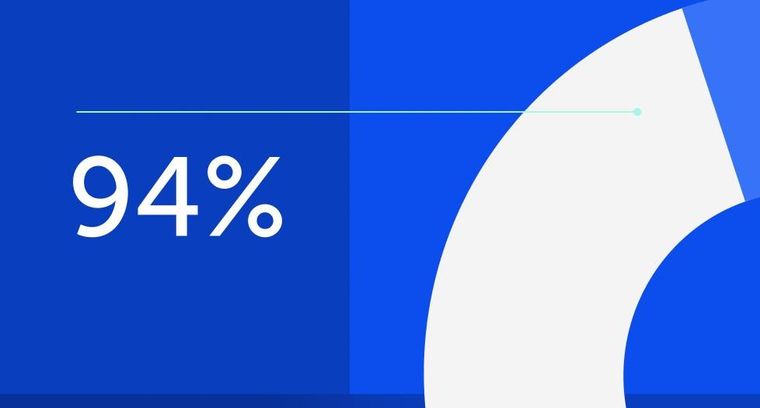
94% of researchers rate our articles as excellent or good
Learn more about the work of our research integrity team to safeguard the quality of each article we publish.
Find out more
ORIGINAL RESEARCH article
Front. Mar. Sci., 09 August 2022
Sec. Marine Pollution
Volume 9 - 2022 | https://doi.org/10.3389/fmars.2022.928576
We describe the initial bulk and compound specific composition of the liquid oil spilled during the Deepwater Horizon (DwH) disaster. The emphasis is on the target hydrocarbon compounds typically found in highest concentrations and on those of concern from a toxicological perspective (i.e., the target normal alkanes and isoprenoids, and PAHs on U.S. Environmental Protection Agency’s (EPA) priority list with their alkyl homolog compounds), and/or those relevant for forensic fingerprinting of spill residues (i.e., sulfur containing PAHs and biomarker compounds). Weathering changed the oil’s composition in various environmental compartments. These compositional changes and potential environmental impacts of the remaining weathered residues are presented in this paper. Biodegradation occurred in both surface and subsurface environments while photooxidation primarily modified and removed hydrocarbons in floating oil slicks. The volatile, soluble and highly labile C1 to C10 hydrocarbons were rapidly degraded in the water column and/or emitted to the atmosphere (evaporation). The semi-volatile hydrocarbons (labile C10 to C25) that remained in the water column and floating oil on the water’s surface were lost from oil residues during weathering. The heavy nonvolatile and insoluble hydrocarbons (recalcitrant C25+) were least affected by initial weathering processes in 2010. The composition of the residual oil fraction in surface floating oil was further altered by the addition of oil soluble oxy hydrocarbons produced from photooxidation. During 2011 and 2012 the resulting highly insoluble recalcitrant C25+ oily residues remained on the shorelines, bottom sediments, or bound to suspended particulates in the water column, with detectable residues mostly returning to near pre-spill levels by 2015 to 2020. Some recalcitrant oil residues can still be found at various locations, including some coastal environments (e.g., marshes), or deep-water sediments, at very low levels, ten years after the spill.
The 2010 Deepwater Horizon (DwH) oil spill in the northern Gulf of Mexico was the largest, longest lasting (84 days), and deepest oil spill accident in US waters. Liquid oil and natural gas were emitted from April 22 to July15, 2010, initially from kinked portions of the riser pipe, and finally after cutting the riser to permit capping efforts, from the Macondo well’s blowout preventer (Reddy et al., 2011; McNutt et al., 2012). As the liquid oil and gas rose, most of the natural gas and approximately 11% of the liquid oil were advected to the southwest of the drilling rig at between 1100 and 1300 meters of the water column. The liquid oil was advected as dispersed tiny droplets. Larger droplets of liquid oil rose via buoyancy to the surface taking ~3 to >20 hours, depending upon droplet size. During ascension, smaller oil droplets were influenced by dissolution and limited biodegradation. Upon surfacing, portions of the liquid oil (30-40% by weight) evaporated rapidly. Photooxidation of floating oil produced new oxy hydrocarbons that changed the properties and environmental partitioning compared to the parent hydrocarbon content of the spilled oil. Some of these oxy hydrocarbons enabled rapid emulsion formation with the weathered floating oil potentially affecting the effectiveness of response measures such as dispersant application. A portion of the surface weathered oil was dispersed back into the water column by action of wind and waves, a process enhanced by the surface spraying of chemical dispersants. In-situ-burning (ISB) of herded oil at the surface also caused portions of burned oily residues to partition back into the water column. Dispersants were also applied into the jetting oil at the kinked riser and well head, mostly between the middle of May until well capping on July 15, 2010, impacting the partitioning of oil compounds between different water phases (sea surface, deep plume, surface layer) (Hemmer et al., 2011; Chakraborty et al., 2012; Paris et al., 2012; Kleindienst et al., 2015; Deleo et al., 2016; Olson et al., 2017; Crowley et al., 2018; Doyle et al., 2018; Driskell and Payne, 2018). A variety of physical, chemical, and biological mechanisms rapidly transformed, removed, and redispersed spilled oil and dissolved gases. By the end of the summer 2010, the initial composition of hydrocarbons in the emitted source oil was substantially changed via weathering processes such as evaporation, dissolution, photochemical oxidations, and microbial degradation.
Understanding the environmental carbon cycle that occurs on geological timescales is a first step towards understanding the chemistry of oil spills. Since the rise of autotrophy, organic matter has been formed from chemically stable carbon dioxide (CO2) via various biological processes, dominated by photosynthesis. Through photosynthesis, energy from sunlight is used to break the carbon-oxygen bonds of CO2 and the hydrogen-oxygen bonds of H2O, and this process forms new molecules containing carbon, hydrogen, and oxygen with a general elemental ratio of {CH2O}. In essence, this process stores the solar energy in the carbon and hydrogen molecular bonds and represents a reduction in the oxidation state of carbon from +4 in CO2 to 0. Photosynthesis is an endothermic reduction reaction requiring the input of solar energy for producing biomass. On the other hand, when the energy in biomass is used for metabolism (e.g., respiration), the reverse oxidation reactions recycle biomass back to CO2, i.e., metabolism involves exothermic reactions releasing energy needed to sustain life.
When some of the organic matter (e.g., from large forests or algae sinking to the seafloor) is buried under reducing (anoxic) conditions, this prevents respiration of the buried organic matter back to CO2. On geological timescales, as the organic matter accumulates in the subsurface, the heat and pressure in the earth’s crust allows further transformation of buried and preserved biomass to highly reduced chemical species, such as those that compose peat, lignite, coal, natural gas, and crude oil (mostly C and H compounds with smaller amounts of compounds also containing nitrogen, sulfur, and oxygen heteroatoms - NSO). This fossilized organic matter, which consists largely of hydrocarbons formed hundreds of millions of years ago, has oxidation states of -1 (aromatics) or -2 (saturates). The radioactive carbon isotope (14C) content of this fossilized organic matter has been depleted. When extracted from the earth, these hydrocarbons become the major energy source of current civilizations, as combustion of fossil fuels releases the stored chemical energy and recycles hydrocarbons by oxidizing them back to CO2 (Peters et al., 2004).
For hydrocarbon oxidation reactions to occur, four conditions must be met. First, the material (e.g., fuel) to be oxidized must be in a reduced state. Second, oxygen or another electron acceptor must be present. Third, the oxidant and fuel must be suitably mixed. And fourth, a mechanism to overcome the activation energy must be provided to the mixture of oxidant and fuel. Activation energy for oxidation of reduced forms of carbon, such as hydrocarbons, may be provided in the form of a spark to get the combustion reaction going or as a catalyst (e.g., specialized microbial enzymes) that lowers the activation energy and thus allow metabolism reactions to proceed at near ambient temperatures. Figure 1 outlines the process of photochemically producing biomass from CO2 and then the diagenetic and catagenetic formation of crude oil from some of the preserved biomass. Figure 1 also outlines the relative elemental compositions of biomass and crude oil fossil fuels and includes their specific combustion energies.
Figure 1 An overview of molecular transitions that occur over geological timescales during the Slow Environmental Carbon Cycle. Carbon dioxide in the atmosphere and seawater is converted to living organisms by photosynthesis. The C, H and O ratio content of this biomass is ~CH2O. When a small portion of the biomass is preserved and buried in deep reservoirs over many millions of years, this material is converted to a liquid hydrocarbon fluid with average molecular ratio of CH1.75 (mostly saturate compounds with a smaller proportion of aromatic compounds) based on C and H percent compositions. The heats of combustion (combustion energy) for typical biomass and crude oils show how much energy is stored in the C and H bonds of these two fuels.
Carbon and hydrogen containing compounds do not necessarily react to directly produce CO2, they can go through a sequence of reactions that each lessens the stored energy content of the molecular products. Each addition of an oxygen atom to a carbon-containing molecule, for example, lowers the stored energy content in that molecule. This process continues until all carbon atoms in the molecule have been converted to CO2, the terminal product of carbon oxidations. Therefore, typically, environmental degradation of hydrocarbons via microbial and/or photooxidation proceeds on varying time scales through a series of intermediate molecules that are dependent on highly variable environmental circumstances. Coupling of the two degradation processes, wherein photooxidation produces intermediate species that are more amenable to biodegradation, has also been postulated (Harriman et al., 2017).
CO2 is not the only possible end product of carbon degradation in the environment. In oxygen depleted settings, reduced forms of carbon are metabolized by specific types of microbes, through pathways using nitrate, iron, manganese, or sulfate as terminal electron acceptors, or by fermentation. For example, methanogenic hydrocarbon degradation occurring over geological timescales is responsible for the formation of heavy oil and bitumen deposits, along with methane gas. Microbial degradation coupled to sulfate as the electron acceptor is a frequent anaerobic pathway in anoxic sediments and generates hydrogen sulfide (H2S) gas. During these processes, hydrocarbons are transformed to a complex mixture of metabolites, including high molecular weight NSO-bearing products (Gieg et al., 2010; Head et al., 2014; Oldenburg et al., 2017; Kostka et al., 2020). In general, anaerobic microbial processes have a higher activation energy and lower enthalpy than O2 oxidation reactions, resulting in much slower degradation rates than the pathways involving oxygen as the electron acceptor (Head et al., 2014).
Crude oil is the common name for a very complex mixture of many thousands of distinct chemical species, including predominantly hydrocarbon type compounds (i.e., containing only carbon and hydrogen with carbon negative oxidation states), and some amounts of heteroatom-bearing hydrocarbon compounds containing nitrogen, oxygen, sulfur, or metals, collectively termed non-hydrocarbons (Overton et al., 2016). Crude oil is found as complex fluids that are extracted from reservoirs below the earth’s surface (Overton et al., 2020). Most crude oils, regardless of their geographic source, are made up of the same types of molecular species, but the relative quantities of specific molecules vary between crude oils from different locations as their compositions are dependent upon the reservoir’s location, depth, initial biomass composition, and age. Consequently, the quantities of specific molecular fractions in crude oils will vary with the source reservoir so that each reservoir’s crude oil is unique in its exact quantitative chemical composition (National Research Council, 1985).
The crude oil’s basic compositions may be described as existing in three environmental “fate fractions”, based on their respective environmental persistence when spilled. The first fate fraction contains those hydrocarbon compounds that are readily lost during weathering in a matter of minutes to hours (the highly labile C1 to C10 fraction). The second fate fraction contains those hydrocarbon components that can be lost to weathering during a time frame of days to weeks or more (the labile C10 to C25 fraction). The third fate fraction contains those compounds that are only slowly lost during weathering with time frames of months to years or more (the recalcitrant C25+ fraction). Note that these fate fractions are operational definitions made to facilitate and aid in understanding environmental fate assessments. Notwithstanding this, the sheer chemical complexity and variability of crude oils’ composition challenges any absolute and clear-cut separation into chemical fractions. Other approaches, such as the ones based on varying solubility of specific molecular fractions in organic solvents and solid/liquid chromatographic separations (the SARA fractionation) or their boiling points, are still widely used both in environmental and petroleum refining contexts (Overton et al., 2016; Radovic, 2021).
Figure 2 outlines the relative compositions of the three fate fractions in the Macondo oil that entered the environment (i.e., the source petroleum fluids from the Macondo well blowout). This source Macondo oil contained compounds that are a gas at ambient temperature and atmospheric pressure, the C1 to C4+ natural gas hydrocarbons, as well as the liquid oil that was collected from the broken riser pipe. The riser source liquid oil reflects liquid oil from the Macondo reservoir. The fate fractions in Figure 2 are based on their molar and mass compositions, as calculated from the carbon number simulated distillation curves of the liquid riser oil from Macondo well, provided by Aeppli et al. (2018). The source oil is commonly known as a “live oil” because it is mixed with natural gas (the C1 to C4+ hydrocarbon compounds). The riser liquid oil, collected in open containers that allowed evaporation of the natural gas, is commonly known as a “dead oil” because most of its very volatile C1 to C4+ components (the natural gas compounds) have evaporated.
Figure 2 Bulk composition of petroleum compounds released into the deep-water environments by the blowout of the Macondo well in 2010, showing the relative proportions of Natural Gas (C1 to C4+) and Liquid Oil (C5 to C44 plus resins and asphaltenes) on a molar and weight basis. Further, in (B), the source petroleum and surface liquid oil’s composition is partitioned between gaseous and volatile components (C1 to C10), readily degradable labile hydrocarbons (C10 to C25), and insoluble recalcitrant mostly solid material containing hydrocarbons larger than C25 (C25+) as calculated with compositional data provided in Ryerson et al., 2011; Ryerson et al., 2012 and Stout et al., 2016.
Figure 3 shows the mass and molar distribution of the riser liquid “dead oil” as calculated from the simulated distillation data via the oil’s carbon number fractions (Spier et al., 2013; Aeppli et al., 2018). Over 90% of the molecules that made up the Macondo liquid oil (the dead oil) are contained in the initial two fates fractions (highly labile C1 to C10 and labile C10 to C25 fractions). By mass these two fractions contained ~68% of the oil. Collectively, most of the hydrocarbon molecules in the first two fates fractions of the spilled oil were lost within weeks to months after the spill (i.e., the summer of 2010) due to environmental weathering processes. These two fates fractions also contain the water-soluble alkanes (<C8 or C9) and aromatic compounds including benzene, alkyl benzenes, and the two to four ringed polycyclic aromatic hydrocarbons (PAHs) and their abundant alkyl homolog analogues. Of these, the four ringed PAHs and their alkyl homologs were the least affected by initial weathering during the summer of 2010.
Figure 3 Plots of the results from the simulated distillation of the Macondo oil separated by carbon number and expressed as the weight percent (blue bars, (A) and molar percent (red bars, (B). The molar fractions were calculated assuming that the molecular weight of each carbon number fraction was estimated from the carbon number multiplied by 13.75 (assuming average CH1.75 molar ratio based on C and H percent composition in the Macondo crude). Ninety percent of molecules in the initially spilled liquid Macondo oil were contained in the fractions with carbon numbers (86.6% C and 12.6% H) less than C25, while this fraction accounted for 68% of the liquid oil’s initial mass. (Modified from Aeppli et al., 2018 with permission).
Since crude oil is such a complex mixture of thousands of compounds, scientists have traditionally used a select, relatively small number of representative hydrocarbon compounds as analytical targets, including a suite of alkanes, PAHs, and forensic biomarkers, when studying crude oil spills and oil weathering. These target compounds have been selected based on their high individual concentrations in typical oil, as well as their known capacity to cause harm during environmental exposures (i.e., acute and/or chronic toxicities). The target alkane compounds, analyzed by GC and GC-MS type analytical methods, include normal alkanes up to n-C35 or n-C40, as well as the isoprenoids (branched alkanes) pristane (C19H40) and phytane (C20H22). Target aromatics include two to six ringed PAH compounds and their respective C1 to C3 or C4 alkyl homologs, and includes the sulfur containing benzo, dibenzo and naphthyl-benzo thiophenes and their respective alkyl homologs. Forensic biomarkers are the hopane and sterane type molecules in oils that were present in the source biomass material and whose chemical structures is maintained in the reservoir’s crude oil composition, thus enabling source-spill correlations, and the identification of spilled oil and its residues in the environment. See Table 1 for target compound identifications and their GC-MS quantitative ions.
Table 1 Quantitation ions for Parent and Alkyl Homolog PAHs, Alkanes, and Biomarker Compounds in Crude Oil.
Figure 4 shows the quantitative composition of the target alkanes and aromatic hydrocarbons found in the liquid dead oil collected from the Macondo well’s riser piping. While the target compounds are representative of the hydrocarbon mixture that makes up the Macondo and other common crude oils, quantitatively these selected “target” compounds only accounted for ~13% of the total mass of the spilled liquid Macondo oil. Thus, they represent the major compound classes found in conventional crude oils but not the entire mass of the spilled crude oil. Note that some other crude oil types, such as heavy, unconventional crudes, and oil sands derived bitumen will contain a substantial amount of non-hydrocarbon species in their recalcitrant C25+ fractions, and these compounds are not amenable to conventional GC-based analysis due to their extremely low volatility. Complementary analytical tools are needed to better assess the complete high molecular weight molecular compositions of these heavy crude oils (Radovic, 2021).
Figure 4 The composition of the liquid source oil spilled from the Macondo well blowout obtained from gas chromatographic analysis using Flame Ionization Detector (GC-FID, from Stout et al., 2016) and Mass Spectrometer operating in the Selected Ion Monitoring mode (GCMS-SIM). The GC-FID chromatogram shows the full range of hydrocarbon compounds in this liquid oil, from ~n-C4 to n-C36+ including benzene and the C1 and C2 alkyl benzenes. Most hydrocarbons in oil samples are analyzed by GCMS analytical techniques that can quantitate selected specific target analytes that include: a) normal alkanes from n-C10 to n-C35+ including isoprenoid compounds pristine and phytane; b) Polycyclic Aromatic Hydrocarbons (PAH) and their C1 to C3 or C4 alkyl homologs as well as sulfur containing PAH compounds Dibenzothiophene and Naphthylbenzothiophene and their alkyl homologs. Additionally, the compositional isomer profiles of the C1 to C3 alkyl chrysenes are shown for the spilled Macondo oil. The target compounds account for ~13% of the weight of the liquid source oil, and saturate compounds represent ~94% of this weight while the target aromatics account for 6%.
Of the target compounds identified in Figure 4, the target alkanes were by far the most abundant, and accounted for 94% of all target compounds in unweathered Macondo well oil. These alkanes represent about 12% of the weight of the initial liquid oil. The two to six ringed PAH compounds and their alkyl homologs plus the dibenzo and naphthyl benzo thiophenes and their alkyl homologs, identified as target aromatic compounds in Table 1, account for ~6% of this target compound composition. This means that ~2% of the weight in the fresh un-weathered Macondo oil was from the target aromatic compounds. In essence, scientists use analytical methods that quantitate ~13% of fresh liquid oil’s specific hydrocarbons to follow how the whole composition of spilled oil’s hydrocarbons changes during environmental weathering.
Target aromatics are less abundant, but important because their unique structure and bonding arrangement increase their water solubility as well as their ability to react with oxygen via certain enzyme- and light-mediated oxidation reactions. This reactivity of aromatic compounds makes them more bioavailable to many types of marine and terrestrial organisms including shrimp, crabs, finfish, marine mammals, and humans (Oost et al., 2003; Allan et al., 2012). PAHs are moderately persistent in the environment and can be bioaccumulated in the tissues of animals (Douben, 2003). However, metabolism of PAHs by oxidative-enzyme systems to water soluble oxidized compounds and depuration products limits their biomagnification through trophic chains (Meadors et al., 1995). This metabolic oxidation can also lead to a variety of toxic consequences for the respective organisms (carcinogenicity, mutagenicity). Thus, the aromatic compounds in the target compound list are considered the most dangerous compounds in crude oils to living organisms (Neff and Anderson, 1981; Malins and Hodgins, 1981; French-McCay, 2002; National Research Council, 2003; Douben, 2003; Abdel-Shafy and Mansour, 2016; Hodson, 2017; Marzooghi and Di Toro, 2017; Honda and Suzuki, 2020).
Approximately 90% of the weight of these target alkane and aromatic analytes were found in the composition of the “highly labile C1 to C10” and “labile C10 to C25” fractions as quantified by gas chromatographic methods and compositionally outlined in Figure 2. However, these two fates fractions account for 68% of the weight of the whole oil as measured by simulated distillation methods. Thus, these widely used target compound quantitative analytical method (GC-MS in selected ion mode) can underestimate the mass of the recalcitrant C25+ fraction in whole crude and weathered oil analyses. In addition to the target alkanes and multi ring aromatic compounds, the recalcitrant C25+ fraction contains many cyclic and branched high molecular weight alkanes as well as the high aromatic content resins and asphaltenes, with high proportion of NSO species, not detectable via GC based analytical methods. The recalcitrant C25+ fraction generally contains compounds that are highly water insoluble and are only slowly degraded by microbial oxidations. While the target compound analytical method reflects the fresh oil’s composition as well as the concentration of known toxic compounds in the oil, it does not satisfactorily reflect the quantity of the recalcitrant C25+ compounds, which compose most compounds in residues of heavily weathered oils (i.e., the solid residues left behind after initial environmental weathering).
The chemical composition of the recalcitrant C25+ fraction has been the subject of much analytical research using ultra high-resolution mass spectrometry (FT-ICR-MS) and thin layer chromatography with flame ionization detection (TLC-FID) (Aeppli et al., 2012; Ruddy et al., 2014; White et al., 2016; Aeppli et al., 2018). Most of this fraction initially contains multi-ringed aromatic and NSO aromatic compounds, resins, and asphaltene molecular structures that are arranged into very large molecules with molecular weights of up to 1000 Daltons (McKenna et al., 2013). Crude oils, especially light crude oils, generally contains relatively small quantities of these very large molecules that can also contain trace heavy metals such as nickel and vanadium chelated in a porphyrin type molecular structure. These very large molecules in crude oils are not generally useful as a source of fuel and are particularly resistant to microbial degradation. Refinery residues containing these types of large insoluble compounds are commonly used as roofing tar, road asphalt or in bunker fuels for ships.
It is important to point out that photochemical weathering of floating crude oils will produce large oil-soluble oxy hydrocarbons that are operationally described as asphaltenes, i.e., they are insoluble in light alkane solvents but soluble in more polar organic solvents like toluene. These oxidized hydrocarbons are not chemically identical to native crude oil asphaltenes, as they have a high oxygen content (Ruddy et al., 2014). However, these photochemically produced oxy hydrocarbons became a major component of the recalcitrant C25+ fraction in solid residues lingering from spilled oil weathering. Further, these oxy hydrocarbons are not detectable using traditional GC-MS analytical methods. However, they are detectable using complementary methods, most notably, ultrahigh-resolution mass spectrometry, in crude oil and crude oil residues (Aeppli et al., 2012; Ruddy et al., 2014; Wise, et al., 2022).
The molecular properties (size, weight, and structures) of the hydrocarbon and non-hydrocarbon compounds that make up crude oils determines the oil’s individual chemical and physical properties. These individual properties of the specific crude oil components are comingled with the properties of all the other thousands of compounds that make up liquid petroleum to establish the continuum of chemical and physical properties that are measurable in each crude oil. These collective properties determine the oil’s initial viscosity, water solubility, volatility, pour point, toxicity, and bioavailability, as well as the oil’s ability to be degraded by sunlight and microorganisms.
Crude oils with a large proportion of relatively small, low molecular weight compounds in the highly labile C1 to C10 and labile C10 to C25 fractions, i.e., light oils like South Louisiana Crudes and the Macondo well oil, have low viscosities, relatively high-water solubilities, high vapor pressures, low pour points, and are more readily biodegradable and photooxidizable. Fresh, light crude oils thus not only have impacts on the water’s surface, but some components readily dissolve into the water column, or evaporate into the air above the oil slick. After oxidative weathering by sunlight, light crude oil can readily form water-in-oil emulsions, and these emulsions continue weathering but at a reduced rate, thus prolonging their residence time and potential for negative effects in the environment. The oil’s aromatics compounds can be toxic in themselves or after oxidation.
Crude oils that are composed of a higher proportion of the high molecular weight compounds (high portions of the recalcitrant C25+ fraction), i.e., heavy oils and bitumen, are comparably more viscous and less volatile, have high pour points, and extremely low water solubilities. Higher alkanes and the larger five to six ringed PAHs, as well as the residue’s resin and asphaltene components, are less bioavailable and only degraded slowly even in the presence of nutrients and oxygen. This is because of the extreme water insolubility of these larger hydrocarbons, thus limiting their mixing ability with the oxidants, and accessibility to light and microbes (Stout and Payne, 2016a; Stout and German, 2018; Passow and Stout, 2020; Karthikeyan et al., 2020). Extremely low solubility components of the recalcitrant C25+ fraction can however be slowly degraded in anaerobic environments (Kostka et al., 2020).
In addition to forming a portion of the surface slick of weathered liquid oil, some of the weathered oil residues can be entrained or entrapped in the water column and can even sink when associated with mineral particles and/or marine oil snow (Passow et al., 2012, 2017; Burd et al., 2020; White et al., 2020). Even though most of the toxic aromatic compounds in viscous heavy crude oils and weathered oil residues are individually more toxic than their smaller aromatic counterparts in the labile C10 to C25 fractions, the larger PAHs are much less water soluble and are contained as part of the solid insoluble residues that tend to sorb to particulate matter. This phase partitioning behavior is the primary reason that most light crude oils are considered more environmentally dangerous than heavy crudes/bitumen. Some exceptions to this assumption occur when insoluble weathered oil residues get ingested as part of the sinking marine oil snow (Van Eenennaam et al., 2018; Passow and Stout 2020).
The environmental fates of the different hydrocarbon compounds that make up crude oils are determined by a complicated series of transport and transformation processes that are collectively known as weathering (Payne et al., 1984; Payne and McNabb, 1984; Mackay and McAuliffe, 1988; Tarr et al., 2016; Passow and Overton, 2021; Farrington et al., 2021; French-McCay et al., 2021). The term fate is typically used to discuss both the spatial distribution and transport of spilled material in the environment as well as the compositional changes of crude oil on the molecular level as the oil is transformed. Removal and transformation processes occur in hours to days depending upon the spilled material and conditions during the early part of the release (Radovic et al., 2021; Farrington 2014; Tarr et al., 2016; Farrington et al., 2021). Evaporation transfers large amounts of light volatile hydrocarbons to the atmosphere, and small amounts of fresh whole oil can be atomized thus producing aerosol particles in the atmosphere (Li et al., 2017).
The initial chemical composition of spilled crude oil will have important implications for its near- and far-field transport as well as on its weathering. Understanding, the weathering processes on the spilled oil’s chemical composition involves multiple scientific disciplines with each discipline focusing on different aspects of weathering. Using the 2010 DwH oil spill as a case study, we have developed diagrams that outline the movement (into the air, water, soils, sediments, marine and mineral particles, and biota) and transformations (i.e., microbial and photooxidation) involved in the oil’s composition during weathering. To aid in understanding the fates of oil spilled into the ocean, these movements, phases, and transformation processes are outlined in Figures 5, 6.
Figure 5 Outline of the phases that are immediately encountered when oil spills into the environment. The circumstances of the spill (source, source composition, location, depth, weather, containing hydrocarbon molecules, etc.) will determine which phases will initially predominate. However, when oil is spilled, its hydrocarbons components are immediately distributed between these phases, and environmental weathering proceeds from the starting point of hydrocarbons in these phases.
Figure 6 Weathering encountered by spilled oil in different physical phases (gases, liquids, solids) in the marine environments following a well blowout. Remedial actions influence the partitioning into the different phases and the subsequent fate of the spilled oil. The figure does not include a possible methane hydrate solid phase that may develop in deep water. The term biomass refers to all living organisms in the marine environment as well as those organisms produced from metabolism of the spilled oil hydrocarbons.
When oil is spilled the components of this complex mixture of hydrocarbons and non-hydrocarbons enter the environment, and immediately begin to partition into the different phases as is shown in Figure 5. Thus, the oil’s initial composition quickly changes, as some compounds evaporate into the air, dissolve into the water, remain floating as liquid oil on the sea surface, or are dispersed below the surface as liquid oil droplets. Micro-droplets (<70 µm) remain suspended in the water column. The relative importance of these different processes depends upon the depth (pressure) and temperature where the spill occurs. As a result of this partitioning, the floating oil differs in composition from the initially spilled oil. Micro-droplets dispersed in the water may act as if they were dissolved in that they move with waves and currents in the water column rather than floating on the water’s surface. However, these micro droplets act like particles in that they can aggregate or be collected by filter feeding plankton.
The hydrocarbons in spilled oil are subjected to a plethora of further transport weathering processes including dilution/dissolution, evaporation, aerosolization, spreading, emulsification, aggregation, and sedimentation (Figure 5) (Payne et al., 1984; Payne and McNabb, 1984; Mackay and McAuliffe, 1988). Weathering also encompasses chemical transformations of the oil’s hydrocarbons via microbial and photochemical oxidations (Overton et al., 1980; Payne and Phillips, 1985; Liu et al., 2012; Tarr et al., 2016). Some oxidative transformations result in net loss of the oxidized compounds from the oil residues into the water column and air, while other photo induced oxidations convert crude oil components into higher molecular weight oxygenated compounds that become part of the oil residues (Hall et al., 2013; Ward et al., 2018) (Figure 6). Weathering, by altering the composition of the original oil, changes the oil’s physical, chemical, and biological (including toxic) properties, its distribution throughout the environment, as well as its response effectiveness to remediating measures.
For example, if fresh light oil, which is relative volatile, contains more water-soluble components, floats and is not very viscous, is spilled onto the sea surface, it easily spreads out from the source. These characteristics also imply that fresh light oil has a high potential for interactions with the many various phases and ecosystems as outlined in Figure 6. Fresh light oil is therefore often considered the most environmentally dangerous form of spilled oil because exposure pathways depend on the presence of oil in the respective ecosystems. Fresh oil exists mainly near the source, where concentrations are highest in the air, water column and on the water surface. As time passes, weathering allows the oil’s hydrocarbon compounds to not only be transformed, but also become diluted and dispersed by spreading. Toxic effects are very dependent on concentration, thus the perception that fresh light oil is more dangerous than weathered oil is based on both exposure as well as high concentrations before dilution. However, many exposure pathways are only now being discovered, e.g., grazing on marine oil snow (Podgorski et al., 2018; Van Eenennaam et al., 2018), or the increased solubility, potentially higher bioavailability, and toxicity of products of photooxidation (Zito et al., 2019). Therefore, this assessment of relative environmental danger may well be modified as we learn more about oil’s interactions in the environment.
As fresh oil weathers, it loses volatile components, including the more water-soluble components (i.e., the benzenes and naphthalenes), and the weathered oil residues become more viscous, emulsified and are more likely to glob or stick together as opposed to spreading out in a thin film while floating. Over time, weathering continues until much of its mass has been degraded, lost, or diluted to below detection, leaving behind solid residues like tarballs, tarmats, surface residue balls (SRBs), vegetated tar mats, or highly oxidized solid residues that form sinking particulates.
During the weathering process, much of the floating liquid oil will mix with water and can emulsify especially after photooxidations, forming a viscous mixture. Emulsified oil is also somewhat more difficult to remediate by skimming, dispersing, or burning. Emulsified oil is sticky and causes adverse effects mainly through covering or smothering as opposed to toxic interactions. However, if emulsified oil is ingested through, for example, the preening of feathers, it can have significant toxic effects on internal organs. Heavily emulsified oil is slower to degrade and will stay in the environment longer (i.e., be weathered slower) than non-emulsified liquid oil. Once stranded on shorelines, most emulsified oils are generally heated by the sun’s energy, causing the emulsifications to separate back into water and spilled viscous black oil residues that mixes with sand/sediment forming surface residue materials (Warnock et al., 2015), which covers rocks and other solid surfaces, or forms vegetated oil mats in coastal wetlands.
Oil spilled into deep-water environments also undergoes rapid weathering processes that initially include dissolution of the soluble light aliphatic (highly labile C1 to C10 fraction) and one to three ringed aromatic compounds from the liquid oil (the C1 to C10 and C10 to C25 fractions). Liquid oil droplets are buoyant and ascend to the surface. However, micro- droplets experience flow resistance from the water column and become essentially neutrally buoyant. These tiny droplets of liquid oil are advected away from their release point along with dissolved components including the emitted natural gas by deep-water currents (Gros et al., 2017; Payne and Driskell, 2018; French-McCay et al., 2018a, French- McCay et al., 2018b; Malone et al., 2018; Pesch et al., 2018; French-McCay et al., 2021). Both dissolved hydrocarbons and liquid oil micro-droplets can interact with marine snow and mineral particles and settle towards the seafloor in association with sinking aggregates (Lombard et al., 2013; Adhikari et al., 2015; Vonk et al., 2015; Adhikari et al., 2016b; Yan et al., 2016; Yan et al., 2016; Wirth et al., 2018; Adhikari et al., 2019). The tiny oil droplets can also undergo biodegradation, both in the water phase and when deposited onto bottom sediments or can be ingested and metabolized by deep-water organisms (Brakstad et al., 2015).
Of all the compounds in crude oils, PAHs are of greatest environmental concern because of their reactivity and toxicity when oxidized by mixed function oxidase enzymes in marine and terrestrial organisms (NRC Oil in the Sea 1985 and 2003). The chemical composition of PAHs in oils, and consequently their ability to inflict harm to marine and terrestrial communities, dramatically changes during weathering. The toxicity of oil’s hydrocarbon components depends upon the specific types of interactions with organisms, routes of exposure, and the component’s concentration. Typically, toxicity is estimated from the concentrations of aromatic compounds (i.e., PAHs) in oil and the various environmental phases, shown in Figure 5, 6, as determined from the quantitative analyses of a selected group of toxic aromatic molecular structures known as the “target aromatic compounds”.
The 5-6 ringed PAH aromatics and the resin/asphaltene portion of crude oils are insoluble and not rapidly biodegradable even in aerobic environments. Interestingly, all oil compounds, rapidly biodegradable or not, can ultimately be anaerobically degraded very slowly when deposited in sediments lacking oxygen, i.e., anoxic conditions (White et al., 2012; Montagna et al., 2013; Fisher et al., 2016).
PAH compounds in the environment originate predominately from two sources, petroleum (petrogenic sources) and combustion of fossil fuels or biomass (pyrogenic sources). Figure 7 provides an overview of the specific concentrations and distributions of the target PAHs in fresh Macondo oil (PAH profiles) and several environmental samples as these spilled compounds weathered during exposures to the elements. Also shown are the total concentrations and profiles of environmental PAHs in a surface sediment (in the lower left frame) that were produced by pyrogenic sources such as marsh fires or combustion of fossil fuels. As shown in the top part of Figure 7, petrogenic PAHs have relative low levels of the unsubstituted 2 to 6 ringed parent PAH compounds and substantially more of the C1 to C4 alkyl homologs of the parent PAH compounds. Conversely, pyrogenic derived PAH compounds are characterized by much higher levels of the parent unsubstituted PAH compounds and less of their respective alkyl homologs as is shown on the bottom left side of Figure 7. The right side shows that, as petrogenic PAHs weather, the two and three ringed PAHs in oils are lost first, and the parent and C1 alkyl homologs are lost more quickly than the C2 to C4 alkyl homologs (orange lines), whereas the four to six ringed PAHs are the last to be removed by weathering. The presence and isomeric position of alkyl substitutions in PAH will influence their rates of biodegradation, and photooxidation (Aeppli et al., 2014; Radović et al., 2014; Albaigés et al., 2016). Finally, peri-condensed structures (e.g., pyrene and benzo[a]anthracene) are more sensitive to photooxidation than cata-condensed ones (e.g., fluoranthene and chrysene) (Radović et al., 2014). High molecular weight (HMW) PAH compounds are generally associated with solid insoluble residues from oil weathering, and this insolubility limits their routes of exposure (Shigenaka et al., 2015; Adhikari et al., 2016a). By examining not only the quantity of PAHs in environmental samples but also their relative compositions (i.e., compositional profiles), scientists can infer their source (natural vs petrogenic vs pyrogenic) as well as assess their respective toxicities and potential routes of exposure (through water solubility and/or ingestion of oil residues).
Figure 7 Chemical compositions and compositional profiles of Petrogenic and Pyrogenic PAH compounds typically found in environmental samples, such as sediments collected from coastal marshes impacted by the 2010 DwH oil spill. (A) shows the PAH quantitative composition and composition profile of the source MC252 liquid Riser oil. The arrows indicate the relative quantities of un-substitutes or parent PAH compounds and the red dashed lines show portions of PAH compounds found with two and three rings and with four rings; (B) shows PAH quantitative composition in a sediment core sample containing no evidence of oil contamination, i.e., no hopane/sterane biomarker compounds and with parent PAH concentrations much higher than its alkyl homologs; (C–F) show PAH quantitative compositions and profiles in progressively more weathered oil residues (from top to bottom) extracted from coastal marsh sediments.
Sunlight causes photooxidations of the oil’s hydrocarbons into oxygenated analogs, and these oxy hydrocarbons have three main fates (Ward and Overton, 2021; Overton et al., 2021; Freeman and Ward, 2022). Some of the photo oxygenated compounds are water soluble and rapidly dissolve. Some newly formed oxy hydrocarbons act as surfactants, which allows and promotes formation of water in oil emulsions. A large portion of oxy hydrocarbons formed from photooxidations are soluble only in the weathered liquid oil residue. These oil-soluble oxy hydrocarbons become a component of the very viscous resin-asphaltene fractions of the weathered oil residues, thus dramatically changing the oil’s residue into a solid, very water insoluble material that only very slowly weathers further.
Biological processes are a major contributor to stranded and below the surface oil weathering. Preferential microbial degradations of specific compounds change the composition and characteristics of the oil. Additionally, in the presence of soluble oil compounds, many microbes release exopolymeric substances (EPS) that act as emulsifiers, surfactants, or dispersants (Rahsepar et al., 2016; Quigg et al., 2016; 2020; Bacosa et al., 2018; Bera et al., 2020). Such EPS may coat oil droplets or form aggregates that scavenge oil droplets and function as microenvironments. Dissolved oil compounds may sorb to biogenic or lithogenic particles in the water column and droplets may be included into sinking marine snow, which transports them to the seafloor (Brooks et al., 2015; Daly et al., 2016; Romero et al., 2015, 2017). Such sedimentation of weathered liquid oil droplets, which usually are less dense than seawater, leads to the oil residue’s sinking and exposure to deep-sea benthic organisms (Montagna et al., 2013; Fisher et al., 2016). Biodegradation rate is thought to be enhanced within the microenvironment of marine oil snow (Wozniak et al., 2019). In addition, the presence of marine snow-oil aggregates can potentially slow down the degradation of hydrocarbons in sediments (Rahsepar et al., 2017; Rahsepar et al., 2022).
During the DwH spill, oil droplets merged when reaching the sea surface, forming oil slicks and eventually oil streamers with vast areas of the northern Gulf’s surface covered by sheens (MacDonald et al., 2015). Because rising times of oil droplets ranged from three to four hours to >100 hours, the degree of weathering of the oil when it reached the sea surface varied. Smaller droplets were much more depleted of their aromatic content than larger ones because their rising speed was slower and because their ratio of surface area to volume larger. The application of dispersants at the wellhead, as during DwH, can additionally increase the partitioning of low-molecular weight aromatics (BTEX) to the water column (Jaggi et al., 2020). Once at the surface, rapid evaporation and photooxidation changed the composition of the floating oil, and thus it’s physical and chemical properties including its density, viscosity, and ability to interact with water to form emulsions (Stout and Payne, 2016b). In addition, it is possible that the use of in-situ-burning (ISB) contributed to the formation of oxidized species that partitioned as dissolved organics into the water column (Jaggi et al., 2019; Tomco et al., 2022), akin to what has been observed for some photooxidation products (Liu and Kujawinski, 2015; Zito et al., 2019). In addition, a portion of gaseous oxidized products of ISB can solubilize in the atmospheric water phase and be recycled back to the sea surface with rainfall.
Oil composition, collected from the floating oil slick at the sea surface off the coast at the mouth of the Mississippi River to when the floating oil stranded on coastal marshes are shown in Figures 8, 9. These compositional profiles reflect the results of the different weathering processes and weathering times before collection in early June 2010. Figure 8 compares the compositional details for two oil samples collected near coastal Louisiana in late May and early June of 2010.
Figure 8 Photos of typical floating emulsified oil (A, B) and suspended just below the surface oil particles (C, D) with their respective alkane chromatograms (E, F) and PAH compositional profiles (G, H). The inserts in the alkane chromatograms are blow-up portions showing the n-C17/pristine and n-C18/phytane pair of petrogenic compounds used to indicate microbial degradation in samples. The subsurface oil flock shows very limited initial biodegradation as compared to no biodegradation of the floating surface oil emulsion. Numbers in red are percent hopane normalized depletion, and in blue are percent sum of target aromatics divided by the sum of aromatics and alkanes. In the source oil, this ratio is ~13%. The subsurface flock was significantly degraded of its light PAH compounds when compared to the floating emulsion. However, the floating emulsion has lost to evaporation light alkanes below C13 when compared to the subsurface flock. PAH methyl isomer homolog profiles of phenanthrene, pyrene and chrysene (G, H) showed weathering in the subsurface flock sample when compared to the floating slick. The samples and pictures were collected and taken offshore from the Birds Foot delta of the Mississippi River during two research cruises in June 2010.
Figure 9 Compositional profiles of typical oil residues collected floating near the mouth of Mississippi River (A) and stranded oil residues on the shoreline of Bay Jimmy Louisiana (B) in 2010. The numbers in red are the hopane calculated depletion of the hydrocarbon residues compared to source liquid oil samples collected from the riser pipe. Similarly, the numbers in blue are the ratios of the sum of the target aromatic to total targeted aromatic and alkane hydrocarbons which is around 13% in the riser oil sample. The n-C17/pristane and n-C18/phytane ratio in floating oils show little change from that found in the liquid riser oil, implying essentially no biodegradation in floating oil (the top left inserts in each chromatogram). The same ratios found in stranded oil residues show significant depletion of the normal alkanes (first peak of each doublet) when compared to the pristine and phytane peaks (second compound in each doublet) indicating microbial depletion of normal alkanes in preference to isoprenoid alkanes. The stranded oil had lost significantly more of the two and three ringed PAH compounds relative to the four ringed PAH compounds when compared to floating oil residues.
Photographs documented the typical appearances of floating and near surface oil (Figure 8). The hopane-normalized emulsified oil slick (Figure 8A) was 66% depleted and the percent aromatics divided by total alkane and aromatics was slightly elevated (13 vs 14) when compared to Macondo source oil (definition of hopane-normalized depletion is in Stout et al., 2017). However, the oily material suspended just below the surface was hopane-normalized depleted by ~96% and their percent aromatics divided by total alkane and aromatics ratio was just 2.6%, indicating that light aromatic compounds had been preferentially removed compared to alkanes from this oily residue. These near surface oily residues were probably from small droplets of liquid oil that had been extensively water washed during their slow transit to the surface with preferential removal of the more water-soluble aromatic compounds compared to similar sized alkanes. Note that the alkane distribution of this near surface material was much less impacted by evaporation when compared to floating oil emulsions. Chemical analysis of hundreds of Natural Resource Damage Assessment (NRDA) samples from the surface and near surface oil (Stout and Payne, 2016a) also revealed that the floating oily residues were heavily depleted of aromatics compounds compared to saturates.
Before stranding on beaches and in coastal marshes, floating oil was subjected to extensive evaporation and photo induced oxidations (Ward et al., 2018; Ward and Overton, 2020). Figure 9 compares the compositional profiles of surface floating oil samples collected near the mouth of the Mississippi River in early June 2010 to similar compositional profiles of recently stranded oily material collected from the marsh shoreline in Barataria Bay, Louisiana (Nixon et al., 2016). The floating oils exhibit an increase in the chromatograms unresolved compounds in addition to evaporative loss of their normal alkanes below ~C13 and most of the naphthalenes and fluorenes PAH compounds. However, the ratios of the n-C17 to pristane and n-C18 to phytane was like that in the source oil (n-C17/Pr = 1.5 and n-C18/Ph = 2.3), meaning these oil samples had not been subjected to biodegradation. Oil residues were subjected to biodegradation only after stranding if deposited in a way that allowed ready access to nutrients and oxygen. The rapid uptake of oxygen to form oxidized petrocarbon compounds in floating oil residues with little change in biodegradation indicators, suggests the oxidations at the surface were photochemically catalyzed and not from microbial degradation (Ward et al. (2018). This matches well with the rapid loss of two and three ringed PAH compounds seen in floating oil residues (Stout et al., 2017) (Figure 9). Photo oxidations supported formation of emulsions, which increased the viscosity of floating oil (Payne and Phillips, 1985). Photooxidation also produced water soluble oxidized petrocarbon compounds, like carboxylic acids from alkanes, PAH alcohols, ketones, and sulfones (Patel et al., 1979; Overton et al., 1980; Niles et al., 2019; Zito et al., 2019; Freeman and Ward, 2022). Many of the higher molecular weight photo-oxidized hydrocarbons, instead of being soluble in water, were soluble in the oil residues and became a significant component of the oily residue’s mass (Ward et al., 2018; Ward et al., 2019). Others solubilized and became a part of the dissolved organic matter, DOM (Liu and Kujawinski, 2015). These amphiphilic oxy hydrocarbon compounds enhanced the floating oil residue’s ability to entrain water and become emulsified, as well as increased its viscosity. Further, these oxy hydrocarbons added to the oil residue’s matrix (i.e., the recalcitrant C25+ fraction) and changed the chemical properties of the photooxidized oil residues. This chemical transformation towards more polar hydrophilic products on the surface of the floating emulsions potentially lowered dispersant effectiveness (Ward et al., 2019). Stranded oil residues coated marsh grasses and formed thick vegetated oil mats that continued to be weathered eventually turning into insoluble solid material coating the impacted marsh surfaces and grasses and persisted until at least 2018.
The production of EPS (exopolymeric substances) by microbes, as a response to oil exposure, also influenced the expression, appearance, and fate of oil at the surface (see pictures in Figure 10). Likely, the cocktail of oxidized water-soluble oil compounds triggered the production of EPS by microbes (Xu et al., 2018a; Xu et al., 2018b, and Xu et al., 2019). Details of specific EPS-oil interactions are only slowly coming to light because of the complexity of both oil and EPS composition (Xu et al., 2018a; Xu et al., 2018b, and Xu et al., 2019 and references therein). During the spill, large mucus-rich particles formed that had a 14C isotopic signature identical to that of the spilled oil (Passow et al., 2019). These appeared to act as biofilms, allowing complex bacterial communities to grow, giving them access to oil and degradation products. Whereas these large bacterial oil agglomerations (BOA) initially floated at the surface, ballasting particles eventually attached to them, and this mucus-rich, bacterial marine snow sank. Sinking of BOAs and other marine oil snow has been referred to as MOSSFA: marine oil snow sedimentation and flocculent accumulation (Daly et al., 2016).
Figure 10 Photos of an emulsified surface slick and bacteria-oil agglomerations (BOA) suspended in the Gulf of Mexico water column just below the surface. The center picture insert (E) is a close-up of the EPS (extra polymeric substances) produced in a laboratory experiment showing the initial formation of MOS particles (marine oil snow) Pictures (A–C, F–H) were kindly provided with permission by the Oceans Future Society.
Compositional changes associated with the weathering of oil that stranded on shorelines are outlined in Figure 11. These bulk compositional changes occurred during the oil’s transit from floating offshore to stranding on sandy beaches and in coastal marshes (Wang and Roberts, 2013). This diagram functionally splits floating oil residues into two fractions, the labile C10 to C25 and the recalcitrant C25+ fractions while assuming that essentially all components in the very labile C1 to C10 fraction had been lost to evaporation and dissolution prior to stranding. The labile C10 to C25 fraction of light floating oil was composed of normal, branched, and cyclic alkanes and two to four ringed parent and alkyl homolog PAH compounds. These chemicals are susceptible to rapid weathering losses caused by evaporation, dissolution, and photo and microbial weathering (Bagby et al., 2017). Most of the floating oil emulsions had been weathered by evaporation and photooxidations with little biodegradation (Ward et al., 2018) as is seen in the unchanged normal to isoprenoid ratios in the floating oils. Once stranded, liquid oil residues stayed on the marsh surface and were mostly weathered by tidal washing, and microbial oxidative processes. These microbial degradations were indicated by the reduction of the n-C17 and n-C18 compounds compared to their respective quantities of the isoprenoid hydrocarbons pristane and phytane in stranded oil residue samples.
Figure 11 An overview of the bulk composition of Deepwater Horizon Oil calculated from simulated distillation data for the liquid source oil. The fresh floating oil sample was estimated from the chemical composition of target alkanes and PAH compounds, assuming that natural gas and very volatile compounds were rapidly lost during the transit of oil drops to the surface. The composition of average floating oil and average stranded oil was estimated from the target compound concentrations as presented in Stout et al., 2016, assuming 50% of the labile C10 to C25 fraction was lost and most of this 50% was converted to oil soluble oxy hydrocarbons that become a component of the C25+ fraction (Ward et al., 2018). Once stranded, oil residues were subjected to biodegradation with daily water washing by tidal flushing converting components of the labile C10 to C25 fraction into CO2 and biomass.
Figure 12 shows alkane chromatographic and PAH compositional profile data in recently stranded oil residue (Figure 12A), as well as a residue found in the top 5 cm of a sediment core collected in 2010 from an impacted coastal marsh (Figure 12B) and buried/entrapped oil residue collected subsurface from a fiddler crab burrow sampled in 2016 (Figure 12C).
Figure 12 Diagram estimating the composition of Deepwater Horizon Oil residues in three fractions as this spilled oil undergoes compositional changes during weathering. These fractions are: C1 to C10, containing hydrocarbons that are low molecular weight compounds and will rapidly be lost to evaporation and or dissolution, this fraction has been depleted in stranded oil residues; C10 to C25, containing moderate molecular weight hydrocarbon and NSO compounds that are susceptible to loss by evaporation, dissolution, microbial weathering and to photo oxidative production to oil soluble and water soluble oxy hydrocarbons products; C25+, containing higher molecular weight hydrocarbons and NSO compounds such as the 5-6 ringed PAH compounds and also includes resins, asphaltenes and oil soluble oxy compounds. As the relative composition of C25+ fraction gets larger, the oil residues become much more viscous, even solid, and are sticky, covering and coating stranded shorelines with an oily material that degrade very slowly. The oil residue shown on the right is from oil entrapped below a surface tarmats in an anaerobic zone, undergoing very slow anaerobic degradation, and still being liquid oil in 2016. The residue on the left is from a surface tarmat that had been heavily weathered, with little C10 to C25 fraction remaining in 2010. The top profile data show the typical chemical composition of weathered liquid oil that came ashore in 2010.
New research has shown that photooxidations of floating oil add to the C25+ fraction by production of oxy compounds that are soluble in the oil, not volatile, and insoluble in water (Niles et al., 2019). Heavily photochemically weathered floating oil residues contain large portions of C25+ chemicals (range) that are not readily lost during weathering, and that have a viscous solid-like appearance and that stick and coat surfaces when stranded on shorelines. Consequently, the shorelines along the northern Gulf were impacted not by a light crude oil, but by an emulsified oil that resembled the composition of a moderate to heavy crude oil. This stranded oil residue contained significant portions of “resin, asphaltenic and oxy hydrocarbon” material (heptane insoluble compounds) in addition to small quantities of its readily weathered C10 to C25 compounds and the target alkanes and larger PAH and alkyl PAH compounds in the C25+ fraction. The implications of this stranded oil’s composition were that a large portion of the stranded material did not get significantly removed from the environment by traditional weathering. Stranded oil on beaches was removed by mechanical cleanup machines. However, stranded oil in marsh environments, inaccessible to mechanical cleanup equipment, retained oily mats even through 2020. Something similar has been observed in the aftermath of the Ixtoc-1 spill (1979), a historic analog of the DwH, when a portion of surfaced oil ended up in marshy, sub-oxic mangrove environments of the Yucatan peninsula, where residues of Ixtoc-1 oil have been recently found, almost 40 years after the spill (Radović et al., 2020).
Depending on physical dynamics, sunken oil residues were buried in intertidal areas off sandy beaches. These submerged oil residue mats degraded very lowly, largely because of their limited water solubility and limited access to oxygen and nutrients. Coastal erosion processes subsequently dislodged these buried submerged oil residue mats during passage of storm and weather fronts. A portion of this dislodged oil residue material was washed upon beaches as tarball like oily residues and surface residue balls following the storm events. Such events of dislodging buried oil residues lasted for several years following the DWH spill and caused episodic re-contamination of coastal areas with small globs of heavily weathered oil residues mixed with sand and detritus (i.e., surface residue ball). Deposited shoreline oil poses exposure risk to intertidal organisms and bivalves such as oysters and clams.
As emulsified weathered and floating oil approached coastal wetlands, particularly at high tides, the oil slicks were washed into and onto coastal grasses, coating the leaves, stems, and sediments typically 10 to 30 meters inland from the marsh edges. Initially, before continued weathering and during the subsequent tidal cycles, some of the stranded oil was moved to previously unoiled coastal marsh areas. This movement of stranded oil on marshes did not significantly penetrate horizontally into marsh structures but remained mostly associated with the first 10 to 30 meters of marsh shoreline. The exception occurred during tropical weather events, when exceptional high tides and high wind energy distributed oily residues throughout previously unoiled interior marsh areas, albeit at much lower and diluted levels of contamination. A small fraction of these shoreline stranded oily residues, perhaps 2-3%, were washed by tidal action back into the first 50 meters or so of offshore/near shore bottom sediments (Michel et al., 2013; Lin et al., 2016; Willis et al., 2016). Continuous coastal erosion in coastal Louisiana increases this process, releasing buried shoreland stranded oil residues back into the waters as the coastline eroded. All residues were heavily weathered and depleted of their PAH content by >97% when sampled. However, target PAH concentrations were still an order of magnitude above pre spill concentrations in 2011/2012 (Turner et al., 2014, Turner et al., 2019) in areas associated with impacted coastal marsh sediments.
Oil residues in marshes showed two distinct weathering trends (Figure 12). Samples generally containing elevated levels of solid like oil residues that were globed together or coated on vegetative leaf structures (oily mats) showed less weathering than samples containing low levels of dispersed oil on sediment particles. Most of the stranded viscous thick oil residues forming the mats stayed on the marsh surface and were further weathered slowly by tidal washing and mostly microbial oxidative processes. Even the composition of the recalcitrant sterane petroleum biomarkers were altered by weathering in these surface oily vegetated mat samples. The eventual composition of these highly weathered oily residues, that made up the vegetative mats, contained hydrocarbon and oxy hydrocarbons founds in the recalcitrant C25+ fractions and retained only small amounts of the labile C10 to C25 hydrocarbon fraction.
In heavily oiled areas of the coastal wetlands, some of the thick emulsified oil on the original marsh surface was also trapped/buried under oiled vegetation mats and wrack. Some of this oil residue became re-buried over time by sediments/soils (Zengel et al., 2016). Figure 13 outlines the compositional profiles of four oil residues that were initially stranded in 2010 but were collected from the same location in the Bay Jimmy area of Barataria Bay, Louisiana in 2016. Surface sediment cores (vegetated tarmat material and oiled sediments) that had been extensively weathered are shown in Figures 13C, D. The upper right sediments showed extensive weathering including changes in the sterane biomarker profiles, in addition to loss of portions of the C1 to C3 alkyl chrysene isomer. Tarmat weathering (Figure 13D) showed less significant loss when compared to oil residues on sediments. This pattern of more severe weathering was generally found in sediment cores with small amounts of oily residues when compared to, for example, the oily residues of the vegetated tarmat material.
Figure 13 Outline of the compositional profiles of four oil residues stranded in coastal marshes in Bay Jimmy area of Barataria Bay Louisiana. The surface soil/sediment core samples impacted by the stranded DwH oil were collected in July 2016. Surface oil residues on sediments (A) and vegetated tarmat (B) were heavily weathered aerobically. Small amounts of stranded oil were trapped in abandoned fiddler crab burrows and under heavily weathered vegetated oil residues mats laying on the marsh shoreline when stranded in 2010. These latter stranded oil samples (C, D) underwent very slow anaerobic degradation over time when compared to surface oil residues in aerobic sands and sediments.
A small but significant portion of the initial stranded liquid oil residue in 2010 was trapped below surface in coastal fiddler crab burrows and under surface oil residue vegetated mats. This trapped oily material, shown on the Figurs 13A, B, weathered very slowly under anaerobic conditions, meaning there was little loss of target alkane and PAH compounds and biomarkers over at least a six-year period since 2010 (i.e., in the labile C10 to C25 fraction). The C1 to C3 isomer profiles of the alkylated chrysene homologs are also shown. Note that the subsurface isomer profiles were not modified when compared to those in the source oil (as shown in Figure 4), while the heavily weathered surface oil residues showed marked weathering of the chrysene isomer homologs. Interestingly, anaerobic degradation of the samples on the Figures 13A, B changed the PAH profiled over a six-year time frame, but the isomeric profiles of the alkyl chrysenes in this 6-year weathered oil closely resembled the isomeric profiles of the initial spilled oil, highlighting the differences between aerobic and anaerobic metabolisms.
Rapid weathering of the stranded oil residues in and on coastal marshes occurred mostly over a three-year period, reducing the quantity of detectable oil residues to near background levels by 2014 (Turner et al., 2014a; Turner et al., 2014b; Fleeger et al., 2015). The solid oily residues in coastal marshes were extensively weathered (depleted) of their target petrogenic PAH and alkane content, and these residues contained, in addition to their resin and native asphaltene content, high molecular weight oil soluble oxygenated hydrocarbon compounds photochemically produced in the floating oil prior to stranding (Ward et al., 2018; Ward et al., 2019). These vegetated tarmats covered anaerobic zones containing moderately weathered liquid oil residues in 2016 and beyond.
Figure 14 provides an overview of the saturate resolved and unresolved hydrocarbon profiles, the PAH concentration profiles, total PAH quantities in ppm, and the percent 2 and 3 ringed PAHs of total PAH compounds in representative samples of stranded oil residues during 2010. Two additional samples were collected in 2016, one of the surface vegetated tarmat and the other from liquid oil seeping from beneath the vegetated tarmat. Oil residues in surface samples, exposed to frequent tidal washing with oxygenated seawater, weathered very quickly, typically by the end of September 2010. In contrast, the composition of the vegetated tarmats changed very little between September 2010 through 2016. However, the tarmats covered portions of oil residues stranded 2010 in an environment depleted of oxygen (Zengel et al., 2015). This oil, trapped in anoxic environments, was only very modestly weathered when sampled in 2016. Further, vegetated oil residue mats inhibited the growth of marsh grasses, causing erosion of the coastline (Lin et al., 2016; Zengel et al., 2016). The fate of the solid oil residues on vegetated mats that have been eroded has not been established.
Figure 14 Overview of the alkane and PAH compositional trends for weathering of Macondo oil once stranded on Louisiana coastal marshes through the summer of 2010 and extended to 2016. Oil stranded in 2010 was weathered rapidly in aerobic environments with daily tidal surface washing with oxygenated seawater. A portion of the stranded oil was buried below vegetated surface tarmats and in fiddler crab burrows in anaerobic conditions, and this oil weathered much more slowing with compositional profiles in 2016 similar to recently stranded 2010 oil residues.
Petroleum that entered the waters of the Gulf of Mexico from the Macondo well blowout caused by the Deepwater Horizon disaster was a mixture of natural gas and a liquid oil saturated with natural gas. Shortly after release from the well’s damaged blowout preventer and ruptured piping, the natural gas dissolved into the surrounding water column and was advected away from the release point generally to the southwest. Much of the liquid oil, which had an initial density of ~0.82 g/ml, rose to the surface with speeds determined by drop sizes. Some small portion of the liquid oil drops, those with very small diameters (<0.1mm), experienced buoyancy rising resistance from the water column and were also advected from the wellhead’s location by deep sea currents. The dissolved natural gas and suspended liquid oil micro droplets (i.e., naturally dispersed oil) formed an intrusion plume ~200 meters above the initial seafloor location around the wellhead. The liquid oil micro droplets and dissolved natural gas moved towards the southwest and their components were detectable during the active portion of the release, from April 22 until capping on July 15, 2010, and for several months thereafter (Stout and Payne, 2016b).
Some of the petroleum fluid emitted from the blowout site was directed through a “riser” collection pipe to surface vessels and recovered. Natural gas was separated and flared while the liquid oil was collected. This liquid oil is the only portion that was collected of the pure liquid oil from the Macondo reservoir and is generally known as the Riser or Source Oil. Other “oil” samples were collected as either water or sediment samples containing liquid oil. These latter types of samples present issues related to accurately determining the fractions of very volatile compounds (i.e., the C1 to C10 fraction) and semi and nonvolatile compounds in the C10 to C25 and C25 + fractions, which also contains non-GC amenable oil material such as resins, asphaltenes, and oil soluble oxy hydrocarbons. Figures 2, 3, 4 outline the compositions of the petroleum fluids emitted from the wellhead as the liquid source oil collected from the riser pipe. These initial bulk compositional data are based on the Simulated Distillation analytical results found in Stout and German (2018) and Aeppli et al (2018).
In water and sediment samples containing oil residues, the C1 to C10 fraction had been essentially removed by evaporation and/or dissolutions. Therefore, the composition of freshly floating surface oil, relative to the Riser fluid, can be estimated to a first approximation as having lost most of its natural gas and volatile organic compound (VOC) content (Figure 11, Stout and German, 2018). Further, photochemical oxidation of approximately half of the labile C10 to C25 fraction occurred, and this oxidized material was converted into water-soluble DOM (Freeman and Ward, 2022) and oil soluble oxy hydrocarbon compounds that became components of the recalcitrant C25+ fraction (Ward et al., 2018). The fractional compositions of the average floating and stranded oil residues was estimated to a first approximations from Stout and German (2018) compositional data for the average floating and stranded oil samples. The main point of this is that, as the surface floating oil weathered by evaporation and photochemical oxidations (Ward et al., 2018; Freeman and Ward, 2022), the liquid floating oil residue was not only enriched in the high molecular weight fraction (C25 +) but also emulsified. Thus, the surface floating oily material that was stranded on beaches and in marshes was composed of a very significant portion of high molecular weight compounds that are solid in nature and very resistant to rapid weathering. In essence, the material stranded had lost 70 to 90% of the target alkane and PAH content (mostly in the C1 to C25 fractions) when compared to the liquid Riser Oil. Because the stranded oil was largely composed of high molecular weight compounds, it was very viscous, insoluble, and weathered slowly. Once stranded, oil residues underwent further weathering as outlines in Figures 11, 12. Aerobic oxidations removed most of the remaining C10 to C25 fraction within months and portions of the C25+ fraction’s PAH analytes, while the remaining solid sticky C25+ fraction persisted on stranded shorelines.
The composition of the weathered oil was significantly changed by loss of components due to evaporation, dissolution, and microbial and photo degradation (see processes in Figures 5, 6). Further, photochemical catalyzed oxidations of the oil’s components (Ward et al., 2018; Freeman and Ward, 2022) resulted in new oxy compounds being produced, some were water soluble and many of these compounds were soluble in the oil residue’s recalcitrant C25+ fraction (resin and asphaltene content) and became components of the residue’s “insoluble in heptane fraction” asphaltene fraction (i.e., the fraction functionally defined as the asphaltene fraction of oil).
The compositional differences of oil weathering in offshore waters and after stranding on beaches and in marsh environments is highlighted in Figures 8, 9. Offshore oil weathered in the deep sea and coastal waters during the summer of 2010 leaving behind oily residues containing mostly the C25+ fraction compounds. Oil residues stranded on beaches were mostly removed by physical labor and mechanical equipment, leaving behind small amounts of solid surface residue ball and some buried mats in intertidal zones. Oil residues stranded on the first 10-20 meters of marsh shoreline covered and coated marsh grasses causing thick viscous oily vegetated mats to be formed. In general, the C10 to C25 fraction of this surface oil weathered over 2-3 years, leaving behind solid residues coating the vegetated mats, and some persist to this day (see picture inserts in Figure 12). Portions of the marsh shoreline were lost to erosion, presumably spreading the insoluble solid oily residues as small particles around the estuary. This erosion process was speeded up by weather events like Tropical Storm Isaac (Bam et al., 2018). Oil residues entrapped under vegetated mats or in fiddler crab burrows weathered very slowly under anaerobic conditions, and small seeps of liquid oil can still be found in heavily impacted marsh areas of Bay Jimmy (as is shown in Figures 12, 13).
As oil weathers, its total amount is substantially reduced by evaporation and dissolution, but the composition of the remaining oil residue is also changed including by production of oxygenated water-soluble and oil-soluble oxy hydrocarbons (Ward et al., 2018; Freeman and Ward, 2022). The residue’s physical, chemical, and toxic properties are thus altered. In general, during weathering oils from the Gulf of Mexico go from a volatile, soluble, non-viscous and less dense than water mixture to a more viscous liquid that readily forms emulsions, and finally to a very viscous or solid, insoluble in water and sticky material residue. Concurrent with these changes in the oil residue’s physical and chemical properties are the oil residue’s ability to be biodegraded and photolyzed, and the speed with which the residue further weathers. In general, for South Louisiana Crude oils like the Macondo oil, initial weathering is very quick, minutes to hours, with well over 50% being lost in days to weeks. Weathering speeds of heavily weathered residues depend upon temporal conditions in the environment, but are much slower, typically taking months to years. Very heavily weathered oil residues, like the material that remains in coastal marshes as vegetated mats, can remain for decades, as observed in some analogous historic spill cases (Metula, Barge Florida, IXTOC -1, Exxon Valdez, etc.).
When oil is spilled, weathering processes reduce the quantity of spilled material that can be identified as oil, and change the residue’s chemical, physical and toxic properties. Impacts maybe expected not only from exposure to the oil at the sea surface, but also from potential exposures to the oil’s hydrocarbons that moved into the air, the water column or onto particles. Over time, hydrocarbon compounds are lost or transformed by photochemical and microbial oxidations. Oxidation leads to the formation of CO2, new biomass (bacteria via oil metabolism), and new water-soluble oxy hydrocarbons containing acidic, phenolic, ketonic, and aldehydic functional groups. Some new oxy hydrocarbons remain as a water insoluble component of the oil’s residue. As weathered residues spread into various environmental compartments, weathering continues, depending on the conditions of the new environment, and thus the type of impacts also change.
Oil spills are acute events, having potential for causing environmental impacts in the air, on the water’s surface, in the water column and bottom sediments, and in organisms inhabiting these impacted areas. However, as oil weathers, the residue become resistant to further rapid compositional changes, and this means that, if not removed by response personnel, residues can remain in environments for extended time, causing long term disruptions of impacted areas. The main point is that most environmental consequences from oil spills are caused by hydrocarbon material whose composition has changed, to lesser or greater degree, when compared to the initial spilled material. In many cases, the alterations represent significant compositional alterations affecting the residue material’s chemical, physical, toxic properties and affecting routes of exposure, and thus their potential for environmental impacts and remediations.
The datasets presented in this study can be found in online repositories. The names of the repository/repositories and accession number(s) can be found below: Gulf of Mexico Research Initiative Information & Data Cooperative (GRIIDC) at https://data.gulfresearchinitiative.org (doi: 10.7266/N7028PZZ; 10.7266/n7- czff-sm91; 10.7266/n7-tph2-3e25; 10.7266/n7-kdeg-nj49).
EO, UP, JR, and PA contributed to the development and writing of this manuscript. All authors contributed to the article and approved the submitted version.
We thank GoMRI “fates” workshop participants: PA, Chris Barker, Michel Boufadel, Peter Brewer, Edward Buskey, Tom Coolbaugh, Emily Maugh Douglas, John Farrington, the late David Hollander, Charlie Henry, Vijay John, Zanfei Liu, Jim Payne, Isabel Romero, Dalina Thrift- Viveros, Terry Wade, and Stephen Wise for sharing their knowledge. EO thanks GoMRI for financial support for this review, and UP thanks GoMRI and the Multi-Partner Research Initiative under Canada’s Ocean Protection Plan for funding her participation in this the review. We thank Rachael L. Stephan for her constructive comments on earlier versions, and we greatly appreciated the constructive and knowledgeable comments of the anonymous reviewers.
Dedicated to the memory and careers of Professors Louis J. Thibodeaux (1939-2020) and Ralph J. Portier (1952-2021), two great LSU colleagues and dear friends, both focused on using their engineering and scientific skills to understand how our natural system works and developing innovative solutions to environmental issues associated with our society’s use of fossil fuels and toxic chemicals. You are dearly missed by colleagues and friends.
The authors declare that the research was conducted in the absence of any commercial or financial relationships that could be construed as a potential conflict of interest.
All claims expressed in this article are solely those of the authors and do not necessarily represent those of their affiliated organizations, or those of the publisher, the editors and the reviewers. Any product that may be evaluated in this article, or claim that may be made by its manufacturer, is not guaranteed or endorsed by the publisher.
Abdel-Shafy H. I., Mansour M. S. M. (2016). A review on polycyclic aromatic hydrocarbons: Source, environmental impact, effect on human health and remediation. Egyptian J. Petroleum 25 (1), 107–123. doi: 10.1016/j.ejpe.2015.03.011
Adhikari P. L., Maiti K., Bam W. (2019). Fate of particle-bound polycyclic aromatic hydrocarbons in the river-influenced continental margin of the northern gulf of Mexico. Mar. pollut. Bull. 141, 350–362. doi: 10.1016/j.marpolbul.2019.02.046
Adhikari P. L., Maiti K., Bosu S., Jones P. R. (2016b). 234Th as a tracer of vertical transport of polycyclic aromatic hydrocarbons in the northern gulf of Mexico. Mar. pollut. Bull. 107, 1–9. doi: 10.1016/j.marpolbul.2016.04.002
Adhikari P. L., Maiti K., Overton E. (2015). Vertical fluxes of polycyclic aromatic hydrocarbons in the northern gulf of Mexico. Mar. Chem. 168, 60–68. doi: 10.1016/j.marchem.2014.11.001
Adhikari P. L., Maiti K., Overton E. B., Rosenheim B. E., Marx B. D. (2016a). Distributions and accumulation rates of polycyclic aromatic hydrocarbons in the northern gulf of Mexico sediments. Environ. pollut. 212, 413–423. doi: 10.1016/j.envpol.2016.01.064
Aeppli C., Carmichael C. A., Nelson R. K., Lemkau K. L., Graham W. M., Redmond M. C., et al. (2012). Oil weathering after the deepwater horizon disaster led to the formation of oxygenated residues. Environ. Sci. Technol. 46 (16), 8799–8807. doi: 10.1021/es3015138
Aeppli C., Nelson R. K., Radović J. R., Carmichael C.A., Valentine D. L., Reddy C. M. (2014). Recalcitrance and degradation of petroleum biomarkers upon abiotic and biotic natural weathering of deepwater horizon oil. Environ. Sci. Technol. 48, 6726–6734. doi: 10.1021/es500825
Aeppli C., Swarthout R. F., O'Neil G. W., Katz S. D., Nabi D., Ward C. P., et al. (2018). How persistent and bioavailable are oxygenated deepwater horizon oil transformation products? Environ. Sci. Technol. 52 (13), 7250–7258. doi: 10.1021/acs.est.8b01001
Albaigés J., Bayona J. M., Radović J. R. (2016). “Photochemical effects on oil spill fingerprinting,” in Standard handbook oil spill environmental forensics (Academic Press), 917–959.
Allan S. E., Smith B. W., Anderson K. A. (2012). Impact of the deepwater horizon oil spill on bioavailable polycyclic aromatic hydrocarbons in gulf of Mexico coastal waters. Environ. Sci. Technol. 46 (4), 2033–2039. doi: 10.1021/es202942q
Athas J. C., Jun K., McCafferty C., Owoseni O., John V. T., Raghavan S. R. (2014). An effective dispersant for oil spills based on food-grade amphiphiles. Langmuir 30 (31), 9285–9294. doi: 10.1021/la502312n
Atlas R. M., Hazen T. C. (2011). Oil biodegradation and bioremediation: a tale of the two worst spills in U.S. history. Environ. Sci. Technol. 45, 6709–6715. doi: 10.1021/es2013227
Bacosa H. P., Kamalanathan M., Chiu M.-H., Tsai S.-M., Sun L., Labonté J. M., et al. (2018). Extracellular polymeric substances (EPS) producing and oil degrading bacteria isolated from the northern gulf of Mexico. PloS One 13 (12). doi: 10.1371/journal.pone.0208406
Bagby S. C., Reddy C. M., Aeppli C., Fisher G. B., Valentine D. L. (2016). Persistence and biodegradation of oil at the ocean floor following deepwater horizon. Proc. Natl. Acad. Sci. U. S. A. 114 (1), E9–E18. doi: 10.1073/pnas.1610110114
Bam W., Hooper-Bui L. M., Strecker R. M., Adhikari P. L., Overton E. B. (2018). Coupled effects of oil spill and hurricane on saltmarsh terrestrial arthropods. PloS One 13 (4), e0194941.
Bera G., Doyle S., Passow U., Kamalanathan M., Wade T. L., Sylvan J. B., et al. (2020). Biological response to dissolved versus dispersed oil. Mar. pollut. Bull. 150, 110713. doi: 10.1016/j.marpolbul.2019.110713
Brakstad O. G., AlmÃ¥s I. K., Krause D. F. (2017). Biotransformation of natural gas and oil compounds associated with marine oil discharges. Chemosphere 182, 555–558. doi: 10.1016/j.chemosphere.2017.05.046
Brakstad O. G., Nordtug T., Throne-Holst M. (2015). Biodegradation of dispersed macondo oil in seawater at low temperature and different oil droplet sizes. Mar. pollut. Bull. 93, 144–152. doi: 10.1016/j.marpolbul.2015.02.006
Brooks G. R., Larson R. A., Schwing P. T., Romero I., Moore C., Reichart G. J., et al. (2015). Sedimentation pulse in the NE gulf of Mexico following the 2010 DWH blowout. PloS One 10, 1–24. doi: 10.1371/journal.pone.0132341
Burd A. B., Chanton J. P., Daly K. L., Gilbert S, Passow U, Quigg A.. 2020. The science behind marine-oil-snow and MOSSFA: Past, present, and future. Progress in Oceanography 187:102398. 10.1016/j.pocean.2020.102398
Chakraborty R., Borglin S. E., Dubinsky E. A., Andersen G., Hazen T. C. (2012). Microbial response to the MC-252 oil and corexit 9500 in the gulf of Mexico. Front. Microbiol. 3, 357. doi: 10.3389/fmicb.2012.00357
Clark R. C. Jr, Brown D. W. (1977). “Properties and analyses in biotic and abiotic systems. chapter 1,” in Effects of petroleum on Arctic and subarctic marine environments and organisms. Ed. Malins D. C. (New York: Academic Press, Inc), 1–89.
Crowley D., French-McCay D., Santos L., Chowdhury B., Markussen R. (2018). Modeling atmospheric volatile organic compound concentrations resulting from a deepwater oil well blowout – mitigation by subsea dispersant injection. Mar. pollut. Bull. 136, 152–163. doi: 10.1016/j.marpolbul.2018.09.001
Daly K. L., Passow U., Chanton J., Hollander D. (2016). Assessing the impacts of oil-associated marine snow formation and sedimentation during and after the deepwater horizon oil spill. Anthropocene, 1–16. doi: 10.1016/j.ancene.2016.01.006
Deleo D. M., Ruiz-Ramos D. V., Baums I. B., Coedes E. E. (2016). Response of deep-water corals to oil and chemical dispersant exposure. Deep Sea Res. Part II: Topical Stud. Oceanogr 129, 137–114.
Douben P. E. (2003). “PAHs: An ecotoxicological perspective,” in Ecological and environmental toxicology series. Ed. Douben P. E. T. (Hoboken: Wiley).
Doyle S. M., Whitaker E. A., De Pascuale V., Wade T. L., Knap A. H., Santschi P. H., et al. (2018). Rapid formation of microbe-oil aggregates and changes in community composition in coastal surface water following exposure to oil and the dispersant corexit. Front. Microbiol. 9. doi: 10.3389/fmicb.2018.00689
Driskell W. B., Payne J. R. (2018). Macondo oil in northern gulf of Mexico waters – part 2: Dispersant-accelerated PAH dissolution in the deepwater horizon plume. Mar. pollut. Bull. 129, 412–419. doi: 10.1016/j.marpolbul.2018.02.057
D’Sa E. B., Overton E. B., Lohrenz S. E., Maiti K., Turner R. E., Freeman A. (2016). Changing dynamics of dissolved organic matter fluorescence in the northern gulf of Mexico following the deepwater horizon oil spill. Environ. Sci. Technol. 50, 4940–4950. doi: 10.1021/acs.est.5b04924
Farrington J. W. (2014). Oil pollution in the marine environment II: Fates and effects of oil spills. Environ. Sci. Policy Sustain Dev. 56, 16–31. doi: 10.1080/00139157.2014.922382
Farrington J. W., Overton E. B., Passow U. (2021). Biogeochemical processes affecting the fate of discharged deepwater horizon gas and oil. Oceanography 24 (1). doi: 10.5670/oceanog.2021.118
Fisher C. R., Montagna P. A., Sutton T. T. (2016). How did the deepwater horizon oil spill impact deep-sea ecosystems? Oceanography 29, 182–195. doi: 10.5670/oceanog.2016.82
Fleeger J. W., Riggio M. R., Mendelssohn I. A., Lin Q., Deis D. R., Johnson D. S. (2019). What promotes the recovery of salt marsh infauna after oil spills? Estuaries Coasts 42 (1), 204–217. doi: 10.1007/s12237-018-0443-2
Freeman D. H., Ward C. P. (2022). Sunlight-driven dissolution is a major fate of oil at sea. Sci. Adv. 8 (7), eabl7605.
French-McCay D., Horn M., Li Z., Jayko K., Spaulding M., Crowley D., et al. (2018b). “Modeling distribution fate and concentrations of deepwater horizon oil in subsurface waters of the gulf of mexico. chapter 31,” in Oil spill environmental forensics case studies. Eds. Stout S., Wang Z. (Elsevier), 683–736. ISBN: 978-O-12-804434-6.
French-McCay D. P., Jayko K., Li Z., Spaulding M. L., Crowley D., l Mendelsohn D., et al. (2021). Oil fate and mass balance for the deepwater horizon oil spill. Mar. pollut. Bull. 171 (2021), 112681. doi: 10.1016/j.marpolbul.2021.112681
Gros J., Socolofsky S. A., Dissanayake A. L., Zhao L., Boufadel M. C., Reddy C. M., et al. (2017). Petroleum dynamics in the sea and influence of subsea dispersant injection during deepwater horizon. Proc. Natl. Acad. Sci. 114 (38), 10065–10070. doi: 10.1073/pnas.1612518114
Hall G. J., Frysinger G. S., Aeppli C., Carmichael C. A., Gros J., Lemkau K. L., et al. (2013). Oxygenated weathering products of deepwater horizon oil come from surprising precursors. Mar. pollut. Bull. 75 (1-2), 140–149. doi: 10.1016/j.marpolbul.2013.07.048
Harriman B., Zito P., Podgorski D. C., Tarr M. A., Suflita J. M. (2017). Impact of photooxidation and biodegradation on the fate of oil spilled during the deepwater horizon incident: Advanced stages of weathering. Environ. Sci. Technol. 51 (13), 7412–7421. doi: 10.1021/acs.est.7b01278
Head I. M., Gray N. D., Larter S. R. (2014). Life in the slow lane; biogeochemistry of biodegraded petroleum containing reservoirs and implications for energy recovery and carbon management. Front. Microbiol. 5. doi: 10.3389/fmicb.2014.00566
Hemmer M. J., Barron M. G., Greene R. M. (2011). Comparative toxicity of eight oil dispersants, Louisiana sweet crude oil (LSC), and chemically dispersed LSC to two aquatic test species. Environ. Toxicol. Chem. 30 (10), 2244–2252. doi: 10.1002/etc.619
Hodson P. V. (2017). The toxicity to fish embryos of PAH in crude and refined oils. Arch. Environ. Contam Toxicol. 73, 12–18. doi: 10.1007/s00244-016-0357-6
Honda M., Suzuki N. (2020). Toxicities of polycyclic aromatic hydrocarbons for aquatic animals. Int. J. Environ. Res. Public Health 17 (4), 1363. doi: 10.3390/ijerph17041363
Jaggi A., Radović J.R., Snowdon L. R., Larter S. R., Oldenburg T. B. P. (2019). Composition of the dissolved organic matter produced during in situ burning of spilled oil. Organic Geochem 138, 103926. doi: 10.1016/j.orggeochem.2019.103926
Jaggi A., Snowdon R. W., Radović J.R., Stopford A., Oldenburg T. B. P., Larter S. R. (2020). “Partitioning of organics between oil and water phases with and without the application of dispersants,” in In deep oil spills (Cham: Springer), 125–138.
Karthikeyan S., Kim M., Heritier-Robbins P., Hatt J. K., Spain J. C., Overholt W. A., et al. (2020). Integrated omics elucidate the mechanisms driving the rapid biodegradation of deepwater horizon oil in intertidal sediments undergoing oxic-anoxic cycles. Environ. Sci. Technol. [Environ Sci. Technol]. ISSN: 1520-5851, 2020 Jul 15.
Kleindienst S., Seidel M., Ziervogel K., Grim S., Loftis K., et al. (2015). Chemical dispersants can suppress the activity of natural oil-degrading microorganisms. Proc. Natl. Acad. Sci. 112, 14900–14905. doi: 10.1073/pnas.1507380112
Kostka J. E., et al. (2020). “Biodegradation of petroleum hydrocarbons in the deep Sea,” in Deep oil spills (Cham: Springer). doi: 10.1007/978-3-030-11605-7_7
Lin Q., Mendelssohn I. A. (2012). Impacts and recovery of the deepwater horizon oil spill on vegetation structure and function of coastal salt marshes in the northern gulf of Mexico. Environ. Sci. Technol. 46 (7), 3737–3743. doi: 10.1021/es203552p
Lin Q., Mendelssohn I. A., Graham S. A., Hou A., Fleeger J. W., Deis D. R. S. (2016). Response of salt marshes to oling from the deepwater horizon spill: Implications for plant growth, soil surface -erosion and shoreline stability. Total Environ. 557-558, 369–377. doi: 10.1016/j.scitotenv.2016.03.049
Li Z., Spaulding M., French McCay D., Crowley D., Payne J. R. (2017). Development of a unified oil droplet size distribution model with application to surface breaking waves and subsea blowout releases considering dispersant effects. Mar. Poll. Bull. 114, 247–257. doi: 10.1016/j.marpolbul.2016.09.008
Liu Y., Kujawinski E. B. (2015). Chemical composition and potential environmental impacts of water-soluble polar crude oil components inferred from ESI FT-ICR MS. PloS One 10 (9), p.e0136376. doi: 10.1145/2818302
Liu Z., Liu J., Zhu Q., Wu W. (2012). The weathering of oil after the deepwater horizon oil spill: insights from the chemical composition of the oil from the sea surface, salt marshes and sediments, environ. Res. Lett. 7(2012), 035302 (14pp). doi: 10.1088/1748-9326/7/3/035302
Lombard F., Guidi L., Kiørboe T. (2013). Effect of type and concentration of ballasting particles on sinking rate of marine snow produced by the appendicularian oikopleura dioica. PloS One 8 (9), e75676. doi: 10.1371/journal.pone.0075676
Lubchenco J., McNutt M. K., Dreyfus G., Murawski S.A, DM K., PT A., et al. (2012). Science in support of the deepwater horizon response. Proc. Natl. Acad. Sci. U.S.A. 109, 20212–20221. doi: 10.1073/pnas.1204729109
MacDonald I. R., Garcia-Pineda O., Beet A., Daneshgar A., Feng L., Graettinger G., et al. (2015). Natural and unnatural oil slicks in the gulf of Mexico. J. Geophys Res. Ocean 120, 3896–3912. doi: 10.1002/2015JC011062
Mackay D., McAuliffe C. D. (1988). Fate of hydrocarbons discharged at sea. Oil Chem. Pollut. 5 (1), 1–20.
Malins D. C.“Effects of petroleum on arctic and subarctic marine environments and organisms,” in V.1: Nature and fates of petroleum. v. 2: Biological effects (New York, San Francisco and London: Academic Press).
Malins D. C., Hodgins H. O. (1981). Petroleum and marine fishes: a review of uptake, disposition, and effects. Environ. Sci. Technol. 15 (11), 1272–1280. doi: 10.1021/es00093a001
Malone K., Pesch S., Schlüter M., Krause D. (2018). Oil droplet size distributions in deep-Sea blowouts: Influence of pressure and dissolved gases. Environ. Sci. Technol. 52 (11), 6326–6333. doi: 10.1021/acs.est.8b00587
Marzooghi S., Di Toro D. M. (2017). A critical review of polycyclic aromatic hydrocarbon phototoxicity models. Environ. Toxicol. Chem. 36, 1138–1148. doi: 10.1002/etc.3722
McKenna A. M., Nelson R. K., Reddy C. M., Savory J. J., Kaiser N. K., Fitzsimmons J. E., et al. (2013). Expansion of the analytical window for oil spill.
McNutt M. K., Camilli R., Crone T. J., Guthrie G. D., Hsieh P. A., Ryerson T. B., et al. (2012). Review of flow rate estimates of the deepwater horizon oil spill. Proc. Natl. Acad. Sci. U. S. A. 109 (50), 20260–20267. doi: 10.1073/pnas.1112139108
Meadors J., Stein J. E., Reichart W. L., Varanasi U. (1995). Bioaccumulation of polycyclic aromatic hydrocarbons by marine organisms. Rev. Environ. contamination Toxicol. 143, 79–165. doi: 10.1007/978-1-4612-2542-3_4
Michel J., Owens E. H., Zengel S., Graham A., Nixon Z., Allard T., et al. (2013). Extent and degree of shoreline oiling: Deepwater horizon oil spill, gulf of Mexico, USA. PloS One 8 (6), e65087. doi: 10.1371/journal.pone.0065087
Montagna P. A., Baguley J. G., Cooksey C., Hartwell I., Hyde L. J., Hyland J. L., et al. (2013). Deep-sea benthic footprint of the deepwater horizon blowout. PloS One 8, e70540. doi: 10.1371/journal.pone.0070540
National Research Council (1985). Oil in the Sea: Inputs, fates and effects (Washington, DC: The National Academies Press). doi: 10.17226/314
National Research Council (2003). Oil in the Sea III: Inputs, fates, and effects (The National Academies Press).
Neff, Anderson (1981). Malins and hodgins 1981; French-McCay 2002 (Hodson: National Research Council (NRC). 2017
Niles S. F., Chacón-Patiño M. L., Chen H., McKenna A. M., Blakney G. T., Rodgers R. P., et al. (2019). Molecular-level characterization of oil-soluble Ketone/Aldehyde photo-oxidation products by Fourier transform ion cyclotron resonance mass spectrometry reveals similarity between microcosm and field samples. Environ. Sci. Technol. 53 (12), 6887–6894. doi: 10.1021/acs.est.9b00908
Nixon Z., Zengel S., Baker M., Steinhoff M., Fricano G., Rouhani S., et al. (2016). Shoreline oiling from the deepwater horizon oil spill. Mar. pollut. Bull. 107, 170–178. doi: 10.1016/j.marpolbul.2016.04.003
Oldenburg T. B., Jones M., Huang H., Bennett B., Shafiee N. S., Head I., et al. (2017). The controls on the composition of biodegraded oils in the deep subsurface–part 4. destruction and production of high molecular weight non-hydrocarbon species and destruction of aromatic hydrocarbons during progressive in-reservoir biodegradation. Organic Geochem 114, 57–80. doi: 10.1016/j.orggeochem.2017.09.003
Olson G. M., Gao H., Meyer B. M., Miles M. S., Overton E. B. (2017). Effects of corexit 9500A on Mississippi canyon crude oil weathering patterns using artificial and natural seawater. Heliyon 3 (3). doi: 10.1016/j.heliyon.2017.e00269
Oost D., Beyer J., Vermeulen N. P. E. (2003). Fish bioaccumulation and biomarkers in environmental risk assessment: a review. Environ. Toxicol. Pharmacol. 13, 57–149. doi: 10.1016/S1382-6689(02)00126-6
Overton E. B., Laseter J. L., Mascarella S. W., Raschke C., Nuiry I., Farrington J. W. (1980). Photochemical oxidation of IXTOC I oil. Proc. Researcher/Pierce IXTOC I Symposium, 341–383.
Overton E. B., Meyer B. M., Miles M. S., Turner R. E., Adhikari P. L. (2021). Understanding the fates and chemical compositions of weathered oil in coastal marshes since the 2010 deepwater horizon oil spill. Int. Oil Spill Conf. 2021 (1), 687183. doi: 10.7901/2169-3358-2021.1.687183
Overton E. B., Wade T. L., Radović J. R., Meyer B. M., Miles M. S., Larter S. R. (2016). Chemical composition of macondo and other crude oils and compositional alterations during oil spills. Oceanography 29 (3), 50–63. doi: 10.5670/oceanog.2016.62
Overton E. B., Wetzel D. L., Wickliffe J. K., Adhikari P. L., et al (2020). “Chapter 3: Spilled oil composition and the natural carbon cycle: The true drivers of environmental fate and effects of oil spills, © springer nature Switzerland AG 2020 33,” in Scenarios and responses to future deep oil spills. Ed. Murawski S. A.doi: 10.1007/978-3-030-12963-7_3
Paris C. B., Le M., Aman Z. M., Subramaniam A., Helgers J., Wang D., et al. (2012). Evolution of the macondo well blowout: Simulating the effects of the circulation and synthetic dispersants on the subsea oil transport. Environ. Sci. Technol. doi: 10.1021/es303197h
Park and Holiday (1999). Available at: www.osha.gov/Publications/Oils_Spill_Booklet_05.11_v4.pdf.
Passow U., Stout S. A. (2020). Character and sedimentation of “lingering”. Macondo Oil to deep-sea after Deepwater Horizon Oil spill. Mar. Chem. 218, 103733.
Passow U., Sweet J., Francis S., Xu C., Dissanayake A. L., et al. (2019). Incorporation of oil into diatom aggregates marine ecology progress series612, 65–86. doi: 10.3354/meps12881
Passow U., Sweet J., Quigg A. (2017). How the dispersant corexit impacts the formation of sinking marine oil snow. Mar. pollut. Bull. 125, 139–145. doi: 10.1016/j.marpolbul.2017.08.015
Passow U., Ziervogel K., Asper V., Diercks A. (2012). Marine snow formation in the aftermath of the deepwater horizon oil spill in the gulf of Mexico. Environ. Res. Lett. 7. doi: 10.1088/1748-9326/7/3/035301
Passow U., Stout S. (2020) Character and Sedimentation of “Lingering” Macondo Oil to the Deep-Sea after the Deepwater Horizon Oil Spill. Marine Chemistry 218 (103733).doi: 10.1016/j.marchem.2019.103733
Passow U., Overton E. B. (2021) The Complexity of Spills: The Fate of the Deepwater Horizon oil, Annal Review Marine Science. Annu. Rev.Mar. Sci 13, 109–36
Patel J. R., Overton E. B., Laseter J. L. (1979). Environmental photo-oxidation of dibenzothiophenes following the Amoco cadiz oil spill. Chemosphere 8, 557–561. doi: 10.1016/0045-6535(79)90102-4
Payne J. R., Driskell W. B. (2018). Macondo oil in northern gulf of Mexico waters – part 1: Assessments and forensic methods for deepwater horizon offshore water samples. Mar. pollut. Bull. 129, 399–411. doi: 10.1016/j.marpolbul.2018.02.055
Payne J. R., Kirstein B. E., McNabb G. D. Jr., Lambach J. L., Redding R.E. Jordan R., Hom W., et al. (1984). “Multivariate analysis of petroleum weathering in the marine environment – subArctic environmental assessment of the alaskan continental shelf, OCSEAP,” in Final report of principal investigators, vol. 21 and 22, 690p.
Payne J. R., McNabb G. D. Jr. (1984). Weathering of petroleum in the marine environment. Mar. Technol. Soc. J. 18 (3), 24–42.
Payne J. R., Phillips C. R. (1985). Photochemistry of petroleum in water. J. Environ. Sci. Technol. 19 (7), 569–579. doi: 10.1021/es00137a602
Pesch S., Jaeger P., Jaggi A., Malone K., Hoffmann M., Krause D., et al. (2018). Rise velocity of live-oil droplets in deep-Sea oil spills. Environ. Eng. Sci. 35 (4), 289–299. doi: 10.1089/ees.2017.0319
Peters K., Walters C., Moldowan J. (2004). “Origin of petroleum,” in The biomarker guide (Cambridge: Cambridge University Press), 252–273. doi: 10.1017/CBO9780511524868.011
Podgorski D. C., Zito P., McGuire J. T., Martinovic-Weigelt D., Cozzarelli I. M., Bekins B. A., et al. (2018). Examining natural attenuation and acute toxicity of petroleum-derived dissolved organic matter with optical spectroscopy, environ. Sci. Technol. 52, 11. doi: 10.1021/acs.est.8b00016
Quigg A., Passow U., Chin W.-C., Bretherton L., Kamalanathan M., Xu C., et al. (2016). The role of microbial exoploymers in determining the fate of oil and chemical dispersants in the ocean. Limnol Oceanogr Lett. 1, 3–26. doi: 10.1002/lol2.10030
Quigg A., Passow U., Daly K. L., Burd A., Hollander D. J., Schwing P. T., et al. (2020). “Chapter 12: Marine oil snow sedimentation and flocculent accumulation (MOSSFA) events: learning from the past to predict the future. pp: 199-224,” in Deep oil spills – facts, fate and effects. Eds. Murawski S. A., Ainsworth C., Gilbert S., Hollander D., Paris C. B., Schlüter M., Wetzel D. (Springer), 608 pp, ISBN: ISBN 978-3-030-11605-7. doi: 10.1007/978-3-030-11605-7
Radović J.R. (2021). “Chapter 7 - chemical assessments of sources, fate, and impacts of marine oil spills,” in Marine hydrocarbon spill assessments. Ed. Makarynskyy O. (Elsevier), 221–243.
Radović J. R., Aeppli C., Nelson R. K., Jimenez N., Reddy C. M., Bayona J. M., et al. (2014). Assessment of photochemical processes in marine oil spill fingerprinting. Mar. pollut. Bull. 79 (1-2), 268–277. doi: 10.1016/j.marpolbul.2013.11.029
Radovic J., Xie W., Silva R., Oldenburg T., Larter S., Zhang C. (2021). Multi-proxy assessment of surface sediments using APPI-P FTICR-MS reveals a complex biogeochemical record along a salinity gradient in the Pearl River estuary and coastal South China Sea. EarthArxiv. doi: 10.31223/X5DP7P
Rahsepar S., Eenennaam J. S.V., Radović J.R., Oldenburg T. B. P., Rijnaarts H. H. M., Murk A. J., et al. (2022). Marine snow-oil interaction affects n-alkane biodegradation in sediment. Water Air Soil pollut. 233 (3), 1–11. doi: 10.1007/s11270-022-05557-1
Rahsepar S., Langenhoff A. M., Smit M. P. J., van Eenennaam J. S., Murk A. J., Rijnaarts H. M. (2017). Oil biodegradation: Interactions of artificial marine snow, clay particles, oil and corexit. Mar. pollut. Bull. 125 (1–2), (186–191). doi: 10.1016/j.marpolbul.2017.08.021
Rahsepar S., Smit M. P. J., Murk A. J., Rijnaarts H. H. M., Langenhoff A. A. M. (2016). Chemical dispersants: Oil biodegradation friend or foe? Mar. pollut. Bull. 108 (1-2), 113–119. doi: 10.1016/j.marpolbul.2016.04.044
Reddy C. M., Arey J. S., Seewald J. S., Sylva S. P., Lemkau K. L., et al. (2011). Composition and fate of gas and oil released to the water column during the deepwater horizon oil spill. Proc. Natl. Acad. Sci. 109, 20229–20234. doi: 10.1073/pnas.1101242108
Romero I. C., Schwing P. T., Brooks G. R., Larson R. A., Hastings D. W., Flower B. P., et al. (2015). Hydrocarbons in deep-sea sediments following the 2010 deepwater horizon blowout in the northeast gulf of Mexico. PloS One 10, 1–23. doi: 10.1371/journal.pone.0128371
Romero I. C., Toro-farmer G., Diercks A., Schwing P., Muller-Karger F., Murawski S., et al. (2017). Large-Scale deposition of weathered oil in the gulf of Mexico following a deep-water oil spill. Environ. pollut. 228, 179–189. doi: 10.1016/j.envpol.2017.05.019
Rouhani S., Baker M. C., Steinhoff M., Zhang M., Oehrig J., Zelo I. J, et al. (2017) Nearshore exposure to Deepwater Horizon oil, Marine Ecology Progress Series, HYPERLINK "https://www.jstor.org/stable/e26403663" Vol. 576 (August 3, 2017), pp. 111–-124
Ruddy R. M., Huettel M., Kostka J. E., Lodobin V. V., Bytheil B. J., Mckenna A. M., et al. (2014). Targeted petroleomics: Analytical investigation of macondo well oil oxidation products from pensacola beach. Energy Fuels 28 (6), 4043–4050. doi: 10.1021/ef500427n
Ryerson T. B., Camilli R., Kessler J. D., Kujawinski E. B., Reddy C. M., Valentine D. L., et al. (2012). Chemical data quantify deepwater horizon hydrocarbon flow rate and environmental distribution. Proc. Natl. Acad. Sci. U.S.A. 109, 20246–20253. doi: 10.1073/pnas.111056410
Ryerson T. B., et al. (2011). Atmospheric emissions from the deepwater horizon spill constrain air–water partitioning, hydrocarbon fate, and leak rate. Geophys Res. 38, L07803. doi: 10.1029/2011GL046726
Shigenaka G., Overton E., Meyer B., Gao H., Miles S. (2015). Physical and chemical characteristics of in-situ burn residue and other environmental oil samples collected during the deepwater horizon spill response. In Interspil Conf., 1–2.
Spier C., Stringfellow W. T., Hazen T. C., Conrad M. (2013). Distribution of hydrocarbons released during the 2010 MC252 oil spill in deep offshore waters. Environ. pollut. 173, 224–230. doi: 10.1016/j.envpol.2012.10.019
Stout S. A., German C. R. (2018). Characterization and flux of marine oil snow settling toward the seafloor in the northern gulf of Mexico during the deepwater horizon incident: Evidence for input from surface oil and impact on shallow shelf sediments. Mar. pollut. Bull. 129, 695–713. doi: 10.1016/j.marpolbul.2017.10.059
Stout S. A., Payne J. R. (2016a). Macondo oil in deep-sea sediments: Part 1 – sub-sea weathering of oil deposited on the seafloor. Mar. pollut. Bull. 111, 365–380. doi: 10.1016/j.marpolbul.2016.07.036
Stout S. A., Payne J. R. (2016b). Chemical composition of floating and sunken in-situ burn residues from the deepwater horizon oil spill. Mar. pollut. Bull. 108, 186–202. doi: 10.1016/j.marpolbul.2016.04.031
Stout S. A., Payne J. R., Emsbo-Mattingly S. D., Baker G. (2016). Weathering of field-collected floating and stranded macondo oils during and shortly after the deepwater horizon oil spill. Mar. pollut. Bull. 105, 7–22. doi: 10.1016/j.marpolbul.2016.02.044
Stout S. A., Rouhani S., Liu B., Oehrig J., Ricker R. W., Baker G., et al. (2017). Assessing the footprint and volume of oil deposited in deep-sea sediments following the deepwater horizon oil spill. Mar. pollut. Bull. 114 (1), 327–342. doi: 10.1016/j.marpolbul.2016.09.046
Tarr M. A., Zito P., Overton E. B., Olson G. M., Adhikari P. L., Reddy C. M. (2016). Weathering of oil spilled in the marine environment. Oceanography 29 (3), 126–135. doi: 10.5670/oceanog.2016.77
Tomco P. L., Duddleston K. N., Driskill A., Hatton J. J., Grond K., Wrenn T., et al. (2022). Dissolved organic matter production from herder application and in-situ burning of crude oil at high latitudes: Bioavailable molecular composition patterns and microbial community diversity effects. J. Hazardous Materials 424, 127598. doi: 10.1016/j.jhazmat.2021.127598
Turner R. E., Overton E. B., Meyer B. M., Miles M. S. (2014a). Hooper-bui 2014. changes in the concentration and relative abundance of alkanes and PAHs from the deepwater horizon oiling of coastal marshes. Mar. pollut. Bull. 86, 291–297. doi: 10.1016/j.marpolbul.2014.07.003
Turner R. E., Overton E. B., Meyer B. M., Miles M. S., McClenachan G., Hooper-Bui L., et al. (2014b). Distribution and recovery trajectory of macondo (Mississippi canyon 252) oil in Louisiana salt marshes. Mar. pollut. Bull. 87, 57–67. doi: 10.1016/j.marpolbul.2014.08.011
Turner R. E., Rabalais N. N., Overton E. B., Meyer B. M., McClenachan G. (2019). Oiling of the continental shelf and coastal marshes over eight years after the 2010 deepwater horizon oil spill. Environ. pollut. 252, 1367–1376. doi: 10.1016/j.envpol.2019.05.134
Van Eenennaam J. S., Rahsepar S., Radović J.R., Oldenburg T. B. P., Wonink J., Langenhoff A. A. M., et al. (2018). Marine snow increases the adverse effects of oil on benthic invertebrates. Mar. pollut. Bull. 126, 339–348. doi: 10.1016/j.marpolbul.2017.11.028
Vonk S. M., Hollander D. J., Murk A. J. (2015). Was the extreme and wide-spread marine oil-snow sedimentation and flocculent accumulation (MOSSFA) event during the deepwater horizon blow-out unique? Mar. pollut. Bull. 100, 5–12. doi: 10.1016/j.marpolbul.2015.08.023
Wang P., Roberts T. M. (2013). Distribution of surficial and buried oil contaminants across sandy beaches along NW Florida and Alabama coasts following tbe deepwater horizon oil spill in 2010. J. Coast. Res. 29(6A) (6A), 2013.
Wang, Stout (2007). Oil spill environmental forensics: Fingerprinting and source identification. doi: 10.1016/B978-0-12-369523-9.X5000-9
Ward C. P, Overton E. B (2020). Deepwater Horizon spill reshaped our understanding of crude oil photochemical weathering at sea: a past, present, and future perspective. Environ. Sci.: Processes Impacts. doi: 10.1039/d0em00027b
Ward C. P., Overton E. B. (2021). “May. synthesis of photochemical transformations of oil in the sea,” in International oil spill conference, vol. 2021, 688899).
Ward C. P., Sharpless C. M., Valentine D. L., Aeppli C., Sutherland K. M., Wankel S. D., et al. (2019). Oxygen isotopes (δ18O) trace photochemical hydrocarbon oxidation at the Sea surface. Geophys. Res. Lett. 46, 6745–6754. doi: 10.1029/2019GL082867
Ward C. P., Sharpless C. M., Valentine D. L., French-McCay D. P., Aeppli C., White H. K., et al. (2018). Partial photochemical oxidation was a dominant fate of_Deepwater horizon surface oil. Environ. Sci. Technol. 52 (4), 1797–1805. doi: 10.1021/acs.est.7b05948
Warnock A. M., Hagen S. C., Passeri D. L. (2015). Marine tar residues: a review. Water Air Soil pollut. 226, 68. doi: 10.1007/s11270-015-2298-5
Watson K. (2014)Spatial and temporal extent of a subsurface hydrocarbon intrusion following the deepwater horizon blowout. In: . Available at: https://scholarcommons.usf.edu/etd/5150.
White H. K., Hsing P.-Y., Cho W., Shank T. M., Cordes E. E., Quattrini A. M., et al. (2012). Impact of the deepwater horizon oil spill on a deep-water coral community in the gulf of Mexico. PNAs 109, 1–6. doi: 10.1073/pnas.1118029109
White A. R., Jalali M., Sheng J. (2020). Hydrodynamics of a rising oil droplet with bacterial extracellular polymeric substance (EPS) streamers using a microfluidic microcosm. Front. Mar. Sci. 7. doi: 10.3389/fmars.2020.00294
White H. K., Wang C. H., Williams P. L., Findley D. M., Thurston A. M., Simister R. L., et al. (2016). Long-term weathering and continued oxidation of oil residues from the deepwater horizon spill. Mar. pollut. Bull. 113, 380–386. doi: 10.1016/j.marpolbul.2016.10.029
Willis J. M., Hester M. W., Rouhani S., Steinhoff M. A., Baker M. C. (2016). Field assessment of the impacts of deepwater horizon oiling on coastal marsh vegetation of Mississippi and Alabama. Environ. Toxicol. Chem. 35 (11), 2791–2797. doi: 10.1002/etc.3450
Wirth M., Passow U., Jeschek J., Hand I., Schulz-Bull D. E. (2018). Partitioning of oil compounds into marine oil snow: Insights into prevailing mechanisms and dispersant effects. Mar. Chem. 206, 62–73. doi: 10.1016/j.marchem.2018.09.007
Wise S. A., Rodgers R. P., Reddy C. M., Nelson R. K., Kujawinski E. B., Wade T. L. (2022) Advances in Chemical Analysis of Oil Spills Since the DeepwaterHorizon Disaster. Critical Reviews in Analytical Chemistry. doi:10.1080/10408347.2022.2039093
Wozniak A. S., Prem P., Obeid W., Waggoner D., Quigg A., Xu C., et al. (2019). Rapid degradation of oil in mesocosm simulations of marine oil snow events. Environ. Sci. Technol. 53, 3441–3450. doi: 10.1021/acs.est.8b06532
Xu C., Xing W., Hatcher P. G., Waggoner D. D., Sun L., Chin W.-C., et al. (2019). Comparison of microgels, extracellular polymeric substances (EPS) and transparent exopolymeric particles (TEP) determined in seawater with and without oil. Mar. Chem. 215. doi: 10.1016/j.marchem.2019.103667
Xu C., Zhang S., Beaver M., Lin P., Sun L., Doyle S. M., et al. (2018b). The role of microbially-mediated exopolymeric substances (EPS) in regulating macondo oil transport in a mesocosm experiment. Mar. Chem. 206, 52–61. doi: 10.1016/j.marchem.2018.09.005
Xu C., Zhang S., Beaver M., Wozniak A. S., Obeid W., Lin Y., et al. (2018a). Decreased sedimentation efficiency of petro-carbon and non-petro-carbon caused by a dispersant for macondo surrogate oil in a mesocosm simulating a coastal microbial community. Mar. Chem. 206, 34–43. doi: 10.1016/j.marchem.2018.09.002
Yan B., Passow U., Chanton J. P., Nöthig E.-M., Asper V., Sweet J., et al. (2016). Sustained deposition of contaminants from the deepwater horizon spill. Proc. Natl. Acad. Sci. 113, E3332–E3340. doi: 10.1073/pnas.1513156113
Zengel S., Bernik B. M., Rutherford N., Nixon Z., Michel J. (2015). Heavily oiled salt marsh following the deepwater horizon oil spill, ecological comparisons of shoreline cleanup treatments and recovery. PloS One 10, e0132324. doi: 10.1371/journal.pone.0132324
Zengel S., Pennings S. C., Silliman B., Montague C., Weaver J., Deis D. R., et al. (2016). Deepwater horizon oil spill impacts on salt marsh fiddler crabs (Uca spp.). Estuaries coasts 39 (4), 1154–1163. doi: 10.1007/s12237-016-0072-6
Keywords: deepwater horizon oil spill, Gulf of Mexico, oil weathering, photooxidation, transport and fates, oil residues
Citation: Overton EB, Adhikari PL, Radović JR and Passow U (2022) Fates of petroleum during the deepwater horizon oil spill: A chemistry perspective. Front. Mar. Sci. 9:928576. doi: 10.3389/fmars.2022.928576
Received: 27 April 2022; Accepted: 07 July 2022;
Published: 09 August 2022.
Edited by:
Andrew James Manning, HR Wallingford, United KingdomReviewed by:
Yuhe He, City University of Hong Kong, Hong Kong SAR, ChinaCopyright © 2022 Overton, Adhikari, Radović and Passow. This is an open-access article distributed under the terms of the Creative Commons Attribution License (CC BY). The use, distribution or reproduction in other forums is permitted, provided the original author(s) and the copyright owner(s) are credited and that the original publication in this journal is cited, in accordance with accepted academic practice. No use, distribution or reproduction is permitted which does not comply with these terms.
*Correspondence: Edward B. Overton, ZWJvdmVydEBsc3UuZWR1
Disclaimer: All claims expressed in this article are solely those of the authors and do not necessarily represent those of their affiliated organizations, or those of the publisher, the editors and the reviewers. Any product that may be evaluated in this article or claim that may be made by its manufacturer is not guaranteed or endorsed by the publisher.
Research integrity at Frontiers
Learn more about the work of our research integrity team to safeguard the quality of each article we publish.