- 1Conservation Biology Division, Northwest Fisheries Science Center, National Marine Fisheries Service, National Oceanic and Atmospheric Administration, Seattle, WA, United States
- 2Pacific States Marine Fisheries Commission, Seattle, WA, United States
- 3Frank Orth and Associates, Seattle, WA, United States
- 4School of Marine and Environmental Affairs, University of Washington, Seattle, WA, United States
- 5National Center for Ecological Analysis and Synthesis, Santa Barbara, CA, United States
- 6Department of Ecology, Evolution, and Marine Biology, University of California, Santa Barbara, Santa Barbara, CA, United States
- 7School of Environmental and Forest Sciences, University of Washington, Seattle, WA, United States
- 8The Nature Conservancy of Washington, Seattle, WA, United States
A huge proportion of the world’s population resides in urban areas along the coast. As cities expand, the ability of coastal ecosystems to provide the benefits people derive from nature, ranging from food from fisheries to coastal defense to maritime transportation and beyond, is in question. While it is well understood that coastal development changes ecosystems, quantitative insights about how terrestrial urbanization fundamentally alters ecosystem structure and function in adjacent freshwater and downstream coastal marine habitats remain rare, though a general expectation is that impacts of terrestrial urbanization will attenuate from land to freshwater to coastal marine habitats. Empirical assessments of these phenomena are especially important for species that rely on freshwater and coastal marine habitats at multiple points in their life cycles, including endangered and threatened Pacific salmon (Oncorhynchus spp.). We investigated associations between landscape-scale urbanization and ecosystem structure (biodiversity of epibenthic invertebrate taxa) and function (benthic net primary productivity and decomposition) in freshwater and coastal marine habitats across six pairs of more and less urbanized, coastal watersheds in Puget Sound, WA, USA, using principal components analysis, analysis of covariance, and Mantel tests. Greater upland urbanization was associated with greater reductions in freshwater biodiversity, measured as the density and evenness of epibenthic invertebrate families. In contrast and surprisingly, however, coastal marine biodiversity (measured as the density and evenness of epibenthic invertebrate families) tended to be higher at more urbanized sites, suggesting the potential role of low to moderate levels of urbanization-related disturbance in determining coastal marine biodiversity patterns. We found no statistical association between urbanization and freshwater and coastal marine ecosystem functions, estimated from changes in accumulated algal biomass on tiles (benthic net primary productivity) and loss of biomass from litter bags (decomposition). In addition, there was no evidence that changes in ecosystem structure and function with urbanization were more severe in freshwater than coastal marine habitats, as might be expected if the land-sea boundary diminished effects of landscape-scale urbanization. Our results suggest that the effects of urbanization can be complex and that attention to terrestrial, freshwater, and coastal marine systems in concert will produce more effective, ecosystem-based management.
1 Introduction
Despite comprising ~2% of the global land surface, urban areas are home to more than half of the world’s human population (United Nations (UN), 2014). In the US, 30-50% of people live in coastal counties, primarily in cities (Crossett et al., 2013). Coastal cities concentrate many social benefits for people because they serve as cultural hubs and centers of commerce and trade (Ernstson et al., 2010), and there is increasing appreciation that they are also places that nature’s benefits are most needed (Granek et al., 2010; Lepczyk et al., 2017; Keeler et al., 2019). However, urban expansion draws down natural resources, consumes undeveloped and agricultural lands, and influences biodiversity and ecosystem functions (Bulleri and Chapman, 2010; Secretariat of the Convention on Biological Diversity (SCBD), 2012; Todd et al., 2019). While it is well understood that coastal development changes ecosystems, a quantitative understanding of the relationship between landscape-scale development, ecosystem structure, and ecosystem function relative to other local, regional, and global influences is generally lacking (Halpern et al., 2009; Ãlvarez-Romero et al., 2011; but see McClelland et al., 1997; Koch et al., 2009).
The literature is replete with hypotheses about processes associated with urban land development that modify ecosystem structure and function (Table 1). These processes include increases in species introductions, nutrient inputs, stream flashiness, sediment runoff, water temperature, low-oxygen areas, toxic contaminants, and extractive use. Such changes can interact to affect other ecosystem properties, such as when eutrophication creates low-oxygen zones that reduce biodiversity (Stramma et al., 2010). It is common to examine impacts of urbanization on biodiversity using indicator taxa, and invertebrate communities are frequently used for such assessments because they are tractable for monitoring purposes and sensitive to environmental changes in predictable ways (Alberti et al., 2007; Clark et al., 2015). Ecologists evaluate a range of important ecosystem functions, including but not limited to primary and secondary production, transformation of organic matter (e.g., via decomposition), and nutrient cycling (Strong et al., 2015). A key factor in deciding which metrics of biodiversity and ecosystem function to monitor and track is to link them to outcomes of interest, including those associated with conservation or restoration actions.
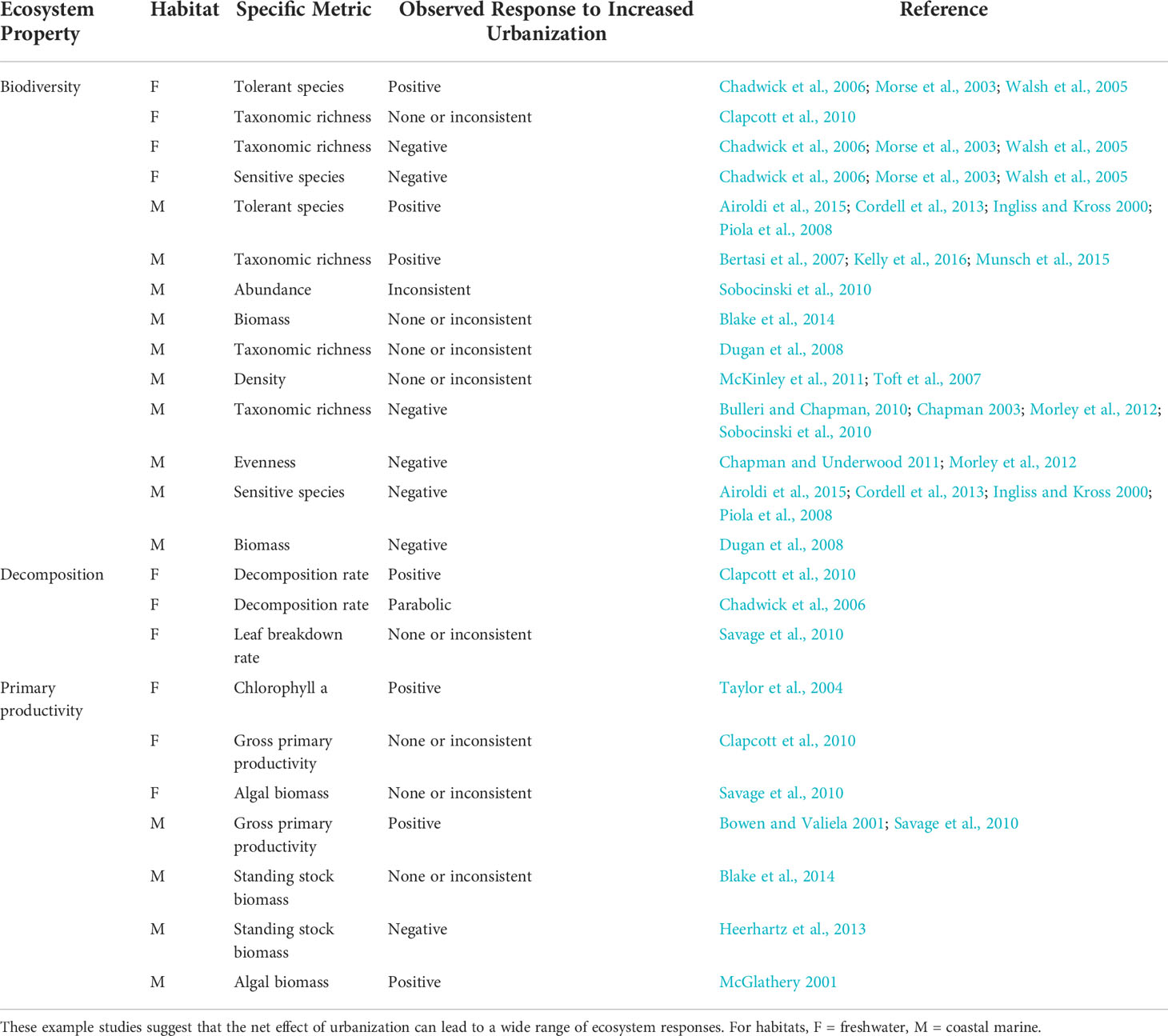
Table 1 Examples of how urbanization, defined here as greater imperviousness and coastal armoring, can modify environmental processes, along with expected relationships between a variety of ecosystem properties.
Often hypotheses about changes in ecosystem structure and function imply that the effects of development should be strongest on land, and attenuate but not necessarily extinguish in coastal marine ecosystems (Beger et al., 2010; Ãlvarez-Romero et al., 2011; Buckner et al., 2018). The rationale for this predicted attenuation is that many of the landscape-scale impacts occur directly in terrestrial habitats (e.g., conversion of natural to impervious surfaces) and that the indirect effects in adjacent freshwater and downstream coastal marine habitats should be comparatively diminished. In some cases, it is clear that the influence of terrestrial inputs, such as dissolved organic carbon, are diminished in freshwater and coastal marine systems, but such findings remain a contentious area of investigation (Brett et al., 2009). Quantitative evidence for specific urban impacts on ecosystem structure and function, such as biotic homogenization and loss of foundation species, is beginning to emerge (Cuffney et al., 2010; Kelly et al., 2016; Lee et al., 2018; Todd et al., 2019; Momota and Hosokawa, 2021). However, few published studies consider the extent to which urbanization effects are linked across land-sea boundaries, despite (a) the potential for direct changes to terrestrial and freshwater habitats to have more marginal impacts within coastal marine habitats, and (b) the clear importance of resolving these connectivity issues for ecosystem management (Stoms et al., 2005; Alberti et al., 2007; Tallis et al., 2008; Beger et al., 2010; Buckner et al., 2018).
Species that move between freshwater and coastal marine habitats may be especially sensitive to changes in biodiversity and ecosystem function caused by urbanization, as they can experience compounding effects across habitats (Gosselin et al., 2018; Friedman et al., 2019). One prominent example comes from Pacific salmon (Oncorhynchus spp.), anadromous species that coexist with humans in heavily populated urban regions as well as in remote and unpopulated areas. Urbanization has been shown to increase toxic runoff and cause geomorphological alterations that affect salmon throughout their life cycle. Changes in biodiversity of salmon prey, along with modifications to ecosystem functions related to the flux of energy, have been linked to salmon productivity and survival (Morley and Karr, 2002; Feist et al., 2017). The biodiversity of invertebrate prey items for juvenile salmon, in both freshwater and coastal marine habitats, is particularly important to early marine growth and survival (Wipfli and Baxter, 2010; Kennedy et al., 2018; Davis et al., 2020), and can be affected by the presence of biogenic habitats like eelgrass and land use change associated with urban development (Francis and Schindler, 2009; Sobocinski et al., 2010; Dethier et al., 2016). Despite concerted efforts in recent years to restore coastal marine habitats for salmon (Munsch et al., 2015; Bilkovic et al., 2016; Munsch et al., 2017), integrative work to consider coupled changes to the structure and function of freshwater and coastal marine habitats, which are physically connected and ecologically linked by the waters, chemicals, and species that move between them, remain relatively rare. While recent research considers changes in land cover across connected freshwater and coastal marine habitats (Bartz et al., 2015), few studies actually estimate changes in freshwater biodiversity of salmon prey or ecosystem functions (such as shifts in riverine flows and metabolism) in relation to analogous biodiversity and ecosystem functions in connected coastal marine habitats. Furthermore, there is much less research available to inform an understanding of how upland and coastal urbanization corresponds to changes in ecosystem structure and function within coastal marine habitats. This information is essential for building a grounded understanding of the ecological changes that have led to the threatened and endangered status of many salmon populations in the US (https://www.fisheries.noaa.gov/species-directory/threatened-endangered) and Canada (https://www.canada.ca/en/environment-climate-change/services/species-risk-public-registry.html), and can allow for more effective outcomes for restoration efforts intended to aid salmon recovery.
The coastal region of Puget Sound, WA, USA, is critical habitat for many populations of Pacific salmon and an excellent test bed for understanding how ecosystem properties that salmon rely upon change along an urban gradient and across the land-sea interface. The Puget Sound is within the larger Salish Sea ecosystem and includes the lands and waters from the crests of the Cascade and Olympic mountains to the coastal marine waters as far south as Olympia, WA, north to the San Juan Islands and west to the mouth of the Strait of Juan de Fuca (Ruckelshaus and McClure, 2007; Figure 1). It is characterized by a full range of coastal land cover, from undeveloped to intensely developed, including uninhabited wilderness areas, three national parks, and densely populated cities (0-232 people km-2, including the metropolitan hubs of Seattle and Tacoma). The population in Puget Sound has increased by more than 1 million since 2010, has more than doubled in the past half century (Manson et al., 2021), and nearly 4 million people live within 20km of the Puget Sound coastline (Kelly et al., 2016), placing enormous anthropogenic pressure on this fjordal ecosystem. Understanding how urbanization affects the biophysical ecosystem in this region is a priority because the 35,000 km2 Puget Sound basin contains thousands of watersheds, many of which include perennial lowland streams and rivers that are habitat for Pacific salmon, including some species listed as threatened or endangered under the U.S. Endangered Species Act (Puget Sound chinook salmon, O. tshawytscha; Hood Canal summer-run chum salmon, O. keta; 16 U.S.C. §§ 1531–1544).
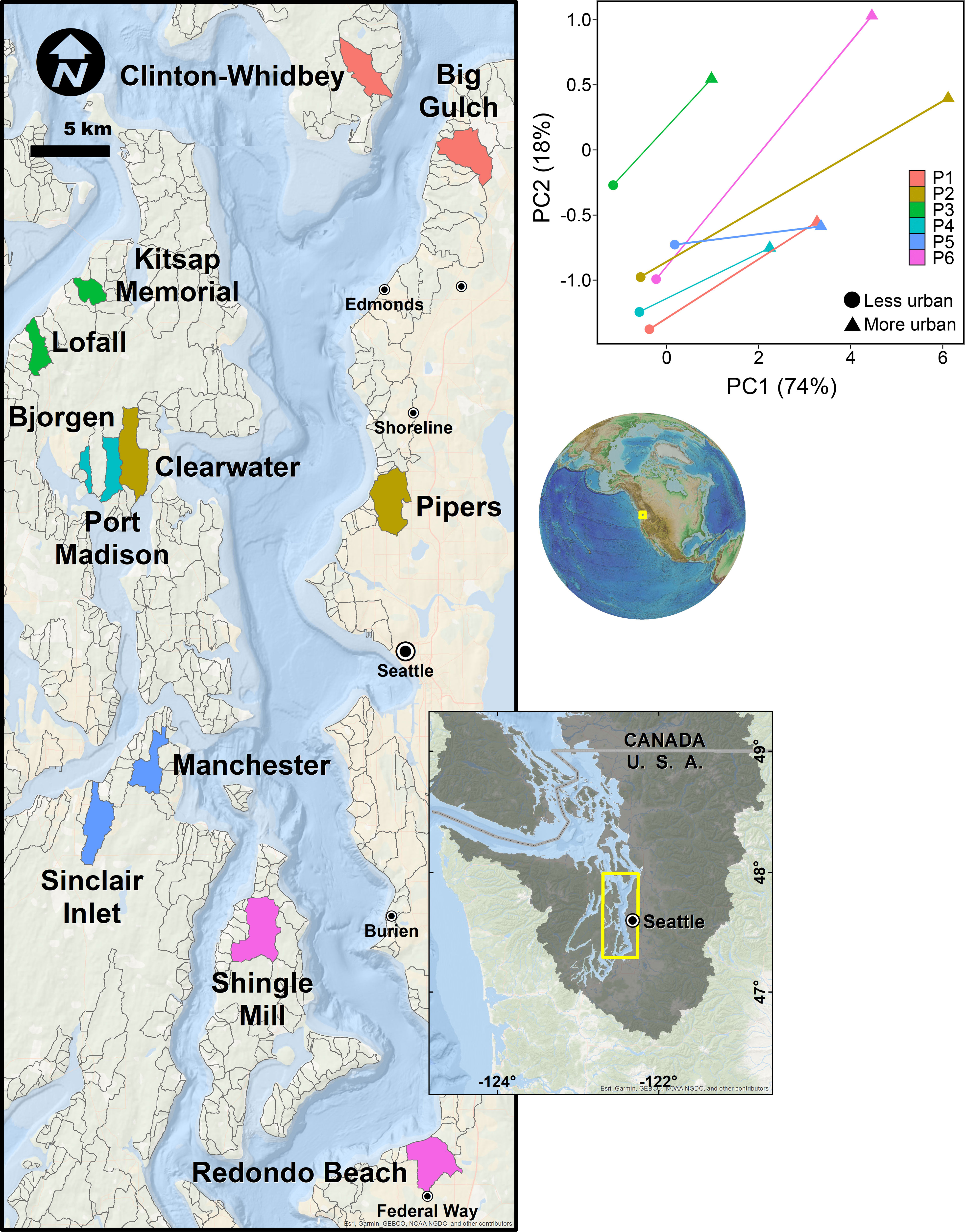
Figure 1 Map of watersheds used for this study (colored polygons), contextualized relative to other candidate watersheds considered (gray outlines), along with a principal components analysis of watershed pairs used to define the urban gradient. Larger values of PC1 and PC2 indicate increasing urbanization.
Here we studied how changes in the biodiversity of salmon prey communities (epibenthic invertebrates), along with variability in net primary productivity and decomposition (two of many ecosystem functions that underpin energy fluxes in salmon habitats), relate to landscape-scale urbanization in the Puget Sound region. By developing an index of urbanization based on land cover data, we were able to choose study sites falling along a gradient from heavily impacted to largely unmodified freshwater and coastal marine habitats. We conducted analyses to determine if (i) increased urbanization lowered biodiversity and reduced ecosystem function, and (ii) the effects of urbanization were stronger in freshwater habitats than in coastal marine habitats. This research adds to a growing body of literature on the effects of cities on ecosystems, and bolsters understanding of how ecosystem properties critical to salmon populations vary with urbanization in freshwater and coastal marine habitats.
2 Materials and methods
2.1 Defining the urban gradient
We quantified watershed scale patterns of urbanization in streams that flowed directly into Puget Sound using ESRI ArcGIS software suite (v.10.1). We used an existing river basin geospatial data layer (PSNERP, 2010) to select watersheds that were ≤1,000 ha, and contained ephemeral and perennial streams (U.S. Environmental Protection Agency & U.S. Geological Survey, 2005). We quantified the degree of urbanization in watersheds that met these criteria, by spatially overlaying them with four existing and spatially comprehensive geospatial data layers. These publicly available data layers capture various aspects of urbanization: imperviousness (i.e., surface areas that reduce infiltration and increase runoff; Schueler et al., 2009), length of roadways (OpenStreetMap contributors, 2012), land use and land cover type (LULC, Fry et al. 2011), and length of shoreline armoring (PSNERP, 2010). For the data layers with a continuous attribute (imperviousness, 0 - 100%, and roadway, density per km2) we calculated area weighted mean values for each watershed. For the data layers with categorical attributes (LULC, shoreline armoring), we calculated the proportion of each watershed that was classified as developed as well as the proportion of the associated shoreline that was armored. Note that we included the extent of shoreline armoring as part of the development of our urbanization index because of the profound impacts this kind of habitat modification can have in coastal marine habitats (Dethier et al., 2016).
Following Alberti et al. (2007) and Spirandelli (2014), we characterized the rural-to-urban gradient by reducing the dimensionality of the four land cover data layers using principal components analysis (PCA) in Primer v6. Prior to the PCA, we normalized the data layers so each had a mean of zero and unit variance to ensure that they were equally influential in describing the urban gradient. The first two principal components (PC1 and PC2) explained 92% of the among-watershed variation in the original four LULC data layers (Table S1; Figure 1). Increasingly positive values of PC1 (74%) corresponded to more human-modified watersheds (specifically, those with greater imperviousness, road, and developed land cover); we refer to PC1 as the ‘upland urbanization index’. Increasingly positive values of PC2 (18%) corresponded primarily to increased armoring, after accounting for effects of imperviousness; we refer to PC2 as the ‘coastal armoring index’.
2.2 Study design
In order to account for well-known spatial variability in both abiotic (e.g., salinity) and biotic (e.g., recruitment) factors (Dethier et al., 2012; Gallego et al., 2020), we collected data on biodiversity of invertebrates and two ecosystem functions (net primary productivity, decomposition) from freshwater and coastal marine habitats within six pairs of watersheds characterized by perennial streams, with similar drainage areas, occurring at similar latitudes, within the same oceanographic basins (5 pairs within Central Puget Sound, 1 pair in Hood Canal), and spanning a wide range of urbanization across Puget Sound (Figure 1, Tables S2–S3). Each pair consisted of a more and less urbanized watershed, such that more urbanized watersheds were defined as those that were more anthropogenically-modified (i.e., higher upland urbanization index and coastal armoring index values). Nested within each watershed, we selected two study sites, one each in freshwater and coastal marine habitats. Because eelgrass (Zostera marina) is a highly influential biogenic habitat in coastal marine environments, we also determined whether the more or less urbanized site in each watershed pair had more eelgrass in the coastal marine habitat (see Supplemental Materials, Table S2) and used this as a controlling factor in the analysis below. For analysis of freshwater invertebrate diversity only (for details of invertebrate collections and estimates of biodiversity see Section 2.3.1), we relied on publicly available data collected from 37 streams throughout Puget Sound to increase our sample size and strength of inference. These data were also analyzed using a paired design (see below).
We developed our paired-watershed study design because we know that several additional covariates describing urbanization are not available at the watershed level in Puget Sound. We conducted initial scoping of several fine-scale covariates (including stream water temperature, coastal marine water temperature, stream flow, stream dimensions, stream canopy cover, stream and coastal marine nutrient concentrations, and wind exposure of coastal marine habitats) and selected site pairs that were qualitatively similar (see Section 1.1 of the Supplemental Materials and Table S4 for details). As many other metrics of urbanization vary spatially, identifying geographically proximate pairs of watersheds allowed us to control for unobserved covariates that could potentially affect the relationship between ecosystem properties and urbanization (Figure 1). Although we could have explored relationships between ecosystem properties and urbanization at our study sites without this paired-watershed study design, doing so would have required that we had data for an even more robust set of potential covariates to include in the analysis. For example, freshwater and coastal marine habitats are influenced by upland topography, the timing and amplitude of tidal fluctuations, and salinity gradients all of which vary geographically in the Puget Sound region (Ruckelshaus and McClure, 2007). While some of this information is publicly available, finer-scale data on covariates that may also influence ecosystem properties are not (for our study specifically and for most cases of conservation concern). Thus, we determined that the paired-watershed study design was the most concise and tractable way to test for an association of urbanization with ecosystem properties in freshwater and coastal marine habitats, while controlling for as many other factors as possible.
Our study focused on the effects of urbanization on biodiversity and two types of ecosystem functions—net primary productivity and decomposition. Changes in biodiversity are a primary concern related to urbanization (Pickett et al., 1997; Aronson et al., 2014; Beninde et al., 2015; Clark et al., 2015). We evaluated two measures of biodiversity, the number of epibenthic macroinvertebrate families (family density), and the distribution of individuals among these families (Simpson diversity). Epibenthic macroinvertebrates are useful indicator species in both freshwater (Morley and Karr, 2002) and coastal marine (Bilkovic et al., 2006) habitats because they are relatively sedentary, tend to respond relatively quickly to changes in local environmental conditions, and their taxonomy is relatively well known. They are especially important prey items in both freshwater and coastal marine habitats for juvenile salmon (Wipfli and Baxter, 2010; Munsch et al., 2015; Kennedy et al., 2018; Davis et al., 2020). Net primary productivity and decomposition are two ecosystem functions thought to respond directly to urbanization, via associated changes in abiotic factors such as nutrients, light, and flow, or indirectly via changes in ecological communities (Table 1). These functions are fundamental to the production of new biomass and cycling of energy in ecosystems (Vitousek et al., 1986; Cebrian and Lartigue, 2004), and in salmon ecosystems in particular (Gende et al., 2002). The choice of a paired-watershed study design allowed us to examine 4 metrics (invertebrate richness, invertebrate diversity, primary production, decomposition) in each of 2 habitat types (coastal marine, freshwater) in 3 different years at ≥12 sites (Tables 2, S3).
2.3 Characterizing variability in urbanization and ecosystem properties
2.3.1 Biodiversity
In coastal marine habitats, we collected epibenthic macroinvertebrates along an ~100 m length of coastline at each of the study sites (n=3 samples site-1 year-1); (Tables 2). Collections occurred in July 2012, 2013, and 2014 using an epibenthic sled (1 m x 1 m opening, 1 mm mesh; Simenstad et al., 1991), towed over 10 m transects at each site (~-0.5m tidal elevation). We sampled the epibenthic community occurring within eelgrass beds at 9 of the study sites, and within mudflats at 3 of the study sites (Table S2), and samples were stored on ice in the field and transferred to ethanol for preservation within 24 hours.
In freshwater habitats, we used existing long-term data on stream invertebrates collected across Puget Sound (Puget Sound Stream Benthos, 2015), rather than collecting our own samples. To ensure temporal comparability between coastal marine and freshwater habitats, we focused on stream macroinvertebrate data collected by a variety of regional agencies and environmental organizations between 2012-2013 in the South Central Basin of Puget Sound (for details about standardized methods, see King County, 2009). Our analyses relied on count data aggregated at the family level to maximize the number of database entries we could use (n=37 watersheds ≤1,000 ha with perennial streams flowing directly into Puget Sound) and to ensure consistency with analysis of invertebrate data we collected from coastal marine habitats. We examined all possible pairings of more and less urbanized watersheds contained within this database, where urbanization was defined using upland urbanization index described above.
For both coastal marine and freshwater habitats, we quantified the number of macroinvertebrates in each taxonomic family (Tables S3, S4), a resolution considered sufficient for capturing major spatial differences in species composition for this region (Dethier and Schoch, 2006). We used the observed number of invertebrate families to estimate family density (number of taxonomic families observed) and Simpson diversity (a measure of evenness) from each sample (with the vegan package in R v3.1; R Core Team, 2014; Oksanen et al., 2015).
2.3.2 Net primary productivity
We assayed benthic freshwater and coastal marine organic matter accrual on pre-rinsed unglazed ceramic tiles (10cm x 10cm x 0.5cm deep; n = 10 site-1) in June-August 2012-2013 (Table 2). This approach primarily tracked algal growth on and immediately above the benthos, and we refer to it as index of net primary productivity (Hixon and Brostoff, 1996; Steinman et al., 2007). In coastal marine habitats, we used plastic ties to attach tiles to the tops of 1m long vertical PVC poles spaced evenly along an ~100m length of coastline (~-0.5m tidal elevation). In the freshwater habitats, we placed tiles exclusively in riffles every 2-5 m along a 100-200m reach. Tiles were attached to hardware cloth using plastic ties, and hardware cloth was attached to the streambed using rebar. We collected tiles from both freshwater and coastal marine habitats after 8-12 weeks to determine organic matter accrual. Organic matter accrual in streams was composed of micro-algae (e.g. benthic diatoms), fungi, microbes, etc., while coastal marine production was dominated by macroalgae such as Ulva spp. Tiles were stored on ice in the field and processed in the lab within 24 hours.
Prior to processing, we rinsed the tiles of sediments over a 500 micron wire mesh sieve and removed macroinvertebrates visible to the naked eye to minimize overestimation of algal organic matter. We scrubbed biofilm from the top surface of each tile using a bristled brush and filtered water. For coastal marine tiles, algal scrapings were placed directly into aluminum weigh boats, dried at 60°C for ≥72 hours, weighed, ashed at 400°C for 2 hours, and reweighed (Hixon and Brostoff, 1996). For freshwater tiles, twenty percent of the resulting 500 mL slurry was filtered onto a pre-ashed, pre-weighed 47-mm GF/F filter. These samples were dried at 60°C for 24 hours, weighed, ashed at 400°C for 2 hours, and reweighed (Steinman et al., 2007). For both freshwater and coastal marine tiles, the ash-free dry mass (AFDM) was taken as an estimate of the organic content of primary producers present on the tiles at the time of collection. We divided this measure of organic content by the amount of time the tiles were deployed to estimate rates of net primary productivity. (Preliminary investigation of tiles collected after 14, 28, 60, 90, and 120 days during a separate 2012 study suggested a linear rate of algal increase over time; Samhouri et al. unpublished data).
2.3.3 Decomposition
To track litter decomposition, we collected freshly fallen, dried maple leaves (Acer spp; freshwater habitats) or rinsed, drift eelgrass (Zostera marina; coastal marine habitats) and deployed these materials in mesh litter bags at each site (n = 8, 10 g bags site-1) (Tables 2). We chose maple leaves and eelgrass because these were the most common types of vegetation found decomposing naturally at our study sites. In the coastal marine habitats, we used plastic ties to attach litter bags to the tops of 1m long vertical PVC poles spaced evenly along an ~100m length of coastline in August 2013 (~-0.5m tidal elevation). In the freshwater habitats, we placed the litter bags exclusively in riffles every 2m -10m along a 100-200m reach in early November 2012 and 2013. Litter bags were attached to rebar (embedded within the streams) using plastic ties. We collected litter bags from both freshwater (1-2 months later) and coastal marine (4-6 weeks later) habitats to determine litter loss rates. Litter bags were stored on ice in the field and processed in the lab within 24 hours.
To minimize overestimation of remaining organic matter, we rinsed the litter bags of sediments over a 500 micron wire mesh sieve and removed macroinvertebrates visible to the naked eye. These pre-processed samples were transferred to aluminum weigh boats and dried at 60°C to constant weight for 24-72 hours, weighed, ashed at 400°C for 2 hours, and reweighed (Bretherton et al., 2011; Nicastro et al., 2012). For both freshwater and coastal marine litter bags, the ash-free dry mass (AFDM) was taken as an estimate of the organic content of litter remaining at the time of collection. Decomposition rates were estimated as the difference between initial litter bag weights (10g) and organic content remaining at the time of collection, divided by the number of days the litters bags were deployed.
2.4 Analyses
2.4.1 Comparisons of ecosystem properties along a continuum of urbanization
Our primary hypothesis was that urbanization would cause reductions in four ecosystem properties (family density, Simpson diversity, net primary productivity, and decomposition) in coastal marine and freshwater habitats. The metrics we used were consistent across coastal marine and freshwater habitats, although due to physiological and other constraints the specific taxa associated with these metrics differed between habitats. The analytical framework we used to evaluate changes in invertebrate family density and Simpson diversity was also similar in both freshwater and coastal marine habitats. Specifically, because urbanization scores for some watershed pairs differed more widely than for others (Figure 1; e.g., compare Pair 3 with Pair 6), we examined pairwise differences in ecosystem properties within each watershed pair. We estimated the disparity in urbanization as the difference in the upland urbanization index (PC1) values or the difference in the coastal armoring index (PC2) values between the more and less urbanized watershed in each pair (hereafter, urbanization differences). Note that because they are strongly correlated with urbanization, other measures of urbanization produce qualitatively similar relationships to those analyzed here (Table A1). Similarly, to estimate the disparity in ecosystem properties, we calculated the difference in the average value of each property between the more and less urbanized watershed in each pair (hereafter, ecosystem property difference). We used ANCOVA to test for the effect of upland urbanization index difference (a linear covariate) and year (a categorical factor) on ecosystem property difference (e.g., Simpson diversity difference). For coastal marine ecosystem properties, we included 2 additional predictor variables in the models: the coastal armoring index difference (a linear covariate) and eelgrass difference (a categorical factor, based on whether the more urbanized site in each pair had more or less eelgrass). This analytical structure accurately reflected the paired design of our study and thus provided the strongest available test of the effects of changes in land cover, while accounting for other covariates (both measured [Table S4] and unmeasured).
Because we used a larger data set of watersheds for freshwater invertebrates, we employed a slightly different set of analyses for freshwater invertebrate family density and Simpson diversity. Specifically, we examined all possible pairings of the 37 watersheds using a Mantel test. Like ANCOVA, a Mantel test is a regression, but it considers variables as distance matrices summarizing all possible pairwise similarities among sample locations. We used a Mantel test to determine whether watershed pairs that were more dissimilar in the upland urbanization index were also more dissimilar in ecosystem properties, while controlling for dissimilarity in the area of the watershed basins from which the invertebrate data were derived. For these analyses, we evaluated 2012 and 2013 data separately, with the upland urbanization index difference rescaled so that positive values reflected smaller differences in upland urbanization in order to avoid excessive Type II error inherent to a Mantel test when the correlations between predictor and dependent variables are expected to be negative (Legendre and Fortin, 2010). Because we had a larger number of watersheds to consider for the analysis of freshwater biodiversity (n=37 rather than n=12 for the other metrics), implementation of a Mantel test provided a more robust assessment of associations between urbanization and these ecosystem properties but would not have been feasible with the smaller sample size available for the two metrics of coastal marine biodiversity, as well as for freshwater and coastal marine net primary productivity and decomposition.
2.4.2 Moving from land to sea: Comparison of the strength of relationships between ecosystem properties and urbanization in freshwater and coastal marine habitats
To test the hypothesis that urbanization was more strongly associated with ecosystem properties within freshwater habitats than within coastal marine habitats, we compared the strength of the upland urbanization index difference effect for biodiversity, net primary productivity, and decomposition across habitats. To ensure that effect sizes were comparable while avoiding confounding effects of different sample sizes between habitats, we standardized each habitat-specific dependent variable (by subtracting the mean and dividing by the standard deviation), conducted a linear regression of it against the upland urbanization index difference to estimate the ‘urbanization’ coefficient, and compared this value (and its 95% CI) across habitats.
3 Results
3.1 Comparisons of ecosystem properties along a continuum of urbanization
The wide range of land cover and ecosystem properties we observed facilitated a strong test for associations between urbanization and ecosystem properties. Specifically, the watersheds in our study spanned 1% to >40% imperviousness and 25% to 100% shoreline armoring. We also found considerable variation in ecosystem properties across our study sites. In coastal marine habitats, invertebrate family density varied 6-fold (range: 3-18 families), Simpson diversity ranged almost an order of magnitude (0.07-0.79), net primary productivity varied by almost 2 orders of magnitude (2.76 x 10-4 – 1.97 x10-2 g day-1), and decomposition rates varied from nil to -0.4 g day-1. In freshwater habitats, invertebrate family density varied almost 5-fold (range: 6-29 families), Simpson diversity varied >3-fold (0.26-0.9), net primary productivity ranged almost a full order of magnitude (1.02 x 10-4 – 8.3 x10-4 g day-1), and decomposition rates spanned a 5-fold range (-0.04 to -0.22 g day-1).
We expected increases in urbanization would cause decreases in ecosystem properties, but instead we found that urbanization effects were context-dependent. In watershed pairs that were similar in upland urbanization index values (i.e., <20% difference), more urbanized coastal marine habitats tended to have higher family density than their less urbanized counterparts (Figure 2A). Counter-intuitively, as the upland urbanization index difference between watersheds within a pair grew large, differences in coastal marine family density became negligible (Figure 2A; p = 0.05). There was not a significant association between coastal marine Simpson diversity difference and the upland urbanization index difference (Figure 2B; p = 0.53), although coastal marine Simpson diversity tended to be higher at the less urbanized site within each site pair (difference values tended to be negative; Figure 2B). The coastal armoring index difference, eelgrass difference, and year effects on the differences in coastal marine family density and coastal marine Simpson diversity were all statistically non-significant.
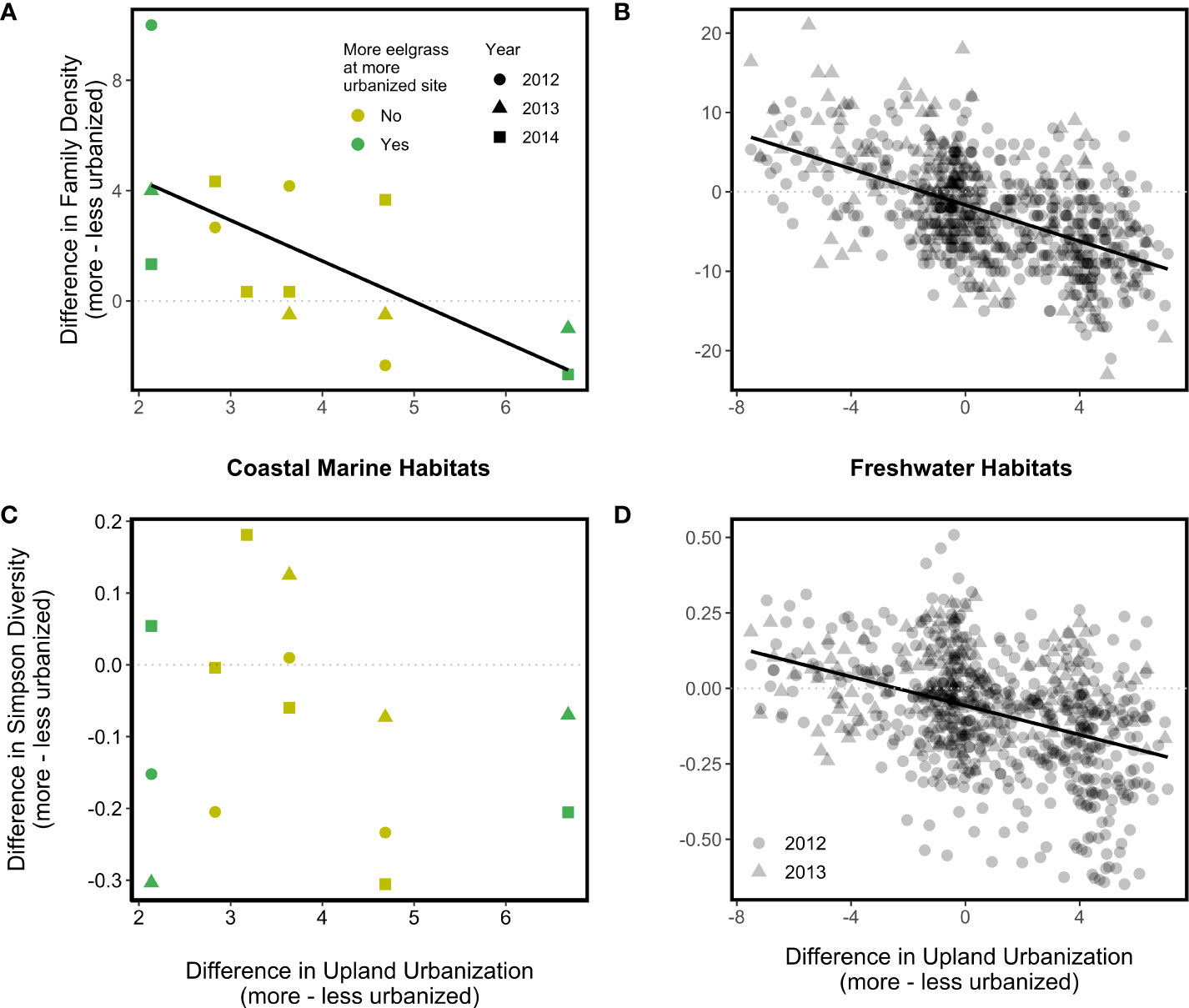
Figure 2 Associations between the upland urbanization difference and disparity between more and less urbanized watersheds in two measures of invertebrate diversity (top, Family Density; bottom, Simpson Diversity) in coastal marine (A, C) and freshwater (B, D) habitats. Solid regression lines indicate statistically significant associations; dotted lines serve as a reference representing zero difference in the diversity indices between more and less urbanized watersheds.
In freshwater habitats, family density difference (Figure 2C) in 2012 (p < 0.001) and 2013 (p < 0.001) and Simpson diversity difference (Figure 2D) in 2012 (p < 0.001) were negatively associated with the upland urbanization index difference, such that more urbanized watersheds tended to have lower family density and Simpson diversity than less urbanized watersheds. The Simpson diversity difference in 2013 also demonstrated a negative relationship with the upland urbanization index difference, but this relationship was nonsignificant (p = 0.11).
There were no significant associations between the upland urbanization index difference or year and differences in the two ecosystem functions we tracked, net primary productivity and decomposition (Figure 3). Similarly, there were no significant effects of the coastal armoring index difference or eelgrass difference on the differences in the two coastal marine ecosystem functions. Qualitatively, coastal marine and freshwater primary production tended to be higher at the less urbanized site within each site pair (difference values tended to be negative; mean value at more urbanized freshwater sites = 2.09 x 10-4 g day-1, mean value at less urbanized freshwater sites = 3.47 x 10-4 g day-1; mean value at more urbanized coastal marine sites = 2.75 x 10-3 g day-1, mean value at less urbanized coastal marine sites = 5.98 x 10-3 g day-1; Figures 3A, B), whereas freshwater decomposition tended to be higher at the more urbanized site within each site pair (difference values tended to be positive; mean value at more urbanized freshwater sites = 0.162 g day-1, mean value at less urbanized freshwater sites = 0.129 g day-1; Figure 3D).
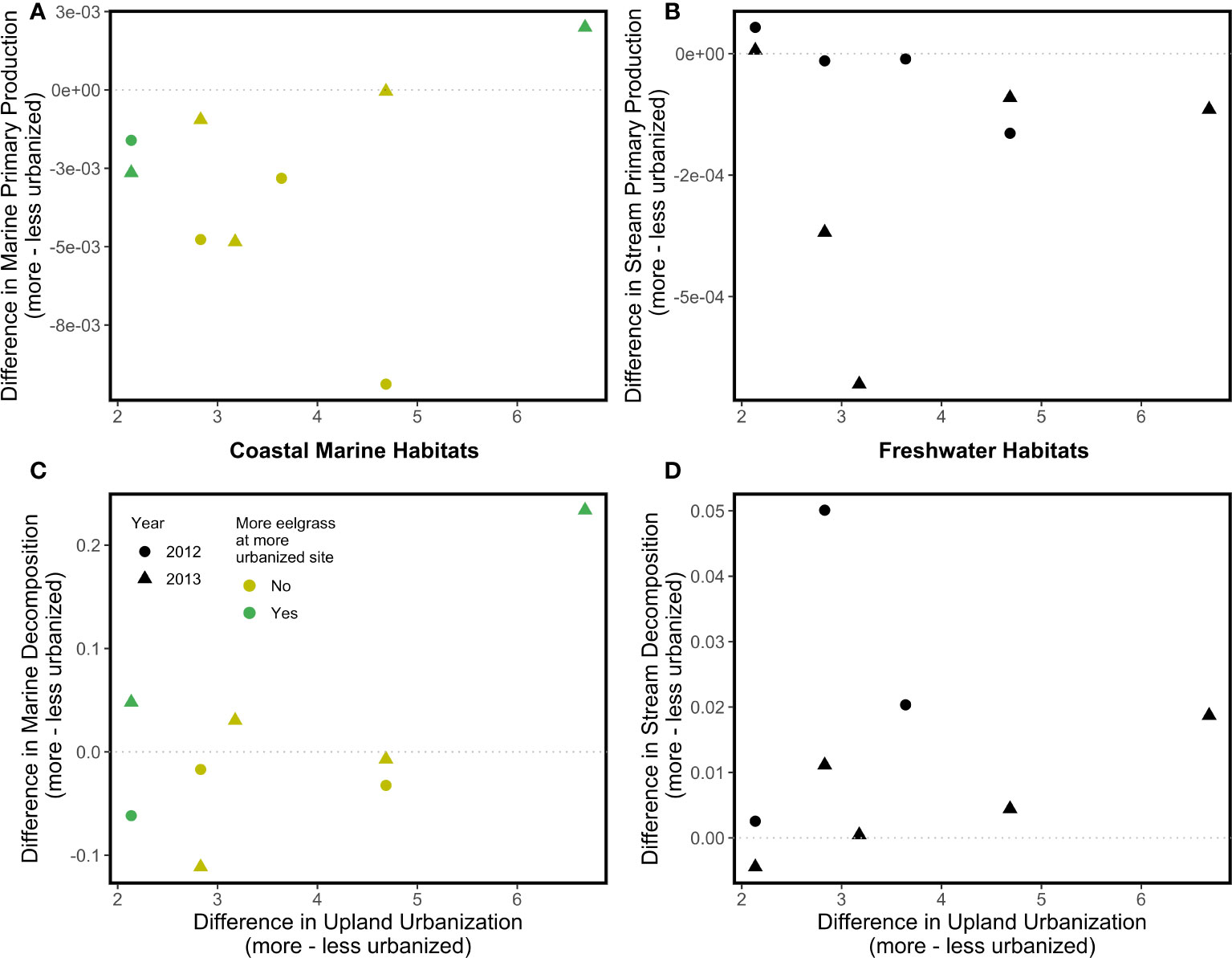
Figure 3 Associations between the upland urbanization difference and disparity between more and less urbanized watersheds in two ecosystem functions (top, Net Primary Productivity; bottom, Decomposition) in coastal marine (A, C) and freshwater (B, D) habitats. No regressions were statistically significant; dotted lines serve as a reference representing zero difference in the ecosystem properties between more and less urbanized watersheds.
3.2 Moving from land to sea: Comparison of the strength of relationships between ecosystem properties and urbanization in freshwater and coastal marine habitats
Differences between more and less urbanized sites in the two measures of biodiversity we tracked were negatively associated with the upland urbanization index difference in coastal marine and freshwater habitats (Section 3.1, Figure 2), as hypothesized. Though we predicted a stronger relationship in freshwater than coastal marine habitats, the strength of associations between family density difference and the upland urbanization index difference, and that between Simpson diversity difference and the upland urbanization index difference, were similar in both coastal marine and freshwater habitats because of high variance in the coastal marine metrics (Figure 4). Because neither net primary productivity difference nor decomposition difference was significantly associated with the upland urbanization index difference in freshwater or coastal marine habitats (i.e., the slopes of these regression lines were not significantly different than zero; Figure 3), we can also reject the hypothesis that urbanization was significantly more associated with freshwater ecosystem functions than coastal marine ecosystem functions.
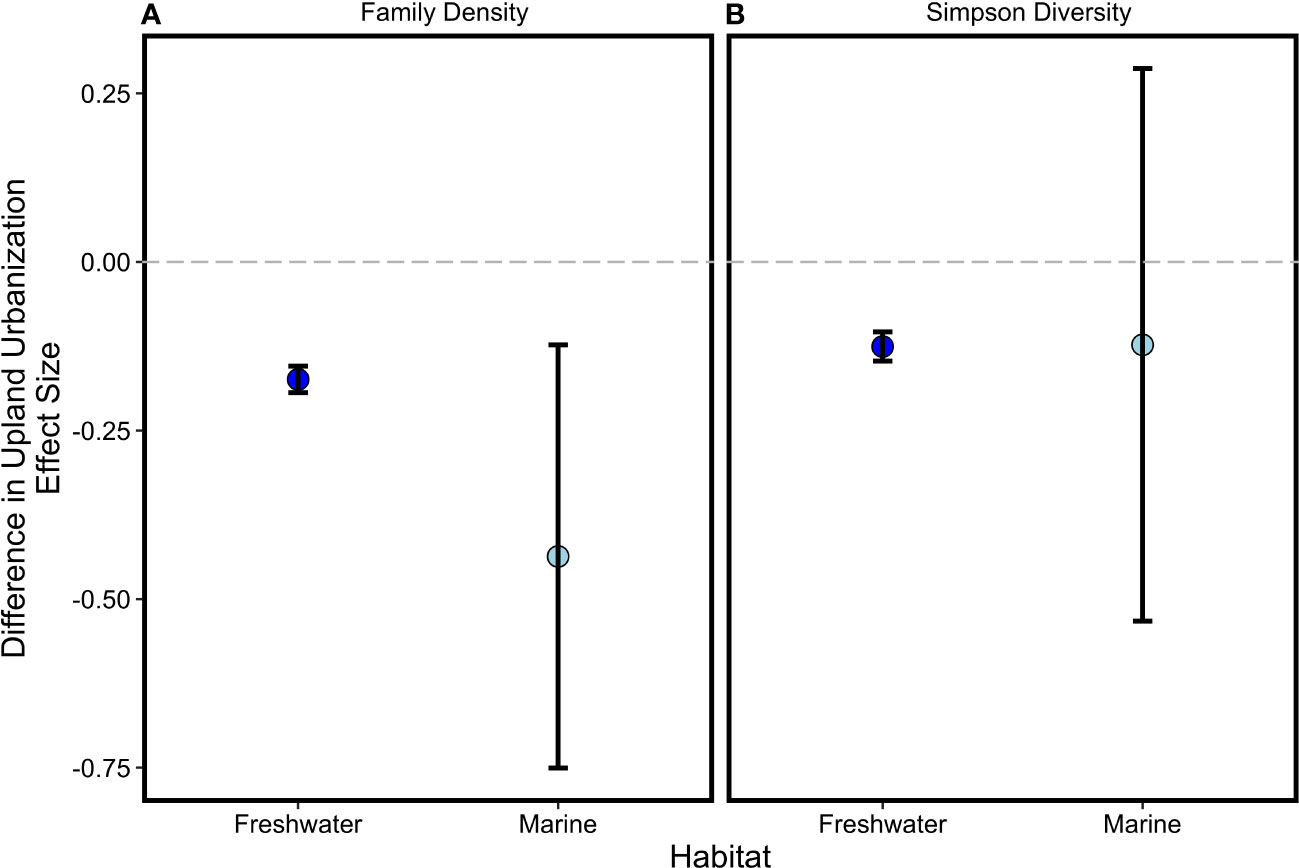
Figure 4 Mean effect sizes (with 95% CI) for relationships between biodiversity differences [(A) family density; (B) Simpson diversity] and differences in the upland urbanization index in freshwater and coastal marine habitats. Effect sizes related to two ecosystem functions (net primary productivity and decomposition) also did not differ between habitat types (not shown).
4 Discussion
As coastal cities expand, it is increasingly important to understand the effects of human activities on terrestrial, freshwater, and coastal marine habitats. Urbanized ecosystems generate a wide range of services on which people depend, including protection from severe weather and climate impacts, improved air and water quality, stress relief, and food production (Grimm et al. 2008; Niemelaä et al., 2011; Lepczyk et al., 2017; Keeler et al., 2019). However, it is often assumed that “ecology [with]in cities” is impaired or diminished relative to that of less-urbanized locations, causing urban areas to rely strongly on unpopulated ecosystems in distant, rural, and undeveloped locations (Jansson, 2013). Here we found biodiversity is not uniformly lower (Figure 2), net primary productivity and decomposition ecosystem functions are not measurably different (Figure 3), and coastal marine habitats are not less affected than freshwater habitats by urbanization (Figure 4). It is of course possible that other, unobserved, elements of urbanization may be associated with declines in biodiversity or ecosystem functions. Nevertheless, our results suggest that discussion of ecology within cities and expectations for the services they can provide require nuanced consideration (Grimm et al. 2008, Dearborn and Kark, 2010; Ives et al., 2016). Further, our findings provide three lessons—one cautionary, another optimistic, and a third motivational—for coastal conservation and management.
Often, conservation and management goals focus on broad terms such as biodiversity, ecosystem functions, and resilience (McLeod and Leslie, 2009). For example, the United Nations Convention on Biological Diversity has developed a set of strategic goals related to “biodiversity” (United Nations (UN), 2010). Regionally, the Washington legislature established an objective to “protect ecosystem biodiversity” in Puget Sound (Revised Code of Washington 90.71.300 Action Agenda – Goals and Objectives) and there are major efforts underway to track progress toward it. However, as our results suggest, terms such as “biodiversity” are quite coarse, making it difficult to discern when changes in biodiversity are meaningful. We found that alternative indicators for a single ecosystem property can conflict, as they did for the two measures of coastal marine biodiversity in our study. Additionally, even if a single indicator (such as Simpson diversity) is preferred, increases in diversity are not universally desirable (e.g., due to introductions of non-indigenous species; Lean, 2021). We found that coastal marine invertebrate family density was higher in Puget Sound at more urbanized sites when the extent of upland urbanization was small relative to paired, less urbanized sites (Figure 2; also see Ives et al., 2016). This finding leaves open the questions of whether small amounts of urbanization-related disturbance can augment diversity. If so, this pattern would provide support for an intermediate disturbance hypothesis related to human impacts (Connell, 1978; Vonshak and Gordon, 2015), whether or not initial increases in diversity within low to moderate urbanization emerge from normatively desirable or nuisance species. Furthermore, a rigorous examination of whether the additional taxonomic families observed at our more urbanized sites are ecologically important to key elements of biodiversity, such as Pacific salmon that rely on a subset of available invertebrate prey during their migration through coastal marine habitats (Munsch et al., 2015; Davis et al., 2020), was beyond the scope of this study. On the other hand, our observations of lower macroinvertebrate family densities at more urbanized sites in freshwater habitats correspond to lower values for the Index of Biological Integrity (Peter et al., 2022). A large literature suggests that, in combination with other changes to freshwater and coastal marine habitats (e.g., geomorphological; Foley et al., 2017), the Index of Biological Integrity is a reasonable proxy of impaired water quality and likely poorer ecosystem conditions for salmon (though any inferences around ecosystem conditions for salmon are best made by capturing a broad suite of factors). The specific contrasts in the two biodiversity metrics and in freshwater versus coastal marine habitats underscore how clarity of purpose for choosing a particular indicator will help to inform actionable strategies for meeting conservation and management goals. It is fully possible that other ecosystem properties not assessed here may vary in predictable ways with urbanization, including effects of urbanization we did not explicitly quantify (e.g., eutrophication and associated oxygen depletion effects). Active efforts to further refine and specify how to measure progress toward biodiversity goals are ongoing in both the UN and Puget Sound contexts (Samhouri and Levin, 2012; McQuatters-Gollop et al., 2019). To these ends, we encourage future research that explores how ecosystem properties vary with urbanization more comprehensively in Puget Sound and beyond.
The second lesson from this research is more optimistic: increasing urbanization was not consistently or significantly associated with greater impairment of two key ecosystem functions in either freshwater or coastal marine habitats (Figure 3). These two functions, net primary productivity and decomposition, are fundamental to “healthy and resilient” ecosystems (Christensen, 1995; Elliott and Quintino, 2007; Samhouri et al., 2010) advocated in calls for widespread implementation of EBM (McLeod and Leslie, 2009). They underpin the production of consumer biomass (including consumers that serve as human foods; Pauly and Christensen, 1995; Brown et al., 2004) and the efficient recycling of energy and nutrients via microbes and macrobes, an archetypal regulating service (MEA, 2005). Though beyond the scope of our study, we believe factors largely independent of urbanization at our study sites—such as the influence of light (net primary productivity) or turbulence (decomposition)—contributed most to the wide range of spatial variability in ecosystem properties we observed. We have embraced a landscape perspective in arriving at this conclusion, and recognize that ecosystem properties could respond to urbanization at a different spatial scale(s) than those addressed here.
In the Puget Sound region and beyond, it is increasingly common to observe environmental policies that call for integrated land-sea planning and ecosystem-based management that crosses traditional social-ecological boundaries (POC, 2003; PSP, 2008; Ãlvarez-Romero et al., 2011; Samhouri and Levin, 2012). The third lesson from this study motivates a call for support of such initiatives, as we found that associations between ecosystem properties and urbanization did not diminish in strength between freshwater and coastal marine habitats. This finding runs counter to others suggesting that allochthonous inputs from land are not as important as internally generated drivers in coastal marine environments (Brett et al., 2009; Steinberg et al., 2010), and echoes more widely accepted understanding of major river influences on coastal marine ecosystems (Diaz and Rosenberg, 2008). The main difference is that in our study, we tested connections within much smaller watersheds, typically thought to be of minimal influence on coastal marine ecosystems (Smith et al., 2003; Halpern et al., 2009). Not only did we fail to distinguish between upland urbanization impacts to freshwater and coastal marine habitats within these watersheds in this study, but in the case of Puget Sound there are widely understood linkages between freshwater and coastal marine habitats generated by species of concern, especially Pacific salmon (Buckner et al., 2018). In light of these results, integrated land-sea planning such as that spearheaded in the Puget Sound region by the Puget Sound Partnership, and elsewhere around the world (Tissot et al., 2009; Takeda and Røpke, 2010), offers strong promise.
In conclusion, rather than providing unequivocal evidence for the negative impacts of urban landscapes on biodiversity and ecosystem functions, our study supports growing evidence that a dichotomy between urbanized and non-urbanized ecological communities is less straightforward than is often assumed (Table 1). This study quantified tractable metrics of ecosystem structure and function across a broad gradient of urbanization, and while the properties on which we focused may not have been perfect or comprehensive, the insights from them dispel some commonly-held assumptions about uniformly negative impacts of urbanization while informing future areas fertile for research. Moving forward, questions around whether ecological responses to urbanization occur at different spatial scales then measured here deserve exploration, as do associated changes in functional diversity, disease, pathogens, and invasion risk (Todd et al., 2019). Similarly, more directed inquiry regarding specific mechanistic relationships using indices of human influence that better reflect underlying processes might provide qualitatively different insights about the impacts of urbanization on ecosystem structure and function. These possibilities are especially intriguing given that the negative association between freshwater diversity and urbanization observed in this study, and established previously (Morley and Karr, 2002), was striking not so much for its mean effect size (Figure 4), but for the amount of unexplained variance around the trend (Figure 2). High variability and relatively weak effects of urbanization suggest a role for context-dependent ecosystem responses, which may require tracking different measures of urban impacts and ecosystem properties. Insights such as these suggest fertile ground for future work to consider urbanization as one of multiple factors, ranging from physical to geomorphological to ecological and beyond, that influence ecosystem structure, function, and the relationship between the two. We especially encourage future research that expands the number of study sites and organizational levels considered, and that simultaneously estimates salmon performance in relation to urbanization-related impacts to Puget Sound ecosystem components. More broadly, our study suggests that integrative, umbrella indicators such as biodiversity are likely most informative when reported with more granular measures reflecting the status of species and habitats of particular concern. Such a portfolio of indicators can then be managed collectively to ensure that whole ecosystems, as well as the parts within them, are making progress toward society’s desired futures (Nicholson et al., 2021).
Data availability statement
The datasets presented in this study can be found in online repositories. The names of the repository/repositories and accession number(s) can be found below: https://github.com/jameals/howmuchcity.
Author contributions
JS, AS, GW, BF, KB, MS, and PL contributed to conception and design of the study. BF, SH, JS, and AS organized the database. JS, AS, BF, SH, RK, JO’D, and AS performed the statistical analysis. JS wrote the first draft of the manuscript. All authors contributed to the article and approved the submitted version.
Acknowledgments
We thank Ellie Canade, Hannah Fotherby, Severa Ziegler, Daniela Ramos, Andrea Wong, Joe Donohoe, Emma Garrison, Jacqui Levy, Lucas Earl, Mira Klein, Emily Buckner, William Zhou, the NWFSC Watershed group, the UW Kelly lab, the Osawas, Eliza Heery, and coastal property owners (especially SPL and RAL at Eglon Creek) for support of this research. We also recognize Blue Moose Farm and Friday Harbor Labs for inspiration.
Conflict of interest
The authors declare that the research was conducted in the absence of any commercial or financial relationships that could be construed as a potential conflict of interest.
Publisher’s note
All claims expressed in this article are solely those of the authors and do not necessarily represent those of their affiliated organizations, or those of the publisher, the editors and the reviewers. Any product that may be evaluated in this article, or claim that may be made by its manufacturer, is not guaranteed or endorsed by the publisher.
Supplementary material
The Supplementary Material for this article can be found online at: https://www.frontiersin.org/articles/10.3389/fmars.2022.931319/full#supplementary-material
References
Airoldi L., Turon X., Perkol-Finkel S., Rius M. (2015). Corridors for aliens but not for natives: effects of marine urban sprawl at a regional scale. Diversity and Distributions 21(7), 755–768. doi: 10.1111/ddi.12301
Alberti M., Booth D., Hill K., Coburn B., Avolio C., Coe S., et al. (2007). The impact of urban patterns on aquatic ecosystems: An empirical analysis in puget lowland sub-basins. Landscape Urban. Plann. 80, 345–361. doi: 10.1016/j.landurbplan.2006.08.001
Ãlvarez-Romero J. G., Pressey R. L., Ban N. C., Vance-Borland K., Willer C., Klein C. J., et al. (2011). Integrated land-Sea conservation planning: The missing links. Annu. Rev. Ecol. Evol. Syst. 42, 381–409. doi: 10.1146/annurev-ecolsys-102209-144702
Aronson R. B., Hilbun N. L., Bianchi T. S., Filley T. R., McKee B. A. (2014). Land use, water quality, and the history of coral assemblages at bocas del toro, panamá. Mar. Ecol. Prog. Ser. 504, 159–170. doi: 10.3354/meps10765
Barbour M. T., Gerritsen J., Snyder B. D., Stribling J. B. (1999). Rapid Bioassessment Protocols for Use in Wadeable Streams and Rivers: Periphyton, Benthic Macroinvertebrates and Fish (2nd ed.). Washington, D.C. USA: U.S. Environmental Protection Agency; Office of Water. 295 pp.
Bartz K. K., Ford M. J., Beechie T. J., Fresh K. L., Pess G. R., Kennedy R. E., et al. (2015). Trends in developed land cover adjacent to habitat for threatened salmon in puget sound, Washington, U.S.A. PloS One 10, e0124415. doi: 10.1371/journal.pone.0124415
Beger M., Grantham H. S., Pressey R. L., Wilson K. A., Peterson E. L., Dorfman D., et al. (2010). Conservation planning for connectivity across marine, freshwater, and terrestrial realms. Biol. Conserv. 143, 565–575. doi: 10.1016/j.biocon.2009.11.006
Benfield E. F. (2006). Decomposition of Leaf Material. in Hauer F. R., Lamberti G. A., Editors. Methods in Stream Ecology. Elsevier, Burlington, Massachusetts, USA. Pages 711–720
Beninde J., Veith M., Hochkirch A. (2015). Biodiversity in cities needs space: a meta-analysis of factors determining intra-urban biodiversity variation. Ecol. Lett. 18, 581–592. doi: 10.1111/ele.12427
Bertasi F., Colangelo M. A., Abbiati M., Ceccherelli V. U. (2007) Effects of an artificial protection structure on the sandy shore macrofaunal community: the special case of Lido di Dante (Northern Adriatic Sea). Hydrobiologia, 586, 2–7. doi: 10.1007/s10750-007-0701-y
Bilkovic D. M., Mitchell M., Mason P., Duhring K. (2016). The role of living shorelines as estuarine habitat conservation strategies. Coast. Manage. 44 (3), 161–174. doi: 10.1080/08920753.2016.1160201
Bilkovic D. M., Roggero M., Hershner C. H., Havens K. H. (2006). Influence of land use on macrobenthic communities in nearshore estuarine habitats. Estuaries. Coasts. 29, 1185–1195. doi: 10.1007/BF02781819
Blake R. E., Duffy J. E., Richardson J. P. (2014) Patterns of seagrass community response to local shoreline development. Estuaries and Coasts 37, 1549–1561. doi: 10.1007/s12237-014-9784-7
Bowen J. L., Valiela I. (2001). The ecological effects of urbanization of coastal watersheds: historical increases in nitrogen loads and eutrophication of Waquoit Bay estuaries. Canadian Journal of Fisheries and Aquatic Sciences 58, 1489–1500. doi: 10.1139/f01-094
Bradford M. A., Tordoff G. M., Eggers T., Jones T. H., Newington J. E. (2002). Microbiota, fauna, and mesh size interactions in litter decomposition. Oikos 99, 317–323. doi: 10.1034/j.1600-0706.2002.990212.x
Bretherton W. D., Kominoski J. S., Fischer D. G., LeRoy C. J. (2011). Salmon carcasses alter leaf litter species diversity effects on in-stream decomposition. Can. J. Fish. Aquat. Sci. 68, 1495–1506. doi: 10.1139/f2011-082
Brett M. T., Kainz M. J., Taipale S. J., Seshan H. (2009). Phytoplankton, not allochthonous carbon, sustains herbivorous zooplankton production. Proc. Natl. Acad. Sci. 106, 21197–21201. doi: 10.1073/pnas.0904129106
Brown J. H., Gillooly J. F., Allen A. P., Savage V. M., West G. B. (2004). Toward a metabolic theory of ecology. Ecology 85, 1771–1789. doi: 10.1890/03-9000
Buckner E. V., Hernández D. L., Samhouri. J. F. (2018). Conserving connectivity: Human influence on subsidy transfer and relevant restoration efforts. Ambio 47, 493–503. doi: 10.1007/s13280-017-0989-4
Bulleri F., Chapman M. G. (2010). The introduction of coastal infrastructure as a driver of change in marine environments. J. Appl. Ecol. 47, 26–35. doi: 10.1111/j.1365-2664.2009.01751.x
Cebrian J., Lartigue J. (2004). Patterns of herbivory and decomposition in aquatic and terrestrial ecosystems. Ecol. Monogr. 74, 237–259. doi: 10.1890/03-4019
Chadwick M. A., Dobberfuhl D. R., Benke A. C., Huryn A. D., Suberkropp K., Thiele J. E., et al (2006). Urbanization affects stream ecosystem function by altering hydrology, chemistry, and biotic richness. Ecological Applications 16, 1796–1807. doi: 10.1890/1051-0761(2006)016[1796:UASEFB]2.0.CO;2
Chapman M. G. (2003) Paucity of mobile species on constructed seawalls: effects of urbanization on biodiversity. Marine Ecology Progress Series 264, 21–29. doi: 10.3354/meps264021
Chapman M. G., Underwood A. J. (2011). Evaluation of ecological engineering of A’rmoured’ shorelines to improve their value as habitat. Journal of Experimental Marine Biology and Ecology 400, 302–313. doi: 10.1016/j.jembe.2011.02.025
Christensen V. (1995). Ecosystem maturity – towards quantification. Ecol. Model. 77, 3–32. doi: 10.1016/0304-3800(93)E0073-C
Clapcott J. E., Young R. G., Goodwin E. O., Leathwick J. R. (2010) APPLIED ISSUES: Exploring the response of functional indicators of stream health to land-use gradients. Freshwater Biology 55, 2181–2199. doi: 10.1111/j.1365-2427.2010.02463.x
Clark G. F., Kelaher B. P., Dafforn K. A., Coleman M. A., Knott N. A., Marzinelli E. M., et al. (2015). What does impacted look like? high diversity and abundance of epibiota in modified estuaries. Environ. Pollut. 196, 12–20. doi: 10.1016/j.envpol.2014.09.017
Connell J. H. (1978). Diversity in tropical rain forests and coral reefs. Science 199, 1302–1310. doi: 10.1126/science.199.4335.1302
Cordell J. R., Levy C., Toft J. D. (2013). Ecological implications of invasive tunicates associated with artificial structures in Puget Sound, Washington, USA. Biological Invasions 15(6), 1303–1318. doi: 10.1007/s10530-012-0366-y
Crossett K., Ache B., Pacheco P., Haber K. (2013) National coastal population report. Available at: http://stateofthecoast.noaa.gov/features/coastal-population-report.pdf.
Cuffney T. F., Brightbill R. A., May J. T., Waite I. R. (2010). Responses of benthic macroinvertebrates to environmental changes associated with urbanization in nine metropolitan areas. Ecol. Appl. 20, 1384–1401. doi: 10.1890/08-1311.1
Davis M. J., Chamberlin J. W., Gardner J. R., Connelly K. A., Gamble M. M., Beckman B. R., et al. (2020). Variable prey consumption leads to distinct regional differences in Chinook salmon growth during the early marine critical period. Mar. Ecol. Prog. Ser. 640, 147–169. doi: 10.3354/meps13279
Dearborn D. C., Kark S. (2010). Motivations for conserving urban biodiversity. Conserv. Biol. 24, 432–440. doi: 10.1111/j.1523-1739.2009.01328.x
Dethier M. N., Raymond W. W., McBride A. N., Toft J. D., Cordell J. R., Ogston A. S., et al. (2016). Multiscale impacts of armoring on salish Sea shorelines: Evidence for cumulative and threshold effects. Estuarine. Coast. Shelf. Sci. 175, 106–117. doi: 10.1016/j.ecss.2016.03.033
Dethier M. N., Ruesink J., Berry H., Sprenger A. G. (2012). Decoupling of recruitment from adult clam assemblages along an estuarine shoreline. J. Exp. Mar. Biol. Ecol. 422–423, 48–54. doi: 10.1016/j.jembe.2012.04.009
Dethier M. N., Schoch G. C. (2006). Taxonomic sufficiency in distinguishing natural spatial patterns on an estuarine shoreline. Mar. Ecol. Prog. Ser. 306, 41–49. doi: 10.3354/meps306041
Diaz R. J., Rosenberg R. (2008). Spreading dead zones and consequences for marine ecosystems. Science 321, 926–929. doi: 10.1126/science.1156401
Dugan J. E., Hubbard D. M., Rodil I. F., Revell D. L., Schroeter S. (2008). Ecological effects of coastal armoring on sandy beaches. Marine Ecology 29, 160–170. doi: 10.1111/j.1439-0485.2008.00231.x
Elliott M., Quintino V. (2007). The estuarine quality paradox, environmental homeostasis and the difficulty of detecting anthropogenic stress in naturally stressed areas. Mar. Pollut. Bull. 54, 640–645. doi: 10.1016/j.marpolbul.2007.02.003
Ernstson H., Redman C. L., Meffert D. J., Davis G., Alfsen C., Elmqvist T. (2010). Urban transitions: On urban resilience and human-dominated ecosystems. AMBIO 39, 531–545. doi: 10.1007/s13280-010-0081-9
Feist B. E., Buhle E. R., Baldwin D. H., Spromberg J. A., Damm S. E., Davis J. W., et al. (2017). Roads to ruin: conservation threats to a sentinel species across an urban gradient. Ecol. Appl. 27 (8), 2382–2396. doi: 10.1002/eap.1615
Foley M. M., Warrick J. A., Ritchie A., Stevens A. W., Shafroth P. B., Duda J. J., et al. (2017). Coastal habitat and biological community response to dam removal on the elwha river. Ecol. Monogr. 87, 552–577. doi: 10.1002/ecm.1268
Francis T. B., Schindler D. E. (2009). Shoreline urbanization reduces terrestrial insect subsidies to fishes in north American lakes. Oikos 118, 1872–1882. doi: 10.1111/j.1600-0706.2009.17723.x
Friedman W. R., Martin B. T., Wells B. K., Warzybok P., Michel C. J., Danner E. M., et al. (2019). Modeling composite effects of marine and freshwater processes on migratory species. Ecosphere 10, e02743. doi: 10.1002/ecs2.2743
Fry J., Xian G., Jin S., Dewitz J., Homer C., Yang L., et al. (2011). Completion of the 2006 national land cover database for the conterminous united states. Photogramm. Eng. Remote Sens. 77, 858–864.
Gallego R., Jacobs-Palmer E., Cribari K. J., Kelly R. P. (2020). Environmental DNA metabarcoding reveals winners and losers of global change in coastal waters. Proc. R. Soc. Ser. B 287, 20202424. doi: 10.1098/rspb.2020.2424
Gende S. M., Edwards R. T., Willson M. F., Wipfli. M. S. (2002). Pacific salmon in aquatic and terrestrial ecosystems: Pacific salmon subsidize freshwater and terrestrial ecosystems through several pathways, which generates unique management and conservation issues but also provides valuable research opportunities. BioScience 52, 917–928. doi: 10.1641/0006-3568(2002)052[0917:PSIAAT]2.0.CO;2
Gosselin J. L., Zabel R. W., Anderson J. J., Faulkner J. R., Baptista A. M., Sandford B. P. (2018). Conservation planning for freshwater–marine carryover effects on Chinook salmon survival. Ecol. Evol. 8, 319–332. doi: 10.1002/ece3.3663
Granek E. F., Polasky S., Kappel C. V., Reed D. J., Stoms D. M., Koch E. W., et al. (2010). Ecosystem services as a common language for coastal ecosystem-based management. Conserv. Biol. 24, 207–216. doi: 10.1111/j.1523-1739.2009.01355.x
Grimm N. B., Faeth S. H., Golubiewski N. E., Redman C. L., Wu J., Bai X., et al. (2008). Global Change and the Ecology of Cities. Science.
Halpern B. S., Ebert C. M., Kappel C. V., Madin E. M. P., Micheli F., Perry M., et al. (2009). Global priority areas for incorporating land-sea connections in marine conservation. Conserv. Lett. 2, 189–196. doi: 10.1111/j.1755-263X.2009.00060.x
Hauer F. R., Lamberti G. A. (2011). Methods in stream ecology. 2nd ed (Burlington, MA USA: Academic Press), 877 pp.
Heerhartz S. M., Dethier M. N., Toft J. D., Cordell J. R., Ogston A. S. (2013) Effects of Shoreline Armoring on Beach Wrack Subsidies to the Nearshore Ecotone in an Estuarine Fjord. Estuaries and Coasts, 37, 1256–1268. doi: 10.1007/s12237-013-9754-5
Hixon M. A., Brostoff W. N. (1996). Succession and herbivory: Effects of differential fish grazing on Hawaiian coral-reef algae. Ecol. Monogr. 66, 67–90. doi: 10.2307/2963481
Inglis G. J., Kross J. E. (2000) Evidence for Systemic Changes in the Benthic Fauna of Tropical Estuaries as a Result of Urbanization. Marine Pollution Bulletin 41, 367–376. doi: 10.1016/S0025-326X(00)00093-X
Ives C. D., Lentini P. E., Threlfall C. G., Ikin K., Shanahan D. F., Garrard G. E., et al. (2016). Cities are hotspots for threatened species. Global Ecol. Biogeography. 25, 117–126. doi: 10.1111/geb.12404
Jansson Å. (2013). Reaching for a sustainable, resilient urban future using the lens of ecosystem services. Ecol. Econ. 86, 285–291. doi: 10.1016/j.ecolecon.2012.06.013
Keeler B. L., Hamel P., McPhearson T., Hamann M. H., Donahue M. L., Meza Prado K. A., et al. (2019). Social-ecological and technological factors moderate the value of urban nature. Nat. Sustainabil. 2, 29–38. doi: 10.1038/s41893-018-0202-1
Kelly R. P., O’Donnell J. L., Lowell N. C., Shelton A. O., Samhouri J. F., Hennessey S. M., et al. (2016). Genetic signatures of ecological diversity along an urbanization gradient. PeerJ 4, e2444. doi: 10.7717/peerj.2444
Kennedy L. A., Juanes F., El-Sabaawi. R. (2018). Eelgrass as valuable nearshore foraging habitat for juvenile pacific salmon in the early marine period. Mar. Coast. Fish. 10, 190–203. doi: 10.1002/mcf2.10018
King County (2009). Puget Sound Stream Benthos: Monitoring Status and Data Management. Prepared by Colton J., Henderson D., Lester D., Simmonds J., Water and Land Resources Division. Seattle, Washington.
Koch E. W., Barbier E. B., Silliman B. R., Reed D. J., Perillo G. M., Hacker S. D., et al. (2009). Non-linearity in ecosystem services: temporal and spatial variability in coastal protection. Front. Ecol. Environ. 7, 29–37. doi: 10.1890/080126
Lean C. H. (2021). Invasive species increase biodiversity and, therefore, services: An argument of equivocations. Conserv. Sci. Pract. 3 (12), e553. doi: 10.1111/csp2.553
Lee T. S., Toft J. D., Cordell J., Dethier M. N., Adams J., Kelly. R. P. (2018). Quantifying the effectiveness of shoreline armoring removal on coastal biota of puget sound. PeerJ 6, e4275. doi: 10.7717/peerj.4275
Legendre P., Fortin M.-J. (2010). Comparison of the mantel test and alternative approaches for detecting complex multivariate relationships in the spatial analysis of genetic data. Mol. Ecol. Resour. 10, 831–844. doi: 10.1111/j.1755-0998.2010.02866.x
Lepczyk C. A., Aronson M. F. J., Evans K. L., Goddard M. A., Lerman S. B., MacIvor. J. S. (2017). Biodiversity in the city: Fundamental questions for understanding the ecology of urban green spaces for biodiversity conservation. BioScience 67, 799–807. doi: 10.1093/biosci/bix079
Manson S., Schroeder J., Van Riper D., Kugler T., Ruggles S. (2021). IPUMS national historical geographic information system: Version 16.0 [2020 census: P.L. 94-171 redistricting data summary file] (Publication no. IPUMS, Minneapolis, MN, USA. doi: 10.18128/D050.V16.0
McClelland J. W., Valiela I., Michener R. H. (1997). Nitrogen-stable isotope signatures in estuarine food webs: a record of increasing urbanization in coastal watersheds. Limnol. Oceanography 42, 930–937. doi: 10.4319/lo.1997.42.5.0930
McGlathery K. J. (2001). Macroalgal blooms contribute to the decline of seagrass in nutrient-enriched coastal waters. Journal of Phycology 37 (4), 453–456. doi: 10.1046/j.1529-8817.2001.037004453.x
McKinley A. C., Miskiewicz A., Taylor M. D., Johnston E. L. (2011). Strong links between metal contamination, habitat modification and estuarine larval fish distributions. Environmental Pollution 159, 1499–1509. doi: 10.1016/j.envpol.2011.03.008
McLeod K. L., Leslie H. (2009). Ecosystem-based management for the oceans. island press (Washington, D.C: Island Press).
McQuatters-Gollop A., Mitchell I., Vina-Herbon C., Bedford J., Addison P. F. E., Lynam C. P., et al. (2019). From science to evidence – how biodiversity indicators can be used for effective marine conservation policy and management. Front. Mar. Sci. 6. doi: 10.3389/fmars.2019.00109
MEA (2005). Millenium ecosystem assessment, ecosystems and human well-being: Scenarios Vol. Volume 2 (Washington: Island Press).
Momota K., Hosokawa S. (2021). Potential impacts of marine urbanization on benthic macrofaunal diversity. Sci. Rep. 11, 1–12. doi: 10.1038/s41598-021-83597-z
Moore J. W., Schindler D. E., Carter J. L., Fox J., Griffiths J., Holtgrieve G. W. (2007). Biotic control of stream fluxes: spawning salmon drive nutrient and matter export. Ecology 88, 1278–1291. doi: 10.1890/06-0782
Morley S. A., Karr J. R. (2002). Assessing and restoring the health of urban streams in the puget sound basin. Conserv. Biol. 16, 1498–1509. doi: 10.1046/j.1523-1739.2002.01067.x
Morley S. A., Toft J. D., Hanson K. M. (2012). Ecological Effects of Shoreline Armoring on Intertidal Habitats of a Puget Sound Urban Estuary. Estuaries and Coasts 35, 774–784. doi: 10.1007/s12237-012-9481-3
Morse C. C., Huryn A. D., Cronan C. (2003). Impervious Surface Area as a Predictor of the Effects of Urbanization on Stream Insect Communities in Maine, U.S.A. Environmental Monitoring and Assessment 89, 95–127. doi: 10.1023/A:1025821622411
Munsch S. H., Cordell J. R., Toft J. D. (2015). Effects of seawall armoring on juvenile pacific salmon diets in an urban estuarine embayment. Mar. Ecol. Prog. Ser. 535, 213–229. doi: 10.3354/meps11403
Munsch S. H., Cordell J. R., Toft J. D. (2017). Effects of shoreline armouring and overwater structures on coastal and estuarine fish: Opportunities for habitat improvement. J. Appl. Ecol. 54 (5), 1373–1384. doi: 10.1111/1365-2664.12906
Nicastro A., Onoda Y., Bishop M. J. (2012). Direct and indirect effects of tidal elevation on eelgrass decomposition. Mar. Ecol. Prog. Ser. 456, 53–62. doi: 10.3354/meps09635
Nicholson E., Watermeyer K. E., Rowland J. A., Sato C. F., Stevenson S. L., Andrade A., et al. (2021). Scientific foundations for an ecosystem goal, milestones and indicators for the post-2020 global biodiversity framework. Nat. Ecol. Evol. 5, 1338–1349.
Niemelaä J., Breuste J. H., Guntenspergen G., McIntyre N. E., Elmqvist T., James P. (Eds.) (2011). Urban ecology: Patterns, processes, and applications (New York, USA: Oxford University Press).
Oksanen J., Blanchet F. G., Kindt R., Legendre P., Minchin P. R., O’Hara R. B., et al. (2015). Vegan: Community ecology package (Helsinki, Finland: R package version 2).
OpenStreetMap contributors (2012) OpenStreetMap - Washington state roads. Available at: http://download.geofabrik.de/north-america/us/washington.html.
Pauly D., Christensen V. (1995). Primary production required to sustain global fisheries. Nature 374, 255–257. doi: 10.1038/374255a0
Peter K. T., Lundin J. I., Wu C., Feist B. E., Tian Z., Cameron J. R., et al. (2022). Characterizing the chemical profile of biological decline in stormwater-impacted urban watersheds. Environ. Sci. Technol. 56 (5), 3159–3169. doi: 10.1021/acs.est.1c08274
Pickett S. T., Burch W. R., Dalton S. E., Foresman T. W., Grove J. M., Rowntree R. (1997). A conceptual framework for the study of human ecosystems in urban areas. Urban. Ecosyst. 1, 185–199. doi: 10.1023/A:1018531712889
Piola R. F., Johnston E. L. (2008). Pollution reduces native diversity and increases invader dominance in marine hard-substrate communities. Diversity and Distributions 14(2), 329–342. doi: 10.1111/j.1472-4642.2007.00430.x
POC (2003). America’s living ocean: Charting a course for Sea change (Washington, D.C: A Report to the Nation).
PSNERP (2010). Puget sound basin PSNERP database. Puget Sound Nearshore Ecosystem Restoration Partnership, Olympia, WA. Downloaded 12 March 2012. http://wagda.lib.washington.edu/data/geography/wa_state/wageol/data/PS_PSNERP_CA_3.0.gdb.zip.
PSP (2008). Puget sound action agenda: Protecting and restoring the puget sound ecosystem by 2020. Puget Sound Partnership, Tacoma, WA, USA.
Puget Sound Regional Council (PSRC) (2015) 2015 regional macroeconomic forecast. Available at: http://www.psrc.org/data/forecasts/econdem/.
Puget Sound Stream Benthos (2015). Samples with T.A. and taxonomy (City of Seattle, King County, Pierce County, and Snohomish County). Available at: https://benthos.kingcounty.gov/https://benthos.kingcounty.gov/.
R Core Team (2014). R: A language and environment for statistical computing (Vienna, Austria: R Foundation for Statistical Computing). Available at: http://www.R-project.org/.
Rantz S. E., et al. (1992). Measurement and computation of streamflow: Volume 1. Measurement of stage and discharge. U.S. Geological Survey Water-Supply Paper 2175. U.S. Government Printing Office, Washington D.C.
Revised Code of Washington 90.71.300 Action Agenda – Goals and Objectives. Available at: https://app.leg.wa.gov/RCW/default.aspx?cite=90.71.300.
Ruckelshaus M., McClure M. (2007). Sound science: Synthesizing ecological and socioeconomic information about the puget sound ecosystem (Seattle, WA, USA: US Dept. of Commerce, National Oceanic and Atmospheric Administration (NMFS), Northwest Fisheries Science Center).
Samhouri J. F., Levin P. S. (2012). Linking land- and sea-based activities to risk in coastal ecosystems. Biol. Conserv. 145, 118–129. doi: 10.1016/j.biocon.2011.10.021
Samhouri J. F., Levin P. S., Ainsworth C. H. (2010). Identifying thresholds for ecosystem-based management (ed SJ bograd). PloS One 5, e8907. doi: 10.1371/journal.pone.0008907
Savage C., Leavitt P. R., Elmgren R. (2010). Effects of land use, urbanization, and climate variability on coastal eutrophication in the Baltic Sea. Limnology and Oceanography 55, 1033–1046. doi: 10.4319/lo.2010.55.3.1033
Schueler T. R., Fraley-McNeal L., Cappiella K. (2009). Is impervious cover still important? Rev. Recent Res. J. Hydrol. Eng. 14, 309–315. doi: 10.1061/(ASCE)1084-0699(2009)14:4(309)
Secretariat of the Convention on Biological Diversity (SCBD) (2012). Cities and biodiversity outlook (Montreal, Canada: Montreal), 64.
Simenstad C. A., Tanner C. D., Thom R. M., Conquest L. L. (1991). Estuarine habitat assessment protocol (Washington: Report to US Environmental Protection Agency, Region 10, Seattle).
Smith S. V., Swaney D. P., Talaue-Mcmanus L., Bartley J. D., Sandhei P. T., McLaughlin C. J., et al. (2003). Humans, hydrology, and the distribution of inorganic nutrient loading to the ocean. BioScience 53, 235–245. doi: 10.1641/0006-3568(2003)053[0235:HHATDO]2.0.CO;2
Sobocinski K. L., Cordell J. R., Simenstad. C. A. (2010). Effects of shoreline modifications on supratidal macroinvertebrate fauna on puget sound, Washington beaches. Estuaries. Coasts. 33, 699–711. doi: 10.1007/s12237-009-9262-9
Spirandelli D. J. (2014). Examining the effects of wastewater infrastructure on puget sound near-shore water quality across a gradient of urbanization (University of Washington. 164 p: Ph.D. Dissertation).
Steinberg P. D., Brett M. T., Bechtold J. S., Richey J. E., Porensky L. M., Smith S. N. (2010). The influence of watershed characteristics on nitrogen export to and marine fate in hood canal, Washington, USA. Biogeochemistry 106, 415–433. doi: 10.1007/s10533-010-9521-7
Steinman A. D., Lamberti G. A., Leavitt P. R. (2007). “Biomass and pigments of benthic algae,” in Methods in stream ecology. Eds. Hauer F. R., Lamberti G. A. (San Diego, California: Academic Press), Pages 357–380.
Stoms D. M., Davis F. W., Andelman S. J., Carr M. H., Gaines S. D., Halpern B. S., et al. (2005). Integrated coastal reserve planning: making the land-sea connection. Front. Ecol. Environ. 3, 429–436. doi: 10.1890/1540-9295(2005)003[0429:ICRPMT]2.0.CO;2
Stramma L., Schmidtko S., Levin L. A., Johnson G. C. (2010). Ocean oxygen minima expansions and their biological impacts. Deep. Sea. Res. Part I.: Oceanographic. Res. Papers. 57, 587–595. doi: 10.1016/j.dsr.2010.01.005
Strong J. A., Andonegi E., Bizsel K. C., Danovaro R., Elliott M., Franco A., et al. (2015). Marine biodiversity and ecosystem function relationships: The potential for practical monitoring applications. Estuarine. Coast. Shelf. Sci. 161, 46–64. doi: 10.1016/j.ecss.2015.04.008
Takeda L., Røpke I. (2010). Power and contestation in collaborative ecosystem-based management: The case of haida gwaii. Ecol. Econ. Special. Section.: Ecol. Distribution. Conflicts. 70, 178–188. doi: 10.1016/j.ecolecon.2010.02.007
Tallis H., Ferdana Z., Gray E. (2008). Linking terrestrial and marine conservation planning and threats analysis. Conserv. Biol. 22, 120–130. doi: 10.1111/j.1523-1739.2007.00861.x
Taylor S. L., Roberts S. C., Walsh C. J., Hatt B. E. (2004) Catchment urbanisation and increased benthic algal biomass in streams: linking mechanisms to management. Freshwater Biology 49, 835–851. doi: 10.1111/j.1365-2427.2004.01225.x
Tissot B. N., Walsh W. J., Hixon M. A. (2009). Hawaiian Islands marine ecosystem case study: Ecosystem- and community-based management in Hawaii. Coast. Manage. 37, 255–273. doi: 10.1080/08920750902851096
Todd P. A., Heery E. C., Loke L. H., Thurstan R. H., Kotze D. J., Swan C. (2019). Towards an urban marine ecology: characterizing the drivers, patterns and processes of marine ecosystems in coastal cities. Oikos 128, 1215–1242. doi: 10.1111/oik.05946
Toft J. D., Cordell J. R., Simenstad C. A., Stamatiou L. A. (2007). Fish Distribution, Abundance, and Behavior along City Shoreline Types in Puget Sound. North American Journal of Fisheries Management 27, 465–480. doi: 10.1577/M05-158.1
United Nations (UN) (2010). Strategic plan for biodiversity 2011-2020, including aichi biodiversity targets in: Decision x/2 (New York, NY: United Nations).
United Nations (UN) (2014). World urbanization prospects: The 2014 revision, highlights (ST/ESA/SER.A/352) (New York, USA: Department of Economic and Social Affairs, Population Division).
U.S. Environmental Protection Agency & U.S. Geological Survey (2005). National hydrography dataset plus – NHDPlus. U.S. Environmental Protection Agency & U.S. Geological Survey, Sioux Falls, SD, USA.
Vitousek P. M., Ehrlich P. R., Ehrlich A. H., Matson P. A. (1986). Human appropriation of the products of photosynthesis. BioScience 36, 368–373. doi: 10.2307/1310258
Vonshak M., Gordon D. M. (2015). Intermediate disturbance promotes invasive ant abundance. Biol. Conserv. 186, 359–367. doi: 10.1016/j.biocon.2015.03.024
Walsh C. J., Roy A. H., Feminella J. W., Cottingham P. D., Groffman P. M., Morgan R. P., et al (2005). The urban stream syndrome: current knowledge and the search for a cure. Journal of the North American Benthological Society 24, 706–723. doi: 10.1899/04-028.1
Wipfli M. S., Baxter C. V. (2010). Linking ecosystems, food webs, and fish production: Subsidies in salmonid watersheds. Fisheries 35, 373–387. doi: 10.1577/1548-8446-35.8.373
Wolman M. G. (1954). A method of sampling coarse river-bed material. Eos, Transactions American Geophysical Union 35, 951–956. doi: 10.1029/TR035i006p00951
Keywords: land-sea, ecosystem structure, ecosystem function, biodiversity, urban gradient, Pacific salmon
Citation: Samhouri JF, Shelton AO, Williams GD, Feist BE, Hennessey SM, Bartz K, Kelly RP, O’Donnell JL, Sheer M, Stier AC and Levin PS (2022) How much city is too much city? Biodiversity and ecosystem functioning along an urban gradient at the interface of land and sea. Front. Mar. Sci. 9:931319. doi: 10.3389/fmars.2022.931319
Received: 28 April 2022; Accepted: 18 August 2022;
Published: 16 September 2022.
Edited by:
Andrea Belgrano, Swedish University of Agricultural Sciences, SwedenReviewed by:
Darcy McNicholl, Fisheries and Oceans Canada, CanadaLucia Fanini, Hellenic Center for Marine Research, Greece
Copyright © 2022 Samhouri, Shelton, Williams, Feist, Hennessey, Bartz, Kelly, O’Donnell, Sheer, Stier and Levin. This is an open-access article distributed under the terms of the Creative Commons Attribution License (CC BY). The use, distribution or reproduction in other forums is permitted, provided the original author(s) and the copyright owner(s) are credited and that the original publication in this journal is cited, in accordance with accepted academic practice. No use, distribution or reproduction is permitted which does not comply with these terms.
*Correspondence: Jameal F. Samhouri, amFtZWFsLnNhbWhvdXJpQG5vYWEuZ292