- 1Department of Marine Sciences and Convergent Technology, Hanyang University, Ansan, South Korea
- 2Department of Oceanography, Pusan National University, Busan, South Korea
- 3Oceanic Climate & Ecology Research Division, National Institute of Fisheries Science, Busan, South Korea
- 4Marine Environmental Research Center, Korea Institute of Ocean Science and Technology, Busan, South Korea
The physiological status of phytoplankton, used to determine the quantity and quality of basic food sources in marine ecosystems, can change rapidly due to ambient environmental conditions (e.g., light, temperature, and nutrients). To understand the physiological characteristics of phytoplankton, the phytoplankton community composition, pigment concentration, primary production, and pigment production rate were estimated at 100% and 1% light depths in the Philippine Sea during the summer of 2019. The predominant phytoplankton classes at both light depths were Prochlorococcus and Synechococcus during the study period. Pigment concentrations, except for photoprotective pigment concentrations (i.e., diadinoxanthin and zeaxanthin), were significantly higher (t-test, p<0.05) at 1% light depth to increase the light-harvesting efficiency. The production rates of these pigments had a weak correlation with primary production at 100% light depth, whereas they showed a strong positive relationship at 1% light depth. Moreover, all photosynthetic pigments had a significantly faster turnover rate at 100% light depth compared with 1% light depth to obtain light energy to repair PSII subunits damaged by strong light. This suggests that the phytoplankton community, especially cyanobacteria (Prochlorococcus and Synechococcus), could use light energy absorbed by newly produced photosynthetic pigments for repairing photoinhibition-damaged PSII as well as for production activity. A further study on photosynthetic pigments responding to light conditions must be conducted for a better understanding of the physiological conditions of phytoplankton.
Introduction
In marine ecosystems, phytoplankton, as the primary producers, play a key role in supporting upper trophic organisms by providing basic food sources through their photosynthesis. Phytoplankton respond sensitively to ambient environmental conditions, causing variations in their physiological characteristics that determine the quality and quantity of food materials available for upper trophic organisms (Kang et al., 2017; Lee et al., 2020). Among various environmental factors, light, essential for photosynthesis, is one of the important factors that control the physiological status of phytoplankton. Phytoplankton pigments absorb light energy for their photosynthesis, and these pigments perform another physiological function called “photoprotection,” protecting them from strong light and ultraviolet radiation (Ting et al., 2002; Eisner et al., 2003; Descy et al., 2009; Kirk, 2011; Croce and Van Amerongen, 2014). Generally, phytoplankton produce photoprotective pigments to protect their cells during photosynthesis under high light intensity, whereas they accumulate photosynthetic pigments for light-harvesting in low light conditions (Falkowski and Owens, 1980; Moore et al., 1995; Lutz et al., 2003; Roy et al., 2011). Thus, investigating variations in phytoplankton pigments under different light conditions provides useful information on the phytoplankton physiological responses that may be closely related to photosynthesis.
The Philippine Sea is located on the edge of the Northwestern Pacific Ocean and belongs to the east marginal sea of the Philippines. The Philippine Sea is an oligotrophic ocean, which is affected by a Pacific warm pool that is an oligotrophic water mass with a high sea-surface temperature (>29 °C) and low sea-surface salinity (<34.5) (Mackey et al., 1995; Messié and Radenac, 2006; Zhao et al., 2010). The predominant phytoplankton group in warm oligotrophic oceans is mostly pico-cell-sized, less than 2 µm, and mainly comprises Prochlorococcus, Synechococcus, and picoeukaryotes (Zhao et al., 2010). These small phytoplankton groups have distinct vertical distributions within the euphotic layer (Partensky et al., 1996; Hickman et al., 2009; Hickman et al., 2010; Zhao et al., 2010). Synechococcus mainly inhabits near-surface waters, whereas Prochlorococcus and picoeukaryotes are observed throughout the euphotic layer, with maximal biomass near the subsurface (Partensky et al., 1996; Hickman et al., 2009; Hickman et al., 2010; Zhao et al., 2010).
In oligotrophic oceans, including the Philippine Sea, light may be an important factor in determining the vertical distribution of phytoplankton, including Prochlorococcus and Synechococcus, along with the light gradient (Claustre and Marty, 1995; Partensky et al., 1999; Ting et al., 2002; Agustí, 2004). To investigate the influence of light on phytoplankton vertical distributions, the relationships between light gradients and phytoplankton community structures have been examined using various models and field observations (Sathyendranath and Platt, 2007; Hickman et al., 2009; Hickman et al., 2010; Luimstra et al., 2020). According to these previous studies, the photosynthetic pigment composition contributes to determining the phytoplankton community compositions in different light environments (Sathyendranath and Platt, 2007; Hickman et al., 2009; Hickman et al., 2010; Luimstra et al., 2020). Additionally, Ting et al. (2002) described how Prochlorococcus is particularly efficient in absorbing enriched blue light at depth compared with Synechococcus, and this light absorption ability gives Prochlorococcus a competitive advantage under low light conditions. Although nutrients are also an important factor in the survival and competition of phytoplankton, Yun et al. (2020) observed a weak impact of nutrient supplies in mesoscale eddies on phytoplankton community composition in the Philippine Sea. This light adaptation in photosynthetic pigments determines the competitive advantages of each phytoplankton group, and it may contribute to the phytoplankton community structure at different depths in oligotrophic oceans. While our understanding of the relationship between photosynthetic pigments and the community structure of phytoplankton is improving, the correlation between these pigments and photosynthetic activity depending on light conditions remains unclear. However, understanding this correlation is essential in the context of projected warming oceans that would possess significantly stratified water conditions, like the Philippine Sea. In this context, light conditions would be the most important environmental factor controlling the physiological properties of phytoplankton in warm oceans. Therefore, our research question is how the variation in light conditions affects the photosynthetic activity and photophysiological characteristics of phytoplankton. We investigated the phytoplankton community composition, pigment concentration, primary production, and pigment production rate in different light environments in the Philippine Sea during the summer of 2019. The specific objectives of this study are (1) to estimate the phytoplankton community composition, pigment concentration, primary production, and pigment production rate in different light environments; and (2) to investigate the relationships between primary production and pigment production rate to discuss the light adaptation strategies and physiological states of phytoplankton.
Materials and methods
Seawater sampling
Our study was conducted in the Philippine Sea, in the Northwestern Pacific Ocean, during the Korean R/V “ISABU” cruise from 27 August to 16 September 2019. Seawater samples were collected at a total of five stations (Figure 1, Table 1) using a CTD (Conductivity–Temperature–Depth) mounted rosette sampler with 12 L Niskin bottles. Seawater samples for phytoplankton pigments and pigment production rates were collected at two light depths [(100 and 1% of surface PAR (photosynthetically active radiation)]. Temperature and salinity within the euphotic layer depth, defined as the depth with 1% intensity of surface PAR, were measured using CTD at the five stations. Surface PAR for determining the two light depths was measured using a PAR sensor (LI-COR underwater 4π light sensor) connected to a CTD-mounted rosette sampler. The mixed layer depth (MLD) was defined as the depth where the temperature difference from the reference depth was more than 0.2 °C, and the reference depth was 10 m in this study (de Boyer Montégut et al., 2004).
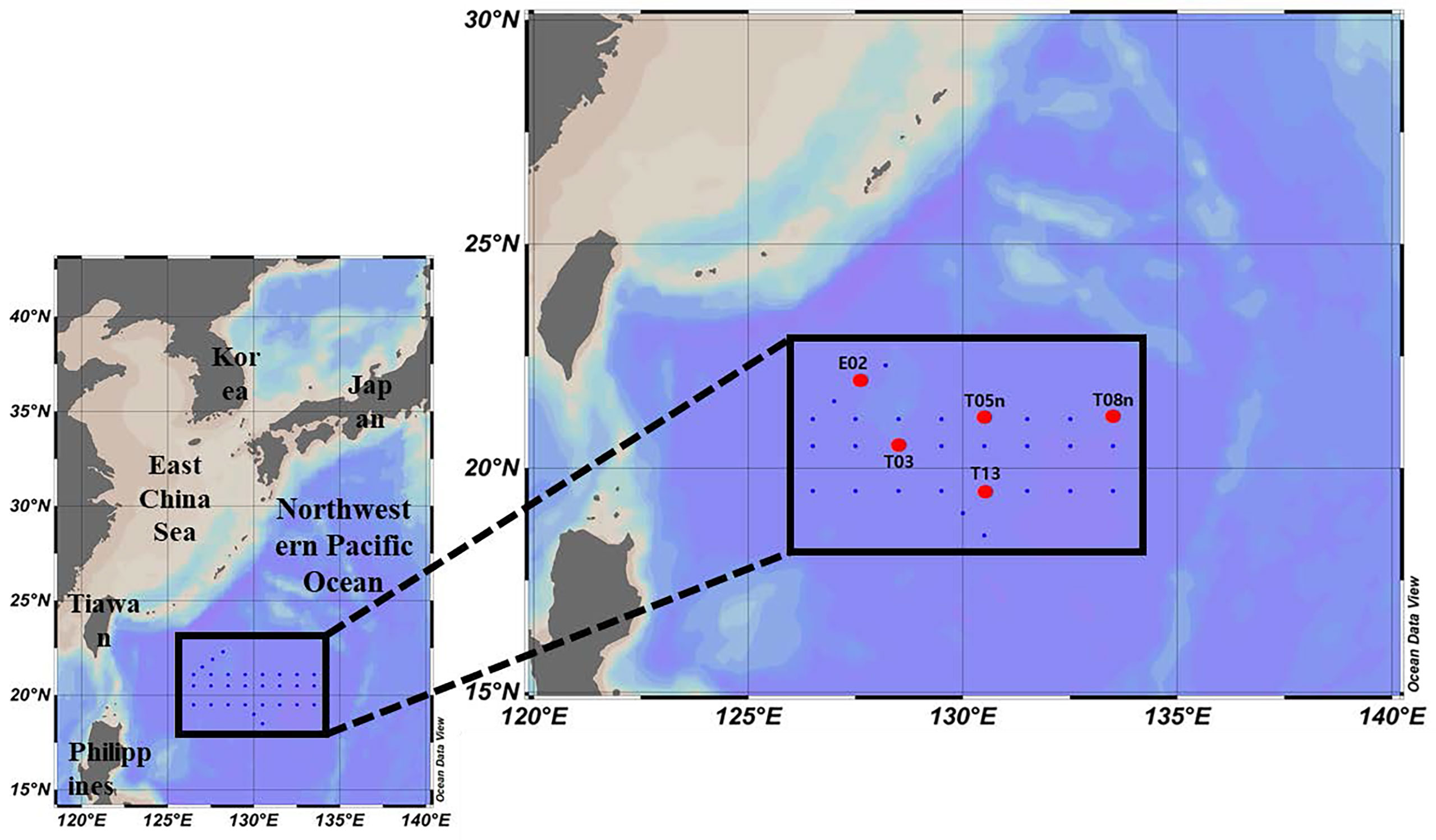
Figure 1 Sampling locations in the Philippine Sea, Northwestern Pacific Ocean (red circle: production station).
Phytoplankton pigment analysis
Each seawater sample (4 L) for phytoplankton pigments (hereafter, “pigments”) was filtered through 47 mm GF/F filters (Whatman) under low vacuum (<20 KPa) conditions. The filters were wrapped with aluminum foil and stored at −80 °C in the dark until pretreatment. In the laboratory, the extraction of the filtered pigment samples was conducted using 4 ml of 100% acetone and 100 µl of internal standard (IS, cantaxanthin) at 4 °C overnight. To eliminate floating matter, the extracts were passed through a 0.2 µm syringe filter (Advantec, hydrophobic). Then, 300 µl of distilled water (DW) was added to the filtrates to achieve optimal resolution during pigment analysis. In this study, we analyzed eleven phytoplankton pigments: fucoxanthin (Fuco), peridinin (Peri), 19’-hexanoloxyfucoxanthin (Hex-fuco), 19’-butanoyloxyfucoxanthin (But-fuco), chlorophyll b (Chl b), monovinyl chlorophyll a (MVChl a), divinyl chlorophyll a (DVChl a), diadinoxanthin (Diadino), violaxanthin (Viola), prasinoxanthin (Pras), and zeaxanthin (Zea). The concentrations of all pigments were measured using a high-performance liquid chromatography (HPLC) system (Agilent Infinite 1260, Santa Clara, CA, USA), following the method of Zapata et al. (2000). The biomarker pigments were identified by comparing their retention times with those of eleven standards (DHI Water and Environment, Hørsholm, Denmark).
Phytoplankton community structure
The relative chlorophyll a (Chl a) biomass of eight phytoplankton communities (diatoms, dinoflagellates, prymnesiophytes, chrysophytes, chlorophytes, prasinophytes, Synechococcus, and Prochlorococcus) was estimated using the chemotaxonomy program CHEMTAX, described by Mackey et al. (1996). To separate the eight phytoplankton communities using CHEMTAX, we used initial pigment ratios (the ratios of pigment to MVChl a or DVChl a) in line with that of Miki et al. (2008), who conducted a study on phytoplankton dynamics in the Northwestern Pacific Ocean (Table S2). CHEMTAX analyses were performed separately for samples from 100% and 1% light depths.
Primary production of phytoplankton
To measure the primary production of phytoplankton, we used a carbon stable isotope (13C) tracer technique (Hama et al., 1983). The seawater samples collected at each depth were transferred to 1 L polycarbonate bottles covered with a screen filter (LEE filters; Garneau et al., 2007) to match each light condition. Then, labeled carbon (NaH13CO3, 99%, Cambridge Isotope Laboratories, Inc.) substrate, which corresponded to approximately 10% of the ambient dissolved inorganic carbon concentration (DIC), was added. After inoculating the substrate, one bottle collected from each depth was incubated in an acrylic incubator at a constant temperature maintained by continuously circulating underway seawater on deck under natural light for 4 to 5 h before noon. The incubated water samples were passed through pre-combusted 25 mm GF/F filters (Whatman) and stored at −20 °C until further pretreatment. In the laboratory, the filter samples for primary production were treated with HCl (36.5%–38.0%), fuming overnight to remove inorganic carbon (Hama et al., 1983). The particulate organic carbon (POC) concentrations and isotope abundances of the carbon were measured using the mass spectrometer (Finnigan Delta+XL) at the Stable Isotope Laboratory of the University of Alaska, Fairbanks, USA. The hourly primary production of phytoplankton was determined using an equation reported by Hama et al. (1983).
Pigment production rate
Studies on the pigment labeling method using 14C were conducted more than 20 years ago (Redalje and Laws, 1981; Goericke and Welschmeyer, 1993a; Goericke and Welschmeyer, 1993b), the main purpose being to estimate the phytoplankton growth rate. Goericke and Welschmeyer (1993a; 1993b) emphasized that labeling experiments should last 24 h to resolve the imbalance between the rates of pigment synthesis and cell growth of phytoplankton under a natural photocycle; whereas, Redalje and Laws (1981) determined that a balance between the specific activity of chl a carbon and total phytoplankton carbon appeared in incubations lasting 6 to 12 h based on data from laboratory cultures. However, the main purpose of using the pigment labeling method in this study was not to estimate the growth rate of phytoplankton but to understand the in situ photophysiological characteristics of phytoplankton. According to previous studies, phytoplankton can adjust their cellular pigment pool within a few hours in response to fluctuations in the light environment (Hitchcock, 1977; Redalje and Laws, 1981; Roy et al., 2011). The 13C-labeling method is widely used to estimate various physiological aspects of in situ phytoplankton (Hama et al., 1983; Ha et al., 2014; Min et al., 2019; Kang et al., 2021). Thus, a modified labeling method using 13C, with a relatively short incubation time (4–5 h), was adopted in this study.
For onboard incubation (4–5 h), labeled carbon (NaH13CO3) substrate was added to each seawater sample in 9 L polycarbonate bottles. Onboard incubation was performed using an acrylic incubator under natural light. After incubation, the samples were filtered through pre-combusted 47 mm GF/F filters (Whatman) and kept at −80°C in the dark until further pretreatment.
In the home laboratory, to extract the biomarker pigments, 100% acetone (4 ml) and IS (100 µl), as used for HPLC pigment analysis (section Phytoplankton pigment analysis), were added to the filter samples and stored at 4 °C in the dark overnight. The extracted biomarker pigments were purified using a 0.2 µm syringe filter (Advantec-hydrophobic). To obtain a detectable carbon isotope abundance when using the mass spectrometer (Finnigan Delta+XL), the pigments were analyzed using a 500 µl injection volume. The pigments were then collected separately based on their retention times from HPLC pigment analysis (section Phytoplankton pigment analysis) using a fraction collector (Agilent-G1364C, Santa Clara, CA, USA) connected to the HPLC system (Agilent Infinite 1260). These fraction processes were repeated three to four times for all pigments. The fractionated pigments were dried using nitrogen gas to remove the solvent, and their abundance of carbon was determined using the mass spectrometer (Finnigan Delta+XL) at the Stable Isotope Laboratory of the University of Alaska, Fairbanks, USA. The pigment production rate was calculated using an equation reported by Ha et al. (2014); Kang et al. (2021):
where ΔPPR is the amount of each carbon pigment photosynthetically produced during incubation, ais is the percentage of 13C atoms in each pigment compound of the incubated sample, ans is the percentage of 13C atoms in each pigment compound of the natural sample, aic is the percentage of 13C atoms in the 13C enriched inorganic carbon, and CCP is the carbon concentration of each pigment at the end of incubation.
To determine the relationship between the light conditions and the production rate for each pigment, excluding pigment concentration (i.e., phytoplankton biomass), the turnover rate of each pigment was calculated thus:
Statistical analysis
A Student’s t-test was performed to validate significant differences in the variables of phytoplankton physiological status (phytoplankton community structure, pigment concentration, ratio of pigment to carbon biomass, primary production, and pigment production rate) between 100% and 1% light depth. A Pearson’s correlation coefficient analysis was conducted to determine the relationship among the variables. These statistical analyses were carried out using SPSS 12.0 for Windows.
Results
Physical characteristics
For all stations, the MLD was 34–53 m (mean ± S. D. = 43.4 ± 6.9 m) (Figure 2, Table 1). The MLD at St. T05n was the lowest whereas it was deepest at St. T03. The euphotic layer depth going from 100% to 1% light depth was 56–130 m (94.2 ± 32.8 m) in this study. The euphotic layer depths (<70 m) at St. T08n and St. T03 were shallower than those at other stations. The MLD was shallower than the euphotic layer depth at all stations, which equated to non-mixing of water masses between 100% and 1% light depth during the study period. The temperatures were 28.97–29.85 °C (29.31 ± 0.36 °C) at 100% light depth and 21.15–26.60 °C (24.29 ± 2.10 °C) at 1% light depth during the study period. The temperatures at 1% light depth were higher at St. T08n and St. T03 than at the other stations. In comparison with temperature, salinity did not vary over a large range. The salinity ranged from 34.28 to 34.51 at 100% light depth and 34.73 to 34.99 at 1% light depth. The average salinity (34.44 ± 0.09) at 100% light depth was slightly lower than that (34.91 ± 0.11) at 1% light depth in this study.
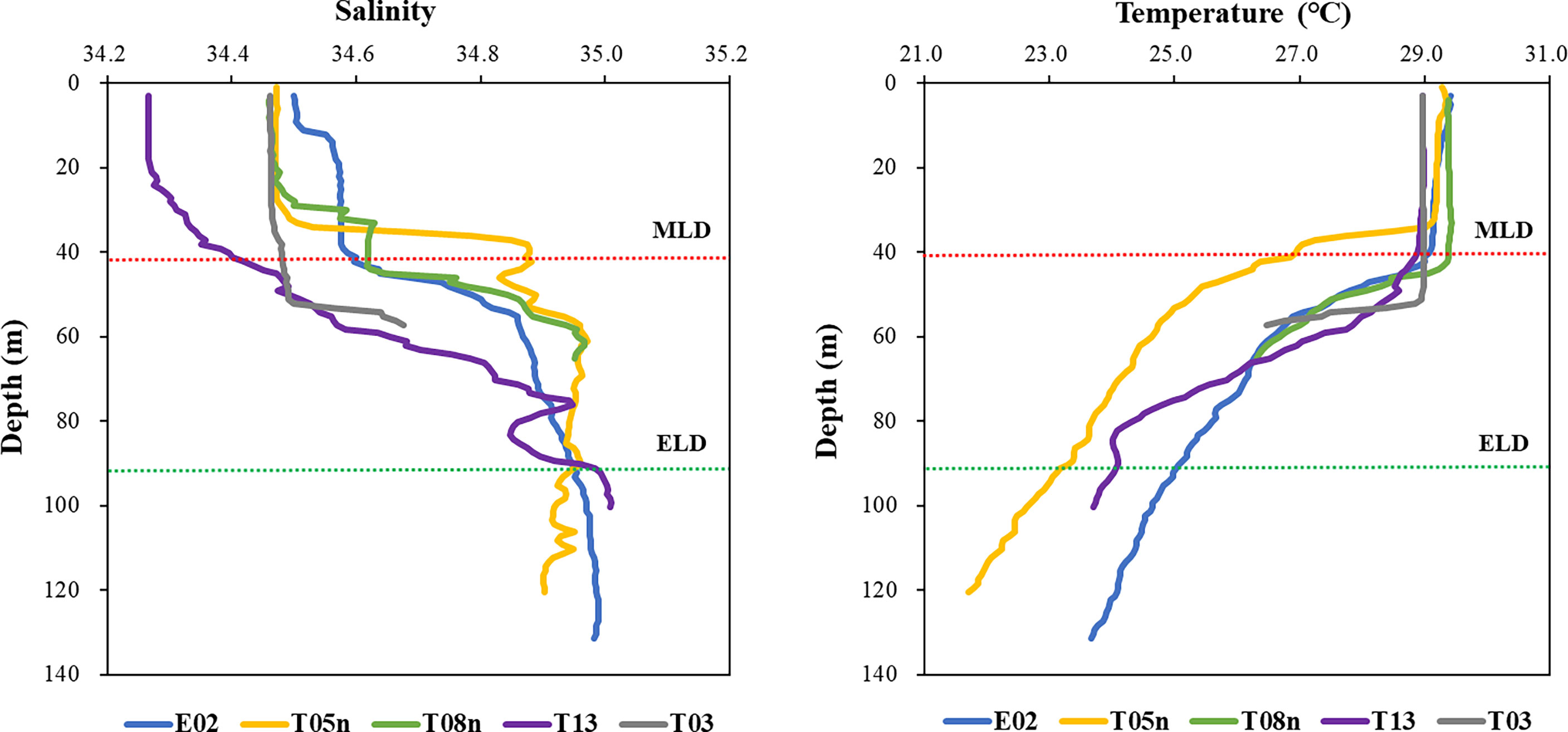
Figure 2 The profiles of salinity and temperature in the Philippines Sea, Northwestern Pacific Ocean. Red and green dash lines represent the average depths of mixed (MLD) and euphotic (ELD) layer, respectively.
Phytoplankton community structure
The phytoplankton community structure obtained from CHEMTAX analysis is shown in Figure 3. At all stations, the average contribution of Prochlorococcus to the total phytoplankton biomass at both light depths was highest (42.3 ± 9.2%), followed by Synechococcus (25.8 ± 17.9%), and then the other groups (below 10%). Prochlorococcus was predominant at both light depths, with an average contribution to the total phytoplankton biomass of 40.0% (S. D. = ± 10.9%) and 44.4% (S. D. = ± 7.8%) at 100% light depth and 1% light depth, respectively (Figure 3). The contribution of Synechococcus was 23.2%–39.6% and 3.1%–43.9% at 100% light depth and 1% light depth, respectively. The average contribution of Synechococcus to the total phytoplankton biomass decreased from 100% light depth (34.5 ± 6.5%) to 1% light depth (17.1 ± 22.1%). Compared to Synechococcus, chlorophytes had a high average contribution (16.7 ± 15.6%) to the total phytoplankton biomass at 1% light depth compared to that (2.2 ± 2.01%) at 100% light depth. Meanwhile, the average contribution of chrysophytes was 13.1% (± 6.1%) and 7.7% (± 5.4%) at 100% light depth and 1% light depth, respectively. Other phytoplankton groups had slight differences in biomass contribution between the two light depths.
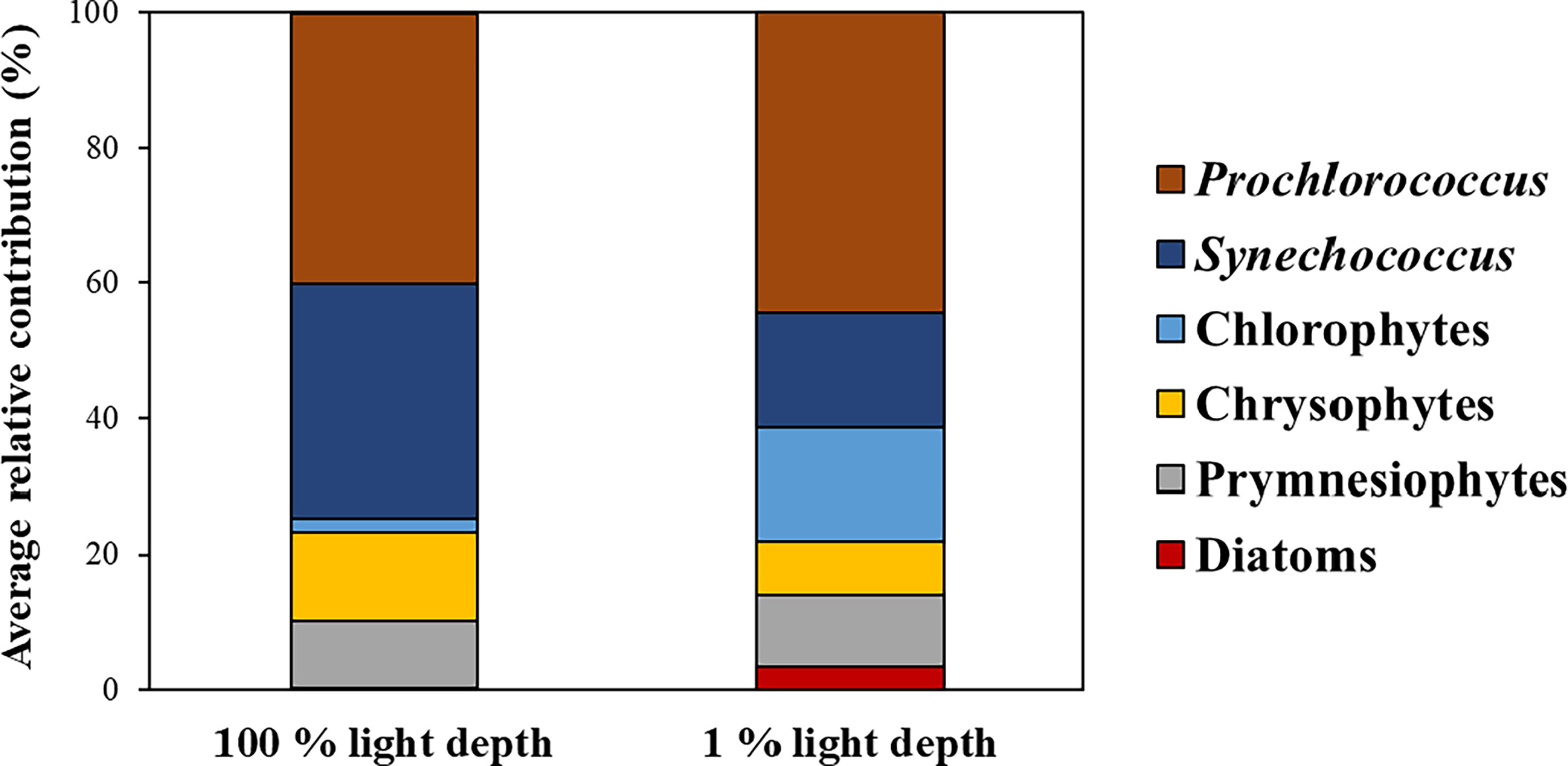
Figure 3 Average contributions of phytoplankton communities to total phytoplankton biomass between the two light depths.
Pigment concentration and the ratio of pigment to carbon biomass
In this study, Peri, Viola, and Pras were not detected between 100% and 1% light depth. Except for Zea and Diadino, the other pigments were significantly higher (t-test, p<0.05) at 1% light depth than at 100% light depth (Table 2). On average, the detected pigment concentrations ranged from 0.002–0.038 mg m−3 at 100% light depth, and Zea, MVChl a, and DVChl a showed the highest concentrations among these pigments. At 1% light depth, the average concentration of these pigments was 0.003–0.121 mg m−3, and Chl b had the highest concentration (0.121 ± 0.100 mg m−3) among the pigments. Furthermore, the average concentration of Chl b changed significantly (t-test, p<0.05) between the two light depths (100% and 1%), the largest such change among the pigments. Similar to the case for the average pigment concentration, the average ratio of pigment to carbon biomass was significantly (t-test, p<0.05) higher at 1% light depth than at 100% light depth (Table S3). The average value of all the ratios changed more than three times between the two light depths. In particular, But-fuco and Chl b had the highest average ratio changes among the pigments.
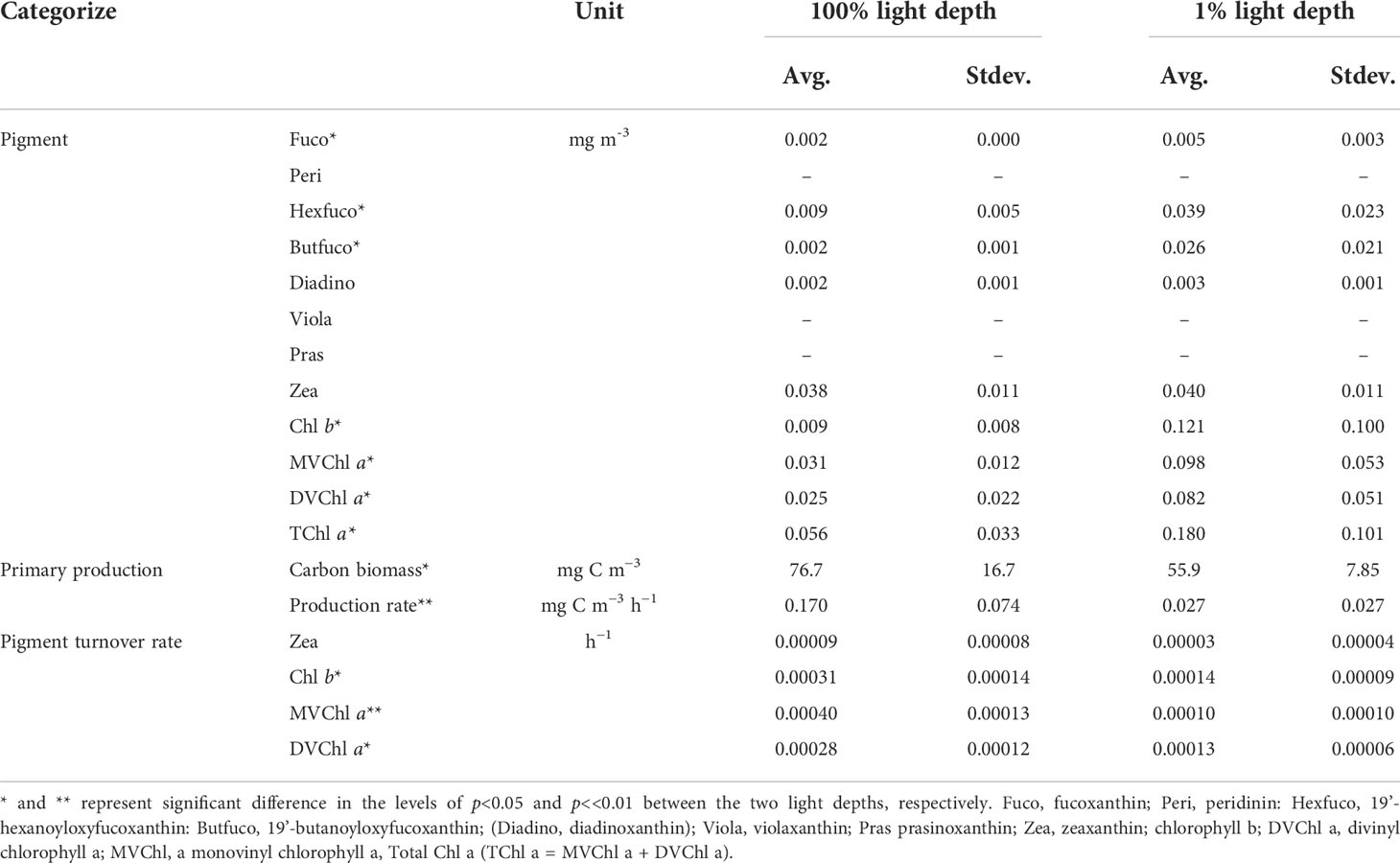
Table 2 Pigment concentrations, primary productions, pigment production rates, and pigment turnover rates between the 100% and 1% light depths in the Philippines Sea, Northwestern Pacific Ocean.
Primary production and pigment production rates
The magnitude of phytoplankton primary production varied between 100% and 1% light depth (Table 2). At 100% light depth, the primary production was 0.045–0.231 mg C m−3 h−1 (0.170 ± 0.074 mg C m−3 h−1). On the other hand, the primary production at 1% light depth was 0.002–0.071 mg C m−3 h−1 (0.027 ± 0.0027 mg C m−3 h−1), which was significantly lower (t-test, p<0.05) than that at 100% light depth. Meanwhile, the production rate of the photosynthetic pigments showed a different vertical distribution for each pigment (Figure 4). On average, the production rate of Chl b was 0.96–5.31 ng C m−3 h−1 (2.41 ± 1.72 ng C m−3 h−1) at 100% light depth and 0.67–29.75 ng C m−3 h−1 (12.67 ± 12.56 ng C m−3 h−1) at 1% light depth. The average production rate of DVChl a was 3.95 ng C m−3 h−1 (± 2.58 ng C m−3 h−1) at 100% light depth and 8.88 ng C m−3 h−1 (± 5.29 ng C m−3 h−1) at 1% light depth. The Chl b and DVChl a production rates were higher at 1% light depth than at 100% light depth, but these differences were not statistically significant (t-test, p >0.05). Compared to these two pigments, the average production rate of MVChl a was slightly higher at 100% light depth than at 1% light depth (Figure 4), with a vertical distribution similar to that of primary production. The MVChl a production rate was 2.69–24.66 ng C m−3 h−1 (9.53 ± 9.14 ng C m−3 h−1) and 0.13–20.02 ng C m−3 h−1 (7.20 ± 8.67 ng C m−3 h−1) at 100% light depth and 1% light depth, respectively. However, the difference in average MVChl a production rate between the two light depths was not significantly different (t-test, p >0.05).
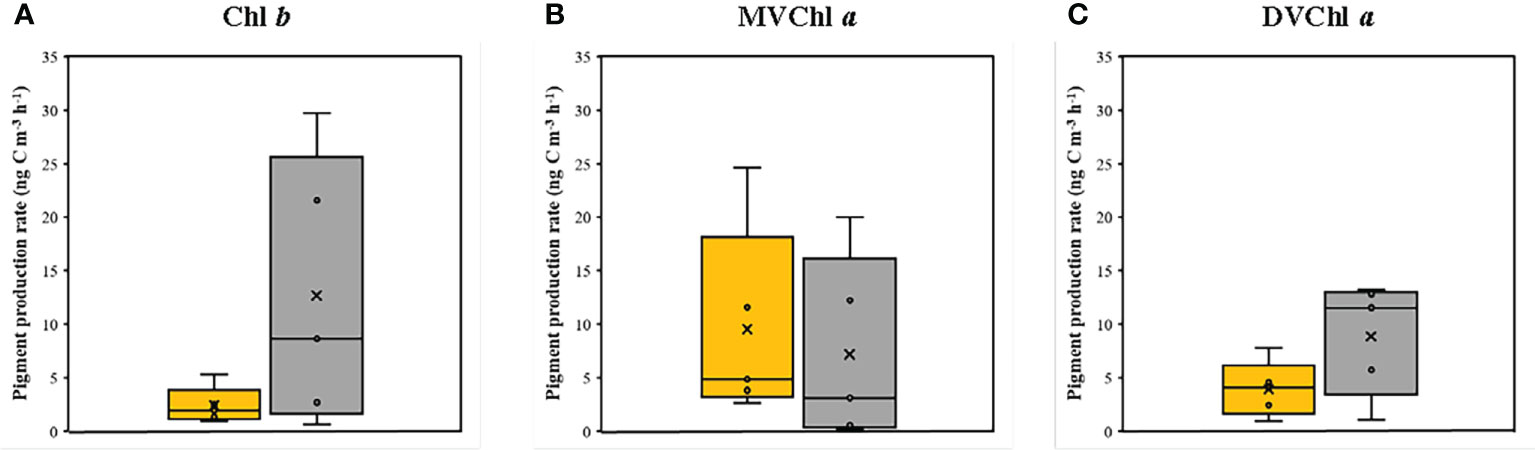
Figure 4 The production rates of (A) Chl b, (B) MVChl a and (C) DVChl a between the two light depths. Yellow and gray boxes represent 100 % and 1 % light depths, respectively. The abbreviations of pigment are shown in Table 2.
Unlike the distinct vertical distributions of each pigment production rate, the turnover rate of three photosynthetic pigments (Chl b, DVChl a, and MVChl a) was significantly higher at 100% light depth than at 1% light depth (Table 2). The turnover rate of Chl b ranged from 0.00018 to 0.00048 h−1 (0.00031 ± 0.00014 h−1) at 100% light depth and 0.00005 to 0.00022 h−1 (0.00014 ± 0.00009 h−1) at 1% light depth. In comparison, the turnover rate of MVChl a ranged from 0.00025 to 0.00054 h−1 (0.00040 ± 0.00013 h−1) at 100% light depth and 0.00000 to 0.00024 h−1 (0.00010 ± 0.00010 h−1) at 1% light depth. The DVChl a turnover rate was 0.00012–0.00024 h−1 (0.00028 ± 0.00012 h−1) and 0.00007–0.00020 h−1 (0.00013 ± 0.00006 h−1) at 100% light depth and 1% light depth, respectively. Among these pigments, MVChl a had the largest difference (>4-fold) in the turnover rate between the different light depths.
The relationship between primary production and photosynthetic pigment production varied with light depth (Figure 5). At 100% light depth, the production rate of Chl b, MVChl a, and DVChl a had a weak correlation (r2 = 0.3697, 0.0184, and 0.002 for Chl b, MVChl a, and DVChl a, respectively) with primary production. On the other hand, the production rate of these pigments had a positive correlation (r2 = 0.8415, 0.9604, and 0.4221 for Chl b, MVChl a, and DVChl a, respectively) with primary production at 1% light depth. In particular, the Chl b and MVChl a production rates had significant relationships (Pearson correlation, p<0.05) with primary production compared with the DVChl a production rate.
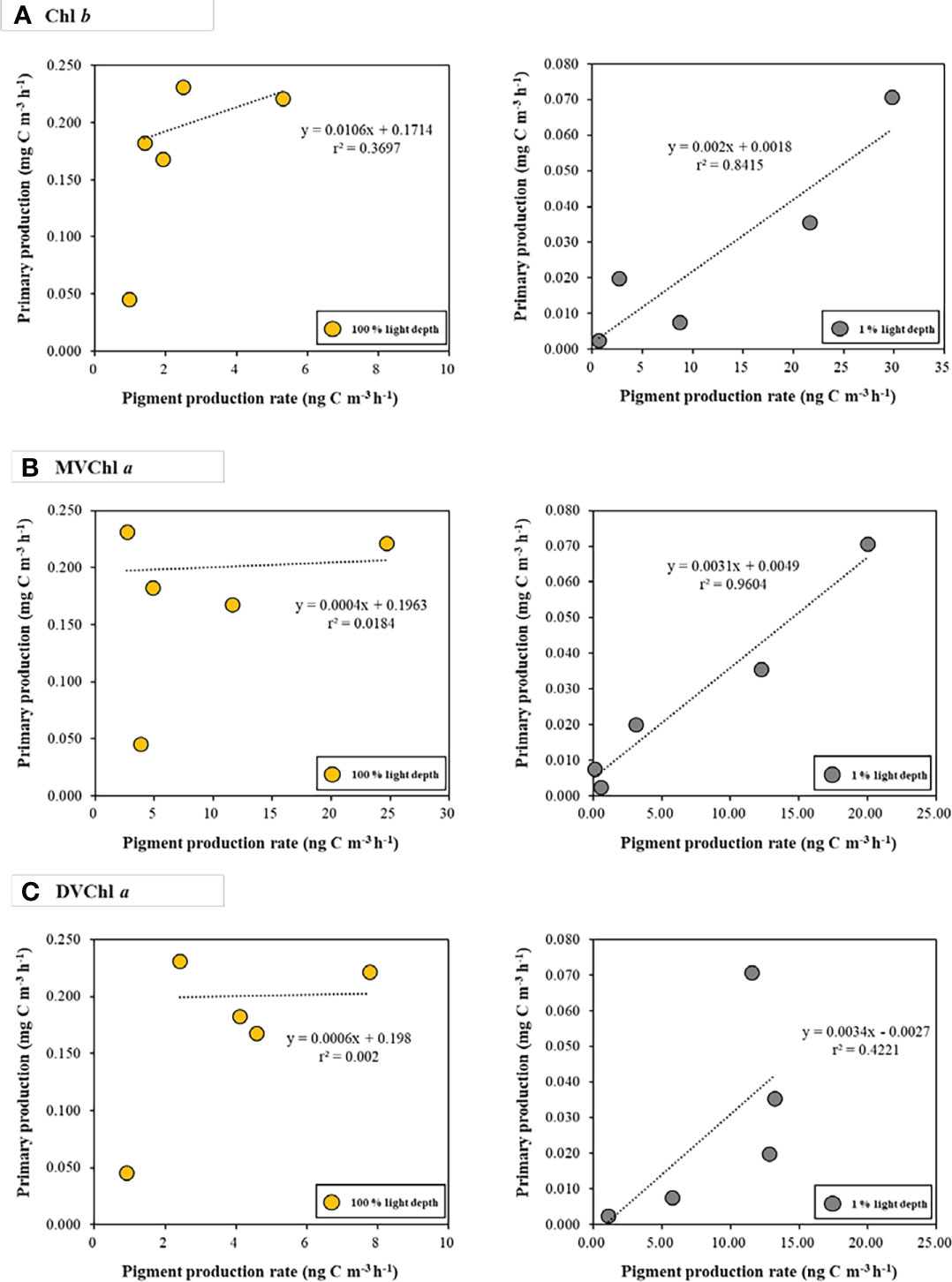
Figure 5 The relationships between photosynthetic pigment production rates (A) Chl b; (B) MVChl a; (C) DVChl a and primary production at the different light depths. Yellow and gray dots represent 100 % and 1 % light depths, respectively.
Discussion
Vertical distribution characteristics of phytoplankton community
During the research period, strong stratification existed within the euphotic layer in the Philippine Sea, Northwestern Pacific Ocean (Figure 2). This suppressed vertical mixing between 100% and 1% light depth, causing phytoplankton at each depth to be constantly exposed to light. This affected the phytoplankton physiological characteristics (i.e., the phytoplankton community, pigment concentration, primary production, and pigment production rate). Thus, we focused on the two light depths (100% and 1% light depth) to determine the effects of different light levels on phytoplankton physiological characteristics.
For each light depth, we observed different vertical distributions of Prochlorococcus, Synechococcus, and chlorophytes. On average, Prochlorococcus was predominant at both light depths, whereas Synechococcus showed a relatively higher contribution at the surface layer compared to 1% light depth and chlorophytes showed the opposite trend to Synechococcus (Figure 3). These vertical distributions have also been observed in other oligotrophic regions (Claustre and Marty, 1995; Veldhuis et al., 2005; Zhao et al., 2010). Zhao et al. (2010) reported that Prochlorococcus and Synechococcus had different abundances between the two light depths in the Philippine Sea, western North Pacific. Their results showed that Synechococcus mainly existed at 100% light depth, whereas Prochlorococcus was predominant at 1% light depth. At each light depth, the change in group abundance was associated with phytoplankton adaptation ability resulting from a pigment composition response to light environments (Figure 3, Falkowski and Owens, 1980; Claustre and Marty, 1995). Prochlorococcus and chlorophytes have Chl b,which is well known for its high efficiency in light absorption, with blue light penetrating to 200 m (Table S1, Claustre and Marty, 1995; Ting et al., 2002). This pigment allows Prochlorococcus and chlorophytes to efficiently harvest light energy in deep water columns where blue light is enriched; thus they can adapt well to surface layers and to deep layers (Morel et al., 1993: Claustre and Marty, 1995; Ting et al., 2002). In contrast to Prochlorococcus and chlorophytes, Synechococcus has a pigment capable of absorbing green light, meaning it predominates in near-surface waters (i.e., above 30 m) (Ting et al., 2002). Additionally, Synechococcus has light-harvesting phycobiliproteins, such as phycocyanin and phycoerythrin, with an absorption maximum at 590–670 nm (yellow-red light) and 535–600 nm (green-yellow light), respectively (Grossman et al., 1993; Ruan et al., 2018). Hence, Synechococcus is more suited to 100% light depth than 1% light depth.
Vertical distribution characteristics of primary production and pigment production
In addition to the phytoplankton community, pigment concentration and primary production also showed distinct vertical distributions between the two light depths (Table 2). Except for photoprotective pigments, the average concentration of photosynthetic pigments was significantly higher (t-test, p<0.05) at 1% light depth than at 100% light depth. In contrast, the average carbon biomass of phytoplankton was observed to be significantly higher (t-test, p<0.05) at 100% light depth than at 1% light depth. These results suggest that phytoplankton have distinct photophysiological states in different light environments. Falkowski and Owens (1980); Lutz et al. (2003), and Shibata et al. (2010) reported that phytoplankton adjust their pigment concentration depending on light conditions. Under low light conditions, phytoplankton increase their cellular photosynthetic pigment content to harvest light energy (Falkowski and Owens, 1980; Prezelin and Matlick, 1980; Richardson et al., 1983; Ramus, 1990; Falkowski and Raven, 2013), and this physiological response leads to an increase in pigment to carbon biomass ratio (Pig/C ratio) (Colijn et al., 1990; Veldhuis and Kraay, 1990; Moore et al., 1995; Lutz et al., 2003; Roy et al., 2011). Our results on Pig/C ratios are similar, depending on light conditions (Table S1). In addition, Chl b, DVChl a, and MVChl a were major photosynthetic pigments in the predominant phytoplankton groups (Prochlorococcus, Synechococcus, and chlorophytes) in this study; thus, the Pig/C ratios of these pigments can indicate their photophysiological states. Based on previous studies and our results, it can be inferred that the three major groups in the Philippine Sea increased their Pig/C ratios to harvest light energy under low light conditions. The average primary production was more than six times higher (t-test, p<0.01) at 100% light depth than at 1% light depth (Table 2). This large difference in primary production may be associated with environmental conditions such as light intensity and nutrients (Pabi et al., 2008; Tremblay et al., 2015; Lee et al., 2019; Zheng et al., 2021). Prior to this study, Yun et al. (2020) observed that picophytoplankton, the presence of which indicates a nutrient-deficient state, were the predominant phytoplankton groups despite the occurrence of mesoscale eddies supplying nutrients to the euphotic layer in the Philippine Sea. Our results on the phytoplankton community also indicated that picophytoplankton, containing Prochlorococcus and Synechococcus, was a major phytoplankton group in the Philippine Sea, suggesting that nutrients were limited at both light depths. Indeed, Pai and Chen (1994) did not detect significant concentrations of major nutrients (phosphate and nitrate) above the thermocline (approximately 250 m) at similar stations in the Philippine Sea. Moreover, Wong et al. (1995) reported that the Philippine Sea has characteristics of oligotrophic water, nearly devoid of nutrients at the top 200 m of the water column. Therefore, primary production between the two light depths is regulated by light intensity rather than nutrient conditions in the Philippine Sea during this research period.
Based on our results, light intensity seems to play a key role in determining the phytoplankton community, photosynthetic pigment concentration, and primary production. However, it remains unclear how phytoplankton produce their pigments for light energy absorption under different light conditions. We estimated the production rates for several major photosynthetic pigments in predominant phytoplankton groups at both light depths and investigated the relationships between pigment production rates and primary production. For each light depth, Chl b and DVChl a had high production rates at 1% light depth, whereas the MVChl a production rate was high at 100% light depth (Figure 4). Concerning the relationships between photosynthetic pigment production rate and primary production, we observed positive correlations only for 1% light depth (Figure 5). The fact that, generally, the pigment concentration increases under low light conditions is well recognized as a physiological response of phytoplankton used to harvest light energy (Falkowski and Owens, 1980; Colijn et al., 1990; Veldhuis and Kraay, 1990; Moore et al., 1995; Lutz et al., 2003; Shibata et al., 2010; Roy et al., 2011; Falkowski and Raven, 2013). Additionally, Colijn et al. (1990) reported a positive linear correlation (r2 = 0.81) between MVChl a and primary production within the euphotic layer in the German Bight. Liu X et al. (2019) observed that surface MVChl a concentrations had a significantly positive correlation (Spearman, r = 0.41, p<0.01) with integrated primary production in the East China Sea. These previous results imply that photosynthetic pigments may be involved in the production activity of phytoplankton via light energy absorption. In this regard, a relatively high MVChl a production rate at 100% light depth differs from the common physiological response of phytoplankton to light conditions. Moreover, the weak correlation between photosynthetic pigment production rate and primary production in this study suggests that phytoplankton do not utilize the light energy absorbed through photosynthetic pigments for primary production only (Figures 4, 5). During photosynthesis, under high light conditions, excess light energy absorbed by chlorophylls generates reactive oxygen species (ROS), resulting in damaged photosystem II (PSII), which is well known as photoinhibition (Liu J et al., 2019). Phytoplankton have mechanisms to protect their PSII from photoinhibition. The most important mechanism involves an increase in intracellular photoprotective pigment content (Siefermann-Harms, 1985; Roy et al., 2011; Falkowski and Raven, 2013). In general, phytoplankton exposed to high irradiance have a relatively high concentration of carotenoids, such as β-carotene and zeaxanthin, which do not transmit excitation energy to the reaction center of PSII, thus protecting the cell from excess light (Siefermann-Harms, 1985; Roy et al., 2011; Falkowski and Raven, 2013). Indeed, the concentration of zeaxanthin, the photoprotective pigment of the major phytoplankton groups (Prochlorococcus and Synechococcus), was similar between the surface layer and 1% light depth, unlike other pigments during the study period (Table 2), and the turnover rate of zeaxanthin in the surface layer (0.00009 h−1) was higher than that at 1% irradiance (0.00003 h−1) (Table 2). These results indicate the possibility that phytoplankton at 100% light depth may have been affected by photoinhibition during the study period. Another mechanism for photoinhibition is a replacement system that repairs the damaged PSII of phytoplankton (Figure S1, Komenda, 2000; Li et al., 2018; Liu J et al., 2019). Li et al. (2018) reviewed PSII photodamage mechanisms and showed that the turnover rate of PSII subunits, consisting of chlorophylls and proteins, increased above 0.02 h−1 under high light conditions (Komenda, 2000; Li et al., 2018; Komenda and Sobotka, 2019; Liu J et al., 2019). Our results on the pigment turnover rate were similar to those of previous studies. During this study period, the surface phytoplankton might have been exposed to high light intensity due to the strong stratification within the euphotic layer (Figure 2). As a result, all photosynthetic pigment turnover rates were significantly higher (t-test, p<0.05) at 100% light depth than at 1% light depth. This implies that phytoplankton quickly produce the photosynthetic pigments needed to acquire additional energy for the restoration of photodamaged PSII subunits under high light intensity (Table 2). In particular, the turnover rate of surface MVChl a was more than four times higher compared to the 1% light depth. This large difference may result in a relatively high MVChl a production rate at 100% light depth (Figure 4). Therefore, phytoplankton might utilize light energy absorbed by newly produced photosynthetic pigments not only for production activity but also for PSII subunit repair under high light intensity.
The production rates of Chl b and MVChl a had strong positive correlations (r2 = 0.8415 and r2 = 0.9604, respectively) with primary production at 1% light depth (Figure 5). These results indicate that Prochlorococcus and chlorophytes produce Chl b and MVChl a for harvesting light energy under low light conditions—this is in accordance with the general physiological response of phytoplankton to light intensity (Colijn et al., 1990; Lutz et al., 2003; Shibata et al., 2010; Roy et al., 2011; Liu X et al., 2019). Unlike Chl b and MVChl a, DVChl a production rates did not have a significant correlation (r2 = 0.4221, p >0.05) with primary production, which may be because of the physiological characteristics of Prochlorococcus containing DVChl a. Prochlorococcus increases Chl b content over DVChl a for light absorption efficiency in low light conditions, and this photophysiological response creates a high ratio of Chl b to DVChl a (Claustre and Marty, 1995; Moore et al., 1995; Ting et al., 2002; Fujiki et al., 2013). Our CHEMTAX result also showed that Prochlorococcus had a high Chl b to DVChl a ratio (1.21) at 1% light depth, indicating that this group changed their pigment composition for light-harvesting under low light intensity (Table S2). In this regard, Prochlorococcus might have used Chl b as a more important photosynthetic pigment than DVChl a during this research period. This light-harvesting strategy could contribute to the relatively weak correlation between the DVChl a production rate and primary production (Figure 5).
Summary and conclusions
In the Philippine Sea, phytoplankton had different relative abundances, pigment concentrations, and primary production between two light depths (100% and 1% light depths). This indicated that light intensity played a key role in determining phytoplankton physiological characteristics. For the different light depths, phytoplankton community composition may have been associated with the varying adaptability of phytoplankton to various light conditions. Except for photoprotective pigments, the photosynthetic pigment concentration was significantly higher at 1% light depth than at 100% light depth. These vertical distributions represent the physiological response of phytoplankton to increase their light-harvesting efficiency. Primary production showed different vertical distributions of photosynthetic pigment concentration between the two light depths. This indicated that light intensity was an important factor in controlling primary production during this research period. In addition to the photosynthetic pigment concentration and the primary production, pigment production rates also had different vertical distribution with light depths. The Chl b and DVChl a production rates were relatively high at 1% light depth, whereas the MVChl a production rate was slightly high at 100% light depth. In addition, we observed significantly fast turnover rates for Chl b, MVChl a, and DVChl a at 100% light depth. These results suggest that phytoplankton would have utilized the light energy absorbed by newly produced photosynthetic pigments not only for production activity but also for the repair of photodamaged PSII subunits under high light intensity. This replacement mechanism could cause a weak correlation between the pigment production rates with the primary production. In other words, optimal photosynthetic activity (i.e., primary production) was not performed by the phytoplankton community at 100% light depth during this research period, especially by cyanobacteria (Prochlorococcus and Synechococcus), which were the predominant phytoplankton classes. Indeed, the negative effect of strong light and warm water on the primary production of cyanobacteria has been previously reported (Kehoe et al., 2015). This suggests that the influence of light conditions on phytoplankton, which is a fundamental marine food source, must be considered to understand variation in marine ecosystems in warming ocean scenarios—such scenarios are expected to expose phytoplankton to high light intensity and warm water temperature conditions. Therefore, our results provide a benchmark for studying the photosynthetic activity of phytoplankton associated with their photosynthetic pigments and dependent on changing light conditions.
Data availability statement
The original contributions presented in the study are included in the article/Supplementary Material. Further inquiries can be directed to the corresponding authors.
Author contributions
CL, JK, and SL conceived the ideas and designed methodology. CL, HB, and DK performed field experiments. CL, JK, HB, YK, SP, and J-OM conducted lab experiment. CL and KJ performed data analysis. CL, JK, and SL wrote the first draft of the manuscript. JK and SL reviewed and edited previous versions of the manuscript. All authors listed have made a substantial, direct, and intellectual contribution to the work and approved it for publication.
Funding
This research was supported by the National Research Foundation of Korea (NRF) grant funded by the Korean government (MSIT; NRF-2019R1A2C1003515). Financial support for DK was provided by ‘Study on Northwestern Pacific Warming and Genesis and Rapid Intensification of Typhoon’, funded by the Ministry of Oceans and Fisheries (20220566). This research was supported by the “Development of assessment technology on the structure variations in marine ecosystem (R2022058)” funded by the National Institute of Fisheries Science (NIFS), Korea.
Acknowledgments
We are grateful to the captain, all of the crew, and researchers for their outstanding assistance during the Korean R/V “ISABU” cruise.
Conflict of interest
The authors declare that the research was conducted in the absence of any commercial or financial relationships that could be construed as a potential conflict of interest.
Publisher’s note
All claims expressed in this article are solely those of the authors and do not necessarily represent those of their affiliated organizations, or those of the publisher, the editors and the reviewers. Any product that may be evaluated in this article, or claim that may be made by its manufacturer, is not guaranteed or endorsed by the publisher.
Supplementary material
The Supplementary Material for this article can be found online at: https://www.frontiersin.org/articles/10.3389/fmars.2022.930690/full#supplementary-material
References
Agustí S. (2004). Viability and niche segregation of prochlorococcus and synechococcus cells across the central Atlantic ocean. Aquat. Microb. Ecol. 36, 53–59. doi: 10.3354/ame036053
Claustre H., Marty J. C. (1995). Specific phytoplankton biomasses and their relation to primary production in the tropical north Atlantic. Deep Res. Part I Oceanogr. Res. Pap. 42, 1475–1493. doi: 10.1016/0967-0637(95)00053-9
Colijn F., Villerius L., Rademaker M., Hammer K. D., Eberlein K. (1990). Changes in spatial distribution of primary production, photosynthetic pigments and phytoplankton species composition during two surveys in the German bight. Neth. J. Sea Res. 25 (1-2), 155–164. doi: 10.1016/0077-7579(90)90016-A
Croce R., Van Amerongen H. (2014). Natural strategies for photosynthetic light harvesting. Nat. Chem. Biol. 10, 492–501. doi: 10.1038/nchembio.1555
de Boyer Montégut C., Madec G., Fischer A. S., Lazar A., Iudicone D. (2004). Mixed layer depth over the global ocean: An examination of profile data and a profile-based climatology. J. Geophys. Res. Ocean 109, 1–20. doi: 10.1029/2004JC002378
Descy J. P., Sarmento H., Higgins H. W. (2009). Variability of phytoplankton pigment ratios across aquatic environments. Eur. J. Phycol. 44, 319–330. doi: 10.1080/09670260802618942
Eisner L. B., Twardowski M. S., Cowles T. J., Perry M. J. (2003). Resolving phytoplankton photoprotective: Photosynthetic carotenoid ratios on fine scales using in situ spectral absorption measurements. Limnol. Oceanogr. 48, 632–646. doi: 10.4319/lo.2003.48.2.0632
Falkowski P. G., Owens T. G. (1980). Light–shade adaptation: TWO STRATEGIES IN MARINE PHYTOPLANKTON. Plant Physiol. 66 (4), 592–595. doi: 10.1104/pp.66.4.592
Fujiki T., Matsumoto K., Saino T., Wakita M., Watanabe S. (2013). Distribution and photo-physiological condition of phytoplankton in the tropical and subtropical north pacific. J. Oceanogr. 69, 35–43. doi: 10.1007/s10872-012-0153-5
Garneau M. È., Gosselin M., Klein B., Tremblay J. É., Fouilland E. (2007). New and regenerated production during a late summer bloom in an Arctic polynya. Mar. Ecol.-Prog. Ser. 345, 13–26. doi: 10.3354/meps06965
Goericke R., Welschmeyer N. A. (1993a). The chlorophyll-labeling method: Measuring specific rates of chlorophyll a synthesis in cultures and in the open ocean. Limnol. Oceanogr. 38 (1), 80–95. doi: 10.4319/lo.1993.38.1.0080
Goericke R., Welschmeyer N. A. (1993b). The carotenoid-labeling method: measuring specific rates of carotenoid synthesis in natural phytoplankton communities. Mar. Ecol.-Prog. Ser. 98 (1), 157–171.
Grossman A. R., Schaefer M. R., Chiang G. G., Collier J. L. (1993). The phycobilisome, a light-harvesting complex responsive to environmental conditions. Microbiol. Rev. 57, 725–749. doi: 10.1128/mmbr.57.3.725-749.1993
Ha S. Y., La H. S., Min J. O., Chung K. H., Kang S. H., Shin K. H. (2014). Photoprotective function of mycosporine-like amino acids in a bipolar diatom (Porosira glacialis): Evidence from ultraviolet radiation and stable isotope probing. Diatom Res. 29, 399–409. doi: 10.1080/0269249X.2014.894945
Hama T., Miyazaki T., Iwakuma T., Takahashi M., Otsuki A., Ichimura S. (1983). Measurement of photosynthetic production of a marine phytoplankton population using a stable 13C isotope. Mar. Biol. 73 (1), 31–36. doi: 10.1007/BF00396282
Hickman A. E., Dutkiewicz S., Williams R. G., Follows M. J. (2010). Modelling the effects of chromatic adaptation on phytoplankton community structure in the oligotrophic ocean. Mar. Ecol. Prog. Ser. 406, 1–17. doi: 10.3354/meps08588
Hickman A. E., Holligan P. M., Moore C. M., Sharples J., Krivtsov V., Palmer M. R. (2009). Distribution and chromatic adaptation of phytoplankton within a shelf sea thermocline. Limnol. Oceanogr. 54, 525–536. doi: 10.4319/lo.2009.54.2.0525
Hitchcock G. L. The time course of photosynthetic adaptation, the growth response and variation in pigment, carbohydrate, and protein of Skeletonema costatum and Detonula confervacea to changes in light intensity. (1977).
Kang J. J., Joo H., Lee J. H., Lee J. H., Lee H. W., Lee D., et al. (2017). Comparison of biochemical compositions of phytoplankton during spring and fall seasons in the northern East/Japan Sea. Deep-Sea Res. Part II-Top Stud. Oceanogr., 143:73–81. doi: 10.1016/j.dsr2.2017.06.006
Kang J. J., Min J. O., Kim Y., Lee C. H., Yoo H., Jang H. K., et al. (2021). Vertical distribution of phytoplankton community and pigment production in the yellow sea and the east china sea during the late summer season. Water 13 (23), 3321. doi: 10.3390/w13233321
Kehoe M., O’Brien K. R., Grinham A., Burford M. A. (2015). Primary production of lake phytoplankton, dominated by the cyanobacterium cylindrospermopsis raciborskii, in response to irradiance and temperature. Inland Waters 5 (2), 93–100. doi: 10.5268/IW-5.2.778
Komenda J. (2000). Role of two forms of the D1 protein in the recovery from photoinhibition of photosystem II in the cyanobacterium synechococcus PCC 7942. Biochim. Biophys. Acta-Bioenerg. 1457, 243–252. doi: 10.1016/S0005-2728(00)00105-5
Komenda J., Sobotka R. (2019). Chlorophyll-binding subunits of photosystem I and II: Biosynthesis, chlorophyll incorporation and assembly. Adv. Bot. Res. 91, 195–223. doi: 10.1016/bs.abr.2019.02.001
Lee D., Jeong J. Y., Jang H. K., Min J. O., Kim M. J., Youn S. H., et al. (2019). Comparison of particulate organic carbon to chlorophyll-a ratio based on the ocean color satellite data at the ieodo and socheongcho ocean research stations. J. Coast. Res. 90, 267–271. doi: 10.2112/SI90-033.1
Lee J. H., Kang J. J., Jang H. K., Jo N., Lee D., Yun M., et al. (2020). Major controlling factors for spatio-temporal variations in the macromolecular composition and primary production by phytoplankton in garolim and asan bays in the yellow Sea. Reg. Stud. Mar. Sci., 36:101269. doi: 10.1016/j.rsma.2020.101269
Li L., Aro E. M., Millar A. H. (2018). Mechanisms of photodamage and protein turnover in photoinhibition. Trends Plant Sci. 23, 667–676. doi: 10.1016/j.tplants.2018.05.004
Liu X., Laws E. A., Xie Y., Wang L., Lin L., Huang B. (2019). Uncoupling of seasonal variations between phytoplankton chlorophyll a and production in the East China Sea. J. Geophys. Res. Biogeosci. 124, 2400–2415. doi: 10.1029/2018JG004924
Liu J., Lu Y., Hua W., Last R. L. (2019). A new light on photosystem II maintenance in oxygenic photosynthesis. Front. Plant Sci. 10. doi: 10.3389/fpls.2019.00975
Luimstra V. M., Verspagen J. M. H., Xu T., Schuurmans J. M., Huisman J. (2020). Changes in water color shift competition between phytoplankton species with contrasting light-harvesting strategies. Ecology 101 (3), e02951. doi: 10.1002/ecy.2951
Lutz V. A., Sathyendranath S., Head E. J., Li W. K. (2003). Variability in pigment composition and opticalcharacteristics of phytoplankton in the LabradorSea and the central north Atlantic. Mar. Ecol. Prog. Ser. 260, 1–18. doi: 10.3354/meps260001
Mackey M. D., Mackey D. J., Higgins H. W., Wright S. W. (1996). CHEMTAX-a program for estimating class abundances from chemical markers: application to HPLC measurements of phytoplankton. Mar. Ecol. Prog. Ser. 144, 265–283. doi: 10.3354/meps144265
Mackey D. J., Parslow J., Higgins H. W., Griffithst F. B., O’sullivan J. E. (1995). Plankton productivity and biomass in the western equatorial pacific: Biological and physical controls. Deep Sea Res. Part II Top. Stud. Oceanogr. 42, 49–533. doi: 10.1016/0967-0645(95)00038-R
Messié M., Radenac M. H. (2006). Seasonal variability of the surface chlorophyll in the western tropical pacific from SeaWiFS data. Deep Res. Part I Oceanogr. Res. Pap. 53, 1581–1600. doi: 10.1016/j.dsr.2006.06.007
Miki M., Ramaiah N., Takeda S., Furuya K. (2008). Phytoplankton dynamics associated with the monsoonin the sulu Sea as revealed by pigment signature. J. Oceanogr. 64, 663–673. doi: 10.1007/s10872-008-0056-7
Min J. O., Ha S. Y., Hur J., Shin K. H. (2019). Primary productivity and photosynthetic pigment production rates of periphyton and phytoplankton in lake paldang using 13C tracer. Korean J. Ecol. Environ. 52, 202–209. doi: 10.11614/ksl.2019.52.3.202
Moore L. R., Goericke R., Chisholm S. W. (1995). Comparative physiology of synechococcus and prochlorococcus: influence of light and temperature on growth, pigments, fluorescence and absorptive properties. Mar. Ecol. Prog. Ser. 116, 259–275.
Morel A., Ahn Y. H., Partensky F., Vaulot D., Claustre H. (1993). Prochlorococcus and synechococcus: a comparative study of their optical properties in relation to their size and pigmentation. J. Mar. Res. 51, 617–649. doi: 10.1357/0022240933223963
Pabi S., van Dijken G. L., Arrigo K. R. (2008). Primary production in the arctic ocean 1998-2006. J. Geophys. Res. Ocean 113:C08005. doi: 10.1029/2007JC004578
Pai S. C., Chen H. Y. (1994). Vertical distribution of cadmium in marginal seas of the western pacific ocean. Mar. Chem. 47 (1), 81–91. doi: 10.1016/0304-4203(94)90015-9
Partensky F., Blanchot J., Lantoine F., Neveux J., Marie D. (1996). Vertical structure of picophytoplankton at different trophic sites of the tropical northeastern Atlantic ocean. Deep Sea Res. Part I Oceanogr. Res. Pap. 43, 1191–1213. doi: 10.1016/0967-0637(96)00056-8
Partensky F., Blanchot J., Vaulot D. (1999). Differential distribution and ecology of prochlorococcus and synechococcus in oceanic waters: a review. Bull. l’inst. Océanogr. Monaco 19, 457–475.
Prezelin B. B., Matlick H. A. (1980). Time course of photoadaptation in the photosynthesis irradiance relationship of a dinoflagellate exhibiting photosynthetic periodicity. Mar. Biol. 58, 85–96. doi: 10.1007/BF00396119
Ramus J. (1990). A form-function analysis of photon capture for seaweeds. Hydrobiologica 204 (205), 65–71. doi: 10.1007/BF00040216
Redalje D. G., Laws E. A. (1981). A new method for estimating phytoplankton growth rates and carbon biomass. Mar. Biol. 62 (1), 73–79. doi: 10.1007/BF00396953
Richardson K., Beardall J., Raven J. A. (1983). Adaptation of unicellular algae to irradiance: an analysis of strategies. New Phycol. 93, 157–191. doi: 10.1111/j.1469-8137.1983.tb03422.x
Roy S., Llewellyn C. A., Egeland E. S., Johnsen G. (2011). Phytoplankton pigments: characterization, chemotaxonomy and applications in oceanography (Cambridge University Press).
Ruan Z., Prášil O., Giordano M. (2018). The phycobilisomes of synechococcus sp. are constructed to minimize nitrogen use in nitrogen-limited cells and to maximize energy capture in energy-limited cells. Environ. Exp. Bot. 150, 152–160. doi: 10.1016/j.envexpbot.2018.01.015
Sathyendranath S., Platt T. (2007). Spectral effects in bio-optical control on the ocean system bio-optical properties of phytoplankton remote sensing of ocean colour species succession primary production phytoplankton functional types mixed-layer physics. Oceanologia 49, 5–39.
Shibata T., Tripathy S. C., Ishizaka J. (2010). Phytoplankton pigment change as a photoadaptive response to light variation caused by tidal cycle in ariake bay, Japan. J. Oceanogr. 66 (6), 831–843. doi: 10.1007/s10872-010-0067-z
Siefermann-Harms D. (1985). Carotenoids in photosynthesis, I: location in photosynthetic membranes and light-harvesting function. Biochim. Biophys. Acta 811, 325–355. doi: 10.1016/0304-4173(85)90006-0
Ting C. S., Rocap G., King J., Chisholm S. W. (2002). Cyanobacterial photosynthesis in the oceans: the origins and significance of divergent light-harvesting strategies. Trends Microbiol. 10 (3), 134–142. doi: 10.1016/S0966-842X(02)02319-3
Tremblay J. É., Anderson L. G., Matrai P., Coupel P., Bélanger S., Michel C., et al. (2015). Global and regional drivers of nutrient supply, primary production and CO2 drawdown in the changing Arctic ocean. Prog. Oceanogr. 139, 171–196. doi: 10.1016/j.pocean.2015.08.009
Veldhuis M. J. W., Kraay G. (1990). Vertical distribution and pigment composition of a picoplanktonic prochlorophyte in the subtropical north atlantic:a combined study of HPLC-analysis of pigments and flow cytometry. Mar. Ecol. Prog. Ser. 68, 121–127. doi: 10.3354/meps068121
Veldhuis M. J. W., Timmermans K. R., Croot P., van der Wagt B. (2005). Picophytoplankton; a comparative study of their biochemical composition and photosynthetic properties. J. Sea Res. 53, 7–24. doi: 10.1016/j.seares.2004.01.006
Wong G. T. F., Pai S. C., Chung S. W. (1995). Cobalt in the western Philippine Sea. Oceanol. Acta 18 (6), 631–638.
Yun M. S., Kim Y., Jeong Y., Joo H. T., Jo Y. H., Lee C. H., et al. (2020). Weak response of biological productivity and community structure of phytoplankton to mesoscale eddies in the oligotrophic Philippine Sea. J. Geophys. Res.-Oceans 125 (12), e2020JC016436. doi: 10.1029/2020JC016436
Zapata M., Rodríguez F., Garrido J. L. (2000). Separation of chlorophylls and carotenoids from marine phytoplankton: A new HPLC method using a reversed phase C8 column and pyridine-containing mobile phases. Mar. Ecol. Prog. Ser. 195, 29–45. doi: 10.3354/meps195029
Zhao S., Wei J., Yue H., Xiao T. (2010). Picophytoplankton abundance and community structure in the Philippine Sea, western pacific. Chin. J. Oceanol. Limnol. 28, 88–95. doi: 10.1007/s00343-010-9274-0
Keywords: photosynthetic pigment, physiological state, primary production, pigment production rate, light harvesting, PSII replacement, Prochlorococcus
Citation: Lee CH, Kang JJ, Min J-O, Bae H, Kim Y, Park S, Kim J, Kim D and Lee SH (2022) Physiological characteristics of phytoplankton in response to different light environments in the Philippine Sea, Northwestern Pacific Ocean. Front. Mar. Sci. 9:930690. doi: 10.3389/fmars.2022.930690
Received: 28 April 2022; Accepted: 25 July 2022;
Published: 19 August 2022.
Edited by:
Patrick J. Neale, Smithsonian Environmental Research Center (SI), United StatesReviewed by:
Sarat Chandra Tripathy, National Centre for Polar and Ocean Research (NCPOR), IndiaWillem Hendrik Van De Poll, University of Groningen, Netherlands
Copyright © 2022 Lee, Kang, Min, Bae, Kim, Park, Kim, Kim and Lee. This is an open-access article distributed under the terms of the Creative Commons Attribution License (CC BY). The use, distribution or reproduction in other forums is permitted, provided the original author(s) and the copyright owner(s) are credited and that the original publication in this journal is cited, in accordance with accepted academic practice. No use, distribution or reproduction is permitted which does not comply with these terms.
*Correspondence: Jae Joong Kang, amFlanVuZ0BwdXNhbi5hYy5rcg==; Sang Heon Lee, c2FuZ2xlZUBwdXNhbi5hYy5rcg==