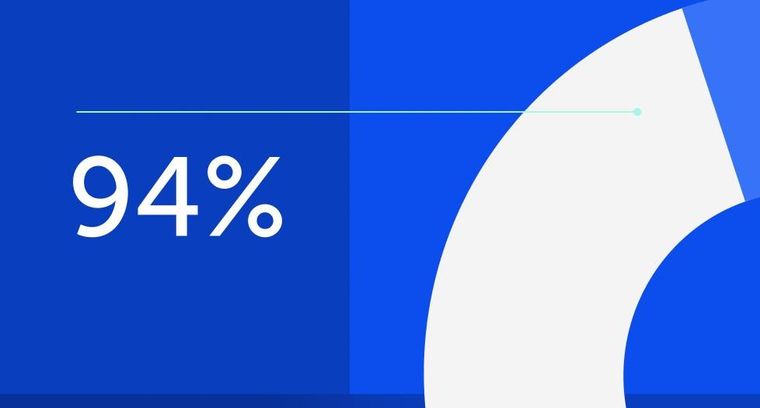
94% of researchers rate our articles as excellent or good
Learn more about the work of our research integrity team to safeguard the quality of each article we publish.
Find out more
ORIGINAL RESEARCH article
Front. Mar. Sci., 03 August 2022
Sec. Marine Evolutionary Biology, Biogeography and Species Diversity
Volume 9 - 2022 | https://doi.org/10.3389/fmars.2022.928617
This article is part of the Research TopicEcological and Genetic Insights into Seaweeds’ Diversity and AdaptationView all 7 articles
The cultivation of hyposaline-tolerant varieties of the red alga Pyropia haitanensis is not only conducive to expanding the area of intertidal seaweed cultivation, but also contributes to preventing eutrophication of coastal waters. Research on the mechanism of hyposaline tolerance of P. haitanensis is an important prerequisite for breeding hyposaline-tolerant varieties. Here, we used proteomics and targeted metabolomics technologies to identify the key proteins and metabolites in thalli of P. haitanensis that changed under two hyposaline stress treatments: 0‰, LSS 0; 5‰, LSS 5. Responses of thalli of P. haitanensis to hyposaline stress included to inhibit protein synthesis, recruit molecular chaperones, and enhance the removal of misfolded proteins to maintain the dynamic balance of protein folding and removal; the response was similar under hypersaline stress. Glycolysis was the main energy supply pathway, and thalli actively maintained the stability of the cell membrane under hyposaline stress, which was distinct from the response to hypersaline stress. Compared with the LSS 0 treatment, P. haitanensis exhibited a more adequate energy supply, more stable endoplasmic reticulum environment and more intact membrane system under the LSS 5 treatment. The results improve understanding of the hyposaline tolerance mechanism of intertidal seaweed and provide a theoretical basis for the development of hyposaline-tolerant varieties.
Because of its delicious taste and high nutritional value, Pyropia/Porphyra (recently named by Neoporphyra) is widely cultivated and has become one of the most important cultivated seaweeds in the world, creating considerable economic benefits. Pyropia can absorb nutrient elements such as N and P from seawater during cultivation (Pedersen et al., 2004), thus providing significantly economic and ecological benefits. However, in the cultivation process, the growth of thalli is limited by water flow and nutrients. The estuarine area environment not only has smooth water flow but is also rich in nutrients, which is very suitable for Pyropia/Porphyra cultivation. Because of the inflow of river water, precipitation and other reasons, the salinity in the estuary environment fluctuates greatly (Bricker et al., 2008). Therefore, breeding a strain of Pyropia/Porphyra with hyposaline tolerance and cultivating it in the estuary area would be valuable for improving the yield and quality of thalli and alleviating eutrophication in estuaries (He et al., 2018).
Pyropia haitanensis, a species native to China, accounts for approximately 70% of the total annual output of Pyropia in China (Fishery Department of China, 2021). To acclimate to hyposaline stress (5‰), P. haitanensis was able to prevent the accumulation of large amounts of reactive oxygen species by enhancing the activity of antioxidant enzymes and content of antioxidant substances; the thalli were found to maintain the homeostasis of protein folding by up-regulating the expression of heat-shock protein (HSPs)-related genes; It was also able to retain the balance of osmotic pressure inside and outside the cells by down-regulating the expression of genes related to the synthesis of osmotic substances such as proline, glutamic acid, and trehalose, ultimately ensuring the survival of the organism under hyposaline stress (Wang et al., 2020). However, under hyposaline stress, the response mechanism that enables P. haitanensis to maintain protein synthesis, membrane system homeostasis and energy supply is still unclear. Since P. haitanensis mainly lives in the intertidal zone, it will periodically experience hypersaline stress along with the tidal changes. After rehydration at high tide, P. haitanensis was still able to quickly return to the normal physiological state and showed strong resistance to hypersaline stress (Blouin et al., 2011; Lu et al., 2016). Our previous studies proved that P. haitanensis can maintain its homeostasis by transporting Na+ across the cell membrane, accumulating osmotic substances, and increasing antioxidant enzyme activities under hypersaline stress (Wang et al., 2019). P. haitanensis can maintain vital cellular functions under hypersaline stress by enhancing its own energy metabolism and protein processing ability through changes in the proteome (Wen et al., 2020). However, there have been few studies of the similarities and differences in the response mechanisms of P. haitanensis to hypersaline and hyposaline stresses.
Proteins constitute the biochemical machinery and functional processes enabling organisms to respond to changing environmental conditions. A systematic analysis of proteins through proteomics-based research is an effective way to elucidate the adaptations of organisms to abiotic stresses (Chen and Harmon, 2006). Differing from the traditional data-dependent analyses, data-independent acquisition (DIA) technology can obtain information about all components in the sample without omission, greatly improving the data availability and reducing missing values, and is more suitable for protein detection with large sample size and complex systems (Law and Lim, 2013). Traditional quantitative immunoassay (ELISA, etc.) cannot meet the needs of large-scale target protein validation, whereas multiple reaction monitoring (MRM) is characterized by high sensitivity, good reproducibility and high flux (Kennedy et al., 2014); an effective research strategy is to use MRM to verify proteomics data. Additionally, metabolomics technology can describe the metabolic state of organisms through quantitative analysis of small molecular metabolites. Compared with untargeted metabolomics, targeted metabolomics focuses on the qualitative and quantitative analysis of specific metabolites (Roberts et al., 2012). Therefore, the current study applied DIA technology to screen key proteins with regulatory functions in P. haitanensis thalli under different degrees of hyposaline stress; proteins were then verified by combining MRM technology and targeted metabolomics. The similarities and differences between molecular response mechanisms to hypersaline stress and hyposaline stress were also determined by trend analysis. The present results provide data to underpin research on salt-tolerant molecular mechanisms of Pyropia and breeding salt-tolerant varieties.
The P. haitanensis strain Z-61 analyzed in this study was isolated and purified in the Laboratory of Germplasm Improvements and Applications of Pyropia at Jimei University, Fujian province, China (Chen et al., 2008). Three thalli were cultured in a 500mL aerated flask containing natural seawater and Provasoli’s enrichment solution at 21 ± 1 °C with a 12-h light: 12-h dark photoperiod (50 – 60 μmoL m−2 s−1 photons). The medium was changed every 2 days. When the thalli grew to 20 ± 2 cm, they were exposed to hyposaline treatments by adding water into the natural seawater. In a previous study, we observed that P. haitanensis can acclimate to 5‰ hyposaline stress (LSS 5; approximately 85 mM Na+) treatments, whereas 0‰ hyposaline stress (LSS 0; approximately 0 mM Na+) treatments were sub-lethal for thalli, which can subsequently recover in normal seawater (control; approximately 500 mM Na+). Therefore, we selected 5‰ and 0‰ as hyposaline stress treatments. After 4-h hyposaline stress (LSS 0, LSS 5), and normal treatments, the thalli were harvested and immediately frozen in liquid nitrogen, and then stored at −80 °C. Each treatment was completed with three biological replicates.
Methods for protein extraction, protein hydrolysis, and data analysis were as described previously (Wen et al., 2020). Thereafter, differentially expressed proteins (DEPs) were screened by using a fold change (FC) ≥ 1.5 and P< 0.05 as the criteria for determining significant differences.
The MRM analyses were completed with a QTRAP 6500 mass spectrometer (SCIEX, Framingham, MA, USA) equipped with the LC-20 AD nanoHPLC system (Shimadzu, Kyoto, Japan). The generated raw data file was integrated with Skyline software. All transitions for each peptide were used for quantification unless interference from the matrix was detected. A sample spiked with β-galactosidase was used for label-free data normalization. We used MSstats with the linear mixed effect model, and the P values were adjusted to control the false discovery rate at a threshold of 0.05. All proteins with at least a 1.5-fold difference in abundance (P < 0.05) were considered significant.
A series test-of-cluster analysis was performed by Short Time-series Expression Miner software (Ernst and Bar-Joseph, 2006) on the OmicShare tools platform (www.omicshare.com/tools). The parameters were set as follows: maximum unit change in model profiles between time points = 1; maximum output profiles number = 8; minimum ratio of FC of DEPs = 1.5.
Approximately 200 mg of sample (including control, LSS 0, LSS 5 and 100‰ hypersaline treatment) was weighed and the fatty acids (FAs) content of the thallus was determined as described previously (Li et al., 2006). The oil content was calculated as (S1/S2)×N/M, where S1 = total peak area; S2 = internal standard peak area; N = internal standard dosage; M = sample weight.
Approximately 100 mg of tissue sample was weighed and lyophilized then ground in a 2-mL Eppendorf tube containing a 5-mm tungsten bead for 1 min at 65 Hz in a grinding mill. Metabolites were extracted using 1 mL precooled mixture of methanol, acetonitrile and water (2:2:1 v/v/v) and then placed for 1 h with ultrasonic shaking in an ice bath. Subsequently, the mixture was placed at −20°C for 1 h and centrifuged at 16,000 g for 20 min at 4°C. The supernatants were recovered and concentrated to dryness in vacuum.
A Shimadzu Nexera X2 LC-30AD HPLC was used for separation with mobile phase A: 5% acetonitrile aqueous solution, 10 mM ammonium acetate, pH 9; mobile phase B: 95% acetonitrile aqueous solution, 10 mM ammonium acetate, pH 9. The sample was re-dissolved in 100 µL 50% aqueous methanol. The autosampler was kept at 4°C, the injection volume was 5 µL, the column temperature was 40°C, and the flow rate was 300 µL/min. The mobile phase gradients were: 0–2 min, B maintained at 95%; 2–9 min, B changed linearly from 95% to 70%; 9–10 min, B changed linearly from 70% to 30%; 10–11 min, B maintained at 30%; 11–11.5 min, B solution changed linearly from 30% to 95%; 11.5–15 min, B solution maintained at 95%. One QC sample was set before the start of the sample queue, then one QC sample added for every three samples, and one QC sample after the end of the sample queue to test and evaluate the stability and repeatability of the system.
2,425 proteins were identified from 19,878 peptide fragments (Supplementary Tables 1). To evaluate the reproducibility of the DIA data, a principal component analysis was conducted involving the three replicates for each salt stress treatment Figure 1A showed that the three principal components were separated clearly, PCA1, 2, and 3 accounted for 24.6%, 23.6%, and 13.8% of the total variation, respectively. The correlations among protein quantities between samples were determined based on Pearson correlation coefficients (Figure 1B). The results showed that the three repeated samples under the same treatment had high correlation (≥ 0.975).
Figure 1 Data-independent acquisition analysis of P. haitanensis exposed to hyposaline stress for 4 h. (A) Principal component analysis, with three replicate samples per treatment. (B) Heat map presenting sample correlations. CK, control; LSS 0, 0‰ hyposaline stress; LSS 5, 5‰ hyposaline stress. (C) Number of DEPs in each comparison. (D) Venn diagram of DEPs (increased abundance). (E) Venn diagram of DEPs (decreased abundance). Fold change ≥ 1.5 and P < 0.05.
Compared with the control, a total of 407 DEPs were identified, including 253 and 242 DEPs under the LSS 0 and LSS 5 treatments, respectively (Figure 1C). The abundance of 40 proteins increased and 41 decreased under both stresses (Figures 1D, E). Compared with the LSS 0 treatment, the LSS 5 treatment resulted in more proteins with increased abundance (117 proteins) and fewer proteins with decreased abundance (44 proteins).
Twenty DEPs were randomly selected from the sequenced proteome database, and their abundance under different hyposaline stress treatments was determined with the MRM method to verify the accuracy of the DIA results. The results indicated that the MRM abundance trend of 19 proteins was essentially consistent with the results of DIA (Supplementary Figure 1).
KEGG enrichment of the DEPs showed that amino acid biosynthesis and carbon metabolism contained the most DEPs, each with 18 proteins, followed by the carbon fixation of ribosomes and photosynthetic organisms, which contained 15 and 9 proteins, respectively, under the LSS 0 treatment (Supplementary Figure 2A). Under the LSS 5 treatment, the biosynthesis of secondary metabolites contained the most DEPs, with 37 proteins, followed by carbon metabolism, amino acid biosynthesis, and carbon fixation by photosynthetic organisms, with 19, 17, and 8 proteins, respectively (Supplementary Figure 2B). Accordingly, the DEPs in the carbon metabolism pathway, protein synthesis and processing, and fatty acid synthesis pathway were analyzed in more detail (Supplementary Figure 3).
Compared with the control, LSS 0 treatment inhibited the expression of 9 elongation factor thermo-unstable (EF-Tu) proteins and 4 signal recognition particle analogs Fifty-four-homology (Ffh) proteins; LSS 5 treatment inhibited the expression of 4 EF-Tu proteins and 2 Ffh proteins (Figure 2). The proteins calnexin (CNX), heat shock 70kDa protein (HSP70), heat shock 90kDa protein (HSP90) in the endoplasmic reticulum quality control (ERQC) category, and BiP in the endoplasmic reticulum-associated degradation (ERAD) category were down-regulated expression under the LSS 0 treatment, while were up-regulated expression under the LSS 5 treatment (Figure 2). Additionally, compared with the LSS 0 treatment, 10 proteins related to ubiquitin activating enzyme (E1), ubiquitin binding enzyme (E2), ubiquitin ligase (E3) and 26S proteasome were up-regulated under the LSS 5 treatment.
Figure 2 Response metabolism map of P. haitanensis under hyposaline stress. Red lines represent the carbon metabolism pathway, green lines represent the protein synthesis and processing pathway, and purple lines represent fatty acid metabolism. Values in the tables are changes in content of selected proteins; the left, middle and right columns give values for LSS 0 vs. control, LSS 5 vs. control, and LSS 5 vs. LSS 0 treatments, respectively. The colors reflect the protein content differences relative to the protein’s abundance under control conditions: red, blue, and white correspond to increased, decreased, and unchanged protein contents, respectively. Numbers represent log2 fold change (FC) values for FC ≥ 1.5 and P < 0.05, which are considered significant.
Compared with the control, acetyl-CoA carboxylase was up-regulated in the LSS 5 treatment and down-regulated in the LSS 0 treatment, respectively, while 3-oxoacyl-ACP reductase was up-regulated under both treatments (Figure 2). Furthermore, 16 DEPs were annotated in the carbon metabolism pathway under hyposaline stress. Glucose-6-phosphate isomerase, phosphoglycerate kinase, enolase, transketolase and phosphoribulokinase were up-regulated under both hyposaline treatments. In contrast, triosephosphate isomerase was down-regulated under both treatments. In addition, phosphofructokinase, pyruvate dehydrogenase and fructose-1,6-bisphosphatase were up-regulated only under LSS 5 treatment, while pyruvate kinase was up-regulated only under LSS 0 treatment. Furthermore, isocitrate dehydrogenase and 2-oxoglutarate dehydrogenase were down-regulated only under LSS 0 treatment (Figure 2).
Compared with the control treatment, only the LSS 5 treatment significantly increased the FAs in P. haitanensis thalli (Figure 3A), while the unsaturated fatty acid contents (UFA), unsaturated fatty acid/saturated fatty acid (UFA/SFA) and index of unsaturated fatty acid (IUFA) values showed no significant differences under the two hyposaline treatments (Figures 3B–D). Additionally, compared with the normal treatment and the LSS 0 treatment, the contents of glucose 6-phosphate and pyruvic acid were significantly increased under the LSS 5 treatment (Figures 3E–H).
Figure 3 Effects of hyposaline stress on fatty acid (FA) content and glycolytic intermediates of P. haitanensis. (A) Content of total FAs. (B) Changes in saturated FA (SFA) and unsaturated FA (UFA) content. (C) Ratio of UFA to SFA. (D) Changes of IUFA value of P. haitanensis under hyposaline stress, where (Si, relative content of UFAs; ti, the number of unsaturated bonds contained in the UFAs). Data are mean ± SE for at least three independent biological replicates. Different letters above the bars indicate significant differences among treatments at P< 0.05, as determined by one-way ANOVA followed by Tukey’s test. (E) Glucose 6-phosphate content. (F) Fructose 6-phosphate content. (G) Fructose 1,6-bisphosphate content. (H) Pyruvate content. Data are mean ± SE for at least three independent biological replicates. Different letters above the bars indicate significant differences among treatments at P < 0.05, as determined by one-way ANOVA followed by Tukey’s test.
Compared with the control treatment, a total of 1,094 DEPs were detected under the LSS 5 and 100‰ hypersaline stress (HSS 100; approximately 1,700 mM Na+) (Wen et al., 2020) treatments. The DEPs included 242 under the LSS 5 treatment and 1,009 under the HSS 100 treatment (Wen et al., 2020) (Figure 4A). Among them, 109 proteins were up-regulated and 14 proteins were down-regulated under both treatments (Figures 4B, C). Eight model profiles were obtained by the test-of-cluster analysis, among which Profile 4 (761 proteins, up-regulated under HSS 100 treatment), Profile 3 (104 proteins, down-regulated under HSS 100 treatment), Profile 2 (98 proteins, up-regulated under both treatments) and Profile 7 (34 proteins, down-regulated under LSS 5 treatment and up-regulated under HSS 100 treatment) had significant trend characteristics (Figure 4D and Supplementary Table 2).
Figure 4 Analysis of DEPs in P. haitanensis exposed to hypersaline and hyposaline treatments for 4 h. (A) Number of DEPs in each comparison. (B) Venn diagram of DEPs (increased abundance). (C) Venn diagram of DEPs (decreased abundance). (D) The expression patterns of 1,094 proteins analyzed by model profile. The expression patterns of 1,094 proteins were analyzed and eight model profiles were used to summarize the data. Each box represents a model expression profile. The upper number in the profile box is the model profile number and the lower one is the number of proteins contained; four expression patterns showed significant P values (colored boxes, FC ≥ 1.5 and P < 0.05). (E) Effects of hypersaline and hyposaline treatments on amino acid synthesis, energy metabolism, FA synthesis, protein synthesis and processing of P. haitanensis (Profile 4: green; Profile 3: blue; Profile 2: red; Profile 7: gray).
The KEGG enrichment analysis of the proteins in the above four model profiles revealed that most of the DEPs in each profile were closely related to amino acid metabolism, energy metabolism, lipid metabolism, and protein folding and degradation (Supplementary Figures 4A–D). Further statistical analysis of the DEPs in each profile showed that, compared with the LSS 5 treatment, 54 proteins related to amino acid metabolism, 27 proteins related to energy metabolism, 24 proteins related to lipid metabolism, and 71 proteins related to protein folding and degradation were up-regulated under HSS 100 treatment (Figure 4E).
Compared with the control treatment, the LSS 5 and HSS 100 treatments significantly increased the total FAs in P. haitanensis (Figure 5A). However, there was no significant difference in UFA/SFA and IUFA values of thalli under the LSS 5 treatment, while UFA/SFA and IUFA values showed a significant increasing trend under the HSS 100 treatment (Figures 5B–D). The SFA in thalli of P. haitanensis were composed mainly of palmitic acid (C16:0), while the UFA were composed mainly of eicosapentaenoic acid [C20:5(n-3)], arachidonic acid [C20:4(n-6)] and palmitoleic acid (C16:1); the contents of various FAs were also significantly different under the three treatments (Figures 5E).
Figure 5 Changes in fatty acids (FAs) and related indexes of P. haitanensis after hypersaline and hyposaline treatments for 4 h. (A) Content of total FAs. (B) Changes in saturated FA (SFA) and unsaturated FA (UFA) contents. (C) Ratio of UFAs to SFAs. (D) Changes of IUFA value under salt stress; see Figure 3 for definition of IUFA. (E) The content of various FAs under hypersaline and hyposaline treatments. Different letters above the bars indicate significant differences among treatments at P < 0.05, as determined by one-way ANOVA followed by Tukey’s test. CK, control treatment.
Abiotic stress increases the probability of misfolded or unfolded proteins in organisms, and if these proteins cannot be dealt with in a timely way, they will affect normal physiological activities and even lead to cell death (Liu and Howell, 2010). Our previous study showed that P. haitanensis can maintain the homeostasis of re-folding and degrading of damaged proteins under heat stress, hypersaline stress (Shi et al., 2017; Wang et al., 2019; Wen et al., 2020). It suggested that maintenance of protein homeostasis is crucial for P. haitanensis to adapt to the harsh environment of the intertidal zone.
EF-Tu is an important factor involved in protein synthesis it can control protein synthesis by catalyzing the extension of amino acid chains on the ribosome (Fu et al., 2012). The overexpression of chloroplast EF-Tu in transgenic wheat resulted in improved protection of leaf proteins against thermal aggregation, reduced damage to thylakoid membranes and enhanced photosynthetic capability under heat stress (Fu et al., 2008). Ffh, an analog of SRP54, can recognize signal sequences in nascent peptide chains and mediate the binding of ribosomes to the endoplasmic reticulum membrane, playing an important role in protein synthesis (Walter and Blobel, 1980). From the persent results, we suggest that down-regulated expression of EF-Tu and Ffh-related proteins might inhibited protein synthesis of P. haitanensis to a certain extent (Figure 2). This response might help to avoid the accumulation of misfolded proteins in the thalli under hyposaline stress.
The ERQC system recognizes proteins in the endoplasmic reticulum via CNX and CRT proteins (Helenius and Aebi, 2004); correctly folded proteins are transported to the Golgi apparatus via COP II, while misfolded or unfolded proteins are assisted in refolding by the recruitment of molecular chaperones (Wang et al., 2004). Previous studies revealed that heterologous expression of rice calnexin OsCNX conferred drought tolerance in Nicotiana tabacum (Sarwat and Naqvi, 2013). Previous transcriptome analysis found that P. haitanensis up-regulated the expression of heat-shock protein-related genes in response to hyposaline stress (Wang et al., 2020). Here, we also found differential expressions of HSP70, HSP90 and COPII proteins in P. haitanensis under hyposaline treatments, which were down-regulated under the LSS 0 treatment but up-regulated under the LSS 5 treatment; protein disulfide isomerase was down-regulated only under the LSS 0 treatment (Figure 2). These results indicated that P. haitanensis was able to maintain protein folding and transport correctly folded proteins to the Golgi apparatus under the LSS 5 treatmen, thus, the thalli maintained a stable endoplasmic reticulum environment under the LSS 5 treatment.
Furthermore, the ubiquitin degradation pathway plays a central role in the removal of abnormal proteins under abiotic stress (Walter and Blobel, 1980). Under high temperature stress, P. haitanensis can eliminate damaged or misfolded proteins by inducing the expression of E1, E2, E3 and other ERAD-related genes to regulate protein synthesis (Wang et al., 2018). Similarly, P. haitanensis was able to maintain endoplasmic reticulum homeostasis under hypersaline stress by enhancing the expression of ubiquitination-related proteins and heat-shock proteins (Wen et al., 2020). Therefore, the up-regulated expression of E1, E2, E3, and 26S proteasome in P. haitanensis under the LSS 5 treatment suggested that P. haitanensis could effectively eliminate misfolded proteins and avoid or reduce the harm caused by endoplasmic reticulum stress (Figure 2).
Maintaining the integrity of the plasma membrane by changing the composition and proportions of membrane FAs under salt stress is essential for the normal functioning and growth of plant cells (Singh et al., 2009; Mansour, 2012; Anbu and Sivasankaramoorthy, 2013). Previous studies found that the unsaturation of FAs in membrane lipids was positively correlated with drought tolerance of olive trees (Sofo et al., 2004); stigmasterol treatment improved the stability of plasma membrane and photosynthetic efficiency, and thus enhanced salt tolerance of Linum usitatissimum L. seeds (Bassuany et al., 2014). Conversely, when the integrity of the plant plasma membrane is damaged, it increases the sensitivity of plants to salt stress (Huang, 2006). In the current study, more FAs were synthesized in P. haitanensis under the LSS 5 treatment than in the control (Figure 3A). P. haitanensis can also maintained the composition and ratio of SFAs and UFAs under hyposaline stress. The IUFA value reflects the fluidity of the plasma membrane: the larger the IUFA value, the greater the fluidity of the plasma membranes (Zhang et al., 2009). However, the IUFA value of P. haitanensis showed no significant difference between the control and the hyposaline treatment (Figures 3B–D). Accordingly, P. haitanensis thalli preferred to maintain the integrity of the plasma membrane by increasing the synthesis of FAs under hyposaline stress.
Stable energy metabolism is essential for the life activities of organisms under both normal and stress conditions. Under hyposaline stress, the up-regulated expression of phosphoribulokinase in P. haitanensis would promote the regeneration of RuBP (Figure 2), which plays an important role in the Calvin cycle. Hence, a stable supply of substrates is provided for the subsequent carbon metabolism reactions. In addition, a large number of DEPs were enriched in the glycolysis (EMP) pathway, and their expression tended to be up-regulated overall (Figure 2). Previous research has shown that, under dehydration stress, Pyropia orbicularis can provide energy through the EMP pathway (López–Cristoffanini et al., 2015). In this study, the up-regulated expression of phosphoglycerate kinase (PGK), enolase and pyruvate kinase (PK) also indicated that the EMP pathway of P. haitanensis responded positively to hyposaline stress. An increase in phosphofructokinase [PFK, the rate-limiting enzyme in the EMP pathway (Chitlaru and Pick, 1991)] and PK activities may significantly enhance salt tolerance of mangrove tree (Suzuki et al., 2005). But the up-regulated expression of PFK was found under the LSS 5 treatment. Furthermore, the contents of glucose 6-phosphate (initial reaction substrate) and pyruvate (final reaction product) under the LSS 5 treatment were significantly higher than those in the control and the LSS 0 treatment (Figures 3E, H). All these results indicated that the EMP pathway was important for energy supply in response to hyposaline stress. In a difference from the response to the LSS 0 treatment, the EMP pathway responded more positively to the LSS 5 treatment and provided more metabolites and energy for subsequent reactions.
The pentose phosphate pathway (PPP) can not only generate energy, but also feed its intermediate products, glyceraldehyde 3-phosphate and fructose 6-phosphate, into the EMP; Additionally, NADPH produced by the PPP can maintain photoelectron transmission in Enteromorpha prolifera, and thus improve the salt tolerance of thalli (Li et al., 2014). NADPH produced by PPP can also provide reducing power and then promote the synthesis of plant FAs (Xue et al., 2017). Moreover, desiccation stress induced up-regulation of the transketolase gene in P. haitanensis, and this transketolase gene significantly improved the tolerance of Chlamydomonas reinhardtii to hyperosmotic stress (Shi et al., 2019). Accordingly, the up-regulated expression of transketolase fructose-1,6-bisphosphatase (FBP) and glucose-6-phosphate isomerase (GPI) might enable the thalli to meet the demand for carbon metabolism intermediates under hyposaline stress in a flexible way under LSS 5 treatment.
The trend analysis showed that the proteins enriched in Profile 2 were up-regulated under both hypersaline and hyposaline treatments, which were mainly involved in endoplasmic reticulum protein processing, FA synthesis, and energy metabolism (Supplementary Figure 4). This indicated that the common response mechanisms of P. haitanensis to hypersaline and hyposaline treatments are to enhance the energy metabolism pathways, promote the synthesis of FAs, and enhance the refolding and clearance of misfolded proteins. However, P. haitanensis mainly enhanced the TCA cycle to meet the energy needs of thalli under hypersaline treatment (Wen et al., 2020), while the thalli responded to hyposaline stress by enhancing the EMP pathway (Figures 3E–H).
Although both hypersaline and hyposaline treatments increased the content of FAs in P. haitanensis (Figure 5A), the thalli maintained the composition and proportion of SFAs and UFAs under hyposaline stress, while the content of UFAs significantly increased under hypersaline stress. This suggested that thalli maintained the integrity of the membrane system under hyposaline treatment, while they mainly enhanced the mobility of the membrane system under hypersaline treatment (Figures 5B–D). Organisms can increase the fluidity of the plasma membrane by increasing the degree of unsaturation of individual FAs or by increasing the proportion of UFAs in response to changes in the external environment. For example, increased unsaturation index and membrane fluidity of Saccharomyces cerevisiae lipids enhance their resistance to salinity and freezing stresses (Rodríguez-Vargas et al., 2007). In the FA desaturase FAD2 mutant of A. thaliana, the reduced levels of polyunsaturated FAs in the vacuole and plasma membrane increased its sensitivity to salt stress (Zhang et al., 2012). Here, in a difference from the response to hyposaline stress, the content of C20:5(n-3) in P. haitanensis increased significantly under hypersaline stress, and the content of its biosynthetic precursors C18:0, C18:1, C18:3(n-3), and C18:3(n-6) decreased significantly, which indicated that P. haitanensis was actively accumulating C20:5(n-3) to cope with hypersaline stress (Supplementary Figure 5). C20:5(n-3) is ubiquitous in most marine animals, microalgae, protozoa and seaweeds, but it can hardly be detected in higher terrestrial plants (Sanina et al., 2004). Like other polyunsaturated FAs (PUFA), C20:5(n-3) plays an important role in maintaining membrane fluidity (Yano et al., 1998; Valentine and Valentine, 2004). Furthermore, C20:5(n-3) has strong antioxidant capacity, which contributes to organisms’ ability to resist oxidative stress (Hidetoshi et al., 2008). For example, an Antarctic bacterium changed the fluidity of the plasma membrane by adjusting the content of C20:5(n-3) to cope with changes in external temperature (Nichols et al., 1997). In our study, C20:5(n-3) was the largest component of UFAs in P. haitanensis thalli, suggesting that this FA plays a crucial role in the normal growth, development, and hypersaline stress tolerance of the thalli.
The proteins enriched in Profile 3 that were down-regulated only under hypersaline stress were mainly related to photosynthesis, photosynthesis antenna proteins, and biosynthesis of secondary metabolites (Supplementary Figure 4B and Figure 4E), which indicates that hypersaline stress will have a great impact on photosynthesis and synthesis of secondary metabolites in P. haitanensis. Previous studies showed that the influx of Na+ into cells under hypersaline stress would significantly inhibit Fv/Fm of P. haitanensis (Wang et al., 2019). In contrast, the proteins enriched in Profile 4 that were up-regulated only under hypersaline stress were mainly related to amino acid biosynthesis, aminoacyl t-RNA biosynthesis, protein processing in endoplasmic reticulum, ribosome and the TCA cycle (Supplementary Figure 4A and Figure 4E). P. haitanensis thalli increased the synthesis of amino acids and proteins under hypersaline stress, which provided sufficient materials to enable the stress response. In addition, a large number of proteins related to molecular chaperones and ubiquitination were up-regulated, which would facilitate the correct folding of proteins in ERQC and the removal of misfolded proteins in ERAD in P. haitanensis under hypersaline stress.
In general, the functions of P. haitanensis responses to hyposaline stress are to: 1) ensure the dynamic balance between protein folding and clearance of misfolded proteins; 2) maintain cell membrane integrity and stability; 3) maintain the supply of energy via the EMP. Compared with the LSS 0 treatment, P. haitanensis under the LSS 5 treatment appears to maintain greater energy supply, more stable endoplasmic reticulum environment and more complete membrane systems. In contrast, under hypersaline stress, thalli can maintain their protein synthesis, enhance the fluidity and integrity of the plasma membrane, and enhance the operation of the TCA cycle to maintain the energy supply and enable other response mechanisms. The results of this study help to clarify the various response mechanisms of intertidal thalli to periodic salinity stress (Figure 6).
Figure 6 Model of P. haitanensis response mechanisms under hypersaline and hyposaline stress. The results of this study show that, in response to salt stress, P. haitanensis will: enhance the pentose phosphate pathway (PPP, purple arrows) to meet the demand for sugar metabolism, intermediates and energy, whether under hypersaline stress or hyposaline stress; enhance the endoplasmic reticulum quality control (ERQC, black) and endoplasmic reticulum-associated degradation (ERAD, blue) systems to maintain protein folding in the endoplasmic reticulum, and remove misfolded proteins to maintain the steady state of the endoplasmic reticulum; actively change the type and content of fatty acids (FAs) to maintain the stability or integrity of cell membranes, and so ensure that vital cellular activities can continue, even under salt stress. The EMP (green arrows) is the main energy supply pathway of P. haitanensis under hyposaline stress, while the TCA cycle (red) is the main functional pathway under hypersaline stress. Hyposaline stress inhibits the synthesis of amino acids and proteins. In contrast, hypersaline stress promotes the synthesis of amino acids and proteins.
The raw data generated in this study were deposited in the iProx database (accession number PXD018664).
JW and WW conducted the data analysis and wrote the first draft of the manuscript. KX, DJ and YX participated in the data collection and sample processing. CX and CC contributed to design and interpretation of results. All authors contributed to writing, revising, and approving the submitted version of the manuscript.
This work was supported by the National Natural Science Foundation of China (grant number: 42176117 and 41806185), the National Key R&D Program of China (grant numbers: 2018YFD0900100 and 2018YFD0901500), Fujian Province Science and Technology Major Project (2019NZ08003) and the China Agriculture Research System of MOF and MARA (grant number: CARS-50).
We thank Liwen Bianji, Edanz Editing China (www.liwenbianji.cn/ac) for editing the English text of a draft of this manuscript.
The authors declare that the research was conducted in the absence of any commercial or financial relationships that could be construed as a potential conflict of interest.
All claims expressed in this article are solely those of the authors and do not necessarily represent those of their affiliated organizations, or those of the publisher, the editors and the reviewers. Any product that may be evaluated in this article, or claim that may be made by its manufacturer, is not guaranteed or endorsed by the publisher.
The Supplementary Material for this article can be found online at: https://www.frontiersin.org/articles/10.3389/fmars.2022.928617/full#supplementary-material
Supplementary Table 1 | Proteomic identification results.
Supplementary Table 2 | KEGG function annotation of proteins enriched in various categories.
Supplementary Figure 1 | Verification of DEPs in P. haitanensis under LSS 0 and LSS 5 treatments, based on multiple reaction monitoring. Histogram: DIA results; line chart: MRM results. The relative changes are presented as log2 fold changes.
Supplementary Figure 2 | KEGG enrichment analysis of DEPs in P. haitanensis under hyposaline treatment. (A) The first 30 pathways with significant differences under LSS 0 treatment. (B) The first 30 pathways with significant differences under LSS 5 treatment.
Supplementary Figure 3 | Effects of hyposaline stress on P. haitanensis pathways related to protein metabolism, fatty acid metabolism, and carbon metabolism. The heat map was plotted based on log2 fold change (FC) values from the proteomics dataset. The inside, middle and outside tracks represent the LSS 0, LSS 5 and LSS 5/0 conditions, respectively. FC ≥ 1.5 and P< 0.05.
Supplementary Figure 4 | KEGG enrichment analysis of DEPs under hypersaline and hyposaline treatments. (A) KEGG enrichment analysis of DEPs in Profile 4. (B) KEGG enrichment analysis of DEPs in Profile 3. (C) KEGG enrichment analysis of DEPs in Profile 2. (D) KEGG enrichment analysis of DEPs in Profile 7.
Supplementary Figure 5 | Pattern diagram of unsaturated fatty acid synthesis pathway of P. haitanensis under hypersaline and hyposaline treatments. Data for content of selected fatty acids under different salinity treatments (different colors) are presented as the mean ± SE for at least three independent biological replicates. Different letters above the bars indicate significant differences among treatments at P < 0.05, as determined by one-way ANOVA followed by Tukey’s test.
Anbu D., Sivasankaramoorthy S. (2013). Ameliorative effect of CaCl2 on growth, membrane permeability and nutrient uptake in Oryza sativa grown at high NaCl salinity. Ilns Pl. 8, 14–22. doi: 10.18052/www.scipress.com/ILNS.8.14
Bassuany F. M., Hassanein R. A., Baraka D. M., Khalil R. R. (2014). Role of stigmasterol treatment in alleviating the adverse effects of salt stress in flax plant. J. Agric. Technology. 10, 1001–1020.
Blouin N. A., Brodie J., Grossman A. C., Xu P., Brawley S. H. (2011). Porphyra: a marine crop shaped by stress. Trends Plant Science. 16, 29–37. doi: 10.1016/j.tplants.2010.10.004
Bricker S. B., Longstaff B., Dennison W., Jones A., Boicourt K., Wicks C., et al. (2008). Effects of nutrient enrichment in the nation's estuaries: a decade of change. Harmful. Algae. 8, 21–32. doi: 10.1016/j.hal.2008.08.028
Chen S. X., Harmon A. C. (2006). Advances in plant proteomics. Proteomics. 6, 5504–5516. doi: 10.1002/pmic.200600143
Chen C. S., Ji D. H., Xie C. T., Xu Y., Liang Y., Zheng Y. J., et al. (2008). Preliminary study on selecting the high temperature resistance strains and economic traits of Porphyra haitanensis. Acta Oceanol. Sin. 30, 6. doi: 10.1007/s11676-008-0012-9
Chitlaru E., Pick U. (1991). Regulation of glycerol synthesis in response to osmotic changes in Dunaliella. Plant Physiol. 96, 50–60. doi: 10.1104/pp.96.1.50
Ernst J., Bar-Joseph Z. (2006). STEM: a tool for the analysis of short time series gene expression data. BMC Bioinf. 7, 191. doi: 10.1186/1471-2105-7-191
Fishery Department of China (2021). China Fishery statistical yearbook (in Chinese) (Bei Jing: Chinese Agriculture Press).
Fu J., Ivana M., Vara P. (2012). Roles of protein synthesis elongation factor EF-tu in heat tolerance in plants. J. Botany. 835836, 1–8. doi: 10.1155/2012/835836
Fu J., Momčilović I., Clemente T. E., Nersesian N., Trick H. N., Ristic Z. (2008). Heterologous expression of a plastid EF-tu reduces protein thermal aggregation and enhances CO2 fixation in wheat (Triticum aestivum) following heat stress. Plant Mol. Biol. 68, 277–288. doi: 10.1007/s11103-008-9369-6
Helenius A., Aebi M. (2004). Roles of n-linked glycans in the endoplasmic reticulum. Annu. Rev. Biochem. 73, 1019–1049. doi: 10.1146/annurev.biochem.73.011303.073752
He P. M., Zhang Z. Y., Zhang X. C., Ma J. H. (2018). Seaweed cultivation (in Chinese) (Bei Jing: Science Press).
Hidetoshi O., Yoshitake O., Takanori N. (2008). Significance of antioxidative functions of eicosapentaenoic and docosahexaenoic acids in marine microorganisms. Appl. Environ. Microbiol. 74, 570–574. doi: 10.1128/AEM.02256-07
Huang B. R. (2006). “Cellular membranes in stress sensing and regulation of plant adaptation to abiotic stresses,” in Plant-environment interactions, 3rd edn. CRC Press, (Taylor and Francis, Boca Raton), 1–26.
Kennedy J. J., Abbatiello S. E., Kim K., Yan P., Whiteaker J. R., Lin C. W., et al. (2014). Demonstrating the feasibility of large-scale development of standardized assays to quantify human proteins. Nat. Methods 11, 149–155. doi: 10.1038/nmeth.2763
Law K. P., Lim Y. P. (2013). Recent advances in mass spectrometry: data independent analysis and hyper reaction monitoring. Expert Rev. proteomics. 10, 551–566. doi: 10.1586/14789450.2013.858022
Li Y. H., Beisson F., Pollard M., Ohlrogge J. (2006). Oil content of arabidopsis seeds: The influence of seed anatomy, light and plant-to-plant variation. PHYTOCHEMISTRY 67 (9), 904–915. doi: 10.1016/j.phytochem.2006.02.015
Liu J. X., Howell S. H. (2010). Endoplasmic reticulum protein quality control and its relationship to environmental stress responses in plants. Plant Cell. 22, 2930–2942. doi: 10.1105/tpc.110.078154
Li H., Xie X. J., Zheng Z. B., Sun F. F., Wu S. C., Li M. Y., et al. (2014). Positive correlation between PSI response and oxidative pentose phosphate pathway activity during salt stress in an intertidal macroalga. Plant Cell Physiol., 55, 1395–1403. doi: 10.1093/pcp/pcu063
López–Cristoffanini C., Zapata J., Gaillard F., Potin P., Correa J. A., Contrerasporcia L. (2015). Identification of proteins involved in desiccation tolerance in the red seaweed Pyropia orbicularis (Rhodophyta, bangiales). Proteomics. 15, 3954–3968. doi: 10.1002/pmic.201400625
Lu X. P., Huan L., Gao S., He L. W., Wang G. C. (2016). NADPH from the oxidative pentose phosphate pathway drives the operation of cyclic electron flow around photosystem I in high-intertidal macroalgae under severe salt stress. Physiologia. Plantarum. 156, 397–406. doi: 10.1111/ppl.12383
Mansour M. M. F. (2012). Plasma membrane permeability as an indicator of salt tolerance in plants. Biol. Plantarum. 57, 1–10. doi: 10.1007/s10535-012-0144-9
Nichols D. S., Brown J. L., Nichols P. D., Mcmeekin T. A. (1997). Production of eicosapentaenoic and arachidonic acids by an antarctic bacterium: response to growth temperature. FEMS Microbiology Letters. 152, 349-354. doi: 10.1016/s0378-1097(97)00224-3
Pedersen A., Kraemer G., Yarish C. (2004). The effects of temperature and nutrient concentrations on nitrate and phosphate uptake in different species of Porphyra from long island sound (USA). J. Exp. Mar. Biol. Ecology. 312, 235–252. doi: 10.1016/j.jembe.2004.05.021
Roberts L. D., Souza A. L., Gerszten R. E., Clish C. B. (2012). Targeted metabolomics. Curr. Protoc. Mol. Biol. 98, 30.32. doi: 10.1002/0471142727.mb3002s98
Rodríguez-Vargas S., Sánchez-García A., Martínez-Rivas J. M., Prieto J. A., Randez-Gil F. (2007). Fluidization of membrane lipids enhances the tolerance of Saccharomyces cerevisiae to freezing and salt stress. Appl. Environ. Microbiol. 73, 110–116. doi: 10.1128/AEM.01360-06
Sanina N. M., Goncharova S. N., Kostetsky E. Y. (2004). Fatty acid composition of individual polar lipid classes from marine macrophytes. Phytochemistry. 65, 721–730. doi: 10.1016/j.phytochem.2004.01.013
Sarwat M., Naqvi A. R. (2013). Heterologous expression of rice calnexin (OsCNX) confers drought tolerance in Nicotiana tabacum. Mol. Biol. Rep. 40, 5451–5464. doi: 10.1007/s11033-013-2643-y
Shi J. Z., Chen Y. T., Xu Y., Ji D. H., Chen C. S., Xie C. T. (2017). Differential proteomic analysis by iTRAQ reveals the mechanism of Pyropia haitanensis responding to high temperature stress. Sci. Rep. 7, 44734. doi: 10.1038/srep44734
Shi J. Z., Wang W. L., Lin Y. H., Xu K., Xu Y., Ji D. H., et al. (2019). Insight into transketolase of Pyropia haitanensis under desiccation stress based on integrative analysis of omics and transformation. BMC Plant Biol. 19, 1-16. doi: 10.1186/s12870-019-2076-4
Singh R., Kaushik S., Wang Y. J., Xiang Y. Q., Novak I., Komatsu M., et al. (2009). Autophagy regulates lipid metabolism. Nature. 458, 1131–1135. doi: 10.1038/nature07976
Sofo A., Dichio B., Xiloyannis C., Masia A. (2004). Lipoxygenase activity and proline accumulation in leaves and roots of olive trees in response to drought stress. Physiologia. plantarum. 121, 58–65. doi: 10.1111/j.0031-9317.2004.00294.x
Suzuki M., Hashioka A., Mimura T., Ashihara H. (2005). Salt stress and glycolytic regulation in suspension-cultured cells of the mangrove tree, Bruguiera sexangula. Physiologia. Plantarum. 123, 246–253. doi: 10.1111/j.1399-3054.2005.00456.x
Valentine R. C., Valentine D. L. (2004). Omega-3 fatty acids in cellular membranes: a unified concept - ScienceDirect. Prog. Lipid Res. 43, 383–402. doi: 10.1016/j.plipres.2004.05.004
Walter P., Blobel G. (1980). Purification of a membrane-associated protein complex required for protein translocation across the endoplasmic reticulum. Proc. Natl. Acad. Sci. United. States America. 77, 7112–7116. doi: 10.1073/pnas.77.12.7112
Wang W. L., Chen T. X., Xu Y., Xu K., Ji D. H., Chen C. S., et al. (2020). Investigating the mechanisms underlying the hyposaline tolerance of intertidal seaweed, Pyropia haitanensis. Algal. Res. 47, 101886. doi: 10.1016/j.algal.2020.101886
Wang W. L., Teng F., Lin Y. H., Ji D. H., Xu Y., Chen C. S., et al. (2018). Transcriptomic study to understand thermal adaptation in a high temperature-tolerant strain of Pyropia haitanensis. PloS One 13, e0195842. doi: 10.1371/journal.pone.0195842
Wang W. X., Vinocur B., Shoseyov O., Altman A. (2004). Role of plant heat-shock proteins and molecular chaperones in the abiotic stress response. Trends Plant Science. 9, 244–252. doi: 10.1016/j.tplants.2004.03.006
Wang W. L., Xu Y., Chen T. X., Xing L., Xu K., Xu Y., et al. (2019). Regulatory mechanisms underlying the maintenance of homeostasis in Pyropia haitanensis under hypersaline stress conditions. Sci. Total. Environment. 662, 168–179. doi: 10.1016/j.scitotenv.2019.01.214
Wen J., Wang W. L., Xu K., Ji D. H., Xu Y., Chen C. S., et al. (2020). Comparative analysis of proteins involved in energy metabolism and protein processing in Pyropia haitanensis at different salinity levels. Front. Mar. Sci 7, 415. doi: 10.3389/fmars.2020.00415
Xue J., Balamurugan S., Li D. W., Liu Y. H., Zeng H., Wang L., et al. (2017). Glucose-6-phosphate dehydrogenase as a target for highly efficient fatty acid biosynthesis in microalgae by enhancing NADPH supply. Metab. engineering. 41, 212–221. doi: 10.1016/j.ymben.2017.04.008
Yano Y., Nakayama A., Ishihara K., Saito H. (1998). Adaptive changes in membrane lipids of barophilic bacteria in response to changes in growth pressure. Appl. Environ. Microbiol. 64, 479–485. doi: 10.1089/oli.1.1998.8.67
Zhang J. T., Liu H., Sun J., Li B., Zhu Q., Chen S. L., et al. (2012). Arabidopsis fatty acid desaturase FAD2 is required for salt tolerance during seed germination and early seedling growth. PloS One 7, e30355. doi: 10.1371/journal.pone.0030355
Keywords: Pyropia haitanensis, hyposaline stress, intracellular homeostasis, proteomics, targeted metabolomics
Citation: Wen J, Xu K, Ji D, Xu Y, Chen C, Wang W and Xie C (2022) The mechanism of maintaining intracellular homeostasis in the red alga Pyropia haitanensis under hyposaline stress. Front. Mar. Sci. 9:928617. doi: 10.3389/fmars.2022.928617
Received: 26 April 2022; Accepted: 11 July 2022;
Published: 03 August 2022.
Edited by:
Xiaofeng Lin, Xiamen University, ChinaReviewed by:
Dongmei Wang, Ocean University of China, ChinaCopyright © 2022 Wen, Xu, Ji, Xu, Chen, Wang and Xie. This is an open-access article distributed under the terms of the Creative Commons Attribution License (CC BY). The use, distribution or reproduction in other forums is permitted, provided the original author(s) and the copyright owner(s) are credited and that the original publication in this journal is cited, in accordance with accepted academic practice. No use, distribution or reproduction is permitted which does not comply with these terms.
*Correspondence: Wenlei Wang, d2x3YW5nQGptdS5lZHUuY24=; Chaotian Xie, Y3R4aWVAam11LmVkdS5jbg==
Disclaimer: All claims expressed in this article are solely those of the authors and do not necessarily represent those of their affiliated organizations, or those of the publisher, the editors and the reviewers. Any product that may be evaluated in this article or claim that may be made by its manufacturer is not guaranteed or endorsed by the publisher.
Research integrity at Frontiers
Learn more about the work of our research integrity team to safeguard the quality of each article we publish.