bg-10-1259-2013
Attard K. M., Rodil I. F., Berg P., Norkko J., Norkko A., Glud R. N. (2019). Seasonal metabolism and carbon export potential of a key coastal habitat: The perennial canopy-forming macroalga fucus vesiculosus. Limnol. Oceanog. 64 (1), 149–164. doi: 10.1002/lno.11026
Azam F., Fenchel T., Field J. G., Gray J. S., Meyer-Reil L. A., Thingstad F. (1983). The ecological role of water-column microbes in the sea. Mar. Ecol. Prog. Ser. 10, 257–263.
Bjørrisen P. K. (1988). Phytoplankton exudation of organic matter: Why do healthy cells do it? Limnol. Oceanog. 33 (1), 151–154. doi: 10.4319/lo.1988.33.1.0151
Borchard C., Engel A. (2015). Size-fractionated dissolved primary production and carbohydrate composition of the coccolithophore emiliania huxleyi. Biogeosciences 12 (4), 1271–1284. doi: 10.5194/bg-12-1271-2015
Britton D., Schmid M., Noisette F., Havenhand J. N., Paine E. R., McGraw C. M., et al. (2020). Adjustments in fatty acid composition is a mechanism that can explain resilience to marine heatwaves and future ocean conditions in the habitat-forming seaweed phyllospora comosa (Labillardière) c. agardh. Global Change Biol. 26 (6), 3512–3524. doi: 10.1111/gcb.15052
Collén J., Del Rio M. J., García-Reina G., Pedersén M. (1995). Photosynthetic production of hydrogen peroxide by ulva rigida c. Ag.(Chlorophyta). Planta 196 (2), 225–230. doi: 10.1007/BF00201378
Cornwall C. E., Hurd C. L. (2019). Variability in the benefits of ocean acidification to photosynthetic rates of macroalgae without CO2-concentrating mechanisms. Mar. Freshw. Res. 71 (3), 275–280. doi: 10.1071/MF19134
Desmond M. J., Pritchard D. W., Hepburn C. D. (2015). Light limitation within southern new Zealand kelp forest communities. PLoS One 10 (4), e0123676. doi: 10.1371/journal.pone.0123676
Diaz-Pulido G., McCook L. J., Larkum A. W., Lotze H. K., Raven J. A., Schaffelke B., et al. (2007) Vulnerability of macroalgae of the great barrier reef to climate change. Available at: https://hdl.handle.net/11017/540.
Dobretsov S., Dahms H. U., Harder T., Qian P. Y. (2006). Allelochemical defense against epibiosis in the macroalga caulerpa racemosa var. turbinata. Mar. Ecol. Prog. Ser. 318, 165–175. doi: 10.3354/meps318165
Duarte C. M., Cebrián J. (1996). The fate of marine autotrophic production. Limnol. Oceanog. 41 (8), 1758–1766. doi: 10.4319/lo.1996.41.8.1758
Duggins D. O., Simenstad C. A., Estes J. A. (1989). Magnification of secondary production by kelp detritus in coastal marine ecosystems. Science 245 (4914), 170–173. doi: 10.1126/science.245.4914.170
Fernández P. A., Roleda M. Y., Hurd C. L. (2015). Effects of ocean acidification on the photosynthetic performance, carbonic anhydrase activity and growth of the giant kelp macrocystis pyrifera. Photosynthesis Res. 124 (3), 293–304. doi: 10.1007/s11120-015-0138-5
Fogg G. E. (1983). The ecological significance of extracellular products of phytoplankton photosynthesis. Botanica Marina 26, 3–14. doi: 10.1515/botm.1983.26.1.3
Gerard V. A. (1976). Some aspects of material dynamics and energy flow in a kelp forest in Monterey bay (California: Univ. of California).
Harley C. D., Anderson K. M., Demes K. W., Jorve J. P., Kordas R. L., Coyle T. A., et al. (2012). Effects of climate change on global seaweed communities. J. Phycology 48 (5), 1064–1078. doi: 10.1111/j.1529-8817.2012.01224.x
Hatcher B. G., Chapman A. O., Mann K. H. (1977). An annual carbon budget for the kelp laminaria longicruris. Mar. Biol. 44 (1), 85–96. doi: 10.1007/BF00386909
Kautsky H., Martin G., Snoeijs-Leijonmalm P. (2017). “The phytobenthic zone,” in Biological oceanography of the Baltic Sea (Dordrecht: Springer), 387–455. doi: 10.1007/978-94-007-0668-2_11
Kemp P. F., Lee S., LaRoche J. (1993). Estimating the growth rate of slowly growing marine bacteria from RNA content. Appl. Environ. Microbiol. 59 (8), 2594–2601. doi: 10.1128/aem.59.8.2594-2601.1993
Kirst G. O. (1996). “Osmotic adjustment in phytoplankton and macroalgae,” in Biological and environmental chemistry of DMSP and related sulfonium compounds (Boston, MA: Springer), 121–129. doi: 10.1007/978-1-4613-0377-0_11
Koch M., Bowes G., Ross C., Zhang X. H. (2013). Climate change and ocean acidification effects on seagrasses and marine macroalgae. Global Change Biol. 19 (1), 103–132. doi: 10.1111/j.1365-2486.2012.02791.x
Lønborg C., Carreira C., Jickells T., Álvarez-Salgado X. A. (2020). Impacts of global change on ocean dissolved organic carbon (DOC) cycling. Front. Mar. Sci. 7. doi: 10.3389/fmars.2020.00466
Lane A. L., Kubanek J. (2008). “Secondary metabolite defenses against pathogens and biofoulers,” in Algal chemical ecology (Berlin, Heidelberg: Springer), 229–243.
Livanou E., Lagaria A., Psarra S., Lika K. (2019). A DEB-based approach of modeling dissolved organic matter release by phytoplankton. J. Sea Res. 143, 140–151. doi: 10.1016/j.seares.2018.07.016
Møller E. F., Thor P., Nielsen T. G. (2003). Production of DOC by calanus finmarchicus, c. glacialis and c. hyperboreus through sloppy feeding and leakage from fecal pellets. Mar. Ecol. Prog. Ser. 262, 185–191. doi: 10.3354/meps262185
Mann K. H. (1973). Seaweeds: Their productivity and strategy for growth: The role of large marine algae in coastal productivity is far more important than has been suspected. Science 182 (4116), 975–981. doi: 10.1126/science.182.4116.975
Martin G., Paalme T., Torn K. (2006a). “Seasonality pattern of biomass accumulation in a drifting furcellaria lumbricalis community in the waters of the West Estonian archipelago, Baltic Sea,” in Eighteenth international seaweed symposium (Dordrecht: Springer), 331–337. doi: 10.1007/978-1-4020-5670-3_40
Martin G., Paalme T., Torn K. (2006b). Growth and production rates of loose-lying and attached forms of the red algae furcellaria lumbricalis and coccotylus truncatus in kassari bay, the West Estonian archipelago Sea. Hydrobiologia 554 (1), 107–115. doi: 10.1007/s10750-005-1010-y
Martins I., Marques J. C. (2002). A model for the growth of opportunistic macroalgae (Enteromorpha sp.) in tidal estuaries. Estuarine Coast. Shelf Sci. 55 (2), 247–257. doi: 10.1006/ecss.2001.0900
Mehler A. H. (1951). Studies on reactions of illuminated chloroplasts: I. mechanism of the reduction of oxygen and other hill reagents. Arch. Biochem. Biophysics 33 (1), 65–77. doi: 10.1016/0003-9861(51)90082-3
Meredith M., Sommerkorn M., Cassotta S., Derksen C., Ekaykin A., Hollowed A., et al. (2019). “Polar regions,” in IPCC special report on the ocean and cryosphere in a changing climate. Eds. Pörtner H. O., Roberts D. C., Masson-Delmotte V., P. Zhai M. T., Poloczanska E., Mintenbeck K., Alegría A., Nicolai M., Okem A., Petzold J., Rama B., Weyer N. M. (Cambridge, UK and New York, NY, USA: Cambridge University Press), 203–320.
Miller R. J., Mann K. H., Scarratt D. J. (1971). Production potential of a seaweed–lobster community in eastern Canada. J. Fisheries Board Canada 28 (11), 1733–1738. doi: 10.1139/f71-256
Nelson T. A., Lee D. J., Smith B. C. (2003). Are “green tides” harmful algal blooms? toxic properties of water-soluble extracts from two bloom-forming macroalgae, ulva fenestrata and ulvaria obscura (Ulvophyceae). J. Phycology 39 (5), 874–879. doi: 10.1046/j.1529-8817.2003.02157.x
Newell R. C., Field J. G., Griffiths C. L. (1982). Energy-balance and significance of microorganisms in a kelp bed community. Mar. Ecol. Prog. Ser. 8 (1), 103–113.
Paine E. R., Schmid M., Boyd P. W., Diaz-Pulido G., Hurd C. L. (2021). Rate and fate of dissolved organic carbon release by seaweeds: a missing link in the coastal ocean carbon cycle. J. Phycology 57 (5), 1375–1391. doi: 10.1111/jpy.13198
Pajusalu L., Martin G., Põllumäe A., Paalme T. (2013). Results of laboratory and field experiments of the direct effect of increasing CO2 on net primary production of macroalgal species in brackish-water ecosystems. Proc. Estonian Acad. Sci. 62 (2), 148. doi: 10.3176/proc.2013.2.09
Petasne R. G., Zika R. G. (1997). Hydrogen peroxide lifetimes in south Florida coastal and offshore waters. Mar. Chem. 56 (3-4), 215–225. doi: 10.1016/S0304-4203(96)00072-2
Pomeroy L. R. (1974). The ocean's food web, a changing paradigm. Bioscience 24 (9), 499–504. doi: 10.2307/1296885
Pomeroy L. R., leB. Williams P. J., Azam F., Hobbie J. E. (2007). The microbial loop. Oceanography 20 (2), 28–33.
Pregnall A. M. (1983). Release of dissolved organic carbon from the estuarine intertidal macroalga enteromorpha prolifera. Mar. Biol. 73 (1), 37–42. doi: 10.1007/BF00396283
Reed D. C., Carlson C. A., Halewood E. R., Nelson J. C., Harrer S. L., Rassweiler A., et al. (2015). Patterns and controls of reef-scale production of dissolved organic carbon by giant kelp m acrocystis pyrifera. Limnol. Oceanog. 60 (6), 1996–2008. doi: 10.1002/lno.10154
Rezayian M., Niknam V., Ebrahimzadeh H. (2019). Oxidative damage and antioxidative system in algae. Toxicol. Rep. 6, 1309–1313. doi: 10.1016/j.toxrep.2019.10.001
Roleda M. Y., Hurd C. L. (2019). Seaweed nutrient physiology: application of concepts to aquaculture and bioremediation. Phycologia 58 (5), 552–562. doi: 10.1080/00318884.2019.1622920
Saba G. K., Steinberg D. K., Bronk D. A. (2011). The relative importance of sloppy feeding, excretion, and fecal pellet leaching in the release of dissolved carbon and nitrogen by acartia tonsa copepods. J. Exp. Mar. Biol. Ecol. 404 (1-2), 47–56. doi: 10.1016/j.jembe.2011.04.013
Sieburth J. M. (1969). Studies on algal substances in the sea. III. the production of extracellular organic matter by littoral marine algae. J. Exp. Mar. Biol. Ecol. 3 (3), 290–309. doi: 10.1016/0022-0981(69)90052-5
Smith D. C., Azam F. (1992). A simple, economical method for measuring bacterial protein synthesis rates in seawater using 3H-leucine. Mar. Microb. Food Webs 6 (2), 107–114.
Snoeijs-Leijonmalm P. (2017). “Patterns of biodiversity,” in Biological oceanography of the Baltic Sea (Dordrecht: Springer), 123–191. doi: 10.1007/978-94-007-0668-2_4
Snoeijs-Leijonmalm P., Andrén E. (2017). “Why is the Baltic Sea so special to live in?”. Biological oceanography of the Baltic Sea (Dordrecht: Springer), 23–84.
Sugimura Y., Suzuki Y. (1988). A high-temperature catalytic oxidation method for the determination of non-volatile dissolved organic carbon in seawater by direct injection of a liquid sample. Mar. Chem. 24 (2), 105–131. doi: 10.1016/0304-4203(88)90043-6
Thornton D. C. (2014). Dissolved organic matter (DOM) release by phytoplankton in the contemporary and future ocean. Eur. J. Phycology 49 (1), 20–46. doi: 10.1080/09670262.2013.875596
Twigg I. M., Baltar F., Hall J. R., Hepburn C. D. (2020). Revealing hydrogen peroxide as an external stressor in macrophyte-dominated coastal ecosystems. Oecologia 193 (3), 583–591. doi: 10.1007/s00442-020-04690-0
Voss M., Dippner J. W., Humborg C., Hürdler J., Korth F., Neumann T., et al. (2011). History and scenarios of future development of Baltic Sea eutrophication. Estuarine Coast. Shelf Sci. 92 (3), 307–322. doi: 10.1016/j.ecss.2010.12.037
Vuorinen I., Hänninen J., Rajasilta M., Laine P., Eklund J., Montesino-Pouzols F., et al. (2015). Scenario simulations of future salinity and ecological consequences in the Baltic Sea and adjacent north Sea areas–implications for environmental monitoring. Ecol. Indic. 50, 196–205. doi: 10.1016/j.ecolind.2014.10.019
Weigel B. L., Pfister C. A. (2021). The dynamics and stoichiometry of dissolved organic carbon release by kelp. Ecology 102 (2), e03221. doi: 10.1002/ecy.3221
Wernberg T., de Bettignies T., Joy B. A., Finnegan P. M. (2016). Physiological responses of habitat-forming seaweeds to increasing temperatures. Limnol. Oceanog. 61 (6), 2180–2190. doi: 10.1002/lno.10362
Keywords: macroalage, dissolved organic carbon (DOC), microbial loop, coastal ecosystem function, food web, carbon flow, remineralization, carbon cycles
Citation: Hall JR, Albert G, Twigg IM, Baltar F, Hepburn CD and Martin G (2022) The production of dissolved organic carbon by macroalgae and its consumption by marine bacteria: Implications for coastal ecosystems. Front. Mar. Sci. 9:934229. doi: 10.3389/fmars.2022.934229
Received: 02 May 2022; Accepted: 25 July 2022;
Published: 18 August 2022.
Edited by:
Tilmann Harder, University of Bremen, GermanyReviewed by:
Chaotian Xie, Jimei University, ChinaXuchen Wang, Ocean University of China, China
Hagen Buck-Wiese, Max Planck Society, Germany
Copyright © 2022 Hall, Albert, Twigg, Baltar, Hepburn and Martin. This is an open-access article distributed under the terms of the Creative Commons Attribution License (CC BY). The use, distribution or reproduction in other forums is permitted, provided the original author(s) and the copyright owner(s) are credited and that the original publication in this journal is cited, in accordance with accepted academic practice. No use, distribution or reproduction is permitted which does not comply with these terms.
*Correspondence: Jack R. Hall, amFjay5oYWxsQHV0LmVl