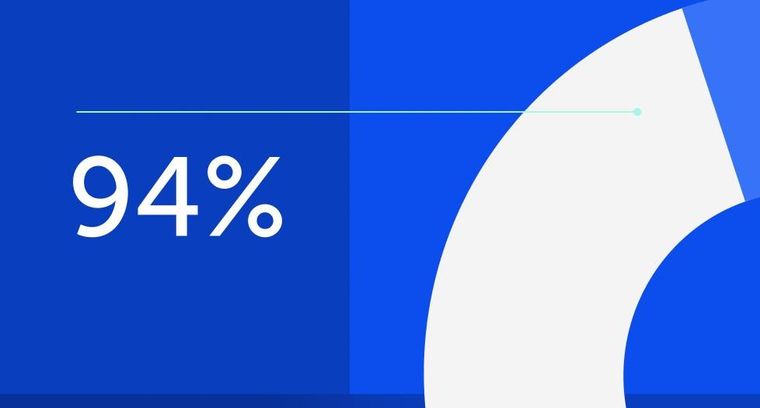
94% of researchers rate our articles as excellent or good
Learn more about the work of our research integrity team to safeguard the quality of each article we publish.
Find out more
ORIGINAL RESEARCH article
Front. Mar. Sci., 04 August 2022
Sec. Marine Conservation and Sustainability
Volume 9 - 2022 | https://doi.org/10.3389/fmars.2022.926442
This article is part of the Research TopicIdentifying and Comparing Important Areas for Marine Sustainable Use and ConservationView all 19 articles
Understanding metapopulation dynamics is critical for effective species conservation, but they are not always considered for marine species due to difficulties in assessing connectivity in marine environments. This is particularly true for species that are rare or threatened, as demographic and life history data are usually sparse. We employ Lagrangian Particle Tracking (LPT) to assess metapopulation dynamics and inform spatial management measures for the Atlantic Mud Piddock (AMP; Barnea truncata), a poorly studied and threatened marine bivalve mollusk in Canada, whose distribution in the country is limited to a single population in the Minas Basin, Nova Scotia. In a series of simulations designed to account for uncertainty in biological attributes of AMP, we identified that sub-populations along the southern coastline of the Minas Basin were the most strongly connected to other sub-populations by acting as the greatest sources and sinks of simulated larvae. Propagules released from the Minas Basin dispersed as far as the US coast of the Gulf of Maine, which harbors the closest known population of AMP outside of the Minas Basin. However, there was no exchange of larvae in the opposite direction, from the US population of AMP in the Gulf of Maine to the Minas Basin. These results suggest that sub-populations in the Minas Basin are self-sustaining (i.e., sub-populations that exchange larvae and ultimately act as a meta-population), supporting the need to protect critical source sites along the southern coastline for the regional persistence of this species. More generally, these results show how LPT outputs can be directly applied to conservation planning, and used to identify key knowledge gaps to address with future work to reduce uncertainty in model predictions.
Maintaining metapopulation connectivity has emerged as a primary goal of species conservation due to its role in supporting resilience in seascapes and landscapes where disturbances are heterogeneous in space and time (Crowder et al., 2000; Botsford et al., 2001; Gaines et al., 2003; Samways et al., 2020). Disturbances can cause significant population loss on local scales, but this can be mitigated by the immigration of individuals or propagules from nearby sub-populations (Brown and Kodric-Brown, 1977; Pulliam, 1988; Heinrichs et al., 2018). Protecting multiple connected sub-populations (i.e., metapopulation) and the mechanisms by which they are connected reduces the likelihood that a species will become extinct due to a local disturbance (Hanski 1998; Cowen et al., 2006; Treml et al., 2008), and supports resilience by promoting genetic diversity (Hanski and Gilpin, 1997). However, it is not always practical to protect entire metapopulations, so sub-populations are often prioritized for conservation based on their relative contribution to overall metapopulation sustainability, with sub-populations identified as primary sources of individuals receiving the highest priority (Crowder et al., 2000).
Conservation measures may fall short of meeting target objectives if they fail to account for source-sink dynamics (Burgess et al., 2014), yet in many cases connectivity is not accounted for in conservation planning (Gilroy & Edwards, 2017). This is likely because of the complexity of describing source-sink dynamics, which traditionally requires detailed estimates of population growth rate based on births, deaths, and emigration/immigration (Holt, 1985; Pulliam, 1988; Runge et al., 2006). Immigration and emigration can be estimated from direct measurements of species movements between sub-populations (e.g., tagging, tracking and mark/recapture studies), but such measures are difficult or impossible to acquire for marine species that are microscopic and/or those that disperse during larval stages. (DiBacco et al., 2006)). As a result, connectivity via larval dispersal remains one of the most common areas of uncertainty in the design and optimization of Marine Protected Areas employed to identify and protect meta-populations (Cowen and Sponagule, 2009; Burgess et al., 2014)
Conservation planning is especially challenging for rare and cryptic species for which distribution and demographic data are usually sparse or incomplete. Genetic analysis may be a powerful tool in these situations for describing population structure (Storfer et al., 2006; Peery et al., 2008), but genetic techniques have their limitations (Lowe and Allendorf, 2010). Moreover, collecting tissue samples for genetic analysis can be difficult and potentially damaging for populations of rare and threatened species. Coupled biological-physical models are a complementary and, in some cases primary method for evaluating population connectivity and assessing source/sink dynamics. Lagrangian particle track (LPT) modelling is used to describe the movements of simulated larvae, spores, sediments, plastics, etc. (i.e. particles) in the ocean environment, and has a range of applications (van Sebille et al., 2018), including modelling larval dispersal and assessing population connectivity (North et al., 2008; Botsford et al., 2009; Davies et al., 2014; van Sebille et al., 2018). Model outputs can be used to quantify larval exchange among extant sub-populations or defined regions and to generate connectivity matrices. Particle tracking is advantageous over other methods of assessing connectivity in that it demands relatively limited empirical biological data and can be used more easily than other methods to investigate connectivity over a range of spatial scales. Moreover, LPT offers the opportunity to analyze connectivity over a range of time scales, including those which are shorter than detected by genetics, and the influence of stochastic events, seasonal and interannual change. Where there is uncertainty in the biology of a species, LPT can be used to explicitly test the effects and sensitivity of unknown parameters on connectivity (e.g., swimming speeds, larval development time [PLD]). Prior studies have shown good concordance between particle modelling results and other methods of assessing connectivity, such as genetics and empirical connectivity data (Haase et al., 2012; Sponagule et al., 2012; Pujolar et al., 2013, Davies et al., 2014).
Barnea truncata, commonly known as the Atlantic mud-piddock (AMP) is a threatened species of bivalve in Canada (COSEWIC, 2009). Throughout its global range, it is found intertidally in soft muds, mudstones, and peats (Frank, 2009), but it’s distribution in Canada is limited to the red mudstone found only at its northern range limit in the Minas Basin, Nova Scotia. The viability of available red mudstone habitat for AMP in the Minas Basin has been variable through time, owing to strong tidal forces, storm-driven coastal erosion, and sediment deposition, leading to sub-population instability in some parts of the Minas Basin (COSEWIC, 2009; Clark et al., 2019). Moreover, climate change, dredging, coastal development, and tidal energy extraction pose more recent and serious threats to the persistence of AMP sub-populations (DFO, 2010; Wu et al., 2015). Given the restricted range of the species in Canada, limited habitat availability, and current and future threats to its population, AMP was listed as Threatened on Schedule 1 of the Species at Risk Act (SARA) in 2017. The nearest known population outside of the Minas Basin is at least 350 km away in the US portion of coastal Gulf of Maine, and currently it is unknown whether these two distant sub-populations are connected. Such information is critical towards understanding whether the AMP population in the Minas Basin is potentially self-sustaining and what implications population loss in the Minas Basin or coastal Gulf of Maine has for the regional persistence of the species.
Identifying critical habitat and describing sub-population connectivity within the Minas Basin and between the US and Canada are key aspects of the AMP recovery strategy (DFO, 2010). In response to this, field surveys were conducted in 2017-2018 to describe the current distribution and extent of known sub-populations of AMP in the Minas Basin, and to provide some qualitative estimates of changes in sub-population sizes at extant sites based on limited historical data (Clark et al., 2019). Clark et al. (2019) identified 16 AMP sub-populations within the Minas Basin, but there is no existing information on sub-population connectivity among these sites (COSEWIC, 2009; Clark et al., 2019). More generally, little is known about the biology of the species, with most information available for Canadian sub-populations originating from personal communications, records of occurrence, and taxonomic and morphological descriptions (COSEWIC, 2009; Clark et al., 2019). There have been only a few studies of AMP throughout its global range published in the primary literature (see COSEWIC, 2009), with one study of larval development rates conducted in Virginia, USA (Chanley, 1965).
In this study, we conduct model experiments to investigate factors influencing metapopulation dynamics of AMP by simulating the dispersal of AMP larvae (i.e. particles) within and outside of the Minas Basin using LPT modelling. We parameterize the model based on the best available information on the species’ distribution, spawning seasonality, larval development, and sinking and swimming behavior. Model outputs are used to describe potential connectivity (hereafter referred to as connectivity) between local, disjunct sub-populations and distinct sub-regions within the Minas Basin. Given uncertainty in some of the biological characteristics of the species, we also investigate how connectivity varies among spawning months, planktonic larval durations (PLD), and larval behaviors (i.e. passively sinking vs. active vertical swimming). Connectivity was also assessed for larvae originating from known sub-populations in the Minas Basin to regions of the Bay of Fundy and Gulf of Maine, which represents the northern range limit of B. truncata outside of Canada (COSEWIC, 2009), and from the Gulf of Maine to the Minas Basin. Our results describe source-sink dynamics and inform the prioritization of sub-populations within the Minas Basin for conservation measures. We highlight key issues for future study on this species, including where future field sampling efforts should be directed to better characterize the distribution of the species in Atlantic Canada and New England, USA.
To simulate spawning and larval dispersal, particles were seeded in particle tracking simulations at 16 sites where AMP are known to occur within the Minas Basin (i.e. suitable habitat, Figure 1), as identified by field surveys in Clark et al. (2019). Sites were defined as circles whose centroid points were located where noted by field surveys (Clark et al., 2019) and size scaled to the population area recorded in Clark et al. (2019). Particles were then randomly seeded throughout each site, with the numbers of particles per site proportional to the total area for each site (total particles summed across sites = 50,000) (Figure 1). To investigate the potential for larvae released in the Gulf of Maine to reach the Minas Basin, particles were released in 2 bands of varying widths (0.5 km and 1 km) that extended continuously along the outer New England coast from Ipswich Bay, Massachusetts to the Canada/US border (0.5 km width band shown in Figure S1). This approach was used because reports of AMP populations along the Gulf of Maine are anecdotal with no coordinates associated with observations (COSEWIC, 2009). Using a band gives the best chance at identifying any location in the Gulf of Maine that potentially contributes as a source of larvae to the Bay of Fundy. The chosen band widths represent our estimate of the potential distribution of larvae spawned from this high intertidal species in the early days of larval release. The 0.5 km band width represents the most likely nearshore distribution of the larvae within a couple days of spawning but including a 1.0 km band allows us account for some uncertainty in this estimate in our assessment of potential connectivity.
Figure 1 (A) Site locations (yellow and black circles) within sub-regional delineations (as colored bands) in the Minas Basin. (B) Broader regional delineations, including the Minas Basin (NW, NE, SW, S, SW 1 and SW 2), inner, middle, and outer regions of the Bay of Fundy (BoF), and northern, middle and southern regions of the Gulf of Maine (GoM).
In addition to advection and diffusion, particles moved vertically according to two particle vertical “behaviors”: 1) sinking (i.e. passive), and 2) sinking plus vertical swimming (i.e. swimming). For swimming simulations, larvae would swim towards a preferred depth at each model time step at a swimming speed that was randomly selected from a normal distribution with a mean and standard deviation of 2.4 and 2 mm s-1, respectively, to a maximum of 3 mm s-1 and a minimum of zero (Chia et al., 1984; Tremblay and Sinclair, 1990). There have been no direct studies of swimming behaviour in AMP, so we based this behaviour in our model on in situ observations of other bivalve larvae studied in the Bay of Fundy (e.g., Tremblay and Sinclair, 1990). The mean preferred depth was set at 15 m, which corresponds approximately to the model’s estimate of the average summer mixed layer depth throughout the Bay of Fundy. Where the bathymetry was shallower than 15 m, the particle’s preferred depth was instead set to half of the bathymetry. A random quantity within the range of 75% of the distance between the preferred depth and the bathymetric depth (to a maximum of 10 m) was added to the preferred depth to give some variation in particle preferred depth in a given location. The sinking rate of particles was set to a random quantity sampled from a normal distribution with a mean and standard deviation of 0.6 and 0.3 mm s-1, respectively, to a maximum of 1.3 mm s-1 and minimum of zero (Mann et al., 1991). If particles reached the surface or lowest depth limit of the model domain or the coastline, they were reflected at a displacement equal to the distance the particle would have travelled out of the model domain.
Planktonic larval duration (PLD) has been estimated at around 35 days from a laboratory study conducted in Virginia, USA (Chanley, 1965). It is expected that the PLD may be longer for the population in the Bay of Fundy, however, as cooler waters typically result in longer larval development time (Widdows, 1991; COSEWIC, 2009; Talmage and Gobler, 2011). Given this uncertainty, we evaluated source/sink connectivity at two maximum PLDs (40 days and 56 days) and implemented a competency period corresponding to the last 8 days of the PLD, during which larvae can settle if they encounter suitable habitat (i.e. realized model PLDs are 33-40 days, and 49-56 days) and particles are assumed to settle at first contact with suitable habitat (defined as suitable habitat sites, as above, or sub-regions defined within the Minas Basin, Bay of Fundy, and Gulf of Maine, see below). Chanley (1965) spawned AMP in Virginia from mid-May through September, but spawning has been observed to start later in the Minas Basin due to colder water temperatures (A. Hebda, pers. Comm.). Therefore, we simulate spawning in July and August.
The hydrodynamic model used in this study is based on the Finite-Volume Community Ocean Model (FVCOM), which solves the free surface, employs mode time split technology, and uses sigma coordinates in the vertical direction and a triangle mesh system in the horizontal directions (Chen et al., 2003; Chen et al., 2006). The highest resolution is in shallow coastal waters, including the Minas Basin. The model topography is based on high resolution survey data (50 to 200 m) from the Canadian Hydrographic Service (CHS) and smoothed to reduce sigma-coordinate pressure gradient truncation errors. The model is vertically discretized into 45 layers based on a hybrid terrain-following coordinate, with the vertical resolution of the model increasing near the surface and bottom (0.3-3m resolution in the surface layers above 15m). The triangle grid system is spatially flexible to fit complex coastal lines with the grid cell size ranging from 0.0028 km2 in near-shore waters to 73.85 km2 in the open ocean. At the same time, flooding and drying processes in tidal flat areas are simulated with mass-conserving wetting and drying treatment. The model domain covers the Bay of Fundy, Gulf of Maine, Georges Bank and Scotian Shelf, extending to the shelf break. The model runs under air forcing, initial conditions and lateral boundary conditions of water elevations, currents, temperature, and salinity derived from the daily reanalysis results of GLORYS12v1 (Jean-Michel et al., 2021). At the ocean boundaries, harmonic constants (amplitudes and phases) of seven major tidal constituents (M2, S2, N2, O1, K1, P1, and Q1) are derived from the tidal dataset of FES2014 (Lyard et al., 2016). The model has been validated with observational data including water elevations, currents, temperature, and salinity. Models were run in FORTRAN, and more detailed information about the model can be found in Feng et al. (2022).
The LPT model used in this study includes the modules for three-dimensional advection, vertical and horizontal turbulence and biological behavior (Feng et al., 2018). The particle tracking model was run offline using hourly data from the hydrodynamic model. The fourth-order Runge-Kutta scheme with a time step of 120 seconds was used to model 3-D particle displacement due to advection, while displacement due to vertical turbulence was modelled with a random walk that accounts for the gradient in vertical diffusivity (Visser, 1997). The time step used in the random walk was set to 5s. Hydrodynamic fields used in the model were from the year 2017.
All particles in the Minas Basin were released at 1 m below the sea surface at the spring high slack tide on two dates: 25 July and 24 August 2017 (Figure S2). Release timing was limited to high spring tides, when adult AMP distributed in the mid to high intertidal are completely submerged (Clark et al., 2019). All combinations of release date and behavior (passive and swimming) were run for 56 days total, ending on 18 September for the July simulations and 19 October for the August simulations (Table S1). In the Gulf of Maine simulations, particles were released at 1 m below the surface on 24 August 2017 at high slack tide and run for 56 days with swimming behaviour, ending on 19 October (Table S1). To investigate the potential for release timing to effect connectivity with the Bay of Fundy, Gulf of Maine particles were also released one hour before and one hour after this time.
Model outputs were analyzed to describe site and sub-regional connectivity in each simulation at two maximum planktonic larval durations (40 and 56 days). Site-to-site connectivity within the Minas Basin was estimated by enumerating the number of particles originating at each site that settled within each of the other site delineations (black circles shown on Figure 1) within an 8-day competency period (33-40 days, 49-56 days). The number of particles arriving in each suitable habitat site was then standardized to the total area of each settlement site (Table 1) to assess the number of particles settled per unit area (m2).
Table 1 Site number, name, and corresponding sub-region, as well as the GPS location (centroid latitude, centroid longitude) size (site area), and the number of particles seeded at each site.
The study area was also delineated to assess connectivity on a sub-regional scale based on knowledge of local bay dynamics. Within the Minas Basin, the intertidal zone was defined as model grid nodes that were dry at any point in time in August (colored polygons shown in Figure 1A), and this intertidal area was divided into 6 sub-regions based on cardinal direction and cross-shore features suspected to affect connectivity [e.g. Economy Point, Burntcoat Head (Figure 1A)]. Outside of the Minas Basin, the Bay of Fundy was delineated into inner, middle, and outer regions, while the Gulf of Maine was divided into northern, middle, and southern regions (Figure 1B). Regional delineations outside of the Minas Basin do not include any definition of suitable habitat or intertidal area. Site-to-region connectivity was assessed by enumerating the number of particles that originated at each site and subsequently arrived within a defined region (intertidal sub-regions within the Minas Basin, northern, middle, and southern Gulf of Maine, and inner, middle, and outer Bay of Fundy) by the end of each competency window. Within the Minas Basin (only), particles ending up in any area outside of defined sites (site to site connectivity analysis) or intertidal sub-regions (site to sub-region connectivity analysis) were designated as arriving in unsuitable habitat. Outside the Minas Basin, there was no designation of suitable or unsuitable habitat.
Site to site connectivity results were analyzed using generalized linear mixed effects models (GLMM). To investigate the relative importance of settlement sub-region and biological factors on settlement patterns across suitable habitat sites, the first model evaluated the effects of PLD (33-40 days, 49-56 days), spawning month (July, August), behavior (passive, swimming), settlement sub-region (NW, NE, S, SE and SW Minas 1 & 2), and the interaction between all pairs of factors on the total number of particles settling within suitable habitat sites within the Minas Basin in each simulation (sampling unit is simulation-site). The second model analyzed the contribution of sub-regions as sources of particles by including source sub-region instead of settlement sub-region in the same model, with all other factors included. Settlement site was included as a random factor in these models, while all other factors were considered fixed. To investigate the effects of biological factors on settlement patterns across regions within the Minas Basin, two generalized linear models (GLMs) were constructed. The first analyzes the effects of PLD (33-40 days, 49-56 days), spawning month (July or August), behavior (passive vs. swimming), settlement sub-region (NW, NE, S, SE and SW Minas 2), and the interaction between all pairs of factors on the total number of particles settling within intertidal area within the Minas Basin. The second regional model analyzed the contribution of sub-regions as sources of particles by including source sub-region instead of settlement sub-region in the same model, with all other factors included. Data are counts did not meet the assumptions of normality and homogeneity of variances, so the nb.glmer function in the nlme package in R (Pinheiro et al., 2020) was used to fit a negative binomial model to the site connectivity data and the glm.nb function from the MASS package in R was fit to the regional connectivity data. Statistical significance was assessed at alpha = 0.05.
In all simulations, regardless of spawning season, behavior and PLD, settlement was highest at sites within the SE Minas and S Minas regions, particularly sites 3, 8, 9, 13, 14, and 16 (Figures 2F–M, S3-S10, Table S3). Most of these particles originated from sites within the same two regions, SE and S Minas (i.e., sites 1, 2, 3, 8, 9, 13, 14, 16) (Figures 4F–M, S3-S10, Table S4). Total settlement at suitable habitat sites within all regions was higher in August compared to July, with a significant interaction between settlement region and spawning month driven by a higher settlement of particles within the S Minas region in August as compared to July (Figures 3A, B, Table 2, Table S3). Sites within the NW, NE and SW Minas 2 regions were larger sources and sinks of particles in August than July (Figures 2A–E, N–P, 3A–D, 4A–E, N–P, Tables 2A, B), but their importance overall as source and sink sites was low in both spawning months relative to the S and SE Minas regions (Figures 2F–M, 3A–D, 4F–M). The effects of behaviour and PLD on settlement were inconsistent across regions, as indicated by a significant interaction with settlement region in statistical models (Table 2), with some regions experiencing higher settlement with longer PLDs and when particles were swimming (Figure 2). In all simulations, most particles settled in unsuitable habitat in the Minas Basin (19-63%) or Inner Bay of Fundy (22-66%, Figure 5), but the percentage of particles retained within the Minas Basin (suitable or unsuitable habitat) was higher for particles exhibiting behavior (swimming: 40-76%, passive: 26-42%) during simulations in both spawning months and at both PLDs (Figure 5).
Figure 2 The number of particles settled at each site (see sites 1-16, Figure 1) in the Minas Basin (A–P) in each simulation. Bar colours show the relative proportion from each source sub-region, and each panel has a colored horizontal line to indicate settlement sub-region. Percentages above each bar indicate the total percent of particles settling at each site in each simulation. Simulations with passive particles have gray shaded backgrounds while simulations with swimming particles have white shaded backgrounds.
Figure 3 (A, B) The mean number of particles settled at suitable habitat sites within each sub-region for the two spawning months, and (C, D) the mean number of particles settling at suitable habitat sites from each source sub-region in the two simulation months. Means are calculated from all simulations in each month to show that the effects of spawning month and source/sink subregions are relatively consistent across simulations. Bars show means +/- standard error.
Figure 4 The number of particles from each source site that settled at suitable habitat sites (A–P) in each simulation. Bar colours show the proportion in each settlement sub-region, and each panel has a colored horizontal line to indicate source sub-region. Percentages above each bar indicate the total percent of particles settling at each site in each simulation. Simulations with passive particles have gray shaded backgrounds while simulations with swimming particles have white shaded backgrounds.
Table 2 Results of generalized linear mixed effects models (GLMM) examining factors affecting settlement at suitable habitat sites within the Minas Basin.
Figure 5 The proportion of total particles in each simulation settling at suitable habitat sites, colored by sub-region within the Minas Basin, as well as unsuitable habitat within the Minas Basin, and regions in the Bay of Fundy and Gulf of Maine.
After standardizing the number of particles settled at each site by the site area, site 16 (S Minas region) received the highest or second highest density of particles of all sites in nearly all simulations (except for July passive, both PLDs) (Figure 6). Select sites within the NW (i.e., sites 6, 11), SW Minas 2 (i.e., 5, 7), and NE Minas Basin (i.e., 15) regions also received relatively high numbers of particles per unit area. These patterns were most prominent in the August simulations (Figure 6).
Figure 6 The number of particles settled at each site in each simulation, standardized by the settlement site area (shown in Figure 1). Bars are colored by the settlement sub-region, and results from July simulations are in the top row (A–D), while results from August simulations are in the bottom row (E–H).
On a sub-regional scale, settlement dynamics were significantly different between July and August (Figures 7, 8, Table 3), though a significant interaction between spawning month and source/settlement sub-region indicates that seasonal patterns varied by sub-region. The majority of particles modelled in July simulations ended up in unsuitable habitat either in the central Minas Basin (12.9-50%) or the Inner Bay of Fundy (29.4-61.6%) (Figures 7G–J, 8, 9A, S11). In July, the highest settlement (p<0.05) in intertidal sub-regions within the Minas Basin (not just at strictly defined suitable habitat sites) occurred in the NW (3.8-8.0% of the total number of particles), SE (2.4-5.8%), and SW Minas 2 (2.3-3.6%) regions (Table S5), though most particles did not settle in suitable habitat sites, particularly for the NW and NE Minas sub-regions (Figures 7A–C, F, 8A–D). Most of the particles arriving in intertidal sub-regions within the Minas Basin originated from sites within the SE Minas sub-region (blue bars, Figure S11, statistical significance in Table S6), and to a lesser extent the S Minas sub-regions (yellow bars, Figure S11, statistical significance in Table S6). Sub-regional patterns were largely similar regardless of behavior and PLD, though there were slightly more particles retained within unsuitable habitat in the Minas Basin and arriving in the S Minas basin sub-region when they were given swimming behavior (26.7-50.0%) vs. passive dispersal (12.9-26.2%), indicated by a statistically significant interaction between behavior and settlement sub-region in statistical models (Figures 7G, 8, S11, Table 3, Tables S5, S6). The effects of PLD on regional settlement dynamics was inconsistent, and this factor interacted with settlement sub-region in statistical models (Figure 7, Table 3).
Figure 7 The number of particles settled by region. Within the Minas Basin, sub-regions represent all intertidal area (not just strictly defined sites), while outside of the Minas Basin, regions include all intertidal and subtidal area. Bar colours represent settlement sub-region, and percentages show the percent of total particles released that settled within that sub-region. Also shown are particles settled in unsuitable habitat in the Minas Basin (G). Panels A-F denote settlement sub-region within the Minas Basin, panels H-J denote settlement within the Bay of Fundy, and K-M denote settlement within the Gulf of Maine. Simulations with passive particles have gray shaded backgrounds while simulations with swimming particles have white shaded backgrounds.
Figure 8 Density plot showing the number of particles settling in 0.005 x 0.005 degree bins within the Minas Basin for the July (A–D) and August (E–H) swimming simulations at PLDs of 40 (A, B, E, F) and 56 (C, D, G, H) days. Yellow colors indicate higher particle numbers, and blue colors indicate lower particle numbers. Note, the total number of particles in some cells was higher than 25, but the scale was capped at this value to show spatial variation more clearly in particle densities (data also shown in Figure 7).
Table 3 Results of generalized linear models (GLMs) examining factors affecting settlement within sub-regions of the Minas Basin.
Figure 9 Results showing examples of connectivity between Minas Basin and the Gulf of Maine. (A) shows the location of particles released from sites within the NW Minas sub-region after 56 days when released in July and given swimming behavior. (B) shows the final locations of particles released in a 1 km width band along the coast of the Gulf of Maine in August 24, 2017 at 17:00 and given swimming behaviour. The green circle on the right panel shows the final location of the only particle that entered the Bay of Fundy from the Gulf of Maine.
This contrasted with sub-regional settlement dynamics in August, in which settlement within intertidal sub-regions within the Minas Basin was higher overall than in July (Figures 7A–F, 8, S12). Also, in August there was a clear trend towards select southern regions within the Minas Basin receiving considerably more particles (i.e., S: 11.6-30.1%, SW Minas 2: 7.8-12.2%) than northern regions (NW: 0.7-4.9%, NE: 2.2-6.2%) (Figures 7A–F, 8, S12, Table S5). The S (yellow bars, Figure S12, Table S6) and SE Minas (blue bars, Figure S12, Table S6) sub-regions contributed the most particles to suitable habitat sites, as compared to sites in other sub-regions. Behavior had a more prominent effect on sub-regional connectivity dynamics in August than July, as indicated by a significant interaction between these two factors (Table 3) with more particles being retained within the Minas Basin when particles were given swimming behavior versus when they dispersed passively (passive: 36-45.1%, swimming: 65.9-77.6%) (Figures 7A–F, 8, S12, Table S5). The majority of the swimming particles in August simulations were retained in unsuitable habitat within the central Minas Basin (15.4-28.8%), or the S Minas Basin (24.8-30.2%), as compared to passive particles in August, which were mainly exported to the Inner Bay of Fundy (50.1-52.7%) or retained within the NW (4.6-4.9%), NE (4.8-6.2%), SE (1.5-2.6%), and SW Minas 1 (0.5-0.9%) or 2 sub-regions (7.8-10.0%, Figures 7A–M, 8, S11, Table S5).
Only particles that were assigned swimming behavior dispersed into the Gulf of Maine (Figure 7). The northern region of the Gulf of Maine received the most particles at a PLD of 49-55 days (July: 337 particles, 0.7%; August: 279 particles, 0.6%) followed by the middle region (July: 47 particles, 0.1%; August: 16 particles, 0.03%), while no particles dispersed to the southern Gulf of Maine (Figures 7, 9A). Fewer particles dispersed into the northern (July: 27 particles, 0.1%, August: 5 particles, 0.01%) and middle regions (July: 0 particles, 0%, August, 3 particles, 0.01%) of the Gulf of Maine at the shorter PLD of 33-40 days.
Particles seeded in the Gulf of Maine generally moved southward with shoreward retention (Figure 9B). There were no particles initialized in the Gulf of Maine that reached the Minas Basin, and effectively no particles (i.e., only one out of 7080 total particles) released entered the southernmost part of the outer Bay of Fundy (Table S2, Figure 9). This particle originated from the northernmost part of the seeded 1.0 km band, while none of particles seeded closer to shore (i.e., 0.5 km band) reached the Bay of Fundy (Table S2). Release timing effected this connectivity somewhat, with the only instance in which particles seeded in the 1 km band reached the Bay of Fundy being when they were release at one hour past slack tide (19:00, Table S2)
In this study, we used LPT modelling to quantify source/sink dynamics among extant sites for the AMP in the Minas Basin, including evaluating how connectivity was affected by variations in spawning seasonality, behavior and planktonic larval duration (PLD). Our results show that sites along the southern coastline of the Minas Basin (S and SE sub-regions) serve as the most important sources and sinks of particles (e.g., Figure 3). This finding was consistent across simulations, regardless of variations in spawning month, behavior, and PLD. Prior field surveys highlighted that the S and SE Minas sub-regions have the highest concentration and most extensive sub-populations of AMP, and largest suitable habitat area in the Minas Basin (Clark et al., 2019). These sub-populations have also remained relatively stable through time as compared to sites in other sub-regions of the Minas Basin, such as Economy Point and Parrsboro in the NW Minas sub-region (Clark et al., 2019). Given the high sub-population density, large cumulative sub-population area, relatively high habitat stability, and strong role of sites in the region as sources and sinks of particles, our results combined with existing field data provide evidence that sites along the S and SE coast of the Minas Basin should be prioritized for protection against coastal development activities that may disturb this critical habitat.
Sub-regional connectivity modelling showed that the NE and NW Minas sub-regions also receive a consistent larval supply from the S and SE Minas sub-regions (Figure 2, S11, 12), and when the number of particles settling in these sub-regions is standardized per unit area, settlement is among the highest of any sub-region within the Minas Basin (Figure 6). Field studies have noted that red mudstone habitat area along the northern coastline of the Minas Basin is relatively small and unstable through time with several AMP sub-populations in that sub-region having been extirpated over the past couple decades due to an increase in the presence of fine silt and sediment (Clark et al., 2019). This suggests that the relatively small sub-populations in this area may be the result of limited suitable habitat, rather than larval supply. However, this also suggests that if there were an increase in suitable habitat in the area due to changes in sedimentation and scouring dynamics, larval supply may promote sub-population establishment and growth.
Though there was a high degree of consistency across simulations in the relative importance of sites as sources and sinks of particles, the overall magnitude of settlement at suitable habitat sites was significantly higher in August as compared to July (Figure 5). Most particles retained within the Minas Basin in July simulations ended up in unsuitable habitat in the central basin and Minas Passage, whereas more particles were transported shoreward to southern sub-regions (e.g., S and SW Minas 2) in August. Monthly changes in circulation patterns are driven by variation in atmospheric conditions, particularly rainfall, winds, temperature, and stochastic events (e.g. storms) (Chen et al., 2006). Our results suggest that even within-season (e.g. summer; July vs. Aug) variation in resulting circulation patterns was sufficient to significantly affect settlement dynamics of AMP. The magnitude of variability due to inter-annual changes in atmospheric forcing conditions is not assessed in this work, which employed 2017 conditions to drive model hydrodynamics. The relatively high sensitivity of our modelling results to monthly changes in hydrodynamic conditions suggests that the impacts of environmental variability on connectivity is an important consideration for future study, including the effects of inter-annual variability and extreme (stochastic) events.
Larval swimming behavior and PLD also affected transport and settlement patterns of AMP to some degree within the Minas Basin, but this effect was inconsistent across sub-regions and spawning months as indicated by statistically significant interactions between behavior, PLD, settlement sub-region, and spawning month. Swimming had a stronger effect on sub-regional connectivity dynamics, with more particles reaching the outer Bay of Fundy and the Gulf of Maine when they were assigned swimming behavior than when they were dispersing passively (Figure 7). Larval swimming speeds are generally comparable to or greater than vertical transport processes (Queiroga and Blanton, 2005). However, high tides in the Minas Basin cause turbulent velocities in the vertical dimension that exceed larval swimming speeds, causing larvae to mix throughout the water column in those sub-regions (Figure S13A). This likely contributed to the inconsistent effect of larval swimming behavior on connectivity within the Minas Basin. By contrast, swimming particles in the Bay of Fundy maintained a noticeably shallower position in the water column (associated with their preferred depth being the mixed layer depth) than particles that dispersed passively (i.e. sinking only), which tended to be distributed throughout or concentrated in bottom layers of the water column due to gravitational sinking (Figure S13B). As a result, swimming particles were transported farther southwestward down the coasts of New Brunswick and Maine than in simulations where particles were sinking only (Figure S13) due to higher mean currents in a southwestward direction near the surface (e.g. Greenberg, 1984) as compared to considerably weaker currents at depth (Figure S14). This is evidence that implemented vertical swimming behavior at swimming velocities realistic to bivalve larvae (Chia et al., 1984) can have a dramatic effect on lateral transport dynamics where turbulent processes are minimal and as horizontal currents vary with depth (Tremblay and Sinclair, 1990; DiBacco et al., 2001; Queiroga and Blanton, 2004; DiBacco and Therriault, 2015).
Across all simulations, most particles (~90-95%) ended up in unsuitable habitat within the Minas Basin or in the Inner Bay of Fundy (Figures 5, 7). No AMP populations have been identified in the Bay of Fundy despite our modelling results that suggest many coastal areas in this region likely receive a high supply of AMP larvae. Despite the lack of mudstone substrate in the Bay of Fundy, the fact that AMP are known to establish in other habitat types (e.g. muds and peats) (COSEWIC, 2009) suggests that larval supply from the Minas Basin could support population establishment in the Inner Bay of Fundy in these substrates (Greenlaw et al., 2012). Our modelling results suggest that future work to characterize suitable AMP substrate types could help establish currently unidentified sub-populations and monitor for the establishment of new populations along the Inner Bay of Fundy.
The US coast of the Gulf of Maine hosts the closest anecdotal populations of the AMP, but these populations are separated by ~350 km from those in the Minas Basin (COSEWIC, 2009). It is currently unknown whether populations in these regions are connected via larval dispersal. Our simulations suggest that there is the potential for larvae released in the Minas Basin to reach the Northern and even the middle coast of Maine. Particles arriving in the Gulf of Maine (295 and 385 total in July and August) originated at all sites within the Minas Basin, but larger sites located in the NW Minas sub-region and closest to the entrance of the Minas Basin (Economy, site 4; Parrsboro, site 10) contributed the most particles (20% and 49% of the particles reaching the Gulf of Maine in July and August). The opposite was not true since particles originating at sites within the Gulf of Maine did not disperse to the Minas Basin. There was only one particle originating at the northern region of the release bands with a width of 1.0 km from shore that dispersed to the western-most region of the Outer Bay of Fundy. This is consistent with regional circulation characterized by surface flow to the southwest along the northern coasts of the Bay of Fundy and Gulf of Maine (Greenberg, 1984). The fact that particles concentrated and released closer to shore (i.e., 0.5 km release band) did not supply any particles to the Bay of Fundy provides additional evidence that AMP larvae released from intertidal areas are unlikely to disperse northwards to the Bay of Fundy or the Minas Basin. Overall, these results indicate some potential for sub-populations within the Minas Basin to supply larvae to the Gulf of Maine, but no connectivity in the opposite direction. Therefore, it is likely that the Minas Basin metapopulation is self-sustaining, further supporting the need for the protection of critical habitat and especially predominant source/sink sites along the southern coastline of the Minas Basin.
Overall, our results demonstrate how outputs from Lagrangian Particle Tracking (LPT) can directly inform conservation planning by elucidating sub-population source/sink dynamics, even for a species with sparse demographic and life history data. Our model was parameterized using the best available information for AMP, including the locations and area of substrate colonized by AMP sub-populations, as well as information for related taxa when it was not available for AMP. We designed our simulations to consider the greatest sources of uncertainty for AMP, which included the spawning seasonality, larval behavior and development time, and compared simulations to describe common patterns in source-sink dynamics. Our simulations consistently pointed to sites along the southern coastline of the Minas Basin as the most highly connected to other sites and sub-regions, both supplying the most particles to suitable habitat within the Minas Basin and receiving the highest number particles across simulations. This is supported by field surveys, which identified the largest and most persistent AMP sub-populations in these areas (Clark et al., 2019). This suggests that sites along the southern coastline likely play any important role in contributing to metapopulation sustainability and should be a priority for spatial conservation measures.
Model outputs also highlight the need for future studies, in particular sensitivity analyses for model parameters that contributed the most to variability in connectivity estimates and dynamics. Of the parameters we considered, settlement dynamics varied most strongly between simulation months, with significantly higher settlement in suitable habitat in August as compared to July. This highlights the sensitivity of our model results to variability in environmental conditions, and points to the need to characterize potential impacts of intra- and interannual variability, extreme events, and climate change on AMP dispersal dynamics. This is particularly important since our model results employed hypodynamic conditions for a single year (2017). Running our model for additional months and years, including climate-based projections, would help to assess the sensitivity of source/sink dynamics to these different parameters. Further, while our results indicate a low potential for connectivity of AMP in the direction of the Gulf of Maine to the Minas Basin, extreme events could alter these dispersal dynamics and effect our conclusions about potential connectivity with Gulf of Maine populations. Nevertheless, prior studies show the same generalized flow dynamics in the Bay of Fundy and Gulf of Maine (Greenberg, 1984; Brickman 2014), with the mouth of the Bay of Fundy representing a well-known biogeographic barrier characterized by limited connectivity from the Gulf of Maine to the Bay of Fundy (Einfeldt et al., 2014; 2017). In addition to the long-term effects of climate change on circulation patterns, the increased frequency and intensity of storms (Knutson et al., 2015) is likely to influence the availability and suitability of habitat within the Minas Basin through changes in sedimentation, which can be modelled and examined in relation to source/sink dynamics (Clark et al., 2019).
Simulation results can also be used to direct further study into the distribution and biology of AMP. In particular, they can be used to inform future efforts to delineate populations that may exist, but are currently unknown, such as in regions of the Inner Bay of Fundy that received a high larval supply or the areas west of Tennycape (site 16) along the southern coastline of the Minas Basin. Our simulation results can also be used to prioritize resources for monitoring areas of the coastline for AMP sub-populations where the amount of suitable habitat may change in the future, for example the NW Minas sub-region where larval supply is relatively high, but sub-population abundance is low due to a lack of suitable habitat. The sensitivity of our modelling results to the seasonal timing of particle release also points to the need for further study into the seasonality of AMP spawning, including the timing of larval release with respect to the tidal cycle and number of spawns per year. Also, better characterizing aspects of their larval behaviour and development during dispersal, including swimming speeds, the response of larvae to environmental cues (e.g. vertical density gradients/mixed layer depth), and environmental effects (e.g., temperature) on larval development rates will improve predictions of source/sink dynamics within the Minas Basin, and further resolve potential linkages between the Minas Basin and Gulf of Maine populations, as particles only dispersed into the Gulf of Maine when they were assigned swimming behavior. It is also important to note that only 50,000 particles were used per simulation due to computational limitations, which is a significant underrepresentation of the actual number of larvae released naturally. True metapopulation connectivity could be established by including data on sub-population density, fecundity at size, larval mortality, and the number of spawns per year into our model, if they become available, and these data would help to elucidate the role of recruitment dynamics in driving the lack of AMP at sites that were extirpated or with small sub-populations (Clark et al., 2019). Genetic analyses could also help to elucidate regional connectivity dynamics between the Minas Basin and Gulf of Maine, which are important to resolve given their implications for the regional persistence of the species.
Our results also help to highlight the other anthropogenic factors that are likely to most affect AMP in the future, particularly those activities that alter circulation dynamics within the Minas Basin. Tidal energy development is expected to significantly alter the magnitude and direction of advective currents (Neill et al., 2009; Wu et al., 2015), which clearly has the potential to impact source/sink dynamics of AMP, as well as the availability of suitable habitat for the species through changes in tidal ranges and sedimentation dynamics (Neill et al., 2009). Source/sink dynamics will also change in response to losses and gains of suitable habitat due to dredging and coastal development, as sub-populations disappear and become established in different areas of the Minas Basin. In particular, activities that threaten sites along the southern coastline of the Minas Basin should be carefully monitored and managed, as they have the greatest potential to impact the stability of the entire metapopulation in the short and long term.
In general, our results provide a useful example of how maintaining metapopulation sustainability can promote the persistence of a species (Hanski and Gilpin, 1997; Cowen et al., 2006; Treml et al., 2008). Suitable habitat is highly dynamic within the Minas Basin by nature of strong tidal flows, with some areas experiencing more frequent disturbances that can cause local species extinctions (Clark et al., 2019). Our results suggest that sub-populations along the southern coastline of the Minas Basin, which have remained relatively stable through time, are likely responsible for enabling sub-population recovery in areas where the availability of suitable habitat is more variable (e.g. northern coastline of the Minas Basin). Moreover, our result show that sub-populations within the Minas Basin may supply larvae to the Gulf of Maine, with the potential for sub-populations in the Minas Basin to mitigate the effects of disturbance on Gulf of Maine populations. It is evident in this case how conservation measures may fall short of promoting species persistence within the Minas Basin and over the broader region if they fail to account for these connectivity dynamics and prioritize key source-sink sub-populations for protection. Though our results would undoubtedly be improved by refinements to model parameters, including estimates of sub-population size, fecundity, and larval mortality, they are immediately useful for designing spatial management measures, and for identifying the most pertinent areas for future research into this poorly understood and threatened marine species.
The raw data supporting the conclusions of this article will be made available by the authors, without undue reservation.
KK conducted the data analysis, led the data interpretation and writing, co-led the experimental and data analysis design and development of study objectives, and provided input on the particle track modelling. WG, YW, and CD co-led the development of the study objectives, experimental and data analysis design, particle track modelling, and provided input on the data interpretation, analysis, and writing. XW conducted the particle track modelling and provided input on the experimental design and writing. All authors contributed to the article and approved the submitted version.
Financial support provided by the Fisheries and Oceans Species At Risk Program to CDB and YW.
We thank Tao Feng for help in the implementation of the particle tracking model and providing FVCOM model results. We also thank P. Crowell at the DFO Species at Risk Program for providing input on the study objectives and design, and data interpretation.
The authors declare that the research was conducted in the absence of any commercial or financial relationships that could be construed as a potential conflict of interest.
All claims expressed in this article are solely those of the authors and do not necessarily represent those of their affiliated organizations, or those of the publisher, the editors and the reviewers. Any product that may be evaluated in this article, or claim that may be made by its manufacturer, is not guaranteed or endorsed by the publisher.
The Supplementary Material for this article can be found online at: https://www.frontiersin.org/articles/10.3389/fmars.2022.926442/full#supplementary-material
Botsford L. W., Hastings A., Gaines S. G. (2001). Dependence of sustainability on the configuration of marine reserves and larval dispersal distance. Ecol. Lett. 4, 144–150. doi: 10.1046/j.1461-0248.2001.00208.x
Botsford L. W., White J. W., Coffroth M.-A., Paris C. B., Planes S., Shearer T. L., et al. (2009). Connectivity and resilience of coral reef metapopulations in marine protected areas: matching empirical efforts to predictive needs. Coral Reefs 28, 327–337. doi: 10.1007/s00338-009-0466-z
Brickman D. (2014). Could ocean currents be responsible for the west to east spread of aquatic invasive species in Maritime Canadian waters? Mar Poll Bull 85, 235–43.
Brown J. H., Kodric-Brown A. (1977). Turnover rates in insular biogeography: effect of immigration on extinction. Ecology 58, 445–449. doi: 10.2307/1935620
Burgess S. C., Nickols K. J., Griesemer C. D., Barnett L. A. K., Dedrick A. G., Satterhwaite E. V., et al. (2014). Beyond connectivity: how empirical methods can quantify population persistence to improve marine protected area design. Ecol. Apps. 24, 257–270. doi: 10.1890/13-0710.1
Chanley P. E. (1965). Larval development of a boring clam, Barnea truncata. Chesapeake Sci. 63, 162–166. doi: 10.2307/1350848
Chen C., Beardsley R. C., Cowles G. (2006). An unstructured grid, finite-volume coastal ocean model (FVCOM) system. Oceanography 19, 78–89. doi: 10.5670/oceanog.2006.92
Chen C., Liu H., Beardsley R. C. (2003). An unstructured grid, finite-volume, three-dimensional, primitive equations ocean model: Application to coastal ocean and estuaries. J. Atmos. Ocean. Technol. 20, 159–186. doi: 10.1175/1520-0426(2003)020<0159:AUGFVT>2.0.CO;2
Chia F.-S., Buckland-Nicks J., Young C. M. (1984). Locomotion of marine invertebrate larvae. Can. J. Zoo 62, 1205. doi: 10.1139/z84-176
Clark C. M., Hebda A., Jones G., Butler S., Pardy G. (2019). Identification of Atlantic mud-piddock habitat in Canadian waters. DFO Can. Tech. Rep. Fish. Aquat. Sci. 3295, iv + 42. doi: 10.1139/z84-176
COSEWIC (2009). COSEWIC assessment and status report on the Atlantic mud-piddock (Barnea truncata) in canada. committee on the status of endangered wildlife in Canada. Ottawa, vii + 42.
Cowen R. K., Paris C. B., Srinivasan A. (2006). Scaling of connectivity in marine populations. Science 311, 522–527. doi: 10.1126/science.1122039
Cowen R. K., Sponagule S. (2009). Larval dispersal and marine population connectivity. Ann. Rev. Mar. Sci. 1, 443–466. doi: 10.1146/annurev.marine.010908.163757
Crowder L. B., Lyman S. J., Figueira W. F., Priddy J. (2000). Source-sink population dynamics and the problem of siting marine reserves. Bull. Mar. Sci. 66, 799–820.
Davies K. T. A., Gentleman W. C., DiBacco C., Johnson C. L. (2014). Fisheries closed areas strengthen scallop larval settlement and connectivity among closed areas and across international open fishing grounds: A model study. Environ. Manage. 56, 587–602. doi: 10.1007/s00267-015-0526-9
DFO (2010). Recovery potential assessment for Atlantic mud-piddock (Barnea truncata). DFO can. Sci. Advis. Sec. Sci. Advis. Rep. 2010/068.
DiBacco C., Levin L. A., Sala E. (2006). “Connectivity in marine ecosystems: the importance of larval and spore dispersal,” in Connectivity conservation. Eds. Crooks K., Sanjayan M. (Cambridge University Press).
DiBacco C., Sutton D., McConnico L. (2001). Vertical migration behavior and horizontal distribution of brachyuran larvae in a low inflow estuary: Implications for bay-ocean exchange. Mar. Ecol. Prog. Ser. 217, 191–206. doi: 10.3354/meps217191
DiBacco C., Therriault T. W. (2015). Reproductive periodicity and larval vertical migration behaviour of European green crab (Carcinus maenas) in a non-native habitat. Mar. Ecol. Prog. Ser. 536, 123–134. doi: 10.3354/meps11422
Einfeldt A. L., Doucet J. R., Addison J. A. (2014). Phylogeography and cryptic introduction of the ragworm Hediste diversicolor (Annelida, Nereididae) in the Northwest Atlantic. Invertebrate Biology 133, 232–241. doi: 10.1111/ivb.12060
Einfeldt A. L., Zhou F., Addison J. A. (2017). Genetic discontinuity in two high dispersal marine invertebrates in the northwest Atlantic. FACETS 2, 160–177. doi: 10.1139/facets-2016-0044
Feng T., Stanley R. R., Wu Y., Kenchington E., Xu J., Horne E. (2022). A high-resolution 3-d circulation model in a complex archipelago on the coastal scotian shelf. J. Geophys. Res. Oceans, e2021JC017791. doi: 10.1029/2021JC017791
Feng T., Wang C., Wang P., Qian J., Wang X. (2018). How physiological and physical processes contribute to the phenology of cyanobacterial blooms in large shallow lakes: A new Euler-Lagrangian coupled model. Water Res. 140, 34–43. doi: 10.1016/j.watres.2018.04.018
Frank B. (2009) Barnea truncata (Say 1822) Atlantic mud-piddock. Jacksonville shell club. Available at: http://www.jaxshells.org/btrun.htm (Accessed March 2009).
Gaines S. D., Gaylord B., Largier J. L. (2003). Avoiding current oversights in marine reserve design. Ecol. Apps. 13, S32–S46. doi: 10.1890/1051-0761(2003)013[0032:ACOIMR]2.0.CO;2
Gilroy J. J., Edwards D. P. (2017). Source-sink dynamics: a neglected problem for landscape-scale biodiversity conservation in the tropics. Curr. Landscape Ecol. Rep. 2, 51–60. doi: 10.1007/s40823-017-0023-3
Greenberg D. (1984). A review of the physical oceanography of the bay of fundy. Can. Tech. Rep. Fish. Aquat. Sci. 1256, 9.
Greenlaw M. E., Gromack A. G., Basquill S. P., MacKinnon D. S., Lynds J. A., Taylor R. B., et al. (2012). A physiographic coastline classification of the scotian shelf bioregion and environs: The Nova Scotia coastline and the new Brunswick fundy shore. DFO Can. Sci. Advis. Sec. Res. Doc., iv + 39. 2012/051.
Haase A. T., Eggleston D. B., Luettich R. A., Weaver R. J., Puckett B. J. (2012). Estuarine circulation and predicted oyster larval dispersal among a network of reserves. Estuar. Coast. Shelf Sci. 101, 33–43. doi: 10.1016/j.ecss.2012.02.011
Hanski I., Gilpin M. E. (Eds.) (1997). Metapopulation biology: Ecology, genetics, and evolution (San Diego, CA: Academic Press).
Heinrichs J. A., Lawler J. J., Schumaker N. H., Wilsey C. B., Monroe K. C., Aldridge A. L. (2018). A multispecies test of source-sink indicators to prioritize habitat for declining populations. Conserve. Biol. 32, 648–659. doi: 10.1111/cobi.13058
Holt R. D. (1985). Population dynamics in two-patch environments: some anomalous consequences of an optimal habitat distribution. Theoret. Pop. Biol. 28, 181–208. doi: 10.1016/0040-5809(85)90027-9
Jean-Michel L., Eric G., Romain B. B., Gilles G., Angélique M., Marie D., et al. (2021). The Copernicus global 1/12° oceanic and sea ice GLORYS12 reanalysis. Front. Earth Sci. 9, 585. doi: 10.3389/feart.2021.698876
Knutson T. R., Sirutis J. J., Zhao M., Tuleya R. E., Bender M., Vecchi G. A., et al. (2015). Global projections of intense tropical cyclone activity for the late twenty-first century from dynamical downscaling of CMIP5/RCP4.5 scenarios. J. Climate 28, 7203–7224. doi: 10.1175/JCLI-D-15-0129.1
Lellouche J.-M., Le Galloudec O., Greiner E., Garric G., Regnier C., Drevillon M., et al. (2018). The Copernicus marine environment monitoring service global ocean 1/12 • physical reanalysis GLORYS12V1: description and quality assessment. Geophys. Res. Abs. 20, 2018–19806.
Lowe W. H., Allendorf F. W. (2010). What can genetics tell us about population connectivity? Mol. Ecol. 19, 3038–3051. doi: 10.1111/j.1365-294X.2010.04688.x
Lyard F. H., Carrere L., Cancet M., Boy J. P., Gegout P., Lemoine J. M. (2016). The FES2014 tidal atlas, accuracy assessment for satellite altimetry and other geophysical applications (Vienna, Austria, Eur. Geosci. Union: EGU General Assembly), 17693.
Mann R., Campos B. M., Luckenbach M. W. (1991). Swimming rate and responses of larvae of three mactrid bivalves to salinity discontinuities. Mar. Ecol. Prog. Ser. 68, 257–269. doi: 10.3354/meps068257
Neill S. P., Litt E. J., Couch S. J., Davies A. G. (2009). The impact of tidal stream turbines on large-scale sediment dynamics. Renew. Energy 34, 2803–2812. doi: 10.1016/j.renene.2009.06.015
North E. W., Schlag Z., Hood R. R., Li M., Zhong L., Gross T., et al. (2008). Vertical swimming behavior influences the dispersal of simulated oyster larvae in a coupled particle-tracking and hydrodynamic model of chesapeak bay. Mar. Ecol. Prog. Ser. 359, 99–115. doi: 10.3354/meps07317
Peery M. Z., Beissinger S. R., House R. F., Bérubé M., Hall L. A., Sellas A., et al. (2008). Characterizing source–sink dynamics with genetic parentage assignments. Ecology. 89, 2746–2759. doi: 10.1890/07-2026.1
Pinheiro J., Bates D., DebRoy S., Sarkar D., R Core Team (2020). Nlme: Linear and nonlinear mixed effects models_ (R package version 3.1-148). Available at: https://CRAN.R-project.org/package=nlme.
Pujolar J. M., Schiavina M., Di Franco A., Melia P., Guidetti P., Gatto M., et al. (2013). Understanding the effectiveness of marine protected areas using genetic connectivity patterns and Lagrangian simulations. Div. Distribr. 19, 1531–1542. doi: 10.1111/ddi.12114
Pulliam R. H. (1988). Sources, sinks, and population regulation. Am. Nat. 132, 652–661. doi: 10.1086/284880
Queiroga H., Blanton J. (2004). Interactions between behaviour and physical forcing in the control of horizontal transport of decapod crustacean larvae. Adv. in Mar. Biol. 47, 109–138.
Queiroga H., Blanton J. (2005). Interactions between behavior and physical forcing in the control of horizontal transport of decapod crustacean larvae. Adv. Mar. Biol. 48, 107–214. doi: 10.1016/S0065-2881(04)47002-3
Runge J. P., Runge M. C., Nichols J. D. (2006). The role of local populations within a landscape context: defining and classifying sources and sinks. Am. Nat. 167, 925–938. doi: 10.1086/503531
Samways M. J., Barton B. S., Birkhofer K., Chichorro G., Deacon C., Fartmann T., et al. (2020). Solutions for humanity on how to conserve insects. Biol. Conserv. 242, 108427. doi: 10.1016/j.biocon.2020.108427
Shanks A. L. (2018). Testing the intermittent upwelling hypothesis: upwelling, downwelling, and subsidies to the intertidal zone. Ecol. Monogr. 88, 22–35. doi: 10.1002/ecm.1281
Sponagule A., Paris C., Walter K. D., Kourafalou V., Alessandro E. D. (2012). Observed and modeled larval settlement of a reef fish to the Florida keys. Mar. Ecol. Prog. Ser. 453, 201–212. doi: 10.3354/meps09641
Storfer A., Murphy M. A., Evans J. S., Goldberg C. S., Robinson S., Spear S. F., et al. (2006). Putting the ‘landscape’ in landscape genetics. Heredity. 98, 128–142. doi: 10.1038/sj.hdy.6800917
Talmage S. C., Gobler C. J. (2011). Effects of elevated temperature and carbon dioxide on the growth and survival of larvae and juveniles of three species of northwest Atlantic bivalves. PloS One 6, e26941. doi: 10.1371/journal.pone.0026941
Tremblay M. J., Sinclair M. (1990). Sea Scallop larvae placopecten magellanicus on georges bank: vertical distribution in relation to water column stratification and food. Mar. Ecol. Prog. Ser. 61, 1–15. doi: 10.3354/meps061001
Treml E., Halpin P., Urban D., Pratson L. (2008). Modeling population connectivity by ocean currents, a graph-theoretic approach for marine conservation. Landscape Ecol. 23, 19–36. doi: 10.1007/s10980-007-9138-y
van Sebille A., Griffies S. M., Abernathey R., Adams T. P., Berloff P., Biastoch A., et al. (2018). Lagrangian Ocean analysis: Fundamentals and practices. Ocean Model. 121, 49–75. doi: 10.1016/j.ocemod.2017.11.008
Visser A. (1997). Using random walk models to simulate the vertical distribution of particles in a turbulent water column. Mar. Ecol. Prog. Ser. 158, 275–281. doi: 10.3354/meps158275
Widdows J. (1991). Physiological ecology of mussel larvae. Aquaculture 94, 147–163. doi: 10.1016/0044-8486(91)90115-N
Keywords: atlantic mud piddock, minas basin, connectivity, particle tracking, larval dispersal, bivalve, conservation, species at risk
Citation: Krumhansl KA, Gentleman WC, Wu Y, Wang X and DiBacco C (2022) Using larval connectivity to inform conservation management of the endemic and threatened Atlantic mud-piddock (Barnea truncata) in the Minas Basin Canada. Front. Mar. Sci. 9:926442. doi: 10.3389/fmars.2022.926442
Received: 22 April 2022; Accepted: 05 July 2022;
Published: 04 August 2022.
Edited by:
Piers Dunstan, Oceans and Atmosphere Commonwealth Scientific and Industrial Research Organisation (CSIRO), AustraliaReviewed by:
Fábio L. Matos, University of Aveiro, PortugalCopyright © 2022 Krumhansl, Gentleman, Wu, Wang and DiBacco. This is an open-access article distributed under the terms of the Creative Commons Attribution License (CC BY). The use, distribution or reproduction in other forums is permitted, provided the original author(s) and the copyright owner(s) are credited and that the original publication in this journal is cited, in accordance with accepted academic practice. No use, distribution or reproduction is permitted which does not comply with these terms.
*Correspondence: Kira A. Krumhansl, S2lyYS5LcnVtaGFuc2xAZGZvLW1wby5nYy5jYQ==
Disclaimer: All claims expressed in this article are solely those of the authors and do not necessarily represent those of their affiliated organizations, or those of the publisher, the editors and the reviewers. Any product that may be evaluated in this article or claim that may be made by its manufacturer is not guaranteed or endorsed by the publisher.
Research integrity at Frontiers
Learn more about the work of our research integrity team to safeguard the quality of each article we publish.