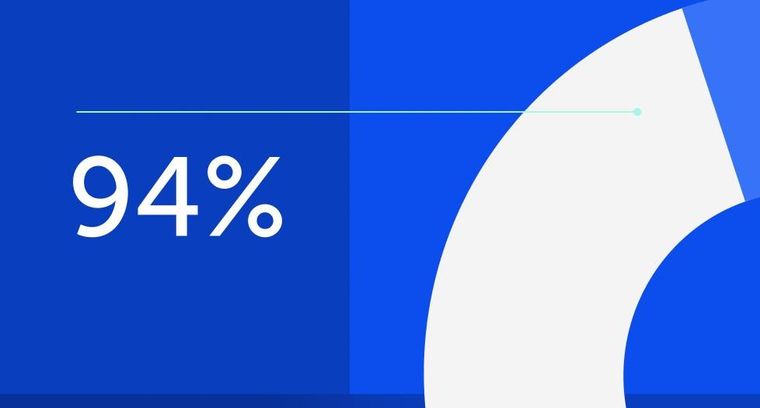
94% of researchers rate our articles as excellent or good
Learn more about the work of our research integrity team to safeguard the quality of each article we publish.
Find out more
ORIGINAL RESEARCH article
Front. Mar. Sci., 02 November 2022
Sec. Marine Conservation and Sustainability
Volume 9 - 2022 | https://doi.org/10.3389/fmars.2022.932963
This article is part of the Research TopicAfrican Ocean Stewardship: Navigating Ocean Conservation and Sustainable Marine and Coastal Resource Management in AfricaView all 18 articles
Mangrove ecosystems are often called “makers of land” due to their ability to promote deposition, trap, and augment sediments. Accurate location- and region-specific elevation information is required to assess and mitigate threats to mangroves caused by their vulnerability to sea-level rise. The provision of land building services by mangroves is primarily sediment-dependent. It is therefore influenced by local factors, including sediment availability and supply. In the present study from Kenya, we measured and examined the variations in surface elevation in mangroves at variable distances from the creek channel using a combination of surface-elevation tables and horizon markers for three years. Elevation changes varied with distance from the creek channel (p < 0.05), with both surface loss and gains recorded. Elevation changes varied between -80 mm (most significant subsidence) and 42 mm (highest accretion) in stations closer to the creek, while farther from the creek (~200 m away), elevation changes ranged between -68 mm (most significant subsidence) and 29 mm (highest accretion). However, net surface elevation changes over the three years showed that shallow subsidence occurred in both stations closer to the creek (-45 ± 7.2 mm) and those farthest from the creek (-20 ± 7.1 mm). At the same time, an average of 18 mm of sediments were accreted above the horizon markers translating to ~9 mm yr-1 of accretion, a rate larger than both the current global rates of sea-level rise (~3.1 mm yr-1) and local measured rates of sea-level rise (3.8 mm yr-1) in Mombasa, a tide-gauge station nearest (~100 km) to the study site. Cumulatively, sediment elevation changes in Vanga indicate that they are outpacing the current rates of sea-level rise. However, they could be vulnerable to predicted and accelerated rates. It, therefore, calls for more holistic management and monitoring of the dynamics within the mangrove forests and adjacent terrestrial hinterlands.
Mangroves are halophytic coastal plants that occupy a highly dynamic environment between the high and low watermarks in tropical and subtropical regions. Mangroves provide numerous ecological and economic services, including fish breeding habitats (Barbier, 2011; Igulu et al., 2014; Woodroffe et al., 2016; Saintilan et al., 2020), sediment traps and blue carbon sinks (Polidoro et al., 2010; Okello et al., 2013; Lang’at et al., 2014; Okello et al., 2020) and physical buffers against coastal erosion, wave action, flooding, and Tsunamis (Dahdouh-Guebas et al., 2005; Mazda and Wolanski, 2009; Ellison, 2015; Phan et al., 2015). However, mangroves and other critical coastal habitats, including temperate marshes, coral reefs, and seagrasses, are confronted by naturally and anthropogenically induced challenges. One of the imminent threats to mangroves is the rising and predicted sea-level rise (SLR) acceleration due to climate change’s consequential effects. This is inevitable as mangroves occupy a highly variable habitat sensitive to upward or downward sea–level change (Figure 1). For example, mangroves can be vulnerable due to low tidal range, e.g., the mangroves of Doula Cameroon, coastal subsidence for mangroves of Tikina Wai, Fiji (Ellison, 2015), and lack of space to migrate to due to human settlement, e.g., in the mangroves of Gazi, Kenya (Di Nitto et al., 2014). However, mangroves are less vulnerable in some regions due to favourable but localized dynamics, e.g., an uplifting coastline for the mangroves of Rufiji, Tanzania (Ellison, 2015). As reported in other studies, mangroves can indirectly moderate the effects of SLR and thus prevent possible loss of mangrove forests due to submergence (Krauss et al., 2014; Lovelock et al., 2015; Ward et al., 2016; Friess and McKee, 2021). Their complex root structure attenuates current flow velocities and enables sediment deposition, consolidation, and accretion (Furukawa and Wolanski, 1996; Krauss et al., 2014). Mangroves are thus inherently adapted to relative sea-level changes. This is further evident from mangrove resilience observed for paleo sea-level fluctuations, covering extensive time scales dating back to the late Oligocene (Soares, 2009; Collins et al., 2017; Woodroffe, 2018; Sugden, 2020). A combination of subsidence, sediment supply, enhanced tidal range and availability of space favor’s the mangroves of the South China Sea (Collins et al., 2017). Although SLR is an imminent threat to mangroves, sea-level drop (SLD), observed in the past, could also be detrimental to mangroves. SLD could be occasioned by land subsidence, a localized and primarily regional process (Figure 1).
Figure 1 Diagram showing the regional and localized dynamic processes controlling both the short- and long-term mangrove surface elevation.
Mangrove habitats have developed strategies to cope with SLR and SLD (Woodroffe et al., 2016). However, SLR could be less difficult to mitigate than SLD, mainly because SLR is gradual and would give time for a gradual response, adaptive measures, and other response/feedback mechanisms (Rogers, 2021). On the contrary, due to tectonic subsidence, SLD would be sudden, and mangroves would have no time to respond or adapt adequately. Research by Collins et al. (2017) has shown that mangroves can keep pace with localized tectonic subsidence of up to 3 mm yr-1. They attributed this to enhanced tidal range coupled with sediment supply, the reworking of sediments and the availability of accommodation space. SLR and SLD have varied effects on mangrove habitats (Gilman et al., 2008; Neubauer, 2008; Polidoro et al., 2010; Ellison, 2015; Ward et al., 2016). High rates of SLR would lead to the submergence of mangrove habitats and subsequent loss of entire ecosystems (Ellison, 2015). For example, with SLR rates of 20 mm yr-1, the Indo-Pacific mangroves are predicted to be submerged by as early as 2070 and 2100 with less than 2 m and 4 m tidal ranges, respectively (Lovelock et al., 2015). Due to local subsidence, SLD would enhance the tidal range, allowing for more sediment supply, deposition, and increased tidal velocities that would assist in reworking sediments to suitable sizes (Collins et al., 2017).
Any mangrove loss would result in a cascade of ecological, environmental, and economic losses of local and global magnitudes (Barbier, 2011). Some of the losses would include the loss of livelihoods (Hamza et al., 2020), wave and tsunami buffers (Dahdouh-Guebas et al., 2005) and ecological benefits (Barbier, 2011; Igulu et al., 2014). The loss of mangroves would also herald the loss of carbon sinks (Kusumaningtyas et al., 2019; Hamza et al., 2020) and ecosystem engineering services (Gutiérrez et al., 2012) that would be detrimental to other habitats.
Climate-change-driven global mean sea-level (GMSL), as derived from satellite altimetry, has been steadily rising since the 1960s and rose at an average rate of 3.2 ± 0.4 mm yr-1 between 1993 and 2009 (Nerem et al., 2018; Dangendorf et al., 2019). This rise was comparable to in-situ measured rates of 2.8 ± 0.8 mm yr-1 obtained for the same period in the tropical Pacific and the Indian Ocean (Church and White, 2011). Mangrove habitat migration, adaptation, and resilience towards current and projected SLR scenarios depend on the elevation equilibrium between mangrove surface elevation and rates of SLR (McKee, 2011; Friess and McKee, 2021). One of the ways that mangroves would respond to SLR has been predicted to be a migration to higher grounds (Di Nitto et al., 2014). This would largely depend on space availability to accommodate the migration (Rogers, 2021). Another is their indirect “engineering” through sediment trapping and promotion of sediment accumulation. This would enable mangroves to grow their surfaces with respect to SLR (Mcivor et al., 2013; Ellison, 2015). The equilibrium between mangrove surface elevation and the SLR rate depends on the net balance between material inputs (siliciclastic and organic material) and material removal via erosion and organic matter decomposition (Neubauer, 2008; Ward et al., 2016).
Additionally, sediment compaction by sediment overload results in water expulsion from the sediment column, leading to compaction and elevation changes. Through the constant supply of sediments and net accretion, it has been argued that mangroves can keep pace with SLR and avoid submergence (Sanders et al., 2008; Ward et al., 2016; Woodroffe et al., 2016). Mangrove surface elevation is a direct function of several biophysical processes, including the supply and deposition of lithogenic and organogenic sediments. Sediments delivered by fluvial systems and flood tides contribute to total sediment accumulation within the ecosystem. The cumulative effect of accretion, erosion, compaction, and subsidence (Figure 2) would lead to a net surface elevation.
Figure 2 Conceptual model of the biophysical processes that control the localized net surface elevation in mangroves. [+] indicating positive/incremental (accretion) influence while [-] indicate a negative (subsidence, loss of surface elevation) influence.
Localized and sudden or excessive input of sediments due to storm surges, floods or tsunamis to a mangrove habitat would disrupt this net equilibrium. The disruption would be detrimental to the survival of mangroves (Okello et al., 2013; Okello et al., 2020). For example, mangrove die-back and loss attributed to massive and sudden sedimentation after the 1997-1998 El-Niño event have been reported in Kenya (Kitheka et al., 2002; Bosire et al., 2014) and in Vietnam (Fagherazzi and Bryan, 2017; Nardin et al., 2021). In general, sediment deposition has been described as episodes of emplacement of sediment particles to a surface. However, sediment accumulation is the net sum of deposition episodes, a combination of deposition, resuspension, and re-deposition (Cahoon and Lynch, 1997; Woodroffe et al., 2016). Net mangrove surface elevation results from sediment accumulation, erosion, land uplift, subsidence, peat production, and root zone expansion (Krauss et al., 2010; Friess et al., 2019). Shallow subsidence is caused by sub-surface processes, including decomposition of organic matter, auto-compaction due to weight of subsequent deposition, and dissolution of minerals, which tend to shrink the volume of sediments (Cahoon and Lynch, 1997; Krauss et al., 2010; Webb et al., 2013). Other studies have shown that biotic processes - peat accumulation controlled by belowground root growth, expansion, and decomposition - can be the main driving factor of sediment accretion and surface elevation changes (Krauss et al., 2008; Kirwan et al., 2013; Crosby et al., 2016). Total sediment accumulation in mangrove ecosystems can also vary in space and time, mainly due to varying mangrove root density and type (Cahoon and Lynch, 1997; Lovelock et al., 2015), lateral and longitudinal distances from the main river and creek channel (Neubauer, 2008). Mangrove habitats’ vulnerability to sea-level changes is extremely site-specific (Woodroffe, 2018). The quantification of localized sedimentation rates and monitoring of the same is required to assess the habitat stability of mangroves and their possible responses to sea-level changes (Neubauer, 2008; Adame et al., 2010; McKee, 2011).
To our knowledge, no in-situ data has been collected or studies on vertical accretion or surface elevation change done within the mangroves of Vanga (Kenya). Most studies in and around the mangroves of Vanga have focused more on fisheries, water quality, mangrove structure and socioeconomics (Ochiewo et al., 2010; Kiteresi et al., 2012; Mungai et al., 2019; Fortnam et al., 2020; Kimani et al., 2020). The mangroves of Vanga have suffered a significant loss of cover than other adjacent mangrove areas in Kenya and Tanzania (Mungai et al., 2019) and within the proposed transboundary conservation area (Figure 3). Due to observed losses, Vanga has been designated as a hotspot of mangrove loss, with an average annual loss of 25 ha (Mungai et al., 2019). This change has been attributed to anthropogenic activities, including harvesting and land-use changes. The loss of mangroves has the cascading effect of diminishing the mangrove-derived services including sediment trapping and retention, consequently affecting sediment accretion rates and local subsidence. However, natural factors such as sea-level change and subsequent influence on sediment dynamics have been assessed and considered. Therefore, this study aims to investigate surface and subsurface elevation changes and quantify current elevation change rates in the mangroves of Vanga.
Figure 3 Location of the study area in Vanga, Kenya, showing the experimental plots perpendicular to the main creek channel.
This study was conducted within the mangroves of Vanga Estuary (4° 39’ 18” S and 39° 13’ 20” E), ~100 km south of Mombasa, Kenya (Figure 3). Vanga Estuary is a river-dominated system with a total mangrove cover area of >3,000 ha (Mungai et al., 2019). The dominant mangroves in the estuary are Rhizophora mucronata, Ceriops tagal, Sonneratia alba and Avicennia marina. This mangrove patch is part of the Vanga-Funzi mangrove block (Kiteresi et al., 2012; Mungai et al., 2019) within the proposed transboundary conservation area (TBCA) between Kenya and Tanzania (Figure 3). This region has annual mean precipitation >1000 mm (IUCN, 2003), and the estuary experiences a semi-diurnal tidal influence. It also receives terrigenous sediment and freshwater from the transboundary Umba River year-round (Tesfamariam et al., 2021). It also benefits from the deposition of autochthonous organic sediments derived from in-situ production and the accumulation of biogenic material (leaves and roots).
Surface elevation changes were measured using surface-elevation tables (SET) and horizon markers (MH). SETs are mechanical, portable, or fixed (or a combination of both) levelling set-ups designed to assist in measuring vertical surface elevation changes (Cahoon and Lynch, 1997; Cahoon et al., 2002; Krauss et al., 2010; McKee, 2011; Webb et al., 2013; Callaway et al., 2015). SETs can account for positive (accretion) and negative (shallow subsidence) elevation changes. MH comprise many water-insoluble materials and is easily distinguishable from sediments. They are used as references in sediments against which periodic measurements of accreted sediments can be made (Lang’at et al., 2014). This study used crystal violet (Ammonium oxalate) powder as our horizon marker mainly due to its local availability. In other studies, white feldspar (kaolin) markers have been utilized (Krauss et al., 2003; McKee, 2011; Lang’at et al., 2014).
On the contrary, MH does not account for sub-surface processes but only measures positive elevation changes (accretion). A combination of both allows for determining both surface accretion and subsidence. Due to the dense mangrove, proximity to Vanga village and vulnerability to vandalism, a sophisticated and relatively elaborate SET experimental set-up (Cahoon and Lynch, 1997; Krauss et al., 2010; Callaway et al., 2015) was not feasible in our study site. Therefore, an improvised low-cost and low-maintenance experimental set-up (Figure 4) was used in this study. The SET-MH were set up in 20 × 20 cm plots within the mangrove stands. In two opposite vertices (Figure 4) of the plots, 3m-long stainless-steel rods (6 mm in diameter) were driven to refusal (e.g., bedrock). The rods were sunk below the ground, and the length exposed above the surface was set at 25. All the experimental SET-MH plots were set up perpendicular to the main channel. Some of the plots (A stations) were close (~10-20 m) to the main channel, while others (C stations) were set up farther away (~150-200 m) from the main channel. The distance was to assess and apportion the influence that frequency and depth of tidal inundation had on sediment elevation changes. The assessment of the influence of mangrove species was not feasible because the Vanga-Funzi mangrove is characterized by mixed stands (Mungai et al., 2019). The lack of distinct zonation along the salinity/tidal gradient posed the challenge of inhibiting the setting up of SETs and MHs in specific species zone for quantification of each species or functional root type influence on mangrove surface elevation changes.
Figure 4 (A) Conceptual illustration (not to scale) of the surface-elevation table and horizon marker and (B) the actual field set up (right) within Vanga mangrove forest, Kenya. Elevation changes were measured along the wooden board levelled using a spirit level, and surface accretion changes were measured based on the accreted sediments above the purple crystal violet horizon marker (see panel B).
Twelve (12) height measurements from the mangrove surface to the heights of the rods were made at twelve points along a spirit-levelled wooden board placed diagonally across each set of rods (Figure 4). The 12 measurements were averaged to obtain a single measurement per plot. Subsequent SET measurements were recorded over three years (between 2017 and 2021), taken every 3 to 4 months within the monitoring. However, there were long periods between i) April 2018 and Feb 2019 and ii) July 2019 and August 2020 when elevation measurements were not taken owing to unavoidable project delays. Measurement of accretion above the MH was done by carefully obtaining 2 × 2 cm cores (blocks) on the edges of the plots using a sharp knife (Figure 4). The height (in cm) of sediment above the MH was recorded, and the block returned to its position (Lang’at et al., 2014). Due to the perturbation of some MH by sesarmid crabs, it was impossible to obtain MH cores and measurements.
Sea-level data was obtained from the Mombasa tide gauge – one of the only two tide gauges along the Kenyan coastline with data available for public use. The Mombasa tide gauge (~100 km north of Vanga) was operationalized in 1986. The tide gauge is located at Mwache Creek, Kilindini Harbor. The creek is geomorphologically different from the Vanga estuary. However, sea-level variations along the entire Kenyan coastline are comparable (Kebede et al., 2010). It is currently part of the Global Ocean Observation System (GOOS) network in the tsunami early warning system context. All the data from the GOOS network are stored in the centralized data repository of the GOOS network at the University of Hawaii Sea Level Centre (UHSLC, https://uhslc.soest.hawaii.edu/).
Mean monthly and hourly sea-level data were obtained to assess long-term sea-level and during-study variations. The elevation of the study site with respect to sea level was estimated barometrically using Van Essen© hydrostatic divers. We obtained relative water levels by determining the hydrostatic pressure differences derived from barometric diver measurements and corrected them for atmospheric pressure. A diver (mini DI701 Cera diver) submerged during tidal flooding and measured water pressure. The Cera diver was tied to mangrove roots close to the mangrove surface (approx. 3 cm) to minimize the error when the divers were not submerged. There is also a possibility of underestimating or overestimating water levels above the mangrove surface in turbulent flood tides. However, waves are typically attenuated in dense mangrove vegetation (Furukawa and Wolanski, 1996; Ndirangu et al., 2017). This minimizes the variations due to turbulence. The barometric diver (mini D1801 Baro diver) was placed high enough in mangrove branches to avoid inundation, including spring tide. The D1801 Baro diver was used to measure only the atmospheric pressure. The Baro diver’s readings were considered representative of the study area since atmospheric pressure does not vary over short distances. The two divers were both installed at station 14A (Figure 3). The hydrostatic pressure is obtained by subtracting the measured atmospheric pressure (obtained using the Baro diver) from the measured water pressure (obtained from the Cera diver). This can be transformed into a water column depth (in m) above the mangrove surface. The high-water levels (HWL, in m) measured by the tide gauge in Mombasa were correlated with the calculated water depths at the mangrove sites at high tides. The two daily high-water levels (HWL) referenced to the official chart datum, the lowest astronomical tide (LAT) recorded by the tide gauge, correlated with the corresponding high-water depths measured within the mangroves the divers. Since the highest pressure is recorded during high water levels, the corresponding high diver pressure measurements represent the maximum water depth. The average difference between the corresponding tide gauge measurement (m LAT) and the water depth (m) measurement from the divers gave an estimate of mangrove surface elevation (m LAT) in the study area.
The average elevation measurements from the 21 SETs (Figure 3) were obtained between Dec 2017 and April 2021. Variations in elevation changes were examined between sites considering the distance from the creek and stations for normality and significance differences using the Shapiro-Wilk test and Two-Way ANOVA, respectively.
The sea level has been rising at Mombasa (4° 4’ 12” S and 39° 23’ 47.65” E), ~100 km north of Vanga. Based on the tide-gauge measurements, the MSL has been rising at a rate of 3.8 mm yr-1 (Figure 5), represented by the slope of the linear trend. The sea-level has been referenced to the mean lowest astronomical tide (LAT). The mean LAT derived from the tide gauge data is 0.81 m, the average of all monthly mean low water (MLW). In comparing water depths derived barometrically in the study (in Vanga) with water levels recorded in the Mombasa tide gauge, the estimated mangrove surface elevation in Vanga is 3.22 m above MSL. The sharp drop in sea level between 2000-2003 could be the possible effect of the decadal-scale 18.6-year lunar cycle (Euler effect) which causes either a sharp fall or rise in sea level (Mckinnell and Crawford, 2007; Baart et al., 2012). The 18.6-year lunar nodal cycle is borne of the change in the inclination of the moon’s orbital path to the plane of the Earth’s equator. The lunar orbital oscillation changes range ±5° relative to 23.5° tilt of the equator relative to the ecliptic (Mckinnell and Crawford, 2007) with an effect on the magnitude of the lunar diurnal tide. The change in inclination modulates the tidal amplitudes and currents (Thiébot et al., 2020), with the largest tidal variations experienced during years of maximum lunar orbital declination at 28.5°.
Figure 5 Plot showing a rising (increasing) trend of sea-level in Mombasa derived from tide gauge data between June 1986 and December 2020. From the linear trend, the sea level in Mombasa has been rising at 3.8 mm yr-1.
From the periodic measurement from the installed SET, we observed both accretion and elevation loss within the mangroves of Vanga. In stations near the creek channel (A stations), elevation changes over the three years varied between -80 and 20 mm (Figure 6), while those farther away from the creek channel (C stations) showed variations ranging between -68.5 and 29.3 mm (Figure 7). However, cumulatively, A stations exhibited mean loss of elevation ranging between -77.7 and -16.4 mm over the monitoring period with an average mangrove elevation loss of -45.2 ± 7.2 mm. On the other hand, C stations experienced an average loss of mangrove elevation between -76 and -2.2 mm with an average of -20 ± 7.1 mm.
Figure 6 Mangrove surface elevation changes measure between Dec 2017 and April 2022 close to the main creek channel within the mangroves of Vanga at (A) stations 2A, 5A, 9A, 13A, 14A and 15A and (B) stations 16A, 19A, 20A, 21A and 22A. The top x-axis represents the stations, while the bottom x-axis is the monitoring time stamps. Error bars represent the standard deviation.
Figure 7 Mangrove surface elevation changes rates between Dec 2017 and April 2021 farther away from the main creek channel within the mangroves of Vanga at (A) stations 2C, 5C, 6C, 9C and 10C and (B) stations 13C, 14C, 15C, 19C and 22C. The top x-axis is the stations, while the bottom x-axis is the monitoring time stamps. Error bars represent the standard deviation.
It was evident that mangrove elevation loss was pronounced in stations closer to the main channel than stations farther away. The elevation changes also showed significant variation between sites i.e. A stations and C stations (F-value = 7.87, p = 0.03), however, the variation between the individual stations although discernible in relative terms, was not significant (F-value = 1.43, p = 0.33). Two years (in 2019) after the placement of horizon markers, the cored subsamples in selected un-perturbed MH plots showed average positive sediment accretion above the MH, ranging between 12.3 and 26.0 mm (Table 1).
This translated to an average surface accretion between 6.1 mm yr-1 (station 14A) and 13 mm yr-1 (stations 2C and 5C). The surface accretion in all stations exceeded the current rates of SLR measured at the Mombasa tide gauge (Figure 5). For example, in station 13A, up to 1.5 cm, equivalent to 15 mm of accretion, was recorded (Figure 8). It represented a cumulative accretion of 7.5 mm yr-1, a value higher than the rate of SLR (3.8 mm yr-1) recorded at the Mombasa tide gauge (Figure 5). The average accretion of 18.0 mm, equivalent to 9 mm yr-1 was measured in all the stations. The A stations showed lesser accretion (7.8 mm yr-1) than the C stations (9.6 mm yr-1). The cumulative elevation loss in A stations (-45.2 mm) and C stations (-20 mm) for three years translates to a mangrove surface elevation loss of -15.1 mm yr-1 and -6.6 mm yr-1, respectively. Comparing these elevation changes with the recorded accretion rates of 7.8 and 9.6 mm yr-1, we can deduce a net mangrove surface loss of 7.3 mm yr-1 in the A stations and a net elevation gain of 3.0 mm yr-1 in the C stations.
Figure 8 (A) Image showing the cored sediments with discernible sediment accretion in station 13A and (B) image showing the effects of crab mud overturning and mixing (in some of the plots set up (e.g., in stations 9A, 16A, 22A).
Mangroves promote sediment trapping, accumulation, and consolidation through their dense and complex root structures. Mangroves are also considered ecosystem engineers (Gutiérrez et al., 2012). Through sediment and organic matter retention, mangroves modify the physical environment and colonize new habitats and platforms. This is vital for mangroves in the face of SLR and as they migrate to higher grounds, but this is also important for other flora and fauna. The mangroves of Vanga have exhibited sediment retention evident by the positive (up to 9.0 mm yr-1, Figure 8) accretion above the MH. However, there is also marked shallow subsidence attributable to subsurface compaction, decomposition of buried OM (Gutiérrez et al., 2012; Lang’at et al., 2014) and possible collapse of fiddler sesarmid burrows. This leads to loss of surface elevation (Kristensen, 2007) and poses a considerable challenge for the mangroves of Vanga, especially with the current and predicted rates of SLR. The surface elevation loss within the mangroves of Vanga of up to -45 mm is relatively high. However, it is comparable to Gazi Bay’s (~50 km north of Vanga), which reported up to -51 mm elevation loss (Lang’at et al., 2014). Similar elevation losses have been associated with mangrove die-back and cover loss due to anthropogenic impacts (Krauss et al., 2014; Lang’at et al., 2014). Indeed, anthropogenic-driven loss of mangroves has been reported in Vanga (Mungai et al., 2019). We could, therefore, partly attribute the localized subsidence to the local mangrove loss. During this study, there was a short-lived upstream disruption of Umba River flow and discharge occasioned by road construction. The disruption was between sampling periods and therefore presented no avenue for monitoring or quantification of its influence. Disruption and obstruction of natural river flow can cause a reduction in the sediment load due to the trapping of sediments or redirection (Okuku et al., 2018). Therefore, it inhibits the benefits of sediment supply to the downstream low-lying coastal habitats, including the growth of mangrove surface elevation. In most African regions, it has been reported that the sediments deposited are mainly minerogenic (Balke and Friess, 2016). However, other studies have reported the dominance of organic sediments (roots, leaves) in other tropical regions (Neubauer, 2008; Krauss et al., 2014). Indeed, the mangroves of Vanga receive more terrigenous sediments (Kimeli et al., 2021). We can, therefore, assume that the disruption reduced the supply of minerogenic sediments and led to the increased dominance of the autochthonous organic sedimentation, predominantly root matter and aboveground biomass, including leaf litter (Rogers et al., 2014; Woodroffe et al., 2016). The dominance of organogenic sediment accumulation and enhanced decomposition would have led to pronounced elevation loss.
Accretion has also been reported to be relatively lower in brackish/saline than freshwater (Neubauer, 2008). This broad generalization has been attributed to saline water’s acceleration of OM decomposition. On the contrary, freshwater promotes the accumulation and preservation of OM, consequently translating to increased mangrove surface elevation. Therefore, applying this generalized conclusion, the mangrove surface elevation loss in Vanga could be qualified. However, this would require further assessment and evaluation. Compared to C stations, the relatively high elevation loss in A stations could result from saline waters brought by flood tides reaching these stations during spring and neap tides, accelerating the decomposition, unlike the C stations being inundated only during spring tides. The experimental plots, both A and C stations, were set up in mixed mangrove stands (primarily a mixture of two or more). Different root morphology could also influence elevation changes. For example, it has been shown that prop roots have better capabilities in attenuating flow currents and promoting settling suspended sediments from estuarine waters; however, they are not as successful as pneumatophores in maintaining sediment elevation for longer periods (Krauss et al., 2003). In Vanga, the non-significance in elevation changes between stations would support the findings of (Krauss et al., 2003), that functional root types, root area and root density may have minimal influence on elevation changes. We further postulate that the effect of mixed stands in Vanga would augment the influence of the different species. Although our study did not evaluate sub-surface process including build-up of autochthonous peat layer and growth of fine roots, they are also controlling factors of elevation change in mangroves (Middleton and McKee, 2001; Mckee et al., 2007).
The elevation loss recorded in this study is also within 11.2 to 2.5 mm yr1, documented for similar ecosystems of fringe and riverine mangroves (Krauss et al., 2014). It is projected that GMSL will rise between 4.3 mm (2.9 – 5.9 mm) and 8.4 mm (6.1 – 11 mm) by the end of this century (2100) under the low (RCP 2.6) and high (RCP 8.5) confidence scenarios, respectively (Shukla et al., 2019). Comparing the projected GMSL with elevation changes within the mangroves, Vanga paints a picture of imminent threat, but the threats can be mitigated with informed interventions and adaptations. This threat is also reported for similar habitats, including the projected loss of ~50% of Mngazana, South Africa estuary mangroves with a 3.7 mm yr-1 SLR rate (Yang et al., 2014; Adams and Rajkaran, 2021), the reported loss of >15,000 ha of mangroves due to SLR in Sundarbans (Rahman et al., 2011; Bomer et al., 2020). A regional assessment in Australia, Singapore, Indonesia, Philippines, Malaysia, and Vietnam using a network of comparable SET elevation measurements indicated a ~6 mm yr-1 elevation deficit with respect to current and predicted SLR rates (Alongi, 2008; Woodroffe et al., 2016). However, the fundamental strength of the mangroves in Asia has been reported to be the presence of multiple species of mangroves (Ward et al., 2016; Woodroffe et al., 2016) that increase resilience due to interspecific interactions and organic matter input (Huxham et al., 2010). In the context of long-time scales, mangroves have exhibited resilience and have hitherto adapted to various elevation changes due to paleo sea-level fluctuations (Krauss et al., 2008; Mcivor et al., 2013; Collins et al., 2017; Saintilan et al., 2020) and land elevation changes occasioned by both shallow and tectonically-driven deep subsidence (Collins et al., 2017). It is evident by the observed mangrove colonization and contribution to the net organic carbon budgets identified as far back as the Oligocene (Collins et al., 2017) and more pronounced in the Holocene (McKee, 2011; Mcivor et al., 2013). The inherent resilience of mangroves to sea-level changes, even on open fringe coasts, is borne from the indirect feedback mechanisms derived from the increased tidal ranges, currents, sediment supply and accommodation space (Rogers, 2021). For example, enhanced tidal range and currents increase the reworking of sediments to sizes suitable for mangroves and create numerous tidal channels that increase rugosity suitable for sediment trapping (Collins et al., 2017).
The current and predicted rates of SLR are essential drivers for policymakers, responsible for long-term adaptation measures, conservation, and protection of coastal habitats and human populations. Information derived from this study forms part of the site-specific empirical data that would inform management towards mitigating the effects of the rising SLR. It adds to the growing but still minimal evidence and information of elevation changes within the mangroves of Kenya and the wider Western Indian Ocean region (Okello et al., 2013; Lang’at et al., 2014; Stringer et al., 2015). The information derived from this study will also form part of a suite of baseline data that would inform the setting up and demarcation of a transboundary conservation area (TBCA) between Kenya and Tanzania (Figure 3). Most management and conservation strategies, including restoration, especially for mangroves, have hitherto focused on the ecological aspects but minimally on sediments which is one of the critical pillars for their success. To mitigate the effects of SLR, artificial embankments and interventions to complement nature-based adaptation and resilience have been suggested (Takagi, 2018; Dasgupta et al., 2019). These artificial embankments are intended to increase the efficiency of tidal damping and velocity attenuation and optimize the mangrove functionality of sediment trapping and consolidation. However, some studies have also cautioned on the long-term efficacies of artificial embankments and dykes and other human-induced interventions (Romañach et al., 2018; Zimmer, 2018).
Vanga has been identified as a hotspot for mangrove degradation and loss. It has been mainly attributed to anthropogenic factors. However, the effects of other biophysical factors, including sediment accretion, shallow and deep subsidence and expected SLR, have been minimally assessed. The current study provides data and information on the elevation changes within the mangroves of Vanga. It reveals that the current rates of elevation changes outpace the current rates of SLR. However, it also reveals the vulnerability of Vanga mangroves to submergence and the threat of the projected accelerated SLR and the influence of shallow subsidence. It also gives impetus to the need for a more holistic look at the management and conservation of mangroves by incorporating biophysical drivers like sedimentation within the context of land-use changes, sources, and sinks. Further monitoring is recommended to capture the elevation change pulses that arise from flash floods, river flow disruption and erosion. We also recommend that more multi- and inter-disciplinary research on the Vanga ecosystem considers the sediment sources, freshwater and sediment discharge, and sediment budgets to build a complete picture.
The datasets analysed for this study can be made available by the authors through the corresponding author on reasonable request.
Conception and design: AKK, JAO, JGK, NK. Field experiment and monitoring: AKK, PB, JAO, SC, MM, JGK. Data analyses: AKK, SC, MM, JAO. AKK wrote the whole initial draft of the manuscript, and JAO, NK, HW, JGK provided critical revisions. All authors contributed to the article and approved the submitted version.
Belgian Flemish VLIR-UOS funded this study through TEAM and Southern Initiatives Transcoast project on “Transboundary coastal processes and human resource utilization patterns as a basis for a Kenya–Tanzania conservation area initiative (Trans-Coast)” (Project Code: ZEIN2016PR425) and the Deutscher Akademischer Austauschdienst (DAAD) doctoral programme scholarship awarded to AK (Ref. 91692241) and the Leibniz Centre for Tropical Marine Research (ZMT), Bremen Germany through the ZMT Academy support to AKK.
The authors recognize the technical support offered during fieldwork and monitoring by Joseph Lang’at (now retired) and George Onduso, both of KMFRI, and Harith of Vanga Beach Management Unit, who acted as our field/community guide.
The authors declare that the research was conducted in the absence of any commercial or financial relationships that could be construed as a potential conflict of interest.
All claims expressed in this article are solely those of the authors and do not necessarily represent those of their affiliated organizations, or those of the publisher, the editors and the reviewers. Any product that may be evaluated in this article, or claim that may be made by its manufacturer, is not guaranteed or endorsed by the publisher.
Adame M. F., Neil D., Wright S. F., Lovelock C. E. (2010). Sedimentation within and among mangrove forests along a gradient of geomorphological settings. Estuar. Coast. Shelf Sci. 86, 21–30. doi: 10.1016/j.ecss.2009.10.013
Adams J. B., Rajkaran A. (2021). Changes in mangroves at their southernmost African distribution limit. Estuar. Coast. Shelf Sci. 248, 107158. doi: 10.1016/j.ecss.2020.107158
Alongi D. M. (2008). Mangrove forests: Resilience, protection from tsunamis, and responses to global climate change. Estuar. Coast. Shelf Sci. 76, 1–13. doi: 10.1016/j.ecss.2007.08.024
Baart F., Van Gelder P. H. A. J. M., De Ronde J., Van Koningsveld M., Wouters B. (2012). The effect of the 18.6-year lunar nodal cycle on regional Sea-level rise estimates. J. Coast. Res. 28, 511–516. doi: 10.2112/JCOASTRES-D-11-00169.1
Balke T., Friess D. A. (2016). Geomorphic knowledge for mangrove restoration: A pan-tropical categorization. Earth Surf. Process. Landforms 41, 231–239. doi: 10.1002/esp.3841
Barbier E. (2011). The value of estuarine and coastal ecosystem services. Ecol. Monogr. 81, 169–193.
Bomer E. J., Wilson C. A., Hale R. P., Hossain A. N. M., Rahman (2020). Surface elevation and sedimentation dynamics in the Ganges-Brahmaputra tidal delta plain, Bangladesh: Evidence for mangrove adaptation to human-induced tidal amplification. Catena 187, 104312. doi: 10.1016/j.catena.2019.104312
Bosire J. O., Kaino J. J., Olagoke A. O., Mwihaki L. M., Ogendi G. M., Kairo J. G., et al. (2014). Mangroves in peril: Unprecedented degradation rates of peri-urban mangroves in Kenya. Biogeosciences 11, 2623–2634. doi: 10.5194/bg-11-2623-2014
Cahoon D., Lynch J. (1997). Vertical accretion and shallow subsidence in a mangrove forest of southwestern Florida, U.S.A. Mangroves Salt Marshes 1, 173–186. doi: 10.1023/A:1009904816246
Cahoon D. R., Lynch J. C., Hensel P., Boumans R., Perez B. C., Segura B., et al. (2002). High-precision measurements of wetland sediment elevation: I. recent improvements to the sedimentation-erosion table. J. Sediment. Res. 72, 730–733. doi: 10.1306/020702720730
Callaway J. C., Cahoon D. R., Lynch J. C. (2015). The surface elevation table-marker horizon method for measuring wetland accretion and elevation dynamics in Methods in biogeochemistry of wetlands (Madison, WI: Soil Science Society of America), 901–917. doi: 10.2136/sssabookser10.c46
Church J. A., White N. J. (2011). Sea-Level rise from the late 19th to the early 21st century. Surv. Geophys. 32, 585–602. doi: 10.1007/s10712-011-9119-1
Collins D. S., Avdis A., Allison P. A., Johnson H. D., Hill J., Piggott M. D., et al. (2017). Tidal dynamics and mangrove carbon sequestration during the oligo-Miocene in the south China Sea. Nat. Commun. 8, 1–12. doi: 10.1038/ncomms15698
Crosby S. C., Sax D. F., Palmer M. E., Booth H. S., Deegan L. A., Bertness M. D., et al. (2016). Salt marsh persistence is threatened by predicted sea-level rise. Estuar. Coast. Shelf Sci. 181, 93–99. doi: 10.1016/j.ecss.2016.08.018
Dahdouh-Guebas F., Jayatissa L. P., Di Nitto D., Bosire J. O., Lo Seen D., Koedam N. (2005). How effective were mangroves as a defence against the recent tsunami? Curr. Biol. 15, R443–R447. doi: 10.1016/j.cub.2005.06.008
Dangendorf S., Hay C., Calafat F. M., Marcos M., Piecuch C. G., Berk K., et al. (2019). Persistent acceleration in global sea-level rise since the 1960s. Nat. Clim. Change 9, 705–710. doi: 10.1038/s41558-019-0531-8
Dasgupta S., Islam M. S., Huq M., Huque Khan Z., Hasib M. R. (2019). Quantifying the protective capacity of mangroves from storm surges in coastal Bangladesh. PloS One 14, e0214079. doi: 10.1371/journal.pone.0214079
Di Nitto D., Neukermans G., Koedam N., Defever H., Pattyn F., Kairo J. G., et al. (2014). Mangroves facing climate change: Landward migration potential in response to projected scenarios of sea level rise. Biogeosciences 11, 857–871. doi: 10.5194/bg-11-857-2014
Ellison J. C. (2015). Vulnerability assessment of mangroves to climate change and sea-level rise impacts. Wetl. Ecol. Manage. 23, 115–137. doi: 10.1007/s11273-014-9397-8
Fagherazzi S., Bryan K. R. (2017). Buried alive or washed away: The challenging life of mangroves in the Mekong delta. Nardin Source Oceanogr. 30, 48–59. doi: 10.2307/26201897
Fortnam M., Atkins M., Brown K., Chaigneau T., Frouws A., Gwaro K., et al Multiple impact pathways of the 2015–2016 El Niño in coastal Kenya. Ambio 50, 174–179. doi: 10.1007/s13280-020-01321-z
Friess D. A., McKee K. L. (2021). “The history of surface-elevation paradigms in mangrove biogeomorphology,” in Dynamic sedimentary environments of mangrove coasts (Amsterdam, The Netherlands: Elsevier), 179–198. doi: 10.1016/b978-0-12-816437-2.00007-0
Friess D. A., Rogers K., Lovelock C. E., Krauss K. W., Hamilton S. E., Lee S. Y., et al. (2019). The state of the world’s mangrove forests: Past, present, and future. Annu. Rev. Environ. Resour. 44, 89–115.
Furukawa K., Wolanski E. (1996). Sedimentation in mangrove forests. Mangroves Salt Marshes 1, 3–10. doi: 10.1023/A:1025973426404
Gilman E. L., Ellison J., Duke N. C., Field C. (2008). Threats to mangroves from climate change and adaptation options: A review. Aquat. Bot. 89, 237–250. doi: 10.1016/j.aquabot.2007.12.009
Gutiérrez J. L., Jones C. G., Byers J. E., Arkema K. K., Berkenbusch K., Commito A., et al. (2012). “Physical ecosystem engineers and the functioning of estuaries and coasts,” Wolanski E, McLusky DS (eds.) Treatise on Estuarine and Coastal Science (Netherlands: Elsevier Inc) 53–81. doi: 10.1016/B978-0-12-374711-2.00705-1
Hamza A. J., Esteves L. S., Cvitanovic M., Kairo J. (2020). Past and present utilization of mangrove resources in Eastern Africa and drivers of change. J. Coast. Res. 95, 39–44. doi: 10.2112/SI95-008.1
Huxham M., Kumara M. P., Jayatissa L. P., Krauss K. W., Kairo J., Langat J., et al. (2010). Intra- and interspecific facilitation in mangroves may increase resilience to climate change threats. Philos. Trans. R. Soc B Biol. Sci. 365, 2127–2135. doi: 10.1098/rstb.2010.0094
Igulu M. M., Nagelkerken I., Dorenbosch M., Grol M. G. G., Harborne A. R., Kimirei I. A., et al. (2014). Mangrove habitat use by juvenile reef fish: Meta-analysis reveals that tidal regime matters more than biogeographic region. PLoS One 9, e114715. doi: 10.1371/journal.pone.0114715
IUCN (2003). Eastern Africa Programme 2003: The pangani basin: A situation analysis Nairobi, Kenya: IUCN-EARO Publications Unit. xvi + 104.
Kebede A. S., Nicholls R. J., Hanson S., Mokrech M. (2010). Impacts of climate change and sea-level rise: A preliminary case study of Mombasa, Kenya. J. Coast. Res. 28, 8–19. doi: 10.2112/JCOASTRES-D-10-00069.1
Kimani P., Wamukota A., Manyala J. O., Mlewa C. M. (2020). Actors’ perceptions of government performance in support of value chain development in marine small-scale fisheries in Kenya. Mar. Policy 122, 104221. doi: 10.1016/j.marpol.2020.104221
Kimeli A., Cherono S., Mutisya B., Tamooh F., Okello J., Westphal H., et al. (2021). Tracing organic matter sources in the estuarine sediments of vanga, Kenya, and provenance implications. Estuar. Coast. Shelf Sci. 263, 107636. doi: 10.1016/j.ecss.2021.107636
Kirwan M. L., Langley J. A., Guntenspergen G. R., Megonigal J. P. (2013). The impact of sea-level rise on organic matter decay rates in Chesapeake bay brackish tidal marshes. Biogeosciences 10, 1869–1876. doi: 10.5194/bg-10-1869-2013
Kiteresi L. I., Okuku E. O., Mwangi S. N., Ohowa B., Wanjeri V. O., Okumu S., et al. (2012). The influence of land based activities on the phytoplankton communities of shimoni-vanga system, Kenya. Int. J. Environ. Res. 6, 151–162. doi: 10.22059/ijer.2011.482
Kitheka J. U., Ongwenyi G. S., Mavuti K. M. (2002). Dynamics of suspended sediment exchange and transport in a degraded mangrove creek in Kenya. Ambio 31, 580–587. doi: 10.1579/0044-7447-31.7.580
Krauss K. W., Allen J. A., Cahoon D. R. (2003). Differential rates of vertical accretion and elevation change among aerial root types in Micronesian mangrove forests. Estuar. Coast. Shelf Sci. 56, 251–259. doi: 10.1016/S0272-7714(02)00184-1
Krauss K. W., Cahoon D. R., Allen J. A., Ewel K. C., Lynch J. C., Cormier N. (2010). Surface elevation change and susceptibility of different mangrove zones to sea-level rise on pacific high islands of Micronesia. Ecosystems 13, 129–143. doi: 10.1007/s10021-009-9307-8
Krauss K. W., Lovelock C. E., McKee K. L., López-Hoffman L., Ewe S. M. L., Sousa W. P. (2008). Environmental drivers in mangrove establishment and early development: A review. Aquat. Bot. 89, 105–127. doi: 10.1016/j.aquabot.2007.12.014
Krauss K. W., Mckee K. L., Lovelock C. E., Cahoon D. R., Saintilan N., Reef R., et al. (2014). How mangrove forests adjust to rising sea level. New Phytol. 202, 19–34. doi: 10.1111/nph.12605
Kristensen E. (2007). Mangrove crabs as ecosystem engineers; with emphasis on sediment processes. Journal of Sea Research 59, 30–43. doi: 10.1016/j.seares.2007.05.004
Kusumaningtyas M. A., Hutahaean A. A., Fischer H. W., Pérez-Mayo M., Ransby D., Jennerjahn T. C. (2019). Variability in the organic carbon stocks, sources, and accumulation rates of Indonesian mangrove ecosystems. Estuar. Coast. Shelf Sci. 218, 310–323. doi: 10.1016/j.ecss.2018.12.007
Lang’at J. K. S., Kairo J. G., Mencuccini M., Bouillon S., Skov M. W., Waldron S., et al. (2014). Rapid losses of surface elevation following tree girdling and cutting in tropical mangroves. PloS One 9, 1–8. doi: 10.1371/journal.pone.0107868
Lovelock C., Cahoon D., Friess D., Guntenspergen G., Krauss K., Ruth R., et al. (2015). The vulnerability of indo-pacific mangrove forests to sea-level rise. Nature 426, 559–563. doi: 10.1038/nature15538
Mazda Y., Wolanski E. (2009). Hydrodynamics and modeling of water flow in mangrove areas (Amsterdam, The Netherlands: Elsevier). doi: 10.1016/B978-0-444-53103-2.00008-9
Mcivor A., Spencer T., Möller I., Spalding M. D. (2013). The response of mangrove soil surface elevation to sea level rise natural coastal protection series: Report 3. Cambridge Coastal Research Unit Working Paper 42 The Nature Conservancy and Wetlands International, 59. Available at: http://coastalresilience.org/science/mangroves/surface-elevation-and-sea-level-rise
McKee K. L. (2011). Biophysical controls on accretion and elevation change in Caribbean mangrove ecosystems. Estuar. Coast. Shelf Sci. 91, 475–483. doi: 10.1016/j.ecss.2010.05.001
Mckee K. L., Cahoon D. R., Feller I. C. (2007). Caribbean Mangroves adjust to rising sea level through biotic controls on change in soil elevation. Glob. Ecol. Biogeogr. 16, 545–556. doi: 10.1111/j.1466-8238.2007.00317.x
Mckinnell S. M., Crawford W. R. (2007). The 18.6-year lunar nodal cycle and surface temperature variability in the northeast pacific. J. Geophys. Res. Ocean. 112, 2002. doi: 10.1029/2006JC003671
Middleton B., McKee K. (2001). Degradadon of mangrove fissues and implications for peat formation in belizean island forests. J. Ecol. 88, 818–828.
Mungai F., Kairo J., Mironga J., Kirui B., Mangora M., Koedam N. (2019). Mangrove cover and cover change analysis in the transboundary area of Kenya and Tanzania during 1986–2016. J. Indian Ocean Reg. 15, 157–176. doi: 10.1080/19480881.2019.1613868
Nardin W., Vona I., Fagherazzi S. (2021). Sediment deposition affects mangrove forests in the Mekong delta, Vietnam. Cont. Shelf Res. 213, 104319. doi: 10.1016/j.csr.2020.104319
Ndirangu M. D., Chira R. M., Wang’ondu V., Kairo J. G. (2017). Analysis of wave energy reduction and sediment stabilization by mangroves in gazi bay, Kenya. Bonorowo Wetl 7, 83–94. doi: 10.13057/bonorowo/w070205
Nerem R. S., Beckley B. D., Fasullo J. T., Hamlington B. D., Masters D., Mitchum G. T. (2018). Climate-change–driven accelerated sea-level rise detected in the altimeter era. Proc. Natl. Acad. Sci. U. S. A. 115, 2022–2025. doi: 10.1073/pnas.1717312115
Neubauer S. C. (2008). Contributions of mineral and organic components to tidal freshwater marsh accretion. Estuar. Coast. Shelf Sci. 78, 78–88. doi: 10.1016/j.ecss.2007.11.011
Ochiewo J., de la Torre-Castro M., Muthama C., Munyi F., Nthuta J. M. (2010). Socio-economic features of sea cucumber fisheries in southern coast of Kenya. Ocean Coast. Manage. 53, 192–202. doi: 10.1016/j.ocecoaman.2010.01.010
Okello J. A., Kairo J. G., Dahdouh-Guebas F., Beeckman H., Koedam N. (2020). Mangrove trees survive partial sediment burial by developing new roots and adapting their root, branch and stem anatomy. Trees - Struct. Funct. 34, 37–49. doi: 10.1007/s00468-019-01895-6
Okello J. O., Schmitz N., Kairo J. G., Beeckman H., Dahdouh-Guebas F., Koedam N. (2013). Self-sustenance potential of peri-urban mangroves: A case of mtwapa creek Kenya. J. Environ. Sci. Water Resour. 2, 277–289.
Okuku E. O., Tole M., Bouillon S. (2018). Role of a cascade of reservoirs in regulating downstream transport of sediment, carbon and nutrients: Case study of tropical arid climate tana river basin. Lakes Reserv. Res. Manage. 23, 43–55. doi: 10.1111/lre.12206
Phan L. K., Van Thiel De Vries J. S. M., Stive M. J. F. (2015). Coastal mangrove squeeze in the Mekong delta. J. Coast. Res. 31, 233–243. doi: 10.2112/JCOASTRES-D-14-00049.1
Polidoro B. A., Carpenter K. E., Collins L., Duke N. C., Ellison A. M., Ellison J. C., et al. (2010). The loss of species: Mangrove extinction risk and geographic areas of global concern. PloS One 5 (4), e10095. doi: 10.1371/journal.pone.0010095
Rahman A. F., Dragoni D., El-Masri B. (2011). Response of the sundarbans coastline to sea level rise and decreased sediment flow: A remote sensing assessment. Remote Sens. Environ. 115, 3121–3128. doi: 10.1016/j.rse.2011.06.019
Rogers K. (2021). Accommodation space as a framework for assessing the response of mangroves to relative sea-level rise. Singap. J. Trop. Geogr. 42, 163–183. doi: 10.1111/SJTG.12357
Rogers K., Saintilan N., Woodroffe C. D. (2014). Surface elevation change and vegetation distribution dynamics in a subtropical coastal wetland: Implications for coastal wetland response to climate change. Estuar. Coast. Shelf Sci. 149, 46–56. doi: 10.1016/j.ecss.2014.07.009
Romañach S. S., DeAngelis D. L., Koh H. L., Li Y., Teh S. Y., Raja Barizan R. S., et al. (2018). Conservation and restoration of mangroves: Global status, perspectives, and prognosis. Ocean Coast. Manage. 154, 72–82. doi: 10.1016/j.ocecoaman.2018.01.009
Saintilan N., Khan N. S., Ashe E., Kelleway J. J., Rogers K., Woodroffe C. D., et al. (2020). Thresholds of mangrove survival under rapid sea level rise. Science 368, 1118–1121. doi: 10.1126/science.aba2656
Sanders C. J., Smoak J. M., Naidu A. S., Patchineelam S. R. (2008). Recent sediment accumulation in a mangrove forest and its relevance to local Sea-level rise (Ilha grande, Brazil). J. Coast. Res. 242, 533–536. doi: 10.2112/07-0872.1
Soares M. L. G. (2009). A conceptual model for the responses of mangrove forests to sea level rise. J. Coast. Res. SI 56 (Proceedings of the 10th International Coastal Symposium), 267–271. Lisbon, Portugal
Shukla P. R., Skea J., Buendia E.C., Masson-Delmot V. (2019). Climate change and land: an IPCC special report on climate change, desertification, land degradation, sustainable land management, food security, and greenhouse gas fluxes in terrestrial ecosystems Cambridge: Cambridge University Press Cambridge.
Stringer C. E., Trettin C. C., Zarnoch S. J., Tang W. (2015). Carbon stocks of mangroves within the Zambezi river delta, Mozambique. For. Ecol. Manage. 354, 139–148. doi: 10.1016/j.foreco.2015.06.027
Sugden A. M. (2020). Mangroves under sea level rise. Science 368, 1078B. doi: 10.1126/SCIENCE.368.6495.1076-P
Takagi H. (2018). Long-term design of mangrove landfills as an effective tide attenuator under relative Sea-level rise. Sustainability 10, 1045. doi: 10.3390/su10041045
Tesfamariam E., Home P., Gathenya J. (2021). Rainfall-runoff modelling to determine continuous time series of daily streamflow in the umba river, Kenya. Afr. J. Rural Dev. 5, 49–68.
Thiébot J., Guillou S., Droniou E. (2020). Influence of the 18.6-year lunar nodal cycle on the tidal resource of the alderney race, France. Appl. Ocean Res. 97, 102107. doi: 10.1016/J.APOR.2020.102107
Ward R. D., Friess D. A., Day R. H., Mackenzie R. A. (2016). Impacts of climate change on mangrove ecosystems: a region by region overview. Ecosyst. Heal. Sustain. 2, e01211. doi: 10.1002/ehs2.1211
Webb E. L., Friess D. A., Krauss K. W., Cahoon D. R., Guntenspergen G. R., Phelps J. (2013). A global standard for monitoring coastal wetland vulnerability to accelerated sea-level rise. Nat. Clim. Change 3, 458–465. doi: 10.1038/nclimate1756
Woodroffe C. D. (2018). Mangrove response to sea level rise: Palaeoecological insights from macrotidal systems in northern Australia. Mar. Freshw. Res. 69, 917–932.
Woodroffe C. D., Rogers K., McKee K. L., Lovelock C. E., Mendelssohn I. A., Saintilan N. (2016). Mangrove sedimentation and response to relative Sea-level rise. Ann. Rev. Mar. Sci. 8, 243–266. doi: 10.1146/annurev-marine-122414-034025
Yang S. C., Riddin T., Adams J. B., Shih S. S. (2014). Predicting the spatial distribution of mangroves in a south African estuary in response to sea level rise, substrate elevation change and a sea storm event. J. Coast. Conserv. 18, 459–469. doi: 10.1007/s11852-014-0331-2
Keywords: sediments, accretion, mangroves, Vanga, Kenya, sea-level rise, elevation, shallow subsidence
Citation: Kimeli AK, Cherono S, Baya P, Mathinji M, Okello JA, Koedam N, Westphal H and Kairo JG (2022) Surface elevation changes in an estuarine mangrove forest in Vanga, Kenya: Implications for management and mitigation of sea-level rise. Front. Mar. Sci. 9:932963. doi: 10.3389/fmars.2022.932963
Received: 30 April 2022; Accepted: 03 October 2022;
Published: 02 November 2022.
Edited by:
Pierre Failler, University of Portsmouth, United KingdomReviewed by:
Henry Bokuniewicz, The State University of New York (SUNY), United StatesCopyright © 2022 Kimeli, Cherono, Baya, Mathinji, Okello, Koedam, Westphal and Kairo. This is an open-access article distributed under the terms of the Creative Commons Attribution License (CC BY). The use, distribution or reproduction in other forums is permitted, provided the original author(s) and the copyright owner(s) are credited and that the original publication in this journal is cited, in accordance with accepted academic practice. No use, distribution or reproduction is permitted which does not comply with these terms.
*Correspondence: Amon Kibiwot Kimeli, YWtpbWVsaUBrbWZyaS5nby5rZQ==
Disclaimer: All claims expressed in this article are solely those of the authors and do not necessarily represent those of their affiliated organizations, or those of the publisher, the editors and the reviewers. Any product that may be evaluated in this article or claim that may be made by its manufacturer is not guaranteed or endorsed by the publisher.
Research integrity at Frontiers
Learn more about the work of our research integrity team to safeguard the quality of each article we publish.