- 1State Key Laboratory of Tropical Oceanography, South China Sea Institute of Oceanology, Chinese Academy of Sciences, Guangzhou, China
- 2Daya Bay Marine Biology Research Station, Chinese Academy of Sciences, Shenzhen, China
- 3Innovation Academy of South China Sea Ecology and Environmental Engineering, Chinese Academy of Sciences, Guangzhou, China
- 4University of Chinese Academy of Sciences, Beijing, China
Distributed in different elevations of the intertidal zone, mangrove plants suffer different periods of flooding, and with varied adaptability to waterlogging from the physiological structure to the metabolic mechanism. Associated with species distribution, transcriptome sequencing was performed to explore the interspecific differences of molecular response mechanisms among Avicennia marina, Aegiceras corniculatum, and Bruguiera gymnorrhiza under waterlogging stress. Results showed that the counts of differentially expressed genes (DEGs) in A. Marina were the highest after stress, followed by Ae. corniculatum and B. gymnorrhiza. It was found that the functions of genes with high differential expression folds (more than eight folds) in the three plants could be classified into four categories: structural regulation, transport, biosynthesis, and protection. It was also found that A. Marina has strong regulation ability in the metabolic process, which can guarantee energy supply and maintain active biosynthesis under waterlogging conditions. In addition, A. Marina was activated in the ethylene synthesis pathway to promote aerenchyma formation and to avoid root tissue hypoxia. Being different from A. Marina and B. gymnorrhiza, Ae. corniculatum down-regulated the XET, SAMS, and ACCO genes, which were involved in the cell wall regulation or ethylene formation that might indicate a different adaptive mechanism. Alcohol dehydrogenase (ADH) and lactate dehydrogenase (LDH) were cloned from A. Marina, Ae. corniculatum, and B. gymnorrhiza. The cloned genes were named as AmADH, AmLDH, AcADH, AcLDH, BgADH, and BgLDH, respectively. qRT-PCR detection verified that LDHs and ADHs were involved in the response of mangrove plants to waterlogging stress, and interspecific difference was observed. The expressions of AcADH and AcLDH were the most prominent. Combined with transcriptome, it was considered that Ae. corniculatum was more dependent on the expression of AcADH and AcLDH that might compensate the weakness of cell wall regulation, whereas A. Marina was more dependent on the regulation of cell structure reversely.
1. Introduction
Mangrove forests appear in tropical and subtropical interfaces between land and ocean and offer multiple ecosystem services (Wang, 2019; Wang and Gu, 2021). The remarkable adaption ability of mangrove plants to tolerate inundation enables them to inhabit intertidal zones where they tend to be periodically submerged by tide. Species distribution research of mangrove ecosystems demonstrated that zonal and mosaic-pattern distributions existed in mangrove ecosystems from different regions (Wen et al., 2002; Liu et al., 2012). Bruguiera gymnorrhiza commonly locates in the upper tidal flat, whereas the Aegiceras corniculatum is in the middle or low zone. Avicennia marina, as the pioneer, can well adapt to a wider range of tidal zones and always distributes in the area closest to the sea (Figure 1) (Zhang et al., 2001; Almahasheer et al., 2016; Al-Khayat and Alatalo, 2021). Different distributions of mangrove plants mirror their varied adaption abilities to intertidal environmental factors. Water conditions, such as elevation and duration of submergence, can be the critical factors impacting their location. Because of global climate issues, changes in precipitation patterns and sea level rise might break the balance of the mangrove ecosystem and result in unexpected disturbance of water conditions and waterlogging (Allen et al., 2010). Therefore, the interaction between mangrove plants and waterlogging need to be focused.
As gas diffusion rate in water is low, hypoxia or anaerobic metabolism is commonly seen as the main adverse effect resulting from waterlogging (Vartapetian, 2005; Vartapetian, 2006). Common response mechanisms of plants to low-oxygen stress have been plotted by previous research. Avoidance of and survival from anoxia are the two main strategies of plants to cope with waterlogging (Geigenberger, 2003). The structural changes are important to alleviate hypoxia. Aerenchyma is the tissue that provides gases the channel and facilitates gas diffusion between the aerial and submerged tissues (Maricle and Lee, 2002; Evans, 2004; Takahashi et al., 2014). The formation of aerenchyma and elongation contribute to the transportation of O2 from the aerobic tissue to the submerged tissue. As for metabolism, energy shortage is a vital challenge facing hypoxic plants (Blokhina et al., 2001; Dolferus et al., 2001). To decrease oxygen and energy consumption, plants tend to restrict a series of biosynthetic processes (Geigenberger, 2003). Genes involved in pathways of glycolysis and anaerobic fermentation would be induced and then function in metabolism maintenance under low-oxygen condition (Paul and Ferl, 1991; Dolferus et al., 2001). Alcohol dehydrogenase (ADH) and lactate dehydrogenase (LDH), involved in alcoholic and lactic acid fermentation, are proven to be significant and functional in different submerged plants by generating the necessary NAD+ and ATP (Good and Paetkau, 1992; Dolferus et al., 2001; Dolferus et al., 2008; Zhou et al., 2017; Shen et al., 2021).
Transcriptome sequencing research on waterlogging adaptability has been carried out gradually in recent years. Experiment on maize, Sesbania cannabina and Populus × canescens uncovered a series of gene regulations in plant response to waterlogging (Kreuzwieser et al., 2009; Juntawong et al., 2014; Arora et al., 2017). Previous studies explored the waterlogging effects of mangroves by researching their growth, physiological responses, survival rates, and biochemical indexes (Ye et al., 2003; Srikanth et al., 2016; Cheng et al., 2020). Using transcriptome technology to study the adaptability of plants to waterlogging has become a research trend. However, there are few transcriptome studies on the waterlogging response mechanism of mangrove plants. As a special plant subject to periodic tidal waterlogging stress, studies at the transcriptome level can help us further understand its response mechanism. Further, a systematic interpretation of the waterlogging tolerance mechanism in mangrove plants is worth exploring at the molecular level. Free transcriptomes can provide a view of species-specific metabolic activity patterns under certain exposure (Lipaeva et al., 2021).
In this paper, transcriptome sequencing was performed to elucidate the response mechanism of the three mangroves exposed to waterlogging. The diversity of their distribution at the molecular level and the association between their regulation at the molecular level and their natural distribution were also discussed.
2. Materials and methods
2.1. Plant materials and treatment
One-year mangrove plant seedlings were planted in clean sand (watered with 1/2 Hoagland solution every 3 days) and placed in a growth chamber for 1-week domestication (25°C, 75% humidity, 14h light/10h dark cycle). Seedlings in good and similar condition with the other species were selected for waterlogging treatment. To focus on waterlogging as a single impact factor, the chosen mangrove seedlings were transferred in planting pots filled with fresh water and 1/2 Hoagland solution. To better model long-term inundation due to climate change, the treatment was set as continuous waterlogging. Water reached the bottom leaves of the seedlings. After treatment with the corresponding time for 0, 1, 3, 5, 7, and 14 days, the root tips of each seeding were collected and frozen in liquid nitrogen immediately. There were three replicates with three plants in each treatment. All the tissues were stored at −80°C temporarily before use.
2.2. RNA isolation, preparation, and sequencing
Tissues of the three species treated for 0, 3, and 14 days were chosen for transcriptome sequencing. TRIZOL RNA extraction method was used to extract total RNA. The concentration and integrity of RNA were detected by Bioanalyzer 2100 system (Agilent Technologies, CA, USA) and gel electrophoresis. Purity (OD260/280) was tested by Nanodrop (IMPLEN, USA). The quality of each RNA sample was well controlled and guaranteed.
Transcriptome sequencing and database construction were completed by NovoGene (Beijing, China). Sequencing libraries were generated using NEBNext® UltraTM RNA Library Prep Kit for Illumina® (NEB, Massachusetts USA) and index codes were added to attribute sequences to each sample (Parkhomchuk et al., 2009). mRNA was purified from total RNA by poly-T oligo-attached magnetic beads. Fragmentation was carried out in NEBNext First Strand Synthesis Reaction Buffer (5X). First strand cDNA was synthesized by random hexamer primer and M-MuLV Reverse Transcriptase (RNase H -). Second strand cDNA synthesis was subsequently performed using DNA polymerase I and RNase H. Remaining overhangs were converted into blunt ends via exonuclease/polymerase activities. After adenylation of 3′ ends of DNA fragments, NEBNext adaptor with a hairpin loop structure was ligated to prepare for hybridization. To select cDNA fragments of preferentially 250–300 bp in length, the library fragments were purified with AMPure XP system (Beckman Coulter, Beverly, Indianapolis USA). Then, USER Enzyme (NEB) was used with size-selected, adaptor-ligated cDNA before PCR. PCR was performed with Phusion High-Fidelity DNA polymerase, Universal PCR primers, and Index (X) Primer. PCR products were purified (AMPure XP system) and library quality was assessed on the Agilent Bioanalyzer 2100 system.
The Illumina NovaSeq 6000 system was used to sequence and generate the end read. The sequence data were obtained by CASAVA-based recognition. The raw data were cleaned by removing reads containing adapter, reads containing N base, and low-quality reads. The Q20, Q30, and GC contents of the clean data were calculated. The data analysis that followed was based on the clean data.
2.3. Data analysis
The DESeq2 R package (1.20.0) was used to analyze the differential expression of the two groups. The false discovery rate of p-values was controlled by Benjamini and Hochberg’s approach (Benjamini and Hochberg, 1995). Significant differential expression satisfied the conditions of padj < 0.05 and |log2(fold change)| > 1.
Both the Gene Ontology (GO) function and the Kyoto Encyclopedia of Genes and Genomes (KEGG) pathway enrichment analyses were computed by GOseq (1.10.0) and KOBAS (v2.0.12). The analyzed gene sets were those genes with significant differences and annotations to the GO or KEGG database. Heatmaps were piloted by the pheatmap R package (1.0.12).
2.4. Cloning and quantitative expression of ADH and LDH
The degenerate pair of primers dF and dR (Table 1) used for the ADH and LDH gene fragment cloning from three species were designed by the j-CODEHOP tool of Base-By-Base (https://4virology.net/). The PrimeScript™ RT reagent Kit with gDNA Eraser (Takara, Beijing China) was used for the fragmentary cDNA synthesis. After initial denaturation for 5 min at 94°C, then 35 cycles of 94°C denaturation for 30 s, 50°C annealing for 30 s, 72°C extension for 1 min, and a final extension step of 72°C for 10 min, the ADH fragments of approximately 588 bp in length and the LDH fragments of approximately 333 bp were amplified. PCR products were purified from 1% agarose gel electrophoresis. The samples with more than 20 μg/mol were sent for sequencing by the Beijing Genomics Institute (BGI, China).
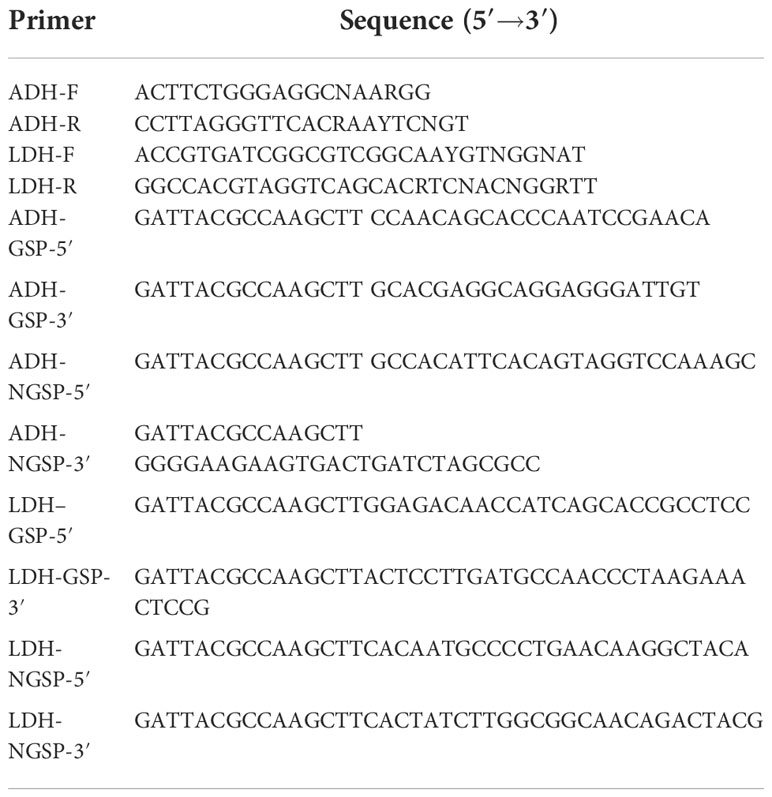
Table 1 Primers for alcohol dehydrogenase (ADH) and lactate dehydrogenase (LDH) fragments and full-length cDNA clone.
The full-length cDNA sequence was obtained by using the SMARTer® RACE 5′/3′ Kit (Clontech, USA) according to the manufacturer’s instructions. The primary PCR reaction was performed using gene-specific primers (GSPs) (Table 1) and a universal primer mix (UPM). The nested PCR reaction was performed using nested gene-specific primers (NGSPs) and a UPM short. The primers mentioned above were designed based on the obtained fragments by Primer (Version 5.0). PCR and nested PCR were performed in Mastercycler® nexus (Eppendorf, Germany) with the following three-step program: 25 cycles of 94°C for 30 s, 69°C for 30 s, and 72°C for 3 min. The PCR products were gel purified by NucleoSpin Gel and PCR Clean-Up Kit (Clontech, USA). Then, the instruction was followed to obtain the full-length cDNA.
The phylogenetic tree was computed by the neighbor-joining algorithm and drawn by MEGA -X (https://www.megasoftware.net/). The SWISS-MODEL (http://swissmodel.expasy.org/) generated the homology modeling structure of the AcADH and AcLDHs.
qPCR was performed in Applied Biosystems StepOnePlusTM Real-Time System with the kit of SYBR Premix Ex TaqTM II reagents (Takara, Japan). The primers of ADHs and LDHs listed in Table 2 were designed based on the results of the full-length cDNA and transcriptome. The 18S rRNA was used as the internal reference gene of Ae. corniculatum and B. gymnorrhiza, and the Actin gene was the internal reference gene of A. Marina. The PCR procedure was as follows: 95°C for 10 min, 40 cycles at 95°C for 10 s, 60°C for 60 s, and 72°C for 30 s. Each qRT-PCR reaction was performed with three replicates. The expressions of ADHs and LDHs were quantified by the 2-△△CT method.
3. Results
3.1. Quality, annotation, enrichment, and differentially expressed genes (DEGs)
Transcriptome sequencing of all the samples yielded 187.58 G of raw reads and 184.51 G of clean reads (removed the adapter, unrecognized bases, and low-quality reads). Q30 of all samples reached more than 93; the average value was 93.86. The average GC content value was about 45%, and the transcriptome sequencing data results were accurate and the data were credible, which met the requirements for subsequent analysis.
Comprehensive gene function information of each unigene were annotated by comparing with seven databases, including NCBI’s non-redundant protein sequences (Nr), NCBI’s nucleotide sequences (Nt), Protein family (Pfam), Clusters of Orthologous Groups of proteins/euKaryotic Ortholog Groups (KOG/COG), Swiss-Prot, Kyoto Encyclopedia of Genes and Genomes (KEGG), and Gene Ontology (GO). There were 112,614 unigenes (68.74%) successfully annotated in at least one database but only 16,768 unigenes (10.23%) annotated in all seven databases (Table 3). Successful annotation rate in the NR database was the highest (59.79%), the annotation rates of GO (Gene Ontology) and KO (KEGG Ontology) databases, which are commonly used for metabolic pathway analysis were 48.34% and 27.17%, respectively.
Genes annotated by GO can be classified into cellular component, molecular function, and biological process (Figure 2). It was observed that the cellular process, metabolic process, binding, catalytic activity, cellular anatomical entity, and intracellular were highly enriched in the three mangrove plants. The KEGG enrichment shows that “Translation” and “Folding, sorting and degradation” pathways enriched 7,408 and 3,560 genes, respectively. It meant an intensive regulation on the protein synthesis and processing in mangrove root tissues under waterlogging. In addition, “Signal transduction (551 genes)”, “Transport and catabolism (3550 genes)”, and “Cell growth and death (1848 genes)” enriched significantly, reflecting the regulation of the root tissues under stress to a certain extent.
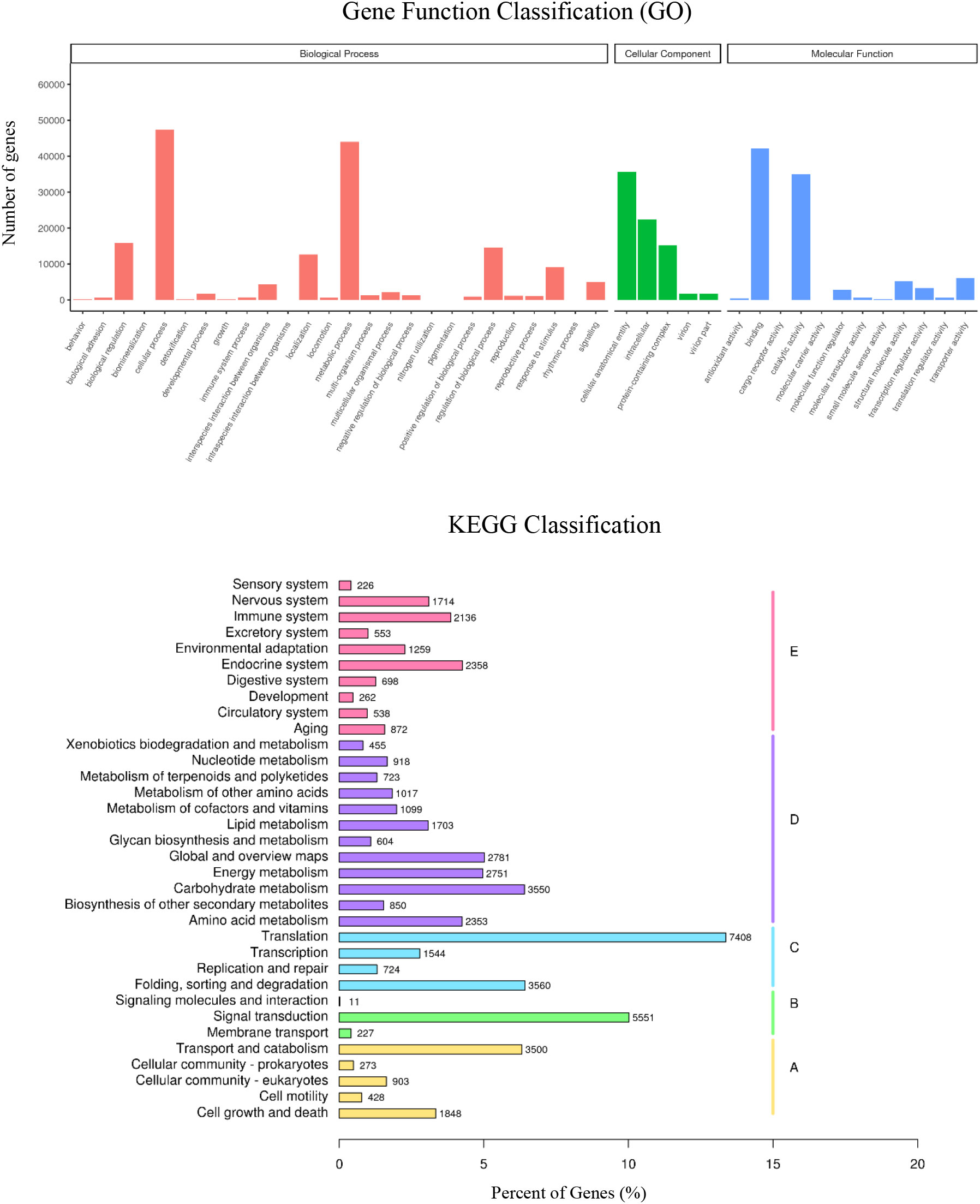
Figure 2 Gene Ontology (GO) function and Kyoto Encyclopedia of Genes and Genomes (KEGG) metabolic pathway classification. A: Cellular Processes, B: Environmental Information Processing, C: GeneticInformation Processing,D: Metabolism, E: Organismal Systems.
The total amount of DEGs in each species under 3- and 14-day waterlogging treatments compared to 0 day was counted, and the results are shown in Figure 3. The number of DEGs in A. Marina was the highest: 3,143 and 6,450 after 3- and 14-day stress exposure, respectively. Among them, 2,611 genes were upregulated and 532 genes were downregulated after 3 days, and 5,102 genes were upregulated and 1,348 genes were downregulated after 14 days. The counts of DEGs in Ae. corniculatum root tissues treated for 3 and 14 days were 3,255 and 1,283, respectively. A total of 1,456 genes were upregulated and 1,799 genes were downregulated after 3 days; 807 genes were upregulated and 476 genes were downregulated after 14 days. There were 1,460 and 1,220 genes differentially expressed in B. gymnorrhiza root tissues treated for 3 and 14 days. There were 592 genes upregulated and 868 genes downregulated in 3-day-treated root tissues. There were 678 genes upregulated and 542 genes downregulated in 14-day-treated tissues.
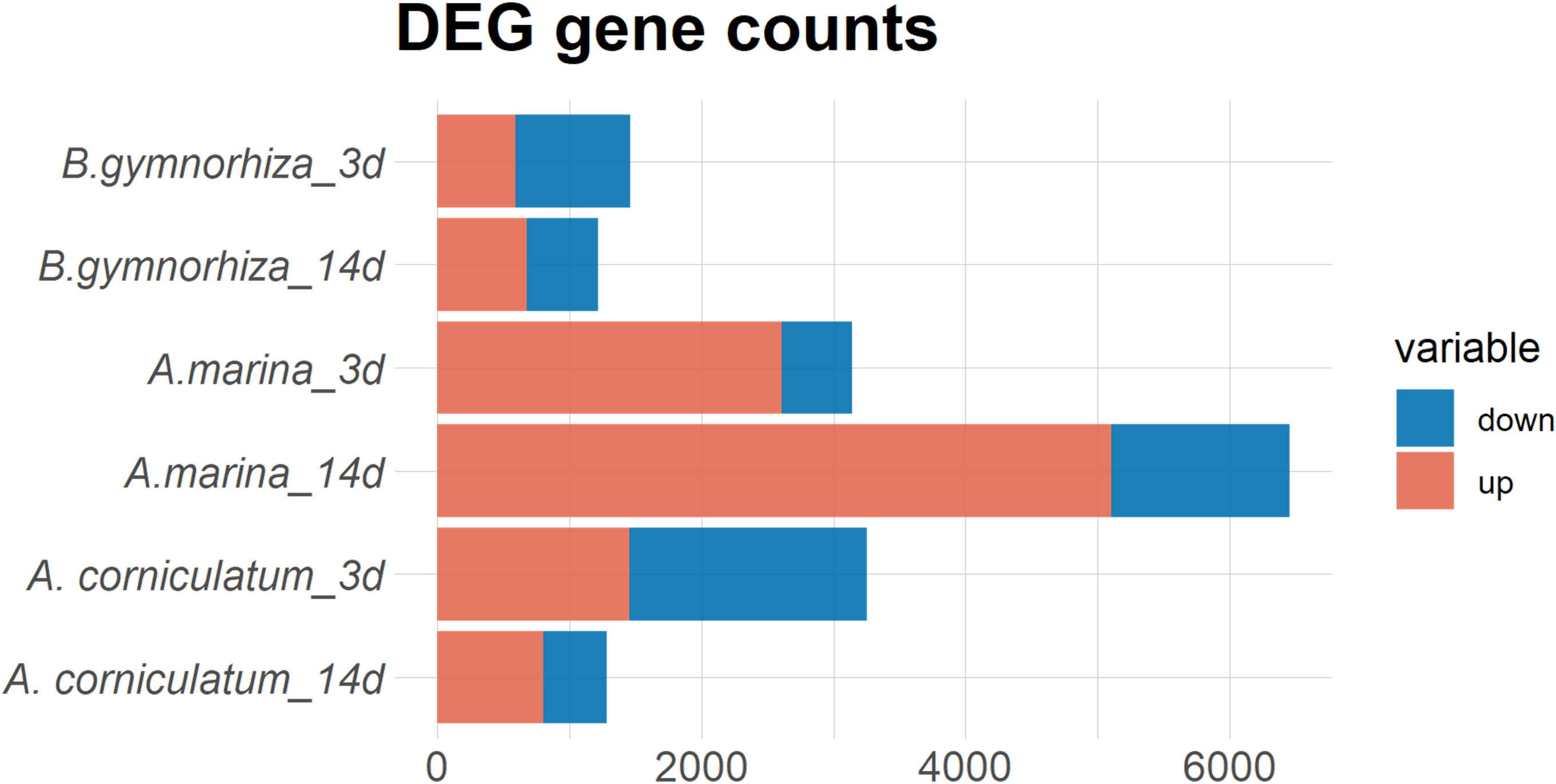
Figure 3 Differentially expressed genes (DEGs) statistics of the treated groups (3 and 14 days) compared with the 0-day group.
3.2. DEGs with high change folds
To better explore the regulatory mechanisms in three mangroves, DEGs with obvious changes (more than eightfold) were filtered (Table 4). The results (log2 [FPKFM]) are illustrated by the heat map in Figure 4.
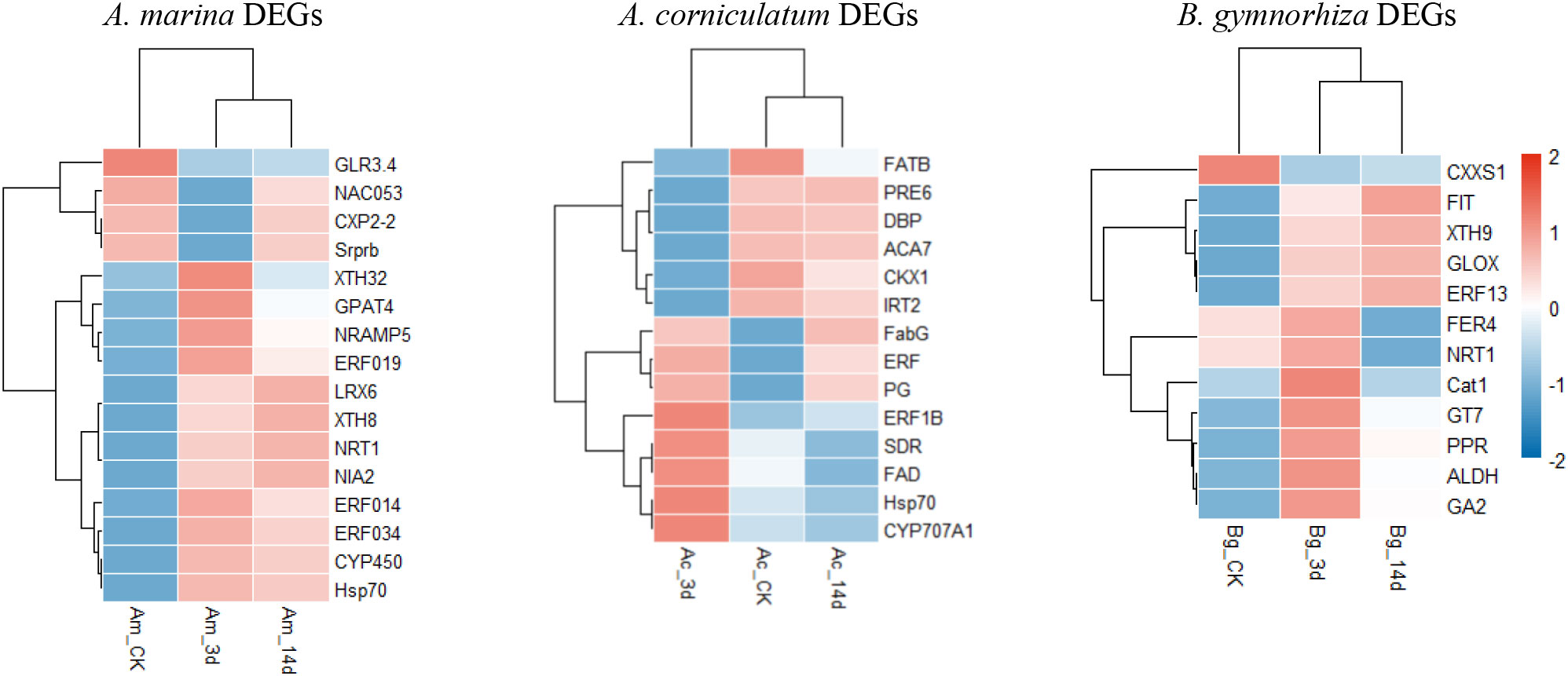
Figure 4 Differentially expressed genes (DEGs) information and Gene Ontology (GO) enrichment of Avicennia marina, Aegiceras corniculatum, and Bruguiera gymnorrhiza.
The selected DEGs of A. Marina exhibited mainly two patterns of regulation, namely, up and down and their functions could be summarized as biosynthesis, cell wall structure regulation, transportation, and stress protection. To be specific, the upregulated genes and their functions were as follows: xyloglucan endotransglucosylase/hydrolase32, participates in cell wall construction of growing tissues; glycerol-3-phosphate 2-O-acyltransferase 4, involved in lipid poly-esters biosynthesis (Yang et al., 2010); metal transporter Nramp5, involved in iron uptake; ethylene-responsive transcription factor (ERF), involved in the regulation of metabolism and response to stimuli; leucine-rich repeat extensin-like protein 6, modulates cell morphogenesis by regulating cell wall formation and assembly (Herger et al., 2019); NRT1, nitrate and auxin transporter; Hsp70, protects plant cells from increased stress conditions; and CYP450, important in development and defense. The downregulated genes are glutamate receptor 3.4 that acts as a negative regulator of lateral root initiation and development (Vincill et al., 2013) and NAC domain-containing protein 53 that is involved in in reactive oxygen species accumulation.
In Ae. corniculatum, there were two major regulation modes: upregulation first and then recovery and downregulation first and then recovery. The upregulated genes with more than eightfold changes were as follows: FabG, catalyzes the first reductive step in the elongation cycle of fatty acid biosynthesis; polygalacturonase (PG), depolymerizes glycosidic bonds of pectic acid (Yahia and Carrillo-Lopez, 2018); fatty acid desaturase (FAD), maintains the proper structure and functioning of biological membranes; CYP707A1, involved in the oxidative degradation of abscisic acid; and ERF1B and Hsp70. Other genes were downregulated: palmitoyl-acyl carrier protein thioesterase (FATB) that plays an important role in the synthesis of saturated fatty acids and also regulates cell growth (Bonaventure et al., 2003); transcription factor PRE6; dehydration responsive element binding proteins that strengthen tolerance to environmental stresses (Huang and Liu, 2006); alpha carbonic anhydrase 7 that reverses the hydration of carbon dioxide; cytokinin dehydrogenase 1 that catalyzes the oxidation of cytokinin; and iron-regulated transporter 2. Structure regulation, stress adaptability, and biosynthesis were also regulated by Ae. corniculatum. However, compared with A. Marina, there were fewer upregulated genes that can contribute to plant adaptability.
As for B. gymnorrhiza, there are only thioredoxin-like protein CXXS1, which was downregulated (Serrato et al., 2008). Among the upregulated genes, aldehyde dehydrogenase is a kind of NAD(P)+-dependent enzyme; catalase is an antioxidant enzyme; ferritin-4, chloroplastic, is important for iron homeostasis; iron deficiency-induced transcription factor is essential for the regulation of iron uptake in roots; GT7 is a broad spectrum multifunctional glucosyltransferase; GA2 is involved in the biosynthesis of gibberellin phytohormones; and glyoxal oxidase can catalyze the oxidation of aldehydes to the corresponding carboxylate (Phan et al., 2011). In addition, NRT, ERF, and XTH also appeared in the heatmap of B. gymnorrhiza. The functions of upregulated genes in B. gymnorrhiza were similar to those in A. Marina and Ae. corniculatum, that could be summarized as transport, structural regulation, and homeostasis under stress.
3.3. Glucose supply and glycolysis
Metabolic regulation plays an important role in the survival of waterlogging plants. Hence, KEGG pathways of the starch and sucrose metabolism (KO00500)” and glycolysis/gluconeogenesis (KO00010) were chosen for further discussion.
In the starch and sucrose metabolism pathway (Figure 5), the activity of gene regulation was related to natural distribution, which meant the closer to the sea, the more active gene regulation appeared. It was observed that beta-glucosidase (blgX), beta-fructofuranosidase (sacA), glycogen phosphorylase (GP), hexokinase (HK), pectinesterase (PE), and UDPglucose 6-dehydrogenase (UDGH) were upregulated in A. Marina. Ae. corniculatum downregulated UDGH, PE, and bglB but increased malQ. B. gymnorrhiza increased PE, blgX, and blgB, among others.
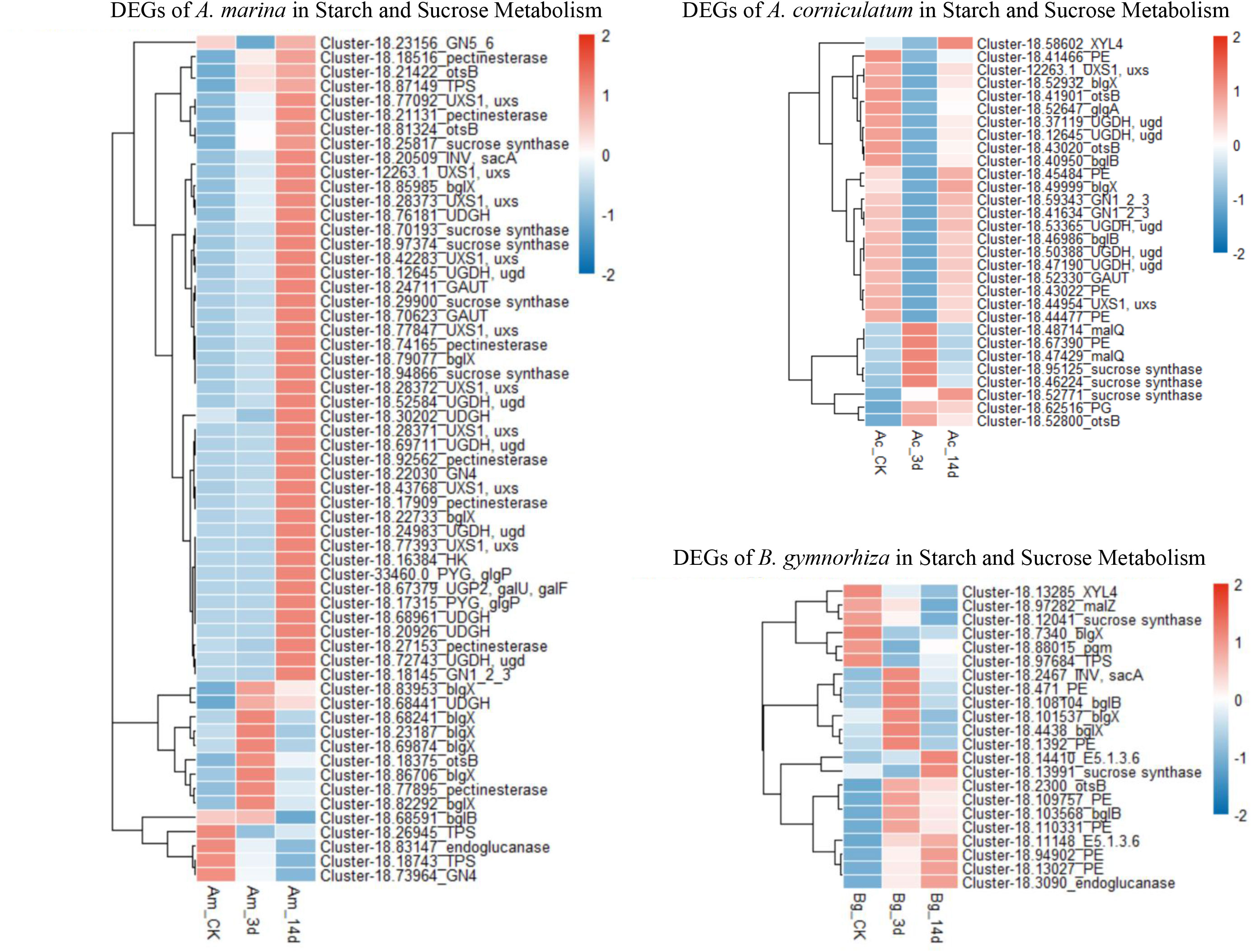
Figure 5 Regulation of starch and sucrose metabolism (KO00500) in Avicennia marina, Aegiceras corniculatum, and Bruguiera gymnorrhiza.
The glycolysis/gluconeogenesis (KO00010) pathway was downstream of the starch and sucrose metabolism (KO00500) pathway and the correlation between distribution and gene regulation also appeared. It could be observed that there were many genes with increased expression in A. Marina, for example, fructose-1, 6-bisphosphatase I (FBPI), glyceraldehyde 3-phosphate dehydrogenase (GAPDH), fructose-bisphosphate aldolase (ALDO), 2,3-bisphosphoglycerate-dependent phosphoglycerate mutase, and PDC (Figure 6). The results showed that A. Marina could maintain the activity of starch and sucrose metabolism pathway and glycolysis pathway after waterlogging stress, to better cope with hypoxia and energy deficiency. As for Ae. corniculatum, ALDO, PDC, and ADH were upregulated at 3 days, and GAPDH and phosphoglycerate kinase (PGK) were upregulated at both 3 and 14 days. In B. gymnorrhiza, HK, aldose 1-epimeras, and FBPI were downregulated on both 3 and 14 days. In addition, GAPDH, PGK, and PGM were downregulated after 3-day stress exposure.
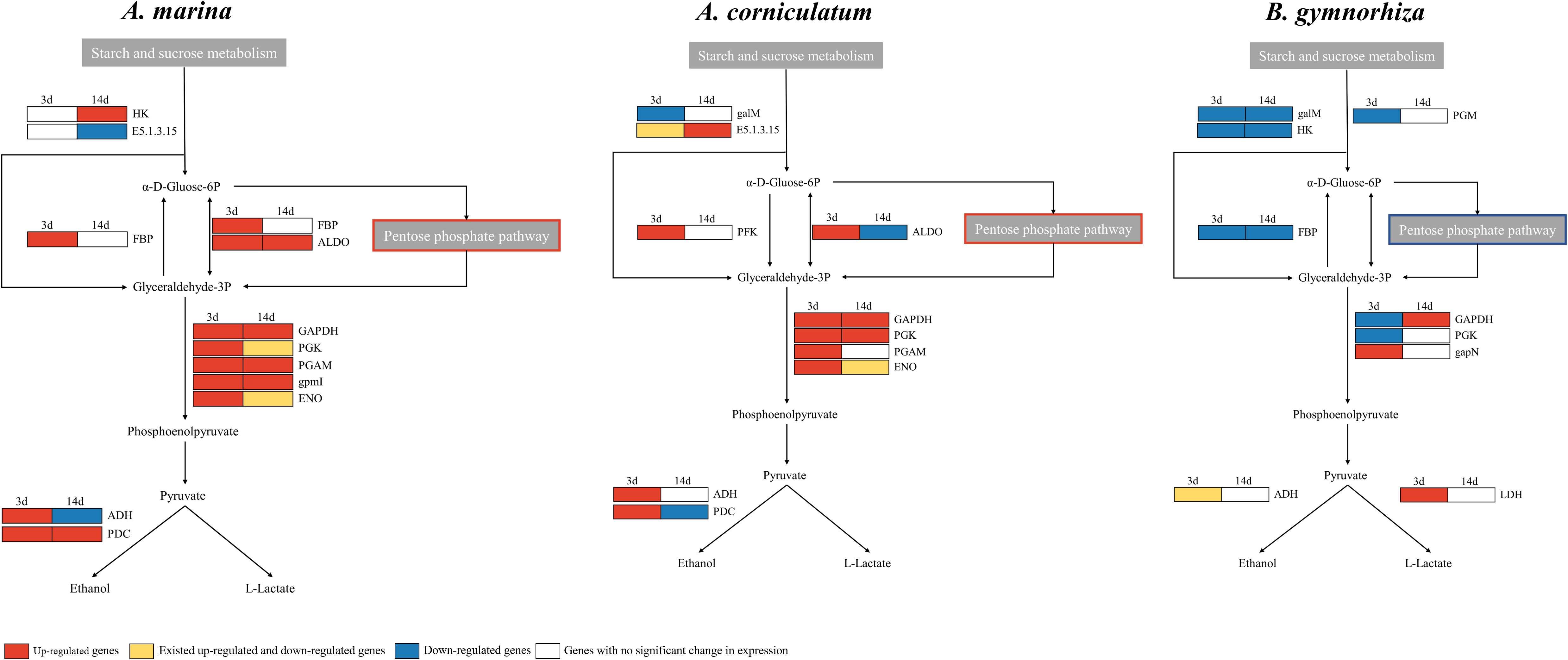
Figure 6 Regulation of glycolysis/gluconeogenesis (KO00010) in Avicennia marina, Aegiceras corniculatum, and Bruguiera gymnorrhiza.
3.4. Ethylene synthesis and cell wall regulation
Ethylene is a signal factor that can stimulate aerenchyma formation in plants. As shown in Figure 7, S-adenosylmethionine synthetase (SAMS), 1-aminocyclopropane-1-carboxylate synthase (ACS), and aminocyclopropanecarboxylate oxidase (ACCO) catalyzed the biosynthesis of ethylene. The regulation on SAMS, ACS, and ACCO in three mangroves were filtered (| log2fc | > 1). In A. marina, SAMS, ACSs, and ACCOs were upregulated, whereas more downregulation appeared in the other two species. On the 14th-day of stress exposure, there were 13 SAMS upregulated in A. marina, three SAMS in B. gymnorrhiza, but only one in Ae. corniculatum. The expression of ACCO increased in B. gymnorrhiza but still decreased in Ae. corniculatum after 14-day inundation. In Figure 7, almost all the genes enriched in the cell wall of Ae. corniculatum were downregulated after 3 days of waterlogging. The main downregulated genes in Ae. corniculatum were XETs (Xyloglucan endotransglycosylases, EC 2.4.1.207), which were the same with up-regulated genes in A. Marina.
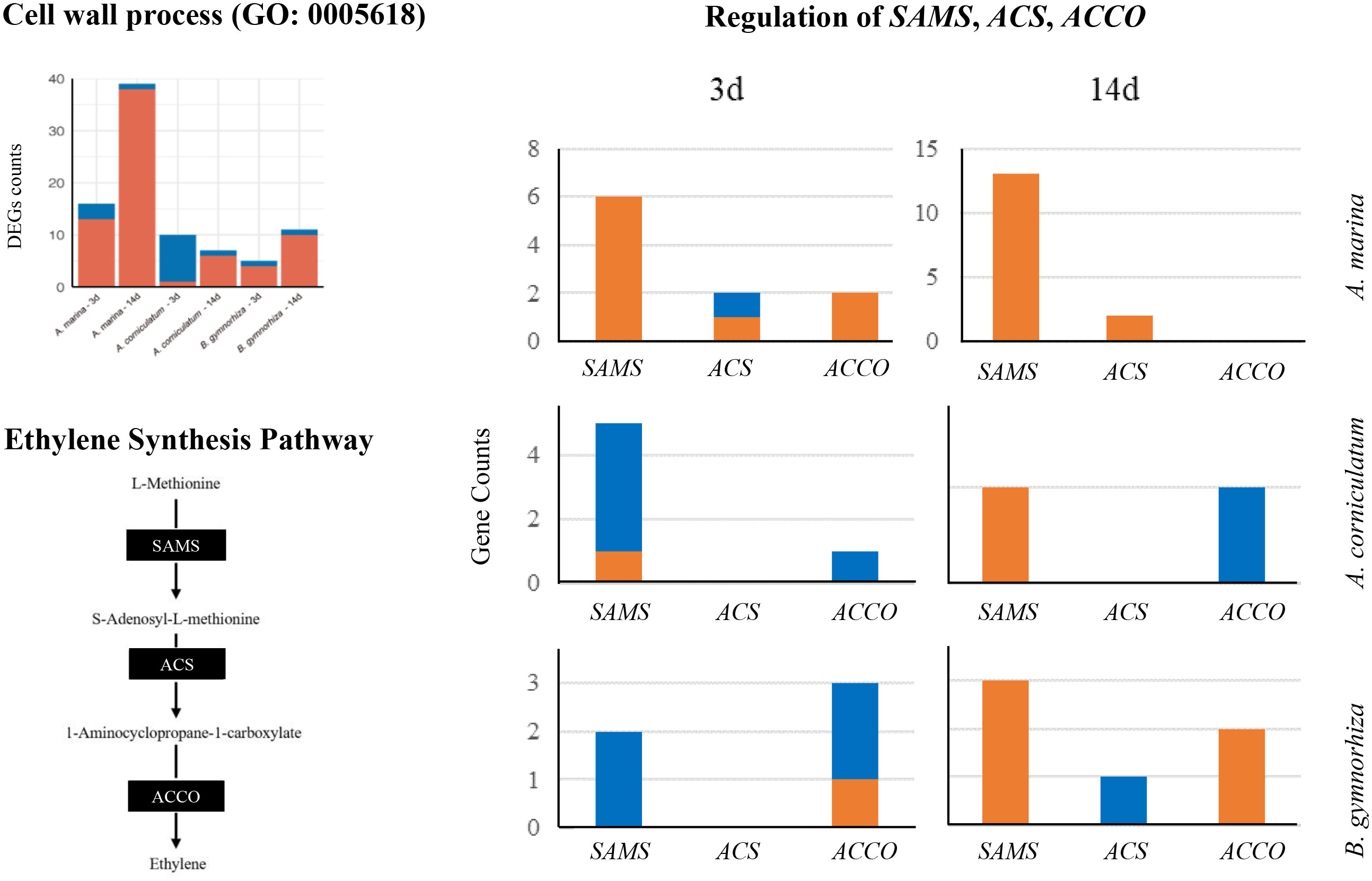
Figure 7 Differentially expressed genes (DEGs) counts of the cell wall process (GO: 0005618), ethylene synthesis pathway, and regulation of SAMS, ACS, and ACCO.
3.5. Clone and expression analysis of ADH and LDH
For further research, ADH and LDH, as key enzymes of anaerobic metabolism, were cloned and then qPCR was performed (Figures 8 and 9). ADHs and LDHs in the three mangrove plants are highly conserved. The amino acid sequences encoded by BgADH, AcADH, and AmADH are different only at positions 186 and 194. The amino acid sequences encoded by BgLDH, AcLDH, and AmLDH are different at positions 38 and 111. Due to the similarity, this paper constructed 3D structures of AcADH and AcLDH as representatives. The structures of AcADH and AcLDH are based on the ADH template of Arabidopsis thaliana (SMTL ID: 4rqu.1) and LDH template of rabbit muscle (SMTL ID: 5nqb.1), respectively. Phylogenetic dendrograms show that predicted ADHs and LDHs of the mangrove plants are in the same clade, indicating similarity.
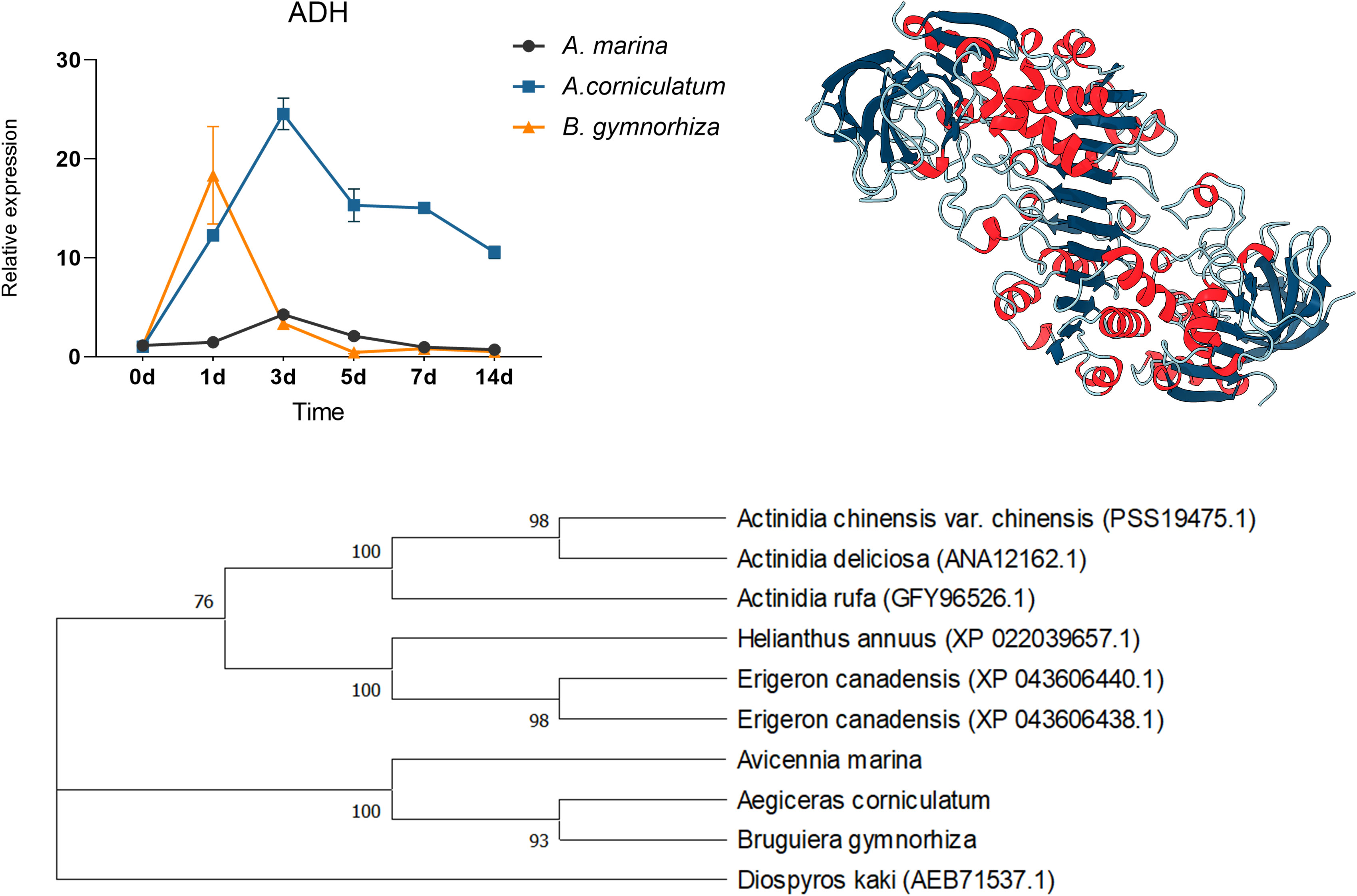
Figure 8 Quantitative expression of ADHs in three mangrove plants, 3D structure of AcADH, and phylogenetic tree of ADHs.
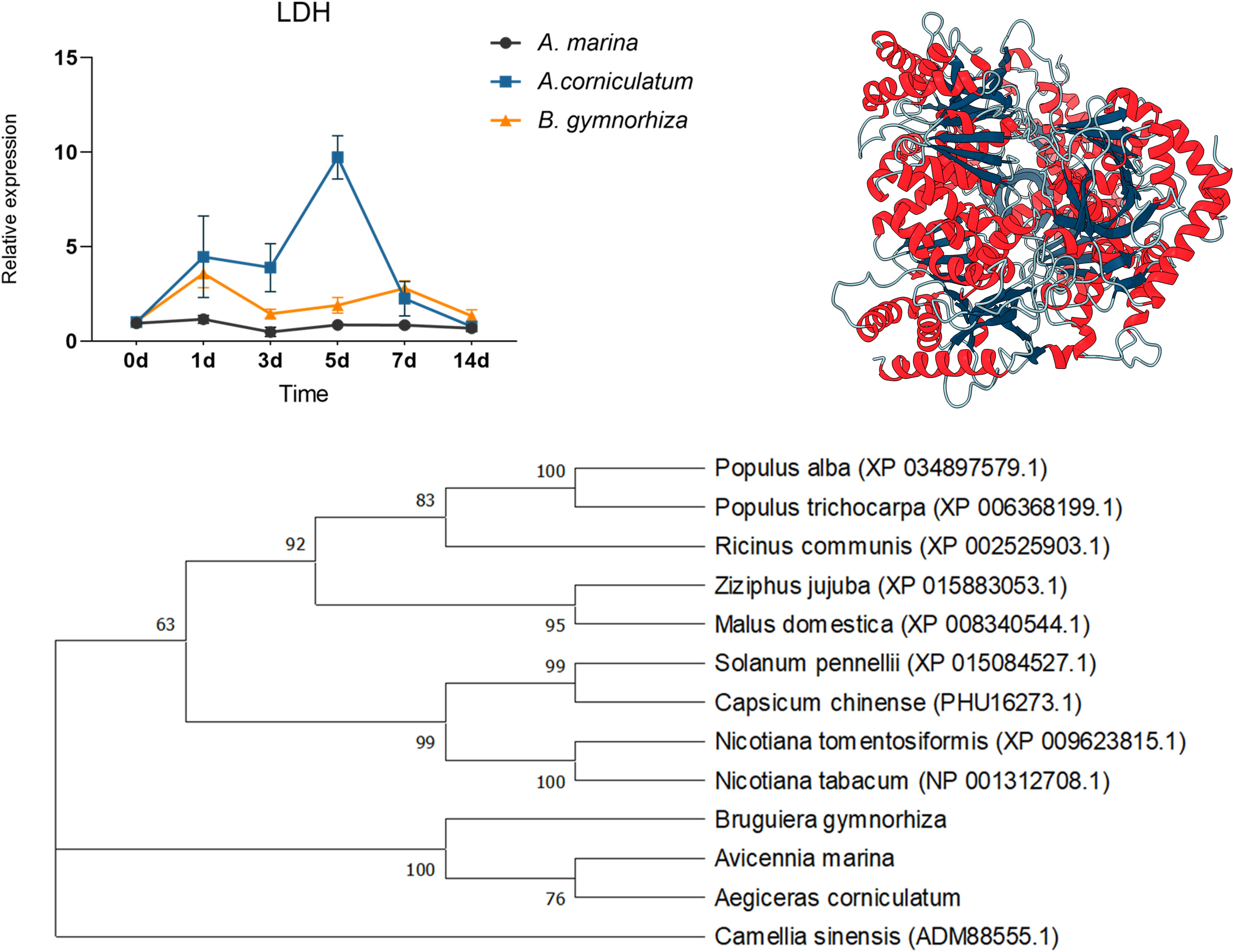
Figure 9 Quantitative expression of LDHs in three mangrove plants, 3D structure of AcLDH, and phylogenetic tree of LDHs.
As shown in Figure 8, ADHs upregulated in three mangrove plants; the relative expression of ADH in A. Marina and Ae. corniculatum peaked on the 3rd day, whereas the highest expression occurred on the 1st day in B. gymnorrhiza. Being different from the other two species, ADH of Ae. corniculatum was maintained at a high level after 5 days of exposure. The expression of LDH in A. Marina did not fluctuate due to waterlogging under all the sampling points of this study. The LDH expression of Ae. corniculatum and B. gymnorrhiza was upregulated within 1 to 7 days, and the fluctuation was more significant in Ae. corniculatum.
4. Discussion
This article mainly discussed the response mechanisms of different mangrove plants to waterlogging stress by focusing on DEGs and regulations of related pathways. The three mangrove species distributed naturally at different elevations in the intertidal zone and showed different tolerance levels to intertidal environmental factors including water condition. In this context, transcriptomes can reveal the specific response mechanism of each species under waterlogging and discover the differences of species at the molecular level.
4.1. Pronounced DEGs mirrored mechanism in each mangrove plant
The pioneer species A. Marina had the most numerous DEGs, followed by Ae. corniculatum second, and B. gymnorrhiza the least. It is worth noting that the number of DEGs was consistent with the natural distribution of the three mangrove species in the intertidal zone after waterlogging. DEGs in A. Marina continued increasing on the 14th day, and there were more upregulated genes than downregulated ones, while the number of DEGs of Ae. corniculatum and B. gymnorrhiza decreased on the 14th day. In addition, there were more downregulated DEGs in the other two species. The waterlogging experiment of Gray Poplar showed that the number of DEGs increased with duration of submergence (5, 24, and 168h), but more genes were down-regulated (Kreuzwieser et al., 2009). From this aspect, A. Marina showed the best adaptability to waterlogging stress by continuously upregulating abundant genes. It was considered that plants under waterlogging might need to balance their energy supply by limiting some biological activities (Geigenberger, 2003). This view might account for the downregulation on the 3rd day in Ae. corniculatum and B. gymnorrhiza.
Although the most pronounced DEGs are varied in the three mangrove plants, their functions or general response mechanisms to waterlogging stress can be summed up into four aspects, namely, structure modulation.transportation, biosynthesis, and stress protection.
XTH is key in cell wall extension, and LRXs can coordinate cell wall growth and formation (Herger et al., 2019). The two genes significantly expressed in A. Marina could change root morphogenesis by regulating the cell wall. Under waterlogging condition, the regulation of cell wall is deemed necessary in helping the plants escape from submergence by elongation. XTH also appeared in the list of most pronounced DEGs of A. Marina and B. gymnorrhiza. However, XTH was not observed in list of Ae. corniculatum unexpectedly, which might indicate a weakness. Lateral root growth was observed in Trifolium and was considered as a positive strategy under waterlogging condition (Gibberd et al., 2001). The expression of GLA 3.4 in A. Marina was limited, as a result, lateral root growth was stimulated. Ae. corniculatum also emphasized the growth of lateral roots by expressing PG. It was proved that PG particularly contributes to lateral root outgrowth (Peretto et al., 1992).
The metal and nutrient transporters were significantly regulated. A previous study proved that abiotic stresses would downregulate genes involved in nitrogen uptake (Goel and Singh, 2015). However, NRT, as a nitrogen transporter, increased in both A. Marina and B. gymnorrhiza. The opposite response meant a more assimilation of nitrogen in these two species. Some studies proved that ethylene production increased after high nitrate was exposed (Tian et al., 2009; Zheng et al., 2013). As ethylene is the key to aerenchyma formation, the expression of NRT was also supposed to be an indirect regulation on the structure of root tissue. Ae. corniculatum showed regulation in root morphology and structure by regulating PG; however, genes relevant to aerenchyma formation did not appear or may have been restricted, whereas those like NRT and XTH were increased in A. Marina and B. gymnorrhiza.
On the list of A. Marina, Hsp70, CYP450, NACO51, and ERF maintained the root-cell homeostasis. As for Ae. corniculatum, stability was balanced by FAD, HSP70, ERF, and CYP707A1. The predominant presence of these genes might indicate a timely and effective protection when waterlogging disturbs the root cells of mangrove plants.
4.2. Glucose supply and fermentation
Hypoxia induced by waterlogging restricts mitochondrial respiration. Plant cells shift the principal metabolic strategy from mitochondrial respiration to glycolysis to supply ATP. There are enough studies on hypoxia of plants metabolic to prove that the sugar supply is of great importance for plants to survive from hypoxia (Loreti et al., 2005; Cho et al., 2021). The accumulation of NADH and shortage of NAD+ are not conducive to glycolysis. Hence, fermentation is an important way to regenerate NAD+ from NADH under hypoxia and to generate energy (Cho et al., 2021; Kierans and Taylor, 2021).
In this study, genes working for glucose supply and glycolysis were sorted out for a better comparison among the three mangroves. sacA is a sucrose-hydrolyzing enzyme, and blgX is an enzyme that acts on cellobiose to further break down the structure into monomeric units of glucose (Bielecki and Somiari, 1998; Lewis and Davin, 1999). Both of them were upregulated in A. Marina. In addition, GP, which can limit the degradation of glycogen, was also upregulated in A. Marina. As for Ae. corniculatum, malQ was upregulated to better supply glucose. The regulations not only contributed to meeting the need for glucose but also providing substrate for energy generation. The active genes of metabolism ensured sugar availability in A. Marina and might account for their activity in biosynthetic pathways, as most of the plants need to restrict biosynthesis when waterlogging happens (Gibbs and Greenway, 2003).
Because of the pivotal function of ADH and LDH to promote fermentation, they were cloned from A. Marina, Ae. corniculatum, and B. gymnorrhiza. By constructing phylogenetic trees, we found that ADHs and LDHs from mangrove plants were similar and different from other terrestrial plants. Multiple sequence alignment further confirms conservation of ADHs and LDHs in the three mangroves. However, the results of quantitative expression are quite different in the three mangroves. In this study, the relative expression of ADH and LDH in A. Marina fluctuated slightly, or did not even change. The reason for the stable expression of ADH and LDH in A. Marina might be due to the rapid response in a few hours that the study cannot capture the change. While those in Ae. corniculatum are significant and durable. Combined with the discussion in the next section, the study considered that the high expression of ADH and LDH in Ae. corniculatum might compensate the regulation deficiency of structures.
4.3. Ethylene biosynthesis and cell wall construction
Aerenchyma is a channel for plants to transport oxygen from airy tissues to waterlogged tissues, which forms after submergence and could alleviate hypoxia. The formation of aerenchyma is vital for those wetland plants to adopt to anaerobic conditions (Purnobasuki and Suzuki, 2004). The importance of aerenchyma to mangroves had also been comprehensively reported (Hovenden and Allaway, 1994; Purnobasuki and Suzuki, 2004). Ethylene is the key factor in inducing aerenchyma formation in plant tissues. The water flooding experiment of Jatropha root tissue showed that genes related to ethylene synthesis, such as ACS and ACO, would upregulate to promote ethylene synthesis (Juntawong et al., 2014). Genes related to ethylene synthesis were also regulated in mangrove plants after waterlogging, but a differences existed. The SAMS, ACS, and ACCO of A. marina were upregulated in 3- and 14-day exposure periods. The ethylene synthesis efficiency was improved, indicating that A. marina had sufficient response and adaptability to waterlogging. The upregulated counts of genes related to ethylene synthesis in B. gymnorrhiza was relatively low but still higher than those in Ae. corniculatum. There are descriptions of the difference of formation mechanism and physiological structure, for example, lysigenous aerenchyma formed by programmed cell death while schizogenous aerenchyma formed by the splitting of the connected common cell wall (Ma et al., 2016). The complexity of aerenchyma is also reflected in this study, noting that Ae. corniculatum might be deficient in ethylene synthesis and forming aerenchyma in a different way.
In cell wall process (GO: 0005618, Figure 7), it was found that there are almost no genes upregulated in Ae. corniculatum after a 3-day exposure, and XET genes expression was inhibited. XET is important in cell wall dynamics and plays a key role in the assembly and modifications of cell walls under biotic and abiotic (environmental) stresses (Hrmova et al., 2022). The results demonstrated the difference of cell wall structure regulation in Ae. corniculatum at the early stage (3 days), and the weakness would be addressed through other pathways such as metabolic regulation.
5. Conclusions
To our knowledge, this was the first time the waterlogging adaptive mechanisms of A. Marina, Ae. corniculatum, and B. gymnorrhiza were explored and compared at the transcriptome level. Genes with high differentially expressed folds indicated that the three mangrove plants could commonly regulate from the four aspects: structure, transportation, biosynthesis, and stress protection. Interspecific differences of the three species under continuous waterlogging were observed in this study. The response intensity at transcriptome level was relevant to the natural intertidal distribution of the population. Species naturally distributed closer to the sea in the intertidal zone with a stronger response at the transcriptome level. The number of DEGs was the highest in A. Marina under waterlogging stress, followed by Ae. corniculatum and B. gymnorrhiza. As the pioneer, A. Marina could extensively and abundantly express genes that can shift metabolic pathways and change root morphology, to meet the challenge from submergence. B. gymnorrhiza, which was more sensitive to the submergence, oppositely responded to waterlogging in a gentle way. As for related pathways, genes related to starch and sucrose metabolism were active in A. Marina to ensure glucose supply. Combined with active glycolysis, we speculated that A. Marina could maintain ATP production under stress, which also explained its active biosynthesis process under hypoxia. The relative expression of AcADH and AcLDH was prominent, which might play an important role in the adaptability of Ae. corniculatum. Being different from A. Marina and B. gymnorrhiza, genes involved in ethylene synthesis, aerenchyma formation, and cell wall structure were regulated in a different way in Ae. corniculatum, indicating a specific regulation mechanism of cell wall structure in Ae. corniculatum. The full-length cDNA of ADHs and LDHs was isolated from the three mangrove plants and then, qRT-PCR was performed. The result further emphasized the interspecific differences among the three mangroves.
Data availability statement
The datasets presented in this study can be found in online repositories. The names of the repository/repositories and accession number(s) can be found in the article/Supplementary Material.
Author contributions
B-YS wrote the manuscript and undertook the experiments. Y-SW designed, conceptualized, and supervised the manuscript modification and also undertook the experiments. C-CS also undertook the experiments. All authors contributed to the article and approved the submitted version.
Funding
This research was supported by the International Partnership Program of Chinese Academy of Sciences (No. 133244KYSB20180012), the National Natural Science Foundation of China (Nos. U1901211 and 41876126), the Strategic Priority Research Program of the Chinese Academy of Sciences (Nos. XDA23050200 and XDA19060201), and the National Key Research and Development Program of China (Science & Technology Basic Resources Investigation Program of China, No. 2017FY100700).
Conflict of interest
The authors declare that the research was conducted in the absence of any commercial or financial relationships that could be construed as a potential conflict of interest.
Publisher’s note
All claims expressed in this article are solely those of the authors and do not necessarily represent those of their affiliated organizations, or those of the publisher, the editors and the reviewers. Any product that may be evaluated in this article, or claim that may be made by its manufacturer, is not guaranteed or endorsed by the publisher.
References
Al-Khayat J. A., Alatalo J. M. (2021). Relationship between tree size, sediment mud content, oxygen levels, and pneumatophore abundance in the mangrove tree species avicennia Marina (Forssk.) vierh. J. Mar. Sci. Eng. 9, 100. doi: 10.3390/jmse9010100
Allen C. D., Macalady A. K., Chenchouni H., Bachelet D., Mcdowell N., Vennetier M., et al. (2010). A global overview of drought and heat-induced tree mortality reveals emerging climate change risks for forests. For. Ecol. Manage. 259, 660–684. doi: 10.1016/j.foreco.2009.09.001
Almahasheer H., Duarte C. M., Irigoien X. (2016). Phenology and growth dynamics of avicennia marina in the central red Sea. Sci. Rep. 6, 37785. doi: 10.1038/srep37785
Arora K., Panda K. K., Mittal S., Mallikarjuna M. G., Rao A. R., Dash P. K., et al. (2017). RNAseq revealed the important gene pathways controlling adaptive mechanisms under waterlogged stress in maize. Sci. Rep. 7, 10950. doi: 10.1038/s41598-017-10561-1
Benjamini Y., Hochberg Y. (1995). Controlling the false discovery rate: a practical and powerful approach to multiple hypothesis testing. J. R. Stat. Society: Ser. B (Methodological) 57, 289–300. doi: 10.1111/j.2517-6161.1995.tb02031.x
Bielecki S., Somiari R. I. (1998). Enhancement of invertase activity in organic media for oligosaccharide synthesis. Progress in biotechnology. 15, 423-428. Available at: https://doi.org/10.1016/S0921-0423(98)80062-2
Blokhina O. B., Chirkova T. V., Fagerstedt K. V. (2001). Anoxic stress leads to hydrogen peroxide formation in plant cells. J. Exp. Bot. 52, 1179–1190. doi: 10.1093/jexbot/52.359.1179
Bonaventure G., Salas J. J., Pollard M. R., Ohlrogge J. B. (2003). Disruption of the FATB gene in arabidopsis demonstrates an essential role of saturated fatty acids in plant growth. Plant Cell 15, 1020–1033. doi: 10.1105/tpc.008946
Cheng H., Wu M. L., Li C. D., Sun F. L., Sun C. C., Wang Y. S. (2020). Dynamics of radial oxygen loss in mangroves subjected to waterlogging. Ecotoxicology 29, 684–690. doi: 10.1007/s10646-020-02221-4
Cho H.-Y., Loreti E., Shih M.-C., Perata P. (2021). Energy and sugar signaling during hypoxia. New Phytol. 229, 57–63. doi: 10.1111/nph.16326
Dolferus R., Klok E. J., Ismond K., Delessert C., Wilson S., Good A., et al. (2001). Molecular basis of the anaerobic response in plants. IUBMB Life 51, 79–82. doi: 10.1080/15216540152122058
Dolferus R., Wolansky M., Carroll R., Miyashita Y., Ismond K., Good A. (2008). Functional analysis of lactate dehydrogenase during hypoxic stress in arabidopsis. Funct. Plant Biol. 35, 131–140. doi: 10.1071/FP07228
Evans D. E. (2004). Aerenchyma formation. New Phytol. 161, 35–49. doi: 10.1046/j.1469-8137.2003.00907.x
Geigenberger P. (2003). Response of plant metabolism to too little oxygen. Curr. Opin. Plant Biol. 6, 247–256. doi: 10.1016/S1369-5266(03)00038-4
Gibberd M. R., Gray J. D., Cocks P. S., Colmer T. D. (2001). Waterlogging tolerance among a diverse range of trifolium accessions is related to root porosity, lateral root formation and ‘Aerotropic rooting’. Ann. Bot. 88, 579–589. doi: 10.1006/anbo.2001.1506
Gibbs J., Greenway H. (2003). Review: Mechanisms of anoxia tolerance in plants. i. growth, survival and anaerobic catabolism. Funct. Plant Biol. 30, 1–47. doi: 10.1071/PP98095
Goel P., Singh A. K. (2015). Abiotic stresses downregulate key genes involved in nitrogen uptake and assimilation in brassica juncea l. PLoS One 10, e0143645. doi: 10.1371/journal.pone.0143645
Good A. G., Paetkau D. H. (1992). Identification and characterization of a hypoxically induced maize lactate dehydrogenase gene. Plant Mol. Biol. 19, 693–697. doi: 10.1007/BF00026795
Herger A., Dünser K., Kleine-Vehn J., Ringli C. (2019). Leucine-rich repeat extensin proteins and their role in cell wall sensing. Curr. Biol. 29, R851–r858. doi: 10.1016/j.cub.2019.07.039
Hovenden M. J., Allaway W. G. (1994). Horizontal structures on pneumatophores of avicennia marina (Forsk.) vierh.–a new site of oxygen conductance. Ann. Bot. 73, 377–383. doi: 10.1006/anbo.1994.1047
Hrmova M., Stratilová B., Stratilová E. (2022). Broad specific Xyloglucan:Xyloglucosyl transferases are formidable players in the re-modelling of plant cell wall structures. Int. J. Mol. Sci. 23, 1656. doi: 10.3390/ijms23031656
Huang B., Liu J.-Y. (2006). A cotton dehydration responsive element binding protein functions as a transcriptional repressor of DRE-mediated gene expression. Biochem. Biophys. Res. Commun. 343, 1023–1031. doi: 10.1016/j.bbrc.2006.03.016
Juntawong P., Sirikhachornkit A., Pimjan R., Sonthirod C., Sangsrakru D., Yoocha T., et al. (2014). Elucidation of the molecular responses to waterlogging in jatropha roots by transcriptome profiling. Front. Plant Sci. 5. doi: 10.3389/fpls.2014.00658
Kierans S. J., Taylor C. T. (2021). Regulation of glycolysis by the hypoxia-inducible factor (HIF): implications for cellular physiology. J. Physiol. 599, 23–37. doi: 10.1113/JP280572
Kreuzwieser J., Hauberg J., Howell K. A., Carroll A., Rennenberg H., Millar A. H., et al. (2009). Differential response of gray poplar leaves and roots underpins stress adaptation during hypoxia. Plant Physiol. 149, 461–473. doi: 10.1104/pp.108.125989
Lewis N. G., Davin L. B. (1999). 3.18@ the nature and function of lignins. Pergamon Press (Oxford, England)
Lipaeva P., Vereshchagina K., Drozdova P., Jakob L., Kondrateva E., Lucassen M., et al. (2021). Different ways to play it cool: Transcriptomic analysis sheds light on different activity patterns of three amphipod species under long-term cold exposure. Mol. Ecol. 30, 5735–5751. doi: 10.1111/mec.16164
Liu L., Fan H.-Q., Li C.-G. (2012). Tide elevations for four mangrove species along western coast of guangxi, China. Shengtai Xuebao/ Acta Ecologica Sin. 32, 690–698. doi: 10.5846/stxb201107131038
Loreti E., Poggi A., Novi G., Alpi A., Perata P. (2005). A genome-wide analysis of the effects of sucrose on gene expression in arabidopsis seedlings under anoxia. Plant Physiol. 137, 1130–1138. doi: 10.1104/pp.104.057299
Maricle B. R., Lee R. W. (2002). Aerenchyma development and oxygen transport in the estuarine cordgrasses spartina alterniflora and s. anglica. Aquat. Bot. 74, 109–120. doi: 10.1016/S0304-3770(02)00051-7
Ma Y.-L., Zhou M.-X., Shabala S., Li C.-D. (2016). “Exploration and utilization of waterlogging-tolerant barley germplasm,” in Exploration, identification and utilization of barley germplasm, Academic Press (Salt Lake City UT, USA) 135–179.
Parkhomchuk D., Borodina T., Amstislavskiy V. (2009). Transcriptome analysis by strand-specific sequencing of complementary DNA. Nucleic Acids Res. 37, 123. doi: 10.1093/nar/gkp596
Paul A. L., Ferl R. J. (1991). In vivo footprinting reveals unique cis-elements and different modes of hypoxic induction in maize Adh1 and Adh2. Plant Cell 3, 159–168. doi: 10.1105/tpc.3.2.159
Peretto R., Favaron F., Bettini V., De Lorenzo G., Marini S., Alghisi P., et al. (1992). Expression and localization of polygalacturonase during the outgrowth of lateral roots in allium porrum l. Planta 188, 164–172. doi: 10.1007/BF00216810
Phan H. A., Iacuone S., Li S. F., Parish R. W. (2011). The MYB80 transcription factor is required for pollen development and the regulation of tapetal programmed cell death in arabidopsis thaliana. Plant Cell 23, 2209–2224. doi: 10.1105/tpc.110.082651
Purnobasuki H., Suzuki M. (2004). Aerenchyma formation and porosity in root of a mangrove plant, sonneratia alba (Lythraceae). J. Plant Res. 117, 465–472. doi: 10.1007/s10265-004-0181-3
Serrato A. J., Guilleminot J., Meyer Y., Vignols F. (2008). AtCXXS: atypical members of the arabidopsis thaliana thioredoxin h family with a remarkably high disulfide isomerase activity. Physiologia Plantarum 133, 611–622. doi: 10.1111/j.1399-3054.2008.01093.x
Shen C., Yuan J., Ou X., Ren X., Li X. (2021). Genome-wide identification of alcohol dehydrogenase (ADH) gene family under waterlogging stress in wheat (Triticum aestivum). PeerJ 9, e11861. doi: 10.7717/peerj.11861
Srikanth S., Lum S. K. Y., Chen Z. (2016). Mangrove root: adaptations and ecological importance. Trees 30, 451–465. doi: 10.1007/s00468-015-1233-0
Takahashi H., Yamauchi T., Colmer T. D., Nakazono M. (2014). “Aerenchyma formation in plants,” in Low-oxygen stress in plants: Oxygen sensing and adaptive responses to hypoxia. Eds. Van Dongen ,. J. T., Licausi F. (Vienna: Springer Vienna).
Tian Q.-Y., Sun P., Zhang W.-H. (2009). Ethylene is involved in nitrate-dependent root growth and branching in arabidopsis thaliana. New Phytol. 184, 918–931. doi: 10.1111/j.1469-8137.2009.03004.x
Vartapetian B. (2005). Plant anaerobic stress as a novel trend in ecological physiology, biochemistry, and molecular biology: 1. establishment of a new scientific discipline. Russian J. Plant Physiol. 52, 826–844. doi: 10.1007/s11183-005-0122-6
Vartapetian B. B. (2006). Plant anaerobic stress as a novel trend in ecological physiology, biochemistry, and molecular biology: 2. further development of the problem. Russian J. Plant Physiol. 53, 711–738. doi: 10.1134/S102144370606001X
Vincill E., Clarin A., Molenda J., Spalding E. (2013). Interacting glutamate receptor-like proteins in phloem regulate lateral root initiation in arabidopsis. Plant Cell 25, 1304–1313. doi: 10.1105/tpc.113.110668
Wang Y. S., Gu J. D. (2021). Ecological responses, adaptation and mechanisms of mangrove wetland ecosystem to global climate change and anthropogenic activities. Int. Biodeterioration Biodegradation 162, 105248. doi: 10.1016/j.ibiod.2021.105248
Wen Y.-G., Liu S.-R., Yuan C.-A. (2002). The population distribution of mangrove at yingluogang of Guangxi,China. Acta Ecologica Sin. 22, 1160–1165. doi: 10.3321/j.issn:1000-0933.2002.07.027
Yahia E., Carrillo-Lopez A. (2018). Postharvest physiology and biochemistry of fruits and vegetables. Woodhead Publishing (Sawston, Cambridge, England)
Yang W., Pollard M., Li-Beisson Y., Beisson F., Feig M., Ohlrogge J. (2010). A distinct type of glycerol-3-phosphate acyltransferase with sn-2 preference and phosphatase activity producing 2-monoacylglycerol. Proc. Natl. Acad. Sci. 107, 12040–12045. doi: 10.1073/pnas.0914149107
Ye Y., Tam N. F. Y., Wong Y. S., Lu C. Y. (2003). Growth and physiological responses of two mangrove species (Bruguiera gymnorrhiza and kandelia candel) to waterlogging. Environ. Exp. Bot. 49, 209–221. doi: 10.1016/S0098-8472(02)00071-0
Zhang Q.-M., Sui S.-Z., Zhang Y.-C., Yu H.-B., Wen X.-S. (2001). Marine environmental indexes related to mangrove growth. Acta Ecologica Sin. 21, 1427–1437. doi: 10.3321/j.issn:1000-0933.2001.09.005
Zheng D., Han X., An Y. I., Guo H., Xia X., Yin W. (2013). The nitrate transporter NRT2.1 functions in the ethylene response to nitrate deficiency in arabidopsis. Plant Cell Environ. 36, 1328–1337. doi: 10.1111/pce.12062
Keywords: mangrove plants, waterlogging stress, response mechanism, transcriptome, gene expression
Citation: Su B-Y, Wang Y-S and Sun C-C (2022) Response adaptive mechanisms of three mangrove (Avicennia marina, Aegiceras corniculatum, and Bruguiera gymnorrhiza) plants to waterlogging stress revealed by transcriptome analysis. Front. Mar. Sci. 9:929649. doi: 10.3389/fmars.2022.929649
Received: 27 April 2022; Accepted: 29 November 2022;
Published: 21 December 2022.
Edited by:
Naser A. Anjum, Aligarh Muslim University, IndiaReviewed by:
Hery Purnobasuki, Airlangga University, IndonesiaXiuping Lin, Key Laboratory of Tropical Marine Bio-resources and Ecology (CAS), China
Copyright © 2022 Su, Wang and Sun. This is an open-access article distributed under the terms of the Creative Commons Attribution License (CC BY). The use, distribution or reproduction in other forums is permitted, provided the original author(s) and the copyright owner(s) are credited and that the original publication in this journal is cited, in accordance with accepted academic practice. No use, distribution or reproduction is permitted which does not comply with these terms.
*Correspondence: You-Shao Wang, eXN3YW5nQHNjc2lvLmFjLmNu; Cui-Ci Sun, c2N1aWNpQHNjc2lvLmFjLmNu