- 1Centre for Environment Fisheries and Aquaculture Science, Weymouth, United Kingdom
- 2Centre for Environment Fisheries and Aquaculture Science, Lowestoft, United Kingdom
- 3School of Geography and Sustainable Development, University of St Andrews, St Andrews, United Kingdom
- 4School of Life Sciences, University of Essex, Colchester, United Kingdom
- 5Centre for Ocean and Atmospheric Sciences, School of Environmental Sciences, Univeristy of East Anglia, Norwich, United Kingdom
- 6School of Geography, University of Leeds, Leeds, United Kingdom
Continental shelf sediments store large amounts of organic carbon. Protecting this carbon from release back into the marine system and managing the marine environment to maximize its rate of accumulation could both play a role in mitigating climate change. For these reasons, in the context of an expanding “Blue Carbon” concept, research interest in the quantity and vulnerability of carbon stored in continental shelf, slope, and deep ocean sediments is increasing. In these systems, carbon storage is physically distant from carbon sources, altered between source and sink, and disturbed by anthropogenic activities. The methodological approaches needed to obtain the evidence to assess shelf sea sediment carbon manageability and vulnerability within an evolving blue carbon framework cannot be transferred directly from those applied in coastal vegetated “traditional” blue carbon habitats. We present a toolbox of methods which can be applied in marine sediments to provide the evidence needed to establish where and when marine carbon in offshore sediments can contribute to climate mitigation, focusing on continental shelf sediments. These methods are discussed in the context of the marine carbon cycle and how they provide evidence on: (i) stock: how much carbon is there and how is it distributed? (ii) accumulation: how rapidly is carbon being added or removed? and (iii) anthropogenic pressures: is carbon stock and/or accumulation vulnerable to manageable human activities? Our toolbox provides a starting point to inform choice of techniques for future studies alongside consideration of their specific research questions and available resources. Where possible, a stepwise approach to analyses should be applied in which initial parameters are analysed to inform which samples, if any, will provide information of interest from more resource-intensive analyses. As studies increasingly address the knowledge gaps around continental shelf carbon stocks and accumulation – through both sampling and modelling – the management of this carbon with respect to human pressures will become the key question for understanding where it fits within the blue carbon framework and within the climate mitigation discourse.
Introduction
Continental shelf sediments store large amounts of organic carbon (e.g., 21-73 Tmol C in the upper 10 cm of northwest European shelf sediments; Legge et al., 2020; Figure 1). This carbon is both allochthonous, sourced from terrestrial ecosystems (Santos et al., 2021), and autochthonous, derived from marine primary productivity. Organic carbon (Corg) is transported and transformed in the water column before reaching the seabed where it is further modified or buried (Najjar et al., 2018; LaRowe et al., 2020; Luisetti et al., 2020). Protecting this marine sedimentary carbon from release back into the marine system and managing the marine environment to maximize its accumulation and sequestration would contribute to climate change mitigation.
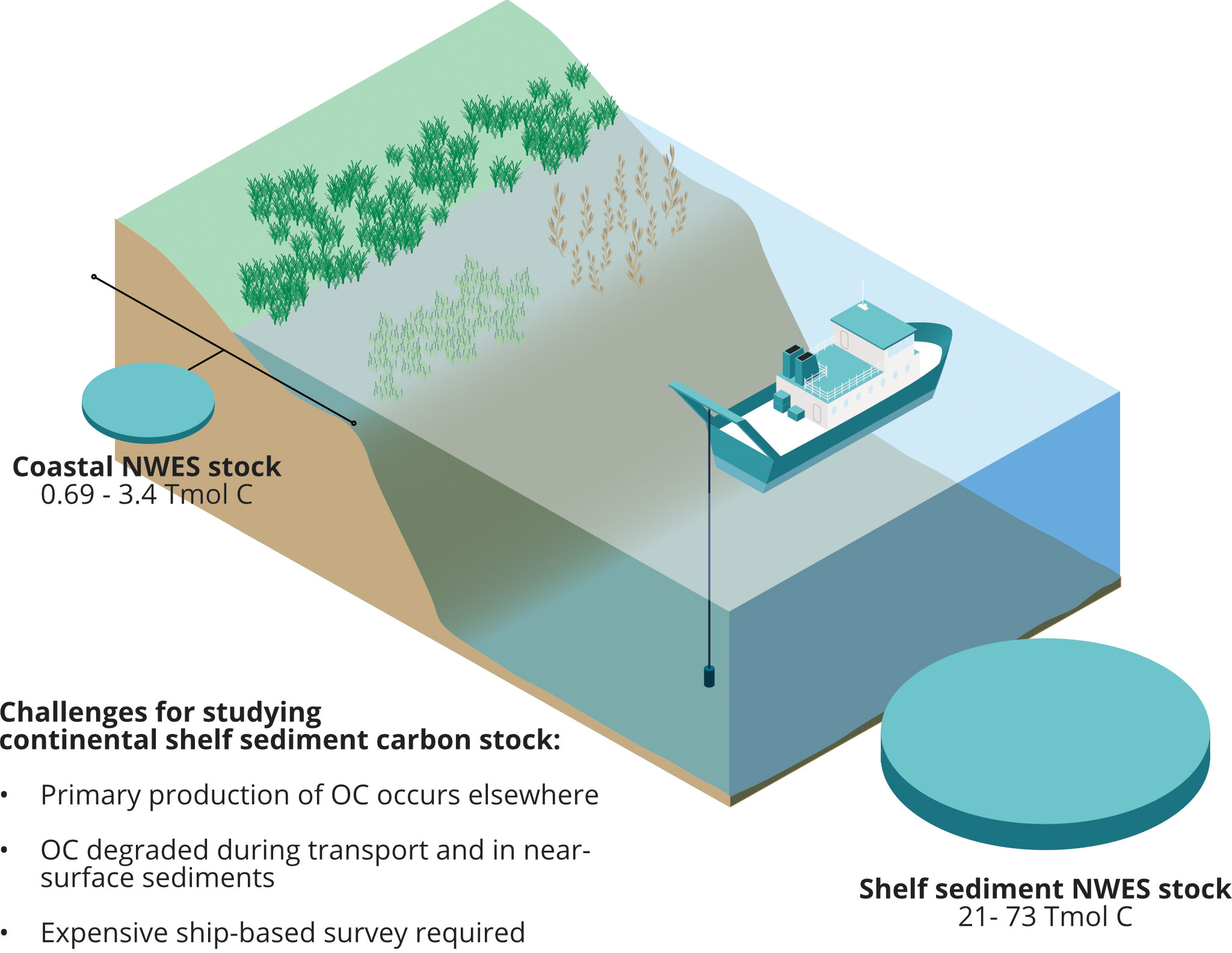
Figure 1 Estimates of marine organic carbon stocks for the northwest European shelf (NWES), from Legge et al., 2020, alongside schematic representation of carbon sampling in continental shelf highlighting some of the challenges compared to coastal blue carbon ecosystems. Cylinder volume is proportional to the mid-point of the coastal and shelf organic carbon stock estimates, with the full estimate range also given. Benthic stock is for the upper 10 cm of sediment. OC: organic carbon.
Blue carbon has become a high-profile topic in conservation and policy discussions, driving the need for a robust scientific evidence base on stocks and accumulation rates of marine carbon (e.g., Lovelock and Duarte, 2019; Macreadie et al., 2019; Christianson et al., 2022). As an emerging research area, blue carbon has been defined differently between studies, and there is ongoing discussion about which elements of marine carbon could and should be included in the blue carbon concept in different contexts (e.g., Krause-Jensen et al., 2018). Lovelock and Duarte (2019) set out criteria to determine if the carbon in a marine ecosystem should be considered as blue carbon from a climate mitigation perspective: removing greenhouse gasses at a significant scale, providing long-term storage for fixed carbon dioxide (CO2), the system being negatively impacted by anthropogenic activities, and the ability to manage the system to maintain or enhance the carbon services without social or environmental harm. Systems which satisfy these conditions are said to be “actionable” if they can be incorporated into existing mitigation policies. Herein, we consider blue carbon to be the organic carbon stored in marine ecosystems which contributes to climate change mitigation in a manageable way.
The traditional blue carbon ecosystems which can currently be included in formal carbon accounting mechanisms (IPCC, 2008; IPCC, 2013) are mangroves, seagrass, and saltmarsh (e.g., Luisetti et al., 2019; Luisetti et al., 2020), but research is increasingly bringing additional systems into the blue carbon discourse, recognising that these habitats (and their carbon) are not isolated from marine carbon cycling in the rest of the ocean (Santos et al., 2021). Macroalgae, for example, are recognized as a significant biomass carbon store, but since carbon is not sequestered long term within the habitat itself it is difficult to include in blue carbon financing frameworks (Krause-Jensen and Duarte, 2016; Krause-Jensen et al., 2018; Dolliver and Connor, 2022; Duarte et al., 2022). Several recent studies and ongoing research aim to improve recognition of the carbon storage ecosystem service macroalgae provide by evidencing their role as a carbon-donor habitat to sediments, such as those considered here (e.g., Trevathan-Tackett et al., 2015; Pessarrodona et al., 2018; Wernberg and Filbee-Dexter, 2018; Kokubu et al., 2019; Ortega et al., 2019; Queirós et al., 2019; Filbee-Dexter et al., 2020).
Similarly, research interest in the quantity and vulnerability of carbon stored in continental shelf, slope and deep ocean sediments is increasing (e.g., Avelar et al., 2017; Atwood et al., 2020; Legge et al., 2020; Smeaton, et al., 2021a; Sala et al., 2021). In these systems, carbon is less directly coupled to primary productivity than in coastal blue carbon habitats. The methodological approaches needed to obtain the evidence to assess shelf sea sediment carbon manageability and vulnerability within an evolving blue carbon framework cannot be transferred directly from those applied in traditional blue carbon habitats: different issues need to be considered, a multi-tool approach taken, and new and emerging techniques used.
Here, we set out how to obtain the evidence needed to establish where and when marine carbon in offshore sediments can contribute to climate mitigation, focusing on continental shelf sediments. The key questions to be addressed in this context are: (i) stock: how much carbon is there and how is it distributed? (ii) accumulation: how rapidly is carbon being added or removed? and (iii) anthropogenic pressures: is carbon stock and/or accumulation vulnerable to manageable human activities? These blue carbon focused questions link directly to the marine carbon cycling questions of carbon provenance and carbon reactivity. This research will provide the foundation upon which to determine if carbon in shelf sediments can be incorporated, without social or environmental harm, into existing climate mitigation frameworks and policy instruments and thus be considered actionable blue carbon.
Marine scientists have a range of measurement and analysis tools available to provide answers to these questions, from routine, rapid, low-cost analyses to highly specialized techniques which are still in development. We discuss these in turn as they relate to the underlying scientific questions. Together, they make up the marine sediment carbon toolbox from which appropriate sampling and analytical approaches can be chosen for the studies which will fill the evidence gaps identified and enable improved assessment of the role of the seabed in climate change regulation.
Carbon stocks
Quantifying stocks
The amount of organic carbon locked away from connection with the atmosphere, or – in the case of sediments, the ocean water column – is the fundamental parameter determining if and where shelf sea sediments satisfy the blue carbon criterion of “significant carbon storage”. The distribution of carbon stocks must be known in order to consider their importance, vulnerability and manageability.
The Blue Carbon Initiative1’s manual designed to provide standardized guidance to managers for assessing carbon stocks and emissions factors (Howard et al., 2014), enumerates the three elements required to quantify stocks: (i) (total) organic soil thickness (depth to underlying hard substrate), (ii) dry bulk density, (iii) organic carbon content (%Corg). In the context of continental shelf sediments, dry bulk density and organic carbon content remain critical, while total soil (sediment) depth is unlikely to be determined and furthermore not immediately relevant in the context of climate mitigation. The depth of interest is that which is likely to be disturbed by human activities, and thus contains “vulnerable” carbon stocks. In traditional blue carbon ecosystems the organic soil thickness is expected to vary from between 10 cm to over 3 m (Howard et al., 2014), and IPCC guidelines recommend the use of 1 m as a standard depth for reporting stocks in the absence of information on total soil depth (IPCC, 2008; IPCC, 2013). In marine sediments, the relevant depth of vulnerability will be influenced by the natural setting (e.g., water depth, sediment type, fauna) and the influence of potential disturbances (bottom trawling, deep-sea mining, dredging and disposal, etc., see Anthropogenic pressures). Many, though certainly not all, existing datasets are limited to near-surface sediments (e.g., upper 10 cm; Diesing et al., 2017; Wilson et al., 2018; Smeaton et al., 2021a) due to standard sampling procedures in routine monitoring programmes. Significant (and unquantifiable) uncertainty in estimated stocks is introduced where data are extrapolated beyond their measurement depth (e.g., to 1 m, Atwood et al., 2020) as it is not expected that Corg content remains constant between near-surface and deeper sediments. Generally, Corg is expected to decrease with depth due to remineralization/diagenesis (e.g., Burdige, 2007; Arndt et al., 2013; LaRowe et al., 2020) such that extrapolating near-surface carbon stocks to e.g., 1 meter depth would yield an overestimation of stock, and that deeply buried organic matter may be less labile and less likely to be remineralized to release CO2 if disturbed (see Characterising stock reactivity)
Determination of dry bulk density and organic carbon content in marine sediments are standard analyses which are routinely carried out by geologists, geochemists, and biogeochemists. Dry bulk density is the ratio of the mass of a dried sediment sample to its original volume. Porosity, the ratio of the volume of ‘voids’ (between sediment grains) to the total (wet) sediment mixture volume, is more frequently reported in marine sediments. Porosity is generally calculated by mass difference of wet and dried sediment, assuming a density for pore fluid (based on salinity) and sediment grains (typically 2650 kg cm-3 for quartz/feldspar) (e.g., Jenkins, 2005; Diesing et al., 2017). Empirical relationships have been used to estimate bulk density from sediment grain size (% mud) and Corg content itself (e.g., Diesing et al., 2017; Silburn et al., 2017; Atwood et al., 2020). The organic carbon content of marine sediments is commonly determined by elemental analyzer, alongside total carbon, inorganic carbon, and organic matter carbon: nitrogen (C:N) ratio (Verardo et al., 1990; Nieuwenhuize et al., 1994; Harris et al., 2001). This well established method, and its advantages over the lower-cost loss on ignition (LOI) approach, which relies on determining a habitat and/or site-specific conversion between organic matter and organic carbon, is described in detail in Howard et al. (2014) in the context of its application in coastal blue carbon habitats. A methodologically simplified approach based on ramped thermal heating alongside CO2 detection by infrared spectrometry has recently been applied to marine sedimentary carbon (Smeaton, et al., 2021a).
Total carbon stocks are usually derived by extrapolating spatially limited observations over a relevant spatial area to generate an inventory. The validity of, or numerical uncertainty in, the estimated total stock then depends on how representative the sampled sites are of the larger area of interest (Howard et al., 2014). Where this is partially addressed by estimating a different stock for different spatial sub-units (e.g., Burrows et al., 2014; Watson et al., 2020; Gregg et al., 2021), additional uncertainty is introduced by the estimation of the extent of the area for each. It is important to recognize the large uncertainty in values obtained by multiplying estimated stock over a large estimated area (discussed for soil Corg in Goidts et al., 2009). Since measurements in marine sediments require sampling from a research vessel, at high cost, achieving adequate spatial resolution for high confidence inventories is particularly challenging. Furthermore, there is a tendency for observations to be biased to near-coast and, because sediment coring is more challenging in coarse sediments, to higher mud-content sediments which are expected to contain high Corg (Figure 5 in Smeaton et al., 2021a). Linking carbon content to explanatory parameters (e.g., particle size, hydro-acoustic reflectance; Hunt et al., 2020) and applying statistical models or machine learning approaches to generate spatially resolved maps (Serpetti et al., 2012; Diesing et al., 2017; Wilson et al., 2018; Atwood et al., 2020; Smeaton et al., 2021a), can help to overcome issues of spatial bias in observations but assumes carbon processing, which controls stock, occurs in the same way across the region of interest. Co-measurement of the key physical and carbon content properties is a fundamental requirement for producing accurate stock assessments therefore determining additional sediment and environment parameters alongside carbon stocks adds significant value to carbon stock measurements.
Marine carbon stocks can also be determined by modelling. Process-based biogeochemical modelling studies focused on shelf sea carbon cycling (e.g., Kühn et al., 2010; Wakelin et al., 2012) generally assume marine carbon ultimately derives from marine primary production and, crucially, that most organic detrital carbon in the benthic system is remineralized over an annual cycle. With this assumption, models typically predict stocks of benthic Corg one to two orders of magnitude lower than observed (Aldridge et al., 2017). This discrepancy is resolved if it is assumed the models follow only the relatively labile carbon most important for correctly modelling the annual recycling of carbon and nutrients, whilst most of the observed carbon stock is highly refractory: does not degrade significantly over annual timescales. However, this means that model results do not, at present, provide appropriate stock values for sediment carbon inventories. Long term storage (sequestration) of highly refractory (non-mineralizable) carbon has been implemented within models, for example by explicitly assuming a background flux (Soetaert et al., 1996) or assuming a fraction of more labile carbon becomes deeply buried and no longer undergoes degradation (Butenschön et al., 2016).
Characterising stock provenance
To apply the blue carbon accounting framework, organic carbon in marine sediments must be linked back to the source of photosynthesis to avoid “double-counting”. For example: macroalgal carbon being accounted for in a carbon valuation of the coastal macroalgae habitat, and then again in offshore marine sediments where it is eventually buried. To protect or enhance the carbon storage potential of shelf sediments, management measures need to be applied not only locally, but also to the key carbon source ecosystems. Furthermore, the vulnerability of organic carbon in marine sediment to disturbance, in terms of release back towards the water column, is determined in part by its provenance as this influences how readily it is broken down and remineralized to CO2.
The primary production of much of the organic carbon subsequently stored in marine sediments occurred elsewhere: on land or in coastal habitats. In shelf seas marine phytoplankton are an important organic carbon source, though much of this carbon is expected to be recycled back to CO2 within ocean waters (e.g., Humphreys et al., 2019; Dang, 2020). In shallow waters, even away from the coast, microphytobenthos also fix carbon at the sediment surface (e.g., Reiss et al., 2007). Fundamentally, linking stored carbon to its source(s) relies on constraining the properties of the source itself: potential sources should be identified and analysed wherever possible, which is more challenging when carbon storage occurs at a distance from the carbon source and molecular properties are modified between source and sink.
In being transported to marine sediments, and after having been deposited there, organic matter is typically transformed by physical (Ausín et al., 2021) and biogeochemical processes (Middelburg, 2018; Kharbush et al., 2020). This chemical alteration makes it more difficult to identify the provenance of different components of sediment organic matter. Moreover, it often renders the organic material molecularly uncharacterizable. Within the sediments, interaction with the mineral surface (Kleber et al., 2021), and with iron minerals in particular (Lalonde et al., 2012) have been suggested as a key mechanisms for this. This fraction of the input organic carbon that is sequestered long-term in marine sediments, largely significantly altered from its original molecular form, is that which is largely resistant to remineralization to inorganic carbon. Organic carbon which is less altered, and thus more reactive/vulnerable to disturbance, can also be buried in marine sediments in environments of low biological activity (e.g., under low oxygen or low temperature conditions; Canfield, 1994).
Methods for determining the provenance of organic carbon in coastal blue carbon ecosystems (tidal marshes, mangrove and seagrass meadows) were recently reviewed by Geraldi et al., (2019). These authors concluded that the more different properties of organic matter that can be determined - in other words: applying a multi-tool approach - the more likely it is a single source can be unambiguously identified. When characterizing organic matter, the methods that provide greater source specificity are those that are performed on the smallest fractions of the bulk material (e.g., compound-specific analyses; see Figure 2.1 in Bianchi and Canuel, 2011). Here we place relevant methodology in the context of continental shelf sediments and consider issues and sources of uncertainty.
Bulk organic matter characterization
The majority of organic carbon stored in marine sediments is molecularly uncharacterizable (termed MUC) and thus cannot be characterized in terms of the biochemical classes (such as proteins or fats) that form living organisms. MUC can often not be extracted from the sediment (e.g., using solvent extraction or acid hydrolysis), and/or cannot by hydrolyzed into recognizable monomers that are amenable to quantification methods; it must be investigated using methods that consider the bulk organic carbon pool. These methods are particularly powerful if they can be related to or calibrated against other established source indicators, or a mechanistic understanding of organic matter transformation and preservation processes.
The most general and routinely used methods, both in marine sediments and in coastal blue carbon habitats, are bulk organic matter carbon to nitrogen ratio [or nitrogen to carbon, see Perdue and Koprivnjak (2007)], and δ13C and δ15N. These analyses are typically used in combination as, for example marine sources of organic carbon can have δ13C ratios between -20 ‰ and -30 ‰ which lie within the range of C3 terrestrial plants (Meyers, 1994), and C:N ratios of soils can fall in the range of those of marine carbon sources (e.g., Zhao et al., 2015). Information from bulk organic matter characterization can be combined using various mixing models: binary (Thornton and McManus, 1994), three end-member (Gordon and Goni, 2003), and Bayesian (Smeaton and Austin, 2017). Within these models, bulk elemental (C:N) and isotopic ratios of Corg sources (i.e., terrestrial soils, vegetation and marine algae) are used to constrain, though not unambiguously identify, the proportion of the Corg originating from each of the sources.
Different isotopic systems (e.g., δ2H, δ18O, δ34S) could potentially provide additional specific information on source and transformations of the organic matter in some settings but they have not yet been applied to marine sediment samples even within coastal ecosystems (Geraldi et al., 2019) and significant methodological development is needed before they can provide additional information in the context of continental shelf carbon sources.
Pyrolysis gas chromatography mass spectrometry (py-GCMS) can be used to investigate the macromolecular composition of sedimentary organic matter, including compound classes such as lignins, cellulose, carbohydrates and proteins. The technique involves heating a sample (e.g., at 700 °C) in an anoxic environment to yield thermal degradation products which are analysed by GCMS. The method has the advantage of not needing solvent extraction, which makes sample preparation less laborious than many biomarker techniques (described in Biomarkers), while also looking at the whole organic carbon pool rather than only the extractable pool, which can constitute as little as ~5% of the total organic carbon (Sparkes et al., 2016).
For example, py-GCMS allowed Kumar et al. (2020) to identify that organic carbon in Ria Formosa Lagoon, Portugal, was dominantly from algal, bacterial and marine macrophyte sources, with very little from terrestrial sources, and that pollution was from spilled petroleum products and sewage. In the Siberian Arctic, py-GCMS allowed differentiation of types of terrestrial organic carbon, with nitriles and alkylbenzenes derived from humified soils, and furfurals linked to much fresher/less degraded polysaccharides (Guo et al., 2004). In the Barents Sea, Stevenson and Abbott (2019) detected ecosystem shifts with distance from the ice edge, based on changing relative proportions of groups such as alkylphenols, alkylbenzenes, polysaccharides, and alkenes plus alkanes. However, uncertainties about the sources of these groups, and about whether groups are specific to particular sources (e.g., chitin is derived from shrimp and zooplankton, but also from peptidoglycan in bacteria), meant that analysis alongside ecological data was necessary to draw firmer conclusions.
One challenge posed by pyrolysis is that thermal degradation yields hundreds of different compounds, leading to complex chromatograms, and data that are difficult to interpret in terms of organic matter source and degradation state. This challenge has been tackled in some cases (Guo et al., 2004; Guo et al., 2009; Sparkes et al., 2016) by selecting and quantifying a sub-set of compounds (e.g., phenol and pyridine) that can be fairly confidently linked to specific sources (lignin and marine proteins, respectively). Although this means that only a sub-set of total organic carbon is being used to draw conclusions, the full pool is nonetheless being accessed by the analysis, and it does provide a systematic means of making comparisons between samples.
Cross referencing pyrolysis results with more established organic carbon source indicators can help to overcome the challenge of compound classes not being unique to certain organic carbon sources. Sparkes et al. (2016) determined marine versus terrestrial source from the ratio of phenols to pyridines in samples from the East Siberian Arctic Shelf. Through additional analysis of samples along offshore transects they were able to show that phenols were dominantly (although not exclusively) terrestrial, and pyridines were dominantly (but not exclusively) marine. They then showed that their pyrolysis-based index correlated well with other terrestrial versus marine indices, including δ13C, R’soil, and the branched and isoprenoid tetraether (BIT) index, which allowed it’s calibration even though the compounds on which it was based were not exclusive to either marine or terrestrial carbon sources.
Biomarkers
Biomarkers are organic molecules that may be traced unambiguously back to a specific biological origin. They constitute an important source-tracking tool for the characterization of aquatic organic matter (Derrien et al., 2019). Organic matter is a highly complex mixture of different types of compounds with varying physio-chemical properties originating from diverse sources. This includes allochthonous sources outside the aquatic system (e.g., higher plants from the terrestrial environment), autochthonous sources within the aquatic system (e.g., plankton and macrophytes such as seagrasses), and anthropogenic sources (e.g., sewage and oil spills). The molecular biomarker approach to source-tracking involves targeting specific classes of organic compounds, such as lipids, pigments, lignin-phenols, carbohydrates, and proteins, which can inform on provenance of Corg present in marine depositional environments. As a targeted approach, biomarkers are selective by nature and thus represent a specific portion of the bulk organic matter pool.
Some lipids (e.g., sterols, fatty acids) and pigments (chlorophylls and degradation products, carotenoids) produced by specific organisms have been widely used to trace carbon sources within marine particulate organic matter; their recalcitrance makes them good indicators of material exported to marine sediments (Kharbush et al., 2020). Lipid biomarkers are widely considered to hold the most promise as indicators of organic carbon provenance (Derrien et al., 2017; Derrien et al., 2019; Geraldi et al., 2019). The lipids used in this context possess all the necessary qualities of a good biomarker, being ubiquitous, chemically and diagenetically stable, and structurally diverse. Lipids (commonly n-alkanes and fatty acids) have been used to track organic carbon provenance in numerous marine environments from diverse sources including anthropogenic, allochthonous, and autochthonous inputs (Derrien et al., 2019).
The n-alkanes are hugely abundant, simple, straight-chain lipid molecules produced by many lifeforms including terrestrial plants, aquatic plants, plankton, and bacteria. Their chain length provides the key diagnostic feature indicative of biological origin, whilst a predominance of an odd- or even- number of carbon atoms in the structure is also informative. Bacteria, algae, and plankton, for example, all produce short-chain n-alkanes (ca. <C20), while plankton exhibit a predominance of an odd number of carbons (15, 17, or 19), and bacteria, an even number of carbon atoms (16, 18, 20). Mid chain n-alkanes (ca C20-25) are associated with aquatic macrophytes such as seagrasses and saltmarsh plants. Long chain n-alkanes (C25-35) are found in the leaf wax of vascular plants and have a predominance of an odd number of carbons (27, 29, 31) and are largely from terrestrial sources. These variations in the n-alkane structure with associated biological origins led to the development of the carbon preference index (CPI) broadly as an indicator of terrestrial versus petrogenic sources (Bray and Evans, 1961). The CPI has since been adapted and developed alongside many other n-alkane biomarker indices including the average chain length (ACL) and numerous other marine-aquatic-terrestrial discriminating ratios capable of identifying organic matter sources (Derrien et al., 2017). Similar indices also exist for other lipids such as fatty acids and are fully reviewed in Derrien et al. (2017).
Whilst biomarkers can identify a wide range of different inputs to bulk organic matter, including providing high resolution for mixed sources (Derrien et al., 2017), like any analytical tool they also have their limitations. For example, constraining the sources of organic matter using biomarkers can sometimes be a challenge within more complex marine environments, such as those with significant coastal inputs in addition to marine and terrestrial sources. Advancing analytical methodologies include the very powerful combination of organic biomarkers, such as lipids, alongside the measurement of their specific isotopic signatures (typically stable C, H, N, O, S), termed compound-specific-isotope-analysis (CSIA). This technique imparts added value to the biomarker approach yielding increased specificity and the ability to discriminate between multiple and sometimes overlapping sources of organic matter. It utilizes the difference in isotopic composition of biomarkers from terrestrial, aquatic, and marine sources, which are all distinct and reflective of the CO2 substrate (e.g., atmospheric, aquatic, marine) used for photosynthesis.
Sikes et al. (2009) combined the n-alkane lipid biomarkers with δ13C to help resolve overlapping and masked sources of organic matter in surface sediments in the Hauraki Gulf, New Zealand. Bulk stable isotope analyses alone could not fully resolve the terrestrial signal, and n-alkane chain length indices were also insufficient due to overlapping and non-unique n-alkane signatures from confounding coastal sources (mangroves, seagrass) alongside the terrestrial and marine inputs. The differences in isotopic composition of biomarkers revealed by CSIA meant that the coastal input could be fully resolved from the terrestrial and marine signals. Sikes et al. (2009) thus demonstrated how an appreciable amount of purely terrestrial-derived organic matter was transported from input locations across the shelf and redistributed and buried in sediments including outer shelf locations towards the continental slope.
The ability to trace and quantify the allochthonous contribution to marine organic matter is essential for accurate carbon accounting (Kharbush et al., 2020). This involves understanding how terrestrial carbon moves through marine ecosystems: the traditional blue carbon ecosystems (mangroves, salt marshes, seagrass), as well as estuaries and continental shelf sediments, and how this carbon may subsequently be sequestered and stored. Lignin biomarkers are a powerful tool to probe the terrestrial contribution. Methodologies that continue to advance our understanding of the various distinct terrestrial inputs include the use of radiocarbon (as an addition to stable carbon and other elements) CSIA of lignin and other biomarkers. Tao et al. (2015) used the abundance and carbon isotope composition (13C and 14C) of n-alkane and fatty acid lipids and lignin-phenols to characterize source, composition, and age of suspended particulate organic carbon from the Yellow River, China. Natural allochthonous Corg inputs via rivers include recently biosynthesized biomass, pre-aged mineral-associated soil, and ‘ancient’ sedimentary rock sources. The latter two contain more refractory carbon as they have undergone prior processing and aging, and this has important implications for subsequent carbon cycling and storage in shelf sediments. Tao et al. (2015) found that, while bulk isotope measurements were relatively uniform for 13C and showed old 14C ages, it was only the differences in the 14C ages of individual biomarkers that could fully reveal the various individual and very different terrestrial Corg sources. Linking the terrestrial Corg to its distinct source or sources informed on the nature of the exported Corg. In the case of the Yellow River, the refractory nature of much of the particulate organic carbon supports the high burial rates observed for terrestrial Corg in the delta area.
eDNA
Environmental DNA (eDNA) refers to traces of DNA left behind in the environment (soil, water, air, or sediment) by organisms through processes such as sloughing, shedding, or injury (Harrison et al., 2019). Since its first use to detect non-native species (Ficetola et al., 2008), eDNA has been used in a range of applications including rare and cryptic species identification, pathogen monitoring, fisheries stock assessments, diet studies, as well as characterizing the biodiversity of entire communities (Lallias et al., 2015; Deiner et al., 2017; Fonseca et al., 2017; Gilbey et al., 2021). DNA makes up approximately 3% of the cellular Corg in sediments (Landenmark et al., 2015) and therefore is adept at determining the provenance of marine carbon. Furthermore, eDNA techniques are fast, inexpensive, and capable of identifying carbon provenance to a finer taxonomic rank (species-level) than other techniques such as biomarkers, and stable isotopes (Reef et al., 2017).
At present, we are only aware of three published studies using eDNA to determine sources of Corg in marine sediments (Reef et al., 2017; Queirós et al., 2019; Ortega et al., 2020). Reef et al. (2017) used eDNA analysis, alongside stable isotope analysis, to identify the origin of Corg in seagrass meadows. Using a chloroplast gene, Reef et al. (2017) were able to identify traces of 150 plant taxa in the sediment. However, 88% of sequences belonged to seagrass, suggesting most Corg was autochthonous, originating within the system. Ortega et al. (2020) applied a similar approach using a ribosomal gene, in conjunction with stable isotope analysis, to identify sources of Corg in seagrass meadows and mangrove forests. A total of 40 plant taxa were identified, showing different communities of plants contributed to blue carbon in mangroves and seagrass meadows. In contrast to seagrass meadows in the Reef et al. (2017) study, Ortega et al. (2020) discovered substantial macroalgal input to stored carbon. The study also investigated if a quantitative relationship existed between i) detected eDNA and sediment eDNA, and ii) detected eDNA and sediment carbon content using mock community experiments. In both instances a high correlation was found, with an R2 of 76% and 86% found in the sediment DNA and carbon experiments, respectively. This suggests that sedimentary eDNA studies may be able to provide interim information on Corg if a stable isotope analysis has yet to be conducted. The Queirós et al. (2019) study used a ribosomal gene to detect eDNA in the top centimeter of sediments, alongside stable isotope analysis and benthic-pelagic process measurements, to understand the contribution of macroalgae to subtidal coastal ocean carbon stocks. One hundred and forty-eight plant taxa from surrounding coastal habitats were detected, suggesting a range of macroalgae species contribute to sedimentary Corg. A method for tracing macroalgal carbon in deeper marine sediments has been developed by the Norwegian Institute for Water Research (NIVA) to investigate long-term sequestration (burial) (d’Auriac et al., 2021). d’Auriac et al. (2021) quantified L. hyperborea and S. latissimi. eDNA levels in marine sediments down to 1.6 meters below the seafloor to estimate their contribution to the organic carbon stock and understand how it varied with time and since deposition.
Despite established protocols for sediment DNA-based biodiversity assessments (Fonseca and Lallias, 2016; Fonseca, 2018; Pawlowski et al., 2022) there remain technical considerations when conducting a marine carbon study. eDNA biodiversity assessments require the collection of a sediment sample, extracting the DNA from the sediment with no prior organism separation, followed by polymerase chain reaction (PCR) to create copies of the eDNA (called amplicons). These are then sequenced (obtaining the nucleotide composition of the eDNA), and the retrieved sequences are taxonomically assigned using either publicly available genetic databases such as BOLD (Ratnasingham and Hebert, 2007) or GenBank (Clark et al., 2016) or bespoke taxonomic libraries.
Every stage of the workflow has aspects that will affect the outcome of the assessment. Factors associated with sampling such as the body size of organisms in sediment, whereby larger organisms are more likely to be detected (Elbrecht et al., 2017), and seasonality and environmental conditions, which affect how quickly DNA decays (Jo and Minamoto, 2021). Similarly other sources of variation to be considered are habitat heterogeneity and sampling effort i.e., organism ability to disperse and its detectability and representativeness through enough biological replicates (Fonseca, 2018; Grey et al., 2018). Methodological considerations include the DNA extraction method, since different extraction techniques will reflect different components of existing biodiversity (Geraldi et al., 2020). Further considerations of technical replicates are needed, such as the number of DNA extractions or PCR replicates used (more replicates give more robust biodiversity estimates) (Ficetola et al., 2015; Fonseca, 2018; Bruce et al., 2021).
During PCR, the primers (short single stranded sequences of DNA) are used to target the sections of eDNA to copy. DNA is copied more readily if there is greater similarity between the primer and the target eDNA. Therefore, DNA from different taxonomic groups will be copied at different rates (based on similarity to the primers) and the quantity of apparent DNA from each taxon will be distorted. This misrepresentation of biodiversity present is commonly referred to as “primer bias” and is inherent to most PCR-based approaches. In marine sediment carbon this means any given set of primers will not provide a complete picture of Corg origins; an assay to identify terrestrial-plant DNA may not be capable of detecting phytoplankton DNA (or vice versa). In practice, this means a standardized primer set is unlikely to work for all marine sediment carbon applications, instead primers should be chosen with careful consideration given to the biodiversity present. Fortunately, it is possible to use multiple primers to test different aspects of biodiversity to ameliorate the effects of primer bias, though analysis cost increases with each additional primer.
The selection of genetic reference databases is also a key step in a marine sediment carbon study (Geraldi et al., 2019). In essence, to identify taxa and assign taxonomic identities from DNA, the taxa must already be present, and correctly identified with the relevant DNA region, on a reference database. If absent or mislabeled, the DNA cannot be identified and can lead to an underestimation, or total exclusion, of Corg sources. This can be combatted in some instances by assigning higher taxonomic resolution (i.e., when species level identification is not possible, similarities to a closely related species on the database may facilitate genus or family level identification). Furthermore, a site-specific reference taxonomic database can be constructed but will require additional time and budget.
A final consideration whether or not the number of sequences produced corresponds to the amount of eDNA, biomass or Corg in the sample. Recent meta-analyses suggest that whilst some quantitative relationship between DNA and biomass exists, there is a high degree of variability, likely depending on a combination of the primers used and the community of organisms surveyed, e.g., unicellular or multicellular (Lamb et al., 2019; Yates et al., 2019). Thus, the sequences retrieved from eDNA would not correspond to the total amount of carbon from those taxa. Although molecular techniques are being refined to facilitate quantitative analysis, at present the extent to which sequences correspond to Corg using a mock community (Ortega et al., 2020) should be explicitly tested before molecular data are used in this manner.
Characterising stock reactivity
Not all organic carbon present in marine sediments at a given time will be stored over timescales greater than weeks to months to years (LaRowe et al., 2020). The total stock of organic carbon can be classified by its biogeochemical reactivity (Alderson et al., 2016), a key biogeochemical model parameter (Arndt et al., 2013). Organic matter reactivity or degradability (which can be defined as susceptibility to removal of a fraction by either biotic or abiotic processes, measured by lifetime e.g., Hansell, 2013) is understood to be a continuum (e.g., LaRowe et al., 2020) from labile (reactive; easily degraded; short lived) to recalcitrant (resistant to rapid microbial degradation; Hansell, 2013) and refractory. The term refractory is defined variability in different studies and used as “a one-fits-all word” for various properties related to Corg reactivity, typically as the opposite of labile in a certain context, see discussion in Hansell, 2013 and Baltar et al., 2021. Understanding sedimentary organic matter’s re-mineralization potential and ability to resist degradation (Doetterl et al., 2016) is vital in estimating its vulnerability to potential natural or anthropogenic disturbance and remineralization of the associated organic carbon.
The balance of organic carbon fractions with various reactivities determines the biodegradability of sedimentary organic matter and in turn the stability of the associated carbon. The rate of microbially-mediated degradation of organic matter can be impacted by the addition of more or of less labile material to a mixture (the priming effect; Bianchi and Canuel, 2011). If disturbed, the organic carbon in sediments dominated by labile organic matter is highly likely to be remineralized. Conversely if sediments dominated by recalcitrant organic matter are disturbed this material would likely be re-deposited as particulate organic carbon on or in the seabed. Only organic carbon of some intermediate degradability is thus relevant to the climate mitigation role of marine sediments.
Organic carbon reactivity is fundamentally linked to the organic matter’s source, discussed in Characterising stock provenance. Black carbon, a refractory carbon fraction formed from incomplete combustion of organic carbon, accounts for 15-30% of the organic matter buried in marine sediments (Middelburg et al., 1999). The most refractory fraction of the black carbon is highly condensed soot, formed from combustion of fossil fuels at high temperatures (Masiello, 2004), which may remain in the atmosphere for months before eventually being buried in sediments. More degradable lightly charred black carbon formed during low temperature biomass burning may reach the marine environment via rivers through soil leaching or erosion (Coppola et al., 2014). Once deposited in sediments, factors including the depth of burial, microbial and redox conditions as well as subsequent disturbance influence the degree of black carbon’s long-term storage. In general, small soot particles withstand degradation and result in long-term storage whereas lower formation temperature/larger size fragments are more susceptible to biotic and chemical degradation (Masiello, 2004).
Chew and Gallagher (2018) show that black carbon can be a major component of organic carbon in seagrass, mangrove and saltmarsh sediment cores and because it is resistant to being re-released back into the atmosphere, as CO2 for example, assert that it should be discounted from any blue carbon storage mitigations being proposed. Taking a different perspective, Ren et al. (2019) highlight that the relatively slow cycling of black carbon in Arctic Ocean sediments makes it an important fraction of inert carbon representing a significant sink of atmospheric CO2, which is likely to increase with global warming and increased melting of permafrost. There is thus a clear need to understand both the overall reactivity of the marine organic matter as well as to identify specific types and sources of organic carbon which are resistant to degradation.
Thermogravimetric analysis (TGA)
Organic matter recalcitrance can be defined in terms of the temperature at which mass is lost during combustion. TGA is an automated process which continuously measures the mass of a sample during ramped heating (e.g., from 0-1000°C at a rate of 10°C min-1). Thermal decomposition through TGA allows the major organic matter fractions to be quantified. Within the literature, the thermal ranges which define these organic matter fractions are variable depending on environment and methodology (Table 1). Within marine sediments these organic matter fractions are thermally defined as labile (200 - 400°C), recalcitrant (400 - 550°C) and refractory (550-650°C) (Capel et al., 2006; Smeaton and Austin, 2022). Focusing on the 200-650°C temperature range removes interference from absorbed water and non-organic material (i.e., calcite).
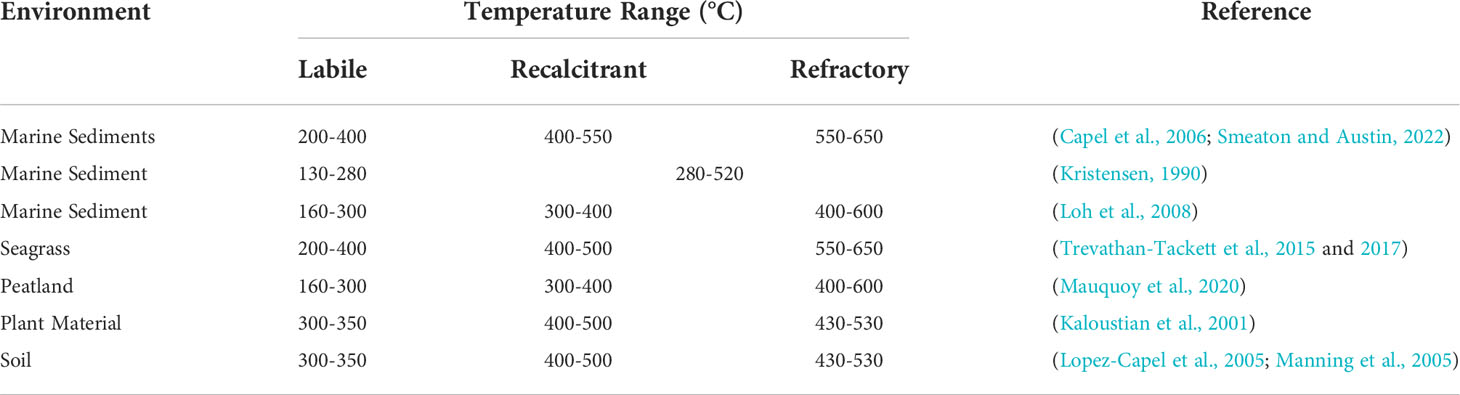
Table 1 Temperature ranges defining organic matter recalcitrance by thermogravimetric analysis in different environments.
Most studies using this thermal approach characterize the organic matter into the three fractions, although some studies go further and use the thermal decomposition data to quantify specific components (e.g., hemicellulose, cellulose, lignin) (Trevathan-Tackett et al., 2015; Trevathan-Tackett et al., 2017) or functional groups such as aliphatic and carboxyl groups (Lopez-Capel et al., 2005; Manning et al., 2005) potentially allowing this approach to be applied to determine the provenance of the organic matter.
The quantification of the different thermal fractions allows the lability of organic matter to be estimated (Smeaton and Austin, 2022). A number of indices have been developed for this purpose ranging from the early Rp index (Kristensen, 1990), which used stepwise thermogravimetry (i.e., Loss on Ignition), to the Refractory index (R-index) and Carbon Reactivity Index (CRI), which utilize TGA data and provide an advanced understanding of the thermal characteristics of organic matter in different environments (Trevathan-Tackett, 2016; Smeaton and Austin, 2022). For example, a CRI value of 0 indicates that the material is fully biodegradable (high reactivity), while a value of 1 indicates that the substance is non-biodegradable (low reactivity) (Smeaton and Austin, 2022).
The gases evolved during TGA can be further analysed by coupling the system to an FTIR (Fourier transform infrared spectroscopy) or GCMS to provide greater understanding of (macro) molecular composition. Within marine sediments these approaches are largely focused on detecting and quantifying pollution (Oudghiri et al., 2015; Becker et al., 2020) but they have the potential to be powerful tools in characterizing organic matter. While understanding the recalcitrance of organic matter is one of the main applications of TGA, the continuous nature of the data produced results in their applicability to many areas including provenance studies (Oudghiri et al., 2015; Becker et al., 2020).
Amino acid index
The amino acid composition of organic matter in marine sediments can be used as an indicator (biomarker) of its degradation state. Total hydrolysable amino acids (THAA) are quantified by reverse-phase HPLC of their fluorescent derivatives (Dauwe and Middelburg, 1998), and their relative abundances used to generate an index for degradation state which indicates the recalcitrance of the remaining material (Dauwe and Middelburg, 1998; Dauwe et al., 1999). This method was developed for North Sea continental shelf sediments, where phytoplankton primary productivity is the main source of organic matter. It has more recently been applied as part of a study of organic matter in the South Yellow Sea and East China Sea sediments (Chen et al., 2021), and in coastal blue carbon habitats (Vaughn et al., 2021). Recently, compound-specific radiocarbon analysis of amino acids has been proposed as a novel method for improving understanding of food webs and sedimentary organic carbon (Blattmann and Ishikawa, 2020; Blattmann et al., 2020).
Black carbon quantification
To measure black carbon, traditional methods require the removal of inorganic carbon using the same methodology as that used to prepare samples for organic carbon analysis, with either sulphurous acid (Verardo et al., 1990; Jablonski et al., 2002; Phillips et al., 2011) or hydrochloric acid (Komada, 2008; Brodie et al., 2011). Following removal of inorganic carbon, there are a number of methods used to quantify fractions of black carbon (Hammes et al., 2007; Table 2). Chew and Gallagher (2018) compare chemo-thermal oxidation (CTO) and chemical nitric acid oxidation (NAO) and conclude that the presence of thermally resistant phytoliths was responsible for differences in black carbon results from these two methods.
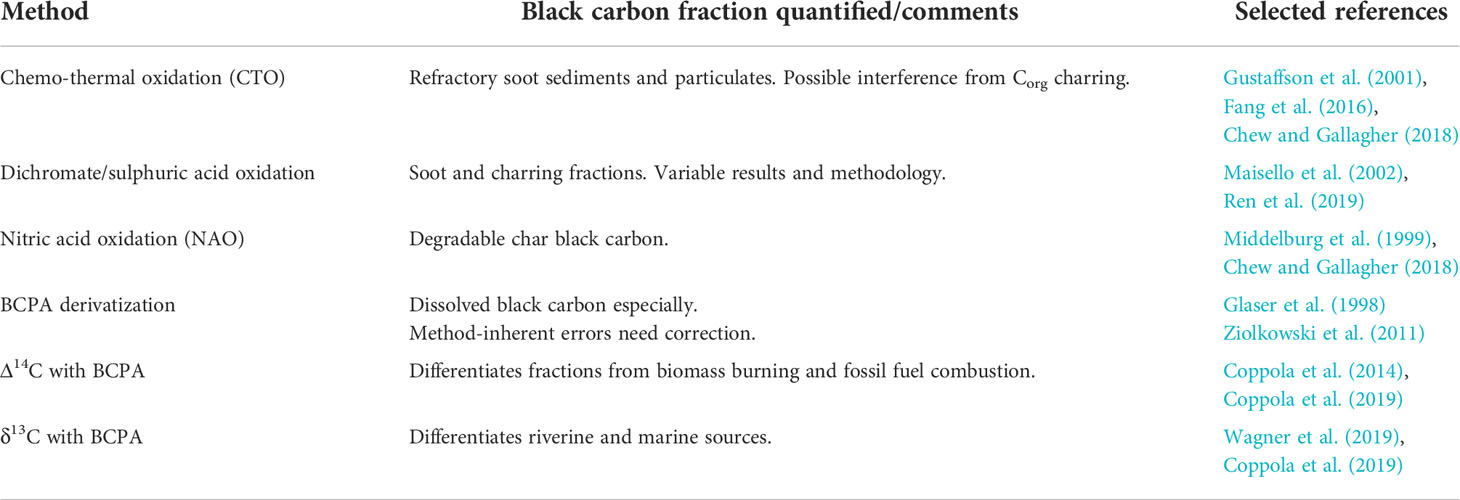
Table 2 Methods used to quantify black carbon, following removal of inorganic carbon (Hammes et al., 2007), including references where these methods have been applied.
Newer instrumentation, based on the temperature gradient method is now being applied for carbon measurements (Smeaton, et al., 2021a) with the advantages that no acid carbonate removal is required to remove inorganic carbonates, and that measurements of particulate organic carbon, black carbon (residual oxidizable carbon (ROC)) as well as inorganic carbon can be derived from a single sample. Results have been shown to be comparable with the more traditional methods (Natali et al., 2020).
The priming effect
Priming occurs when the rate of remineralization of stable organic matter is either increased or decreased by addition of labile organic matter (Sanches et al., 2021). As such, simply measuring the recalcitrance of organic matter in marine sediments may not fully capture the vulnerability of stored carbon when the system is disturbed, i.e., if the disturbance mixes stable organic matter with a labile fraction. Sanches et al. (2021) reviewed studies of priming in aquatic systems and found that the observed magnitude and direction of the effect depended on whether a lab or field-based approach was applied and on the proxies used to measure remineralization. Their meta-analysis concluded that, in general, priming has a positive effect (increased remineralization of stable organic matter with the addition of labile organic matter). They recommend using bacterial growth as a proxy (in preference to production or respiration) or CO2 production as these approaches are most sensitive. For example, van Nugteren et al. (2009) added 13C-enriched diatoms to marine sediment slurries and measured evolved CO2 to observe a 31% increase in remineralization.
Carbon accumulation rates
Quantification of the rate that carbon accumulates in shelf sediments is the key parameter for natural carbon accounting, for which carbon burial is the service of interest (Luisetti et al., 2020). In the context of blue carbon projects, “additionality” must be shown: that management activities lead to an increase in carbon sequestration relative to accumulation rates in the unmanaged system (e.g., Lafratta et al., 2020).
The Blue Carbon Initiative2’s manual designed to provide standardized guidance to managers for assessing carbon stocks and emissions factors (Howard et al., 2014), discusses carbon accumulation and loss in a chapter entitled ‘how to estimate CO2 emissions’ – explicitly emphasizing the motivation of assessing impacts on the atmospheric CO2 budget. The caveat of assuming all, or a given proportion of, sediment carbon accumulation or loss being in connection with the atmosphere is presented therein in this context (Howard et al., 2014). The simple surface elevation table (SET) method often applied in coastal blue carbon ecosystems is not appropriate in marine sediments. Instead, natural radiotracers, including carbon-14 (14C, radiocarbon), lead-210 (210Pb), and thorium-230 (230Th, uranium-thorium dating), are routinely used to determine rates of marine sediment accumulation. 210Pb and 230Th are both part of the 238U decay chain and are deposited from the atmosphere at a relatively constant rate with abundances in marine sediment decreasing with depth as they decay (Appleby and Oldfield, 1978). 14C is absorbed from the atmosphere into the tissue of living organisms, and its abundance decays after death (Anderson et al., 1947; Libby et al., 1949). Other dating tools include tephra chronology (Cage et al., 2011; Davies, 2015), optical luminescence (OSL) dating (Madsen et al., 2005) and direct methods to measure the rate of particle accumulation on the seafloor, like sediment traps (which generally underestimate particle fluxes due to particle solubilization, zooplankton swimmers and hydrodynamics; Subha Anand et al., 2017).
Differentiation between radiotracer techniques is based predominantly on the time interval over which their decay rate (half-life) allows material to be dated. Radiocarbon based age-control is typically used to estimate long-term (100s–1000s of years) sediment accumulation rates, while radionuclide dating (i.e., 210Pb) provides a chronological constraint of modern deposition over the last ~150 years. The limitations of different methods can be partially addressed by combining different radiotracers, but the dates obtained do not always agree (e.g., Jenkins, 2018: shorter-period dates tend to be higher than long-term dates; the Sadler effect; Sadler, 1981). For example, sedimentation rates from 14C are generally found to be lower (slower) than those derived from 210Pb (Baskaran et al., 2017; Smeaton et al., 2021b) because of the effects of sediment compaction (Bird et al., 2004) and diagenetic processes (biogeochemical alteration) that can alter the organic matter (Arndt et al., 2013; LaRowe et al., 2020). Baskaran et al. (2017) suggest that short-lived radioisotopes are better suited to determining modern depositional rates than radiocarbon and recommend using 210Pb where possible when determining sediment accumulation rates.
Radiotracers can also be applied to track carbon through the ocean and or sediment system. While not directly providing organic carbon burial rates, these more focused studies are an integral part of developing a broader understanding of marine carbon cycling. For example: the use of 14C and 35S radiolabeling techniques to track microbial recycling of organic carbon in the sediments (Beulig et al., 2017).
Lead-210-derived sedimentation rates and dating
The process of assigning ages to organic carbon present in specific layers (depth intervals) of marine sediment can be achieved by measuring the activity concentration of co-occurring 210Pb (Tolosa et al., 1996; Pappa et al., 2019). In relatively undisturbed depositional aquatic ecosystems 210Pb abundance decreases exponentially with depth from the seawater-sediment interface due to the radioactive decay of the tracer, allowing the 210Pb depth profile to estimate a sedimentation rate for any substances of interest associated with the sediment. Application of 210Pb dating remains a challenge because of unresolved issues associated with the variety of radioanalytical methodologies available as well as different modelling approaches that play an essential role in deriving sediment accumulation rates from radiotracer measurements (MacKenzie et al., 2011; Garcia-Tenorio et al., 2020).
Disturbances can complicate the straightforward determination of sediment accumulation rates from 210Pb depth profiles. The surface 10-15 cm of the seabed are likely to be disturbed by, e.g., bioturbation, fishing activities and natural resuspension by bottom water currents (see Anthropogenic pressures). Furthermore, dating must account for the additional sources of 210Pb within the sediment, from the in-situ decay of radium-226 (226Ra) and, more problematically, from industrial activities including offshore oil and gas and other mineral processing industries (Sahu et al., 2014; Ahmad et al., 2021). The proportion of 210Pb present in the sediment and from human activity (termed supported 210Pb) does not decay at the same rate as the unsupported 210Pb fraction from atmospheric deposition (Gulliver et al., 2001; Cook et al., 2004). Measuring 226Ra in addition to 210Pb enables the subtraction of supported 210Pb from the total 210Pb measured prior to estimating sedimentation rates and dating sediment layers.
With a half-life of 22.3 years, 210Pb dating is limited to relatively recent sediment ages (up to 150 years). It can therefore be challenging to use this technique in low-sedimentation rate marine environments (<0.15 cm y-1) (De Haas et al., 1997). There are several 210Pb dating models available, that can be applied and help interpret different 210Pb core profiles (Arias-Ortiz et al., 2018; Blaauw et al., 2018). These models have been comprehensively described over the last forty years. The Constant Initial Concentration (CIC) (Robbins, 1978), Constant Rate of Supply (CRS) (Appleby and Oldfield, 1978) and Constant Flux: Constant Sedimentation (CF : CS) (Appleby and Oldfield, 1978) models are the most widely used to estimate sedimentation accumulation rates, but they are associated with numerous assumptions that can sometimes limit their appropriateness in more complex and disturbed aquatic ecosystems (Arias-Ortiz et al., 2018). Very often, the mass accumulation rate, expressed in mg cm-2 yr-1, is also estimated to account for compaction effect (Schmidt et al., 2014). More recently the advantages of a Bayesian approach, as applied in radiocarbon dating (Blaauw et al., 2018), is gaining attention (Aquino-López et al., 2020; Blaauw et al., 2021).
210Pb in environmental matrices, including marine sediments, is commonly determined directly by gamma spectrometry. However, gamma spectrometers equipped with Well-type germanium (Ge) detectors that allow the direct determination of low levels of 210Pb with little sample mass and preparation required are not commonly available. More common and cheaper flat end Ge detectors with much higher limits of detection can provide insufficient levels of accuracy and precision for measuring the very low levels of 210Pb naturally present in the environment (Dal Molin et al., 2018). Alternatively, 210Pb can also be determined indirectly by measuring polonium-210 (210Po), its granddaughter decay product, via alpha spectrometry. Although this alternative traditional radiometric technique requires samples to be digested in strong acid mixtures and treated prior to analysis, the limits of detection and level of accuracy and precision that can be achieved are more appropriate (Hassen et al., 2020). The underlying assumption, that 210Pb is in secular equilibrium with 210Po such that both radionuclides are present at the same concentration, can be verified by performing a second analysis of 210Po at a later stage, i.e., generally, by determining 210Po ingrowth from the decay of 210Pb after three to four months.
To determine 226Ra and correct for supported 210Pb, gamma spectrometry is the most common technique. The sample must be placed in a radon proof container for a minimum of three weeks prior to analysis to ensure secular equilibrium is established before indirect measurement via its two decay products (bismuth-214 and lead-214). 226Ra can be determined indirectly by either measuring emission of radon-222 gas, or directly by alpha-spectrometry, and more recently by ICP-MS, following appropriate sample preparation steps (IAEA, 2010).
Caesium-137 (137Cs) is commonly used as a complementary tool to validate 210Pb dating models. Characteristic abundance peaks of 137Cs observed in marine sediments in the Northern hemisphere can be directly related to nuclear weapon testing events of the late 1950s and early 1960s, and more locally in the UK seas to peak discharges from Sellafield nuclear reprocessing plant in the late 1970s (Environment Agency et al., 2021). Caesium-137 can easily be measured by gamma spectrometry, however, because its concentration is constantly decreasing over time [half-life of 30 years and no major additional characteristic peak since Chernobyl; see Figure 7.9 in Environment Agency et al. (2021)], it is important to optimize the counting time to ensure reliable and accurate results by gamma spectrometry (Garcia-Tenorio et al., 2020).
Radiocarbon dating
The radioactive 14C isotope has a half-life of 5,700 ± 30 years (Kutschera, 2013) which allows for the determination of the age of a carbon bearing material formed over the past 55,000 years (Hajdas et al., 2021). Since the detection of 14C in 1977 using particle accelerators (Bennett et al., 1977), Accelerator Mass Spectrometry (AMS) has become the most used technique in 14C analysis. In AMS, the 14C/13C or 14C/12C ratio in the sample is directly measured resulting in shorter measurement times (days to minutes) and reduced sample requirements (g to µg of C) than previous scintillation methods (Litherland et al., 2011). Recent developments in AMS technologies have resulted in 14C detections at significantly lower energies (Synal et al., 2000), resulting in the reduction in size of AMS’s and the establishment of new sample introduction techniques for direct analysis of CO2 resulting from combustion (EA-AMS) (Haghipour et al., 2019), wet oxidation (Leonard et al., 2013), acid hydrolysis (Molnár et al., 2013) and laser ablation (LA-AMS; Welte et al., 2016).
The data produced by the AMS (Fraction 14C or F14C) allows for conventual 14C ages to be calculated following the approach of Libby (1955) where conventional 14C age = -8,033ln(F14C). To determine the true age of the sample, the conventual 14C age must be calibrated to a curve based on measurements of 14C from samples of known age and location (e.g., northern hemisphere, southern hemisphere, or the marine environment). The IntCal radiocarbon calibration curves (Heaton et al., 2020; Hogg et al., 2020; Reimer, 2020) are a resource constructed from measurements of 14C made in different laboratories on a range of dated samples. The last 13,900 years are constrained by dated tree rings (Reimer, 2020), while beyond this (13,900-55,000 years) lake and marine sediments, speleothems or corals are statistically integrated to create the most accurate representation of past atmospheric 14C (Reimer, 2020).
Today, conventional 14C ages are calibrated using advanced Bayesian statistical tools that include dedicated routines for the different calibration curves, the most commonly used modelling tools are OxCal (Ramsey, 1995; Ramsey et al., 2020) and MatCal (Lougheed and Obrochta, 2016). Calibrated 14C ages are referred to as a calendar age counted backwards from 0 cal BP (before present) which corresponds to 1950 Common Era (CE, previously referred to as AD) or to calendar years Before Common Era (BCE, previously referred to as BC). Post 1950 CE correspond to the age of nuclear testing where atmospheric 14C concentrations measured in CO2 of air and dated tree rings, display significantly elevated 14C concentration (bomb spike) hindering the use of conventional 14C dating approaches (Hua et al., 2013; Santos et al., 2020). To calculate sedimentation and accumulation rates an age-depth model is constructed using the calibrated dates. Classical age-depth models can be constructed in software such as CLAM (Blaauw, 2010), but more commonly Bayesian statistical packages such as OxCal (Ramsey, 1995; Ramsey, 2009), and BACON (Blaauw and Christen, 2011), are applied to create models that fully account for uncertainties allowing for the calculation of sedimentation and accumulation rates.
There is a long history of using 14C to chronologically constrain sedimentary records allowing the calculation of long-term (100s-1000s of years) sedimentation and accumulation rates on the seabed (Kershaw, 1986; Darby et al., 1997; Colman et al., 2002; Santschi and Rowe, 2008; Smeaton et al., 2021c). In marine sediments both organic matter and carbonate (inorganic) material (e.g., shells or foraminifera) can be dated, but organic matter cannot be used to constrain the age of the sediment as the carbon it contains originates from multiple (many unknown) sources with varying compositions and ages. Carbonate material is therefore the preferred sample medium for 14C analysis and age constraint. Living benthic foraminifera are found in the upper most layers of most sediments and therefore the shells of foraminifera buried after death provide material ideally suited for dating as they are representative of past surface sediments from their time of deposition. The number of foraminifera required to measure 14C using a conventional AMS can vary but it is not uncommon to need between 500-1000 individuals, whereas the recent developments in AMS technology (Synal et al., 2000) means that far smaller numbers are now required (<100 individuals) with state-of-the-art systems allowing single foraminifera dating (Wacker et al., 2013; Lougheed et al., 2018). Shells can also be dated but greater care must be taken to ensure they are representative of past surficial sediments and not shells associated with mobile burrowing species (Cage and Austin, 2010; Baltzer et al., 2015).
When calibrating 14C ages derived from material from the marine environment the marine reservoir effect must be considered. The marine reservoir effect occurs when the C in tissues of organisms or inorganic deposits reflects a mix of C sources and ages (Hajdas et al., 2021). The marine reservoir effect was previously accounted for by adding 400 years to an age calibrated using the northern hemisphere calibration curve (Reimer, 2020) which led to large uncertainties. With the production of the Marine20 calibration curve (Heaton et al., 2020) the marine reservoir age can now be robustly accounted for and is directly integrated into the calibration stage. The Marine20 calibration curve has significantly improved age calibration of marine samples, but it remains a global calibration; for greater accuracy local reservoir age corrections can be applied (i.e., Cage et al., 2006) if available.
Uranium/thorium paleo-dating of biologically derived fossil carbonates
For 500 to 100,000-year timescales, thorium-230 (230Th) dating, also referred to as U/Th dating or 238U- uranium-234 (234U)-230Th dating, can be applied. This method relies on the disequilibrium due to solubility differences of uranium and thorium nuclides and involves calculating ages by studying the radioactive relationships between 238U, 234U, and 230Th (Henderson and Anderson, 2003). It is appropriate in closed carbonate systems with no initial 230Th, for example, fossil carbonates present within the terrestrial environment (e.g., rocks), but also marine carbonate formations such as corals (Cheng et al., 2000) and authigenic carbonates. It is very often used as a complementary method to 14C dating of fossil carbonates and for validating results from 14C dating.
Extending thorium-230 (230Th) dating to marine sediments is challenging mainly due to the presence of additional 230Th in materials scavenged from the seawater column. In addition, the typical levels of carbonates found in marine sediments as well as the potential post-depositional weathering, transport and mixing processes can further limit its application (Chen et al., 2020). Nevertheless, modelling and state-of-the-art analytical tools can potentially overcome some of these challenges and thus provide valuable insights into sediment accumulation rates over longer timescales. Geibert et al. (2019) have recently presented an alternative approach using a CRS model, similarly to 210Pb dating and assuming constant rate of supply of 230Th from the seawater. Although alpha spectrometry can provide accurate measurement of all relevant U and Th isotopes, these techniques require labor intensive preparation steps and lengthy counting times in comparison to mass-spectrometry coupled with a laser ablation system. These state-of-the-art techniques have become the preferred analytical option (Robinson et al., 2004; Mas et al., 2020).
Anthropogenic pressures
Only carbon which is subject to manageable “undesirable human impacts”, satisfies the blue carbon criteria of Lovelock and Duarte (2019). The vulnerability of organic carbon in marine sediments to a given disturbance is a function of the organic matter’s source (Characterising stock provenance), and recalcitrance (Characterising stock reactivity). Pressures on, or conversely management and restoration of, terrestrial or coastal ecosystems which act as remote carbon sources (carbon donors) to marine sediments will affect carbon accumulation (Santos et al., 2021). Physical disturbance of the seabed which resuspends near-surface sediments into the oxic water column will recycle labile organic carbon into the ocean and potentially impact the atmospheric carbon system (Sala et al., 2021), although it can also simply re-distribute and redeposit sediments (Rijnsdorp et al., 2021). Seabed disturbance impacts the benthic faunal community, which plays a key role in the biogeochemical processes determining what fraction of deposited carbon is recycled (remineralized) or buried (stored) (de Borger et al., 2021a; de Borger et al., 2021b).
Bottom trawling is the most spatially significant anthropogenic process physically disturbing shelf sediments (Oberle, et al., 2016b; Rijnsdorp et al., 2021), while wind farms have been shown to locally increase organic matter deposition (and recycling) because of faunal colonization of their structure (de Borger et al., 2021a). Other activities, including pipe and cable laying, aggregate extraction, and dredge disposal, are also expected to impact organic matter storage and deposition, although their impacts are more spatially restricted. Some continental shelf sediments are naturally subject to high disturbance, for example due to high-energy tidal bottom water currents (Thompson et al., 2019), which may locally dominate over bottom fishing disturbance (Diesing et al., 2013); these tend not to be sites with significant carbon stocks or accumulation (Diesing et al., 2021) and are therefore less vulnerable to anthropogenic disturbance from a carbon perspective and not good sites for the implementation of management measures.
The impacts of trawling on carbon accumulation and storage are complex and differ between shelf and deeper water slope systems (Palanques et al., 2014; Pusceddu et al., 2014; Oberle et al., 2016b; Paradis et al., 2019). See Epstein et al. (2022) for a review. They depend on the properties of the carbon stock, the type of trawling equipment and frequency of trawling, as well as sediment type and faunal community (Legge et al., 2020). More research is required to improve understanding of these factors in the context of carbon storage across a range of settings. Recent work advancing towards this goal includes that of Bradshaw et al. (2021) and Morys et al. (2021) who made field measurements of the biogeochemical impacts of trawl disturbances in the Baltic Sea, and that of de Borger et al. (2021b) who modelled biogeochemical impacts of trawling scenarios at specific sampled sites in the North Sea. Global scale modelling of trawling impact (e.g., Hiddink et al., 2021; Sala et al., 2021) remains limited by the need to make broad-scale assumptions of the underlying processes and carbon characteristics which control carbon response to pressure.
In addition to their use in geochronology (see Carbon accumulation rates), radionuclides can also be used to evidence disturbance. In an upper section of mixed (typically bioturbated) sediments, 210Pb activity is constant before being expected to decrease exponentially with depth (see Lead-210-derived sedimentation rates and dating) but this pattern is not observed where sediments have been physically disturbed. Physical seabed disturbance can also be confirmed qualitatively by measuring excess of thorium-234 (234Th). 234Th has a very short half-life (24.1 days) and is produced directly from the alpha decay of 238U in the ocean. 238U is a highly soluble and conservative radionuclide in seawater, whereas 234Th is highly particle reactive. As 234Th is preferentially adsorbed onto sinking particles, the natural secular equilibrium between the two radionuclides becomes disturbed. Consequently, an excess of 234Th is expected in the upper layers of undisturbed sediment. An anthropogenic disturbance (e.g., man-made platforms or trawling) can be qualitatively confirmed if the excess of 234Th is not as noticeable as in a separate sediment core collected nearby and known to be undisturbed (e.g., in a Marine Protected Areas; MPAs). 234Th can be determined either by beta counting or gamma spectrometry (Buesseler et al., 2001), whereas 238U is usually measured either by alpha spectrometry or ICP-MS (Forte et al., 2001).
Sampling studies investigating the impact of trawling on carbon accumulation make use of many of the analytical techniques detailed in the sections above. Oberle et al. (2016a) used 3D petroleum fingerprinting alongside 210Pb dating to quantify the depth of disturbance across sites exposed to different trawling pressure. Paradis et al., (2019; 2021) measured both 210Pb and 234Th in a comparison of trawled and un-trawled continental margin sites. Sañé et al. (2013) compared a number of biomarkers between trawled and un-trawled submarine canyon flank sites and found that labile amino acids in particular were less abundant in the disturbed sediments, providing evidence for increased carbon remineralization as a result of trawling disturbance and resulting differences in vulnerability to subsequent disturbance.
Even if the potential impact of trawling on sediment carbon at a particular site was well understood, data on trawling pressure (gear types, frequency) are often incomplete or not available at an appropriate spatial resolution. Trawling pressure maps are typically compiled from fishing GPS-based vessel track information (e.g., vessel monitoring systems, VMS) (e.g., Oberle et al., 2016b; Eigaard et al., 2017; Sala et al., 2021). These are limited by the temporal (and thus spatial) resolution of the fishing vessel location information, requiring application of interpolation techniques (see Russo et al., 2011) and resulting in estimated trawling intensity within spatial grid cells based on the number of times each was visited. Linking trawling pressure to sediment carbon is also complicated by the non-random distribution of trawling with respect to expected high carbon sites, e.g., trawling pressure tends to be higher in high-mud sediments, which are also highest in organic carbon (see Epstein et al., 2022 for further discussion).
Discussion
Gaining and improving understanding of the spatial distribution and variability of carbon stocks and accumulation rates and their vulnerability in shelf sea sediments is essential for their monitoring and management. As recent studies demonstrate (e.g., Sañé et al., 2013; Oberle et al., 2016a; Paradis et al., 2019; Paradis et al., 2021), layers of information derived from multiple analytical techniques are required in marine sediments to provide a mechanistic understanding in settings where carbon storage is physically distant from carbon sources, altered between source and sink, and disturbed by anthropogenic activities. We have outlined an extensive multidisciplinary toolbox of analysis techniques which are needed to achieve this understanding, summarized in Table 3.
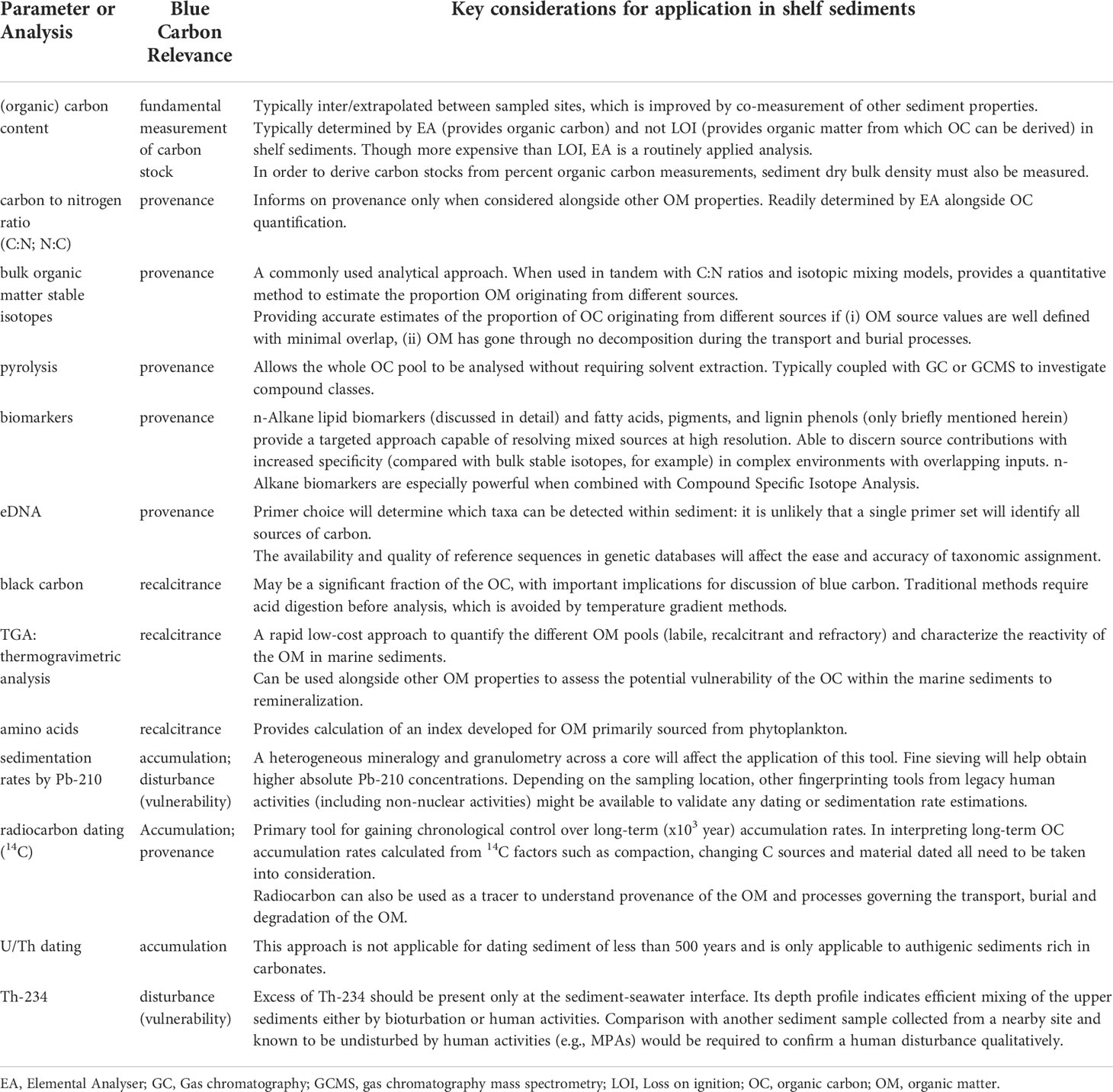
Table 3 Summary of analyses presented and considerations for their application to improving understanding of blue carbon in continental shelf sediments.
The choice of techniques applied by any study will be determined by its specific research questions, and also by available resources. Table 3 provides a starting point with which to approach this decision. Where possible a stepwise approach to analyses should be applied by which initial parameters are analysed to inform which samples, if any, will provide information of interest from more resource-intensive analyses. It is important to distinguish between routine measurements and analyses (e.g., particle grain size, total organic carbon and C:N ratios) and emerging techniques and those which have not traditionally been applied in shelf sediments (e.g., eDNA, compound specific isotopic analyses) where ongoing method development must be considered.
Studies should aim to characterize the system by measuring more than the minimum parameters wherever possible to inform a process-level understanding. Associated explanatory parameters such as bottom water properties and pore water chemistry can allow modelling approaches to be applied, adding value to what can be obtained by measurements alone. Biogeochemical modelling can be used in two ways; to help interpret observations, and to allow scaling up and scenario exploration (for example of climate change). However, a clear understanding of which analytical outputs are needed to model a given scenario and which analytical tools can provide these outputs is needed to fully exploit modelling approaches. For example, scenario studies describing the vulnerability of carbon release to a given a physical disturbance rely on knowledge of the lability of sediment carbon which must come from observation.
To assess the role of continental shelf sediments in the blue carbon framework, the analyses outlined herein – addressing the fundamental blue carbon questions of stock (its abundance, provenance, recalcitrance) and accumulation rate, and vulnerability – must be considered holistically. A key outstanding question is linking measurements to the physical and biogeochemical processes which control carbon stock and accumulation.
As studies increasingly address the knowledge gaps around continental shelf carbon stocks and accumulation – through both sampling and modelling – the management of this carbon with respect to human pressures will become the key question for understanding where it fits within the blue carbon framework and within the climate mitigation discourse (e.g., Christianson et al., 2022). Can the biogeochemical processes which mediate carbon cycling in near-surface sediments be controlled or protected such that carbon burial is maximized? Improved process understanding is required. Controlling trawling and other human activities which disturb the seabed where it acts as a carbon store appears to be a quick win, yet the impacts of disturbed sediment can extend beyond the trawled area on a regional scale (Palanques et al., 2014). If MPAs are to be used as a management tool to protect carbon stocks and accumulation in continental margin sediments (e.g., Roberts et al., 2017; Sala et al., 2021), their locations must be informed by well-mapped carbon stock and accumulation (e.g., Diesing et al., 2021) and process-level understanding (e.g., de Borger et al., 2021b).
Conclusions
The marine environment stores organic carbon in offshore as well as coastal ecosystems. In traditional coastal blue carbon habitats (saltmarsh, seagrass, mangrove) organic carbon is locally produced. Organic carbon in marine sediment is more allochthonous: it has largely been transported from terrestrial, coastal or near-surface sources and has been more significantly altered from its original form during transport and after deposition in near-surface sediments. Organic carbon stock and accumulation rates are smaller locally in subtidal marine sediment than in coastal blue carbon habitats, but larger when their expansive spatial extent is considered. Shelf sea sediments are subject to different anthropogenic pressures, most notably that of disturbance from bottom trawling, and require different carbon management approaches.
To establish if organic carbon stored in continental shelf sediments contributes to climate change mitigation in a manageable way, thus meeting the criteria for blue carbon, organic carbon stock and accumulation rates must be determined alongside carbon properties which inform on its sources and stability and control its vulnerability. A multitude of analyses, brought together here as a toolbox, can be applied to answer these questions. Some analyses are already routinely applied in shelf sea sediments (e.g., percent of organic carbon content) while others continue to be developed for offshore sediment blue carbon application (e.g., eDNA). However, even for the relatively simple determination of stock, lack of co-measured dry bulk density or porosity is often a barrier to including legacy data in calculations and spatial modelling is needed to integrate limited observations and provide a holistic picture. The field is advancing rapidly, with the application of eDNA to link coastal carbon sources to shelf sediment recipient sites and novel carbon reactivity indices being defined to inform on vulnerability. Consistency in methodology between studies through application of the toolbox we present will improve comparability and facilitate data aggregation to develop broad scale understanding.
The link between carbon stored in marine sediments, even at shelf sea water depths, and the atmospheric carbon system is moderated by the oceanic carbon cycle. More sediment carbon data collected alongside explanatory parameters (such as temperature and nutrient concentrations) are needed to better constrain the complex biogeochemical controls on the connectivity between these systems in the context of climate mitigation considerations.
Author contributions
RP and LB provided initial conceptualization of this work. CAG led a workshop attended by all authors (except SKW) where the manuscript was initiated. Workshop discussions provided the basis for the review. CAG facilitated subsequent manuscript drafting and editing. CAG, JA, FDM, VGF, NH, CH, PDL, CM, CS, SKW, and CW drafted sections of the text. All authors contributed to manuscript revision, read, and approved the submitted version.
Acknowledgments
This work was supported by Cefas internal Seedcorn self-investment funding under the project DP440: Blue carbon within climate mitigation and ecosystem service approaches to natural asset assessments, and by Cefas’ Ecosystem Theme science theme. We would like to thank two reviewers and editor Tim Rixen for their constructive comments which improved this review.
Conflict of interest
The authors declare that the research was conducted in the absence of any commercial or financial relationships that could be construed as a potential conflict of interest.
Publisher’s note
All claims expressed in this article are solely those of the authors and do not necessarily represent those of their affiliated organizations, or those of the publisher, the editors and the reviewers. Any product that may be evaluated in this article, or claim that may be made by its manufacturer, is not guaranteed or endorsed by the publisher.
Footnotes
References
Ahmad F., Morris K., Law G. T. W., Taylor K. G., Shaw S. (2021). Fate of radium on the discharge of oil and gas produced water to the marine environment. Chemosphere 273, 129550. doi: 10.1016/j.chemosphere.2021.129550
Alderson D. M., Evans M. G., Rothwell J. J., Boult S. (2016). Classifying sedimentary organics: It is a matter of quality rather than quantity. Prog. Phys. Geogr.: Earth Environ. 40 (3), 450–479. doi: 10.1177/0309133315625864
Aldridge J. N., Lessin G., Amoudry L. O., Hicks N., Hull T., Klar J. K., et al. (2017). Comparing benthic biogeochemistry at a sandy and a muddy site in the celtic sea using a model and observations. Biogeochemistry 135 (1–2), 155–182. doi: 10.1007/s10533-017-0367-0
Anderson E. C., Libby W. F., Weinhouse S., Reid A. F., Kirshenbaum A. D., Grosse A. V. (1947). Natural radiocarbon from cosmic radiation. Phys. Rev. 72, 931–936. doi: 10.1103/PhysRev.72.931
Appleby P. G., Oldfield F. (1978). The calculation of lead-210 dates assuming a constant rate of supply of unsupported 210Pb to the sediment. Catena 5 (1), 1–8. doi: 10.1016/S0341-8162(78)80002-2
Aquino-López M. A., Sanderson N. K., Blaauw M., Sanchez-Cabeza J.-A., Ruiz-Fernandez A. C., Aquino-López J., et al. (2020) A simulation study to compare 210Pb dating data analyses. Available at: http://arxiv.org/abs/2012.06819.
Arias-Ortiz A., Masqué P., Garcia-Orellana J., Serrano O., Mazarrasa I., Marbá N., et al. (2018). Reviews and syntheses: 210Pb-derived sediment and carbon accumulation rates in vegetated coastal ecosystems - setting the record straight. Biogeosciences 15 (22), 6791–6818. doi: 10.5194/bg-15-6791-2018
Arndt S., Jørgensen B. B., LaRowe D. E., Middelburg J. J., Pancost R., Regnier D. P. (2013). Quantifying the degradation of organic matter in marine sediments: A review and synthesis. Earth-Sci. Rev. 123, 53–86. doi: 10.1016/j.earscirev.2013.02.008
Atwood T. B., Witt A., Mayorga J., Hammill E., Sala E. (2020). Global patterns in marine sediment carbon stocks. Front. Mar. Sci. 7. doi: 10.3389/fmars.2020.00165
Ausín B., Bruni E., Haghipour N., Welte C., Bernasconi S. M., Eglinton T. I. (2021). Controls on the abundance, provenance and age of organic carbon buried in continental margin sediments. Earth Planet. Sci. Lett. 558, 116759. doi: 10.1016/j.epsl.2021.116759
Avelar S., van der Voort T. S., Eglinton T. I. (2017). Relevance of carbon stocks of marine sediments for national greenhouse gas inventories of maritime nations. Carbon Balance Manage. 12:10. doi: 10.1186/s13021-017-0077-x
Baltar F., Alvarez-Salgado X. A., Arístegui J., Benner R., Hansell D. A., Herndl G. J., et al. (2021). What is refractory organic matter in the ocean? Front. Mar. Sci. 8: 642637. doi: 10.3389/fmars.2021.642637
Baltzer A., Mokeddem Z., Goubert E., Lartaud F., Labourdette N., Fournier J., Bourillet J-F. (2015). “‘The “Turritella Layer”: A Potential Proxy of a Drastic Holocene Environmental Change on the North–East Atlantic Coast’,” in Sediment fluxes in coastal areas Eds:Maanan M., Robin M. (Dordrecht:Springer), 3–21.
Baskaran M., Bianchi T. S., Filley T. (2017). Inconsistencies between 14C and short-lived radionuclides-based sediment accumulation rates: effects of long-term remineralization. J. Environ. Radioact. 174, 10–16. doi: 10.1016/j.jenvrad.2016.07.028
Becker R., Altmann K., Sommerfeld T., Braun U. (2020). Quantification of microplastics in a freshwater suspended organic matter using different thermoanalytical methods – outcome of an interlaboratory comparison. J. Anal. Appl. Pyrolysis 148, 104829. doi: 10.1016/j.jaap.2020.104829
Bennett C. L., Beukens R. P., Clover M. R., Gove H. E., Liebert R. B., Litherlan A. E., et al. (1977). Radiocarbon dating using electrostatic accelerators: Negative ions provide the key. Science 198, 508–510. doi: 10.1126/science.198.4316.508
Beulig F., Røy H., Glombitza C., Jørgensen B. B. (2017). Control on rate and pathway of anaerobic organic carbon degradation in the seabed. Proc. Natl. Acad. Sci. U. S. A. 115 (2), 367–372. doi: 10.1073/pnas.1715789115
Bianchi T. S., Canuel E. A. (2011). Chemical biomarkers in aquatic ecosystems (Princeton, NJ:Princeton University Press). doi: 10.23943/princeton/9780691134147.001.0001
Bird M. I., Fifield L. K., Chua S., Goh B. (2004). Calculating sediment compaction for radiocarbon dating of intertidal sediments. Radiocarbon 46, 421–435. doi: 10.1017/S0033822200039734
Blaauw M. (2010). Methods and code for “classical” age-modelling of radiocarbon sequences. Quaternary Geochronol. 5 (5), 512–518. doi: 10.1016/j.quageo.2010.01.002
Blaauw M., Aquino-Lopez M., Christen J. A. (2021). “‘Bayesian improvements to 210Pb dating’,” EGU general assembly, online, 19-30 Apr 2021, EGU21–E1596. doi: 10.5194/egusphere-egu21-1596
Blaauw M., Christen J. A. (2011). Flexible paleoclimate age-depth models using an autoregressive gamma process. Bayesian Anal. 6 (3), 457–474. doi: 10.1214/11-BA618
Blaauw M., Christen J. A., Bennett K. D., Reimer P. J. (2018). Double the dates and go for Bayes — Impacts of model choice, dating density and quality on chronologies. Quaternary Sci. Rev. 188, 58–66. doi: 10.1016/j.quascirev.2018.03.032
Blattmann T. M., Montluçon D. B., Haghipour N., Ishikawa N. F., Eglinton T. I. (2020). Liquid chromatographic isolation of individual amino acids extracted from sediments for radiocarbon analysis. Front. Mar. Sci. 7,174. doi: 10.3389/fmars.2020.00174
Blattmann T. M., Ishikawa N. F. (2020). Theoretical amino acid-specific radiocarbon content in the environment: Hypotheses to be tested and opportunities to be taken. Front. Mar. Sci. 7, 302. doi: 10.3389/fmars.2020.00302
Bradshaw C., Jakobsson M., Brüchert V., Bonaglia S., Mörth C. M., Muchowski J., et al. (2021). Physical disturbance by bottom trawling suspends particulate matter and alters biogeochemical processes on and near the seafloor. Front. Mar. Sci. 8, 683331. doi: 10.3389/fmars.2021.683331
Bray E. E., Evans E. D. (1961). Distribution of n-paraffins as a clue to recognition of source beds. Geochim. Cosmochim. Acta 22 (1), 2–15. doi: 10.1016/0016-7037(61)90069-2
Brodie C. R., Leng M. J., Casford J. S. L., Kendrick C. P., Lloyd J. M., Yongqiang Z, et al. (2011). Evidence for bias in C and N concentrations and δ13C composition of terrestrial and aquatic organic materials due to pre-analysis acid preparation methods. Chem. Geol. 282 (3–4), 67–83. doi: 10.1016/j.chemgeo.2011.01.007
Bruce K., Blackman R., Bourlat S., Hellström A., Bakker J., Bista I., et al. (2021). “A practical guide to DNA-based methods for biodiversity assessment”. Advanced books. doi: 10.3897/ab.e68634
Buesseler K. O., Benitez-Nelson C., Rutgers van der Loeff M., Andrews J., Ball L., Crossin G., Charette M. A. (2001). An intercomparison of small- and large-volume techniques for thorium-234 in seawater. Mar. Chem. 74 (1), 15–28. doi: 10.1016/S0304-4203(00)00092-X
Burdige D. J. (2007). Preservation of organic matter in marine sediments: controls, mechanisms, and an imbalance in sediment organic carbon budgets? Chem. Rev. 107 (2), 467–485.
Burrows M. T., Kamenos N. A., Hughes D. J., Stahl H., Howe J. A., Tett P. (2014). Assessment of carbon budgets and potential blue carbon stores in scotland’s coastal and marine environment. Project Report. Scottish Natural Heritage Commissioned Report No. 761.
Butenschön M., Clark J., Aldridge J. N., Icarus Allen J., Artioli Y., Blackford J., et al. (2016). ERSEM 15.06: A generic model for marine biogeochemistry and the ecosystem dynamics of the lower trophic levels. Geosci. Model. Dev. 9 (4), 1293–1339. doi: 10.5194/gmd-9-1293-2016
Cage A. G., Austin W. E. N. (2010). Marine climate variability during the last millennium: The Loch Sunart record, Scotland, UK. Quaternary Sci. Rev. 29 (13–14), 1633–1647. doi: 10.1016/j.quascirev.2010.01.014
Cage A. G., Davies S. M., Wastegård S., Austin W. E. N. (2011). Identification of the Icelandic Landnám tephra (AD 871±2) in Scottish fjordic sediment. Quaternary Int. 246, 168–176. doi: 10.1016/j.quaint.2011.08.016
Cage A. G., Heinemeier J., Austin W. E. N. (2006). Marine radiocarbon reservoir ages in scottish coastal and fjordic waters. Radiocarbon 48, 31–43. doi: 10.1017/S0033822200035372
Canfield D. E. (1994). Factors influencing organic carbon preservation in marine sediments. Chem. Geol. 114 (3–4), 315–329. doi: 10.1016/0009-2541(94)90061-2
Capel E. L., de la Rosa Arranz J. M., González-Vila F. J., González-Perez J. A., Manning D. A. C. (2006). Elucidation of different forms of organic carbon in marine sediments from the atlantic coast of spain using thermal analysis coupled to isotope ratio and quadrupole mass spectrometry. Org. Geochem. 37 (12), 1983–1994. doi: 10.1016/j.orggeochem.2006.07.025
Chen C. Y., McGee D., Woods A., Pérez L., Hatfield R. G., Edwards R. L., et al. (2020). U-th dating of lake sediments: Lessons from the 700 ka sediment record of lake junín, peru. Quaternary Sci. Rev. 244, 106422. doi: 10.1016/j.quascirev.2020.106422
Chen Y., Hu C., Yang G. P., Gao X. C. (2021). Source, distribution and degradation of sedimentary organic matter in the South Yellow Sea and East China Sea. Estuar. Coast. Shelf Sci. 255, 107372. doi: 10.1016/j.ecss.2021.107372
Cheng H., Adkins J., Edwards R. L., Boyle E. A. (2000). U-Th dating of deep-sea corals. Geochim. Cosmochim. Acta 64 (14), 2401–2416. doi: 10.1016/S0016-7037(99)00422-6
Chew S. T., Gallagher J. B. (2018). Accounting for black carbon lowers estimates of blue carbon storage services. Sci. Rep. 8(2553), 1–8. doi: 10.1038/s41598-018-20644-2
Christianson A. B., Cabré A., Bernal B., Baez S. K., Leung S., Pérez-Porro A., et al. (2022). The promise of blue carbon climate solutions: Where the science supports ocean-climate policy. Front. Mar. Sci. 9, 851448. doi: 10.3389/fmars.2022.851448
Clark K., Karsch-Mizrachi I., Lipman D. J., Ostell J., Sayers E. W. (2016). GenBank. Nucleic Acids Res. 44, D67–D72. doi: 10.1093/nar/gkv1276
Colman S. M., Baucom P. C., Bratton J. F., Cronin T. M., McGeehin J. P., Willard D., et al. (2002). Radiocarbon dating, chronologic framework, and changes in accumulation rates of holocene estuarine sediments from Chesapeake Bay. Quaternary Res. 57 (1), 58–70. doi: 10.1006/qres.2001.2285
Cook G. T., MacKenzie A. B., Muir G. K.P., Mackie G., Gulliver P. (2004). Sellafield-derived anthropogenic 14C in the marine intertidal environment of the NE Irish Sea. Radiocarbon 46 (2), 877–883. doi: 10.1017/S0033822200035918
Coppola A. I., Ziolkowski Lori A., Masiello C. A., Druffel E. R. M. (2014). Aged black carbon in marine sediments and sinking particles. Geophys.ical Res. Lett. 41, 2427–2433. doi: 10.1002/2013GL059068
Coppola A. I., Seidel M., Ward N. D., Viviroli D., Nascimento G. S., Haghipour N. (2019). Carbon pools. Nat. Commun. 10, 4018. doi: 10.1038/s41467-019-11543-9
Dal Molin F., Warwick P. E., Read D. (2018). Under-estimation of 210Pb in industrial radioactive scales. Anal. Chim. Acta 1000, 67–74. doi: 10.1016/j.aca.2017.08.037
Dang H. (2020). Grand challenges in microbe-driven marine carbon cycling research. Front. Microbiol. 11. doi: 10.3389/fmicb.2020.01039
Darby D. A., Bischof J. F., Jones G. A. (1997). Radiocarbon chronology of depositional regimes in the western Arctic Ocean. Deep Sea Res. Part II: Top. Stud. Oceanogr. 44 (8), 1745–1757. doi: 10.1016/S0967-0645(97)00039-8.
d’Auriac M. A., Hancke K., Gundersen H., Frigstad H., Borgersen G. (2021). Blue carbon eDNA – a novel eDNA method to trace macroalgae carbon in marine sediments (Oslo, Norway: Niorwegian Institute for Water Research (NIWA). doi: 10.13140/RG.2.2.10631.93607
Dauwe B., Middelburg J. J., Herman P. M. J., Heip C. H. R. (1999). Linking diagenetic alteration of amino acids and bulk organic matter reactivity. Limnol. Oceanogr. 44 (7), 1809–1814. doi: 10.4319/lo.1999.44.7.1809
Dauwe B., Middelburg J. J. (1998). Amino acids and hexosamines as indicators of organic matter degradation state in North Sea sediments. Limnol. Oceanogr. 43 (5), 782–798. doi: 10.4319/lo.1998.43.5.0782
Davies S. M. (2015). Cryptotephras: The revolution in correlation and precision dating. J. Quaternary Sci. 30(2)114–130. doi: 10.1002/jqs.2766
de Borger E., Ivanov E., Capet A., Braeckman U., Vanaverbeke J., Grégoire M., Soetaert K. (2021a). Offshore windfarm footprint of sediment organic matter mineralization processes. Front. Mar. Sci. 8,632243. doi: 10.3389/fmars.2021.632243
de Borger E., Tiano J., Braeckman U., Rijnsdorp A. D., Soetaert K. (2021b). Impact of bottom trawling on sediment biogeochemistry: A modelling approach. Biogeosciences 18, 2539–2557. doi: 10.5194/bg-18-2539-2021
de Haas H., Boer W., Van Weering T. C. E. (1997). Recent sedimentation and organic carbon burial in a shelf sea: The North Sea. Mar. Geol. 144 (1–3), 131–146. doi: 10.1016/S0025-3227(97)00082-0
Deiner K., Bik H. M., Mächler E., Seymour M., Lacoursière-Roussel A., Altermatt F., et al. (2017). Environmental DNA metabarcoding: Transforming how we survey animal and plant communities. Mol. Ecol. 26 (21), 5872–5895. doi: 10.1111/mec.14350
Derrien M., Brogi S. R., Gonçalves-Araujo R. (2019). Characterization of aquatic organic matter: Assessment, perspectives and research priorities. Water Res. 163, 114908. doi: 10.1016/j.watres.2019.114908
Derrien M., Yang L., Hur J. (2017). Lipid biomarkers and spectroscopic indices for identifying organic matter sources in aquatic environments: A review. Water Res. 112, 58–71. doi: 10.1016/j.watres.2017.01.023
Diesing M., Kröger S., Parker R., Jenkins C., Mason C., Weston K. (2017). Predicting the standing stock of organic carbon in surface sediments of the North–West European continental shelf. Biogeochemistry 135 (1–2),183–200. doi: 10.1007/s10533-017-0310-4
Diesing M., Stephens D., Aldridge J. (2013). A proposed method for assessing the extent of the seabed significantly affected by demersal fishing in the Greater North Sea. ICES J. Mar. Sci. 70 (6), 1085–1096. doi: 10.1093/icesjms/fst066
Diesing M., Thorsnes T., Rún Bjarnadóttir L. (2021). Organic carbon densities and accumulation rates in surface sediments of the North Sea and Skagerrak. Biogeosciences 18 (6), 2139–2160. doi: 10.5194/bg-18-2139-2021
Doetterl S., Berhe A. A., Nadeu E., Wang Z., Sommer M., Fiener P. (2016). Erosion, deposition and soil carbon: A review of process-level controls, experimental tools and models to address C cycling in dynamic landscapes. Earth-Sci. Rev. 154, 102–122. doi: 10.1016/j.earscirev.2015.12.005
Dolliver J., Connor N. O. (2022). Whole system analysis is required to determine the fate of macroalgal carbon: A systematic review. J. Phycol. 58(3), 364–376. doi: 10.1111/jpy.13251
Duarte C. M., Gattuso J.-P., Hancke K., Gundersen H., Filbee-Dexter K., Pedersen M. F., et al. (2022). Global estimates of the extent and production of macroalgal forests. Global Ecol. Biogeogr.31(7), 1422–1439 doi: 10.1111/geb.13515
Eigaard O. R., Bastardie F., Hintzen N. T., Buhl-Mortensen L., Buhl-Mortensen P., Catarino R., et al. (2017). The footprint of bottom trawling in european waters: Distribution, intensity, and seabed integrity. ICES J. Mar. Sci. 74 (3), 847–865. doi: 10.1093/icesjms/fsw194
Elbrecht V., Peinert B., Leese F. (2017). Sorting things out assessing effects of unequal specimen biomass on DNA. Ecol. Evol. 7, 6918–6926. doi: 10.1002/ece3.3192
Environment Agency, Food Standards Agency, Food Standards Scotland, Northern Ireland Environment Agency, Natural Resources Wales, Scottish Environment Protection Agency (2021). Radioactivity in Food and the Environment 2020. RIFE 26. EA, FSA, FSS, NIEA, NRW and SEPA, Bristol, London, Aberdeen, Belfast, Cardiff and Stirling.
Epstein G., Middelburg J. J., Hawkins J. P., Norris C. R., Roberts C. M. (2022). The impact of mobile demersal fishing on carbon storage in seabed sediments. Global Change Biol., 28(9), 2875–2894. doi: 10.1111/gcb.16105
Fang Z., Yang W., Chen M., Zheng M., Hu W. (2016). Abundance and sinking of particulate black carbon in the western Arctic and Subarctic Oceans. Sci. Rep. 6(29959), 1–11. doi: 10.1038/srep29959
Ficetola G. F., Miaud C., Pompanon F., Taberlet P. (2008). Species detection using environmental DNA from water samples. Biol. Lett. 4 (4), 423–425. doi: 10.1098/rsbl.2008.0118
Ficetola G. F., Pansu J., Bonin A., Coissac E., Giguet-Covex C., De Barba M., et al. (2015). Replication levels, false presences and the estimation of the presence/absence from eDNA metabarcoding data. Mol. Ecol. Resour. 15 (13), 543–556. doi: 10.1111/1755-0998.12338
Filbee-Dexter K., Pedersen M. F., Fredriksen S., Norderhaug K. M., Rinde E., Kristiansen T., et al. (2020). Carbon export is facilitated by sea urchins transforming kelp detritus. Oecologia 192 (1), 213–225. doi: 10.1007/s00442-019-04571-1
Fonseca V. G. (2018). Pitfalls in relative abundance estimation using edna metabarcoding. Mol. Ecol. Resour. 18 (5), 923–926. doi: 10.1111/1755-0998.12902
Fonseca V. G., Sinniger F., Gaspar J. M., Quince C., Creer S., Power D. M., et al. (2017). Revealing higher than expected meiofaunal diversity in Antarctic sediments: A metabarcoding approach. Sci. Rep. 7 (1), 1–11. doi: 10.1038/s41598-017-06687-x
Fonseca V. G., Lallias D. (2016). Metabarcoding marine sediments: Preparation of amplicon libraries. Methods Mol. Biol. 1452, 183–196. doi: 10.1007/978-1-4939-3774-5_12
Forte M., Rusconi R., Margini C., Abbate G., Maltese S., Badalamenti P., Bellinzona S. (2001). Determination of uranium isotopes in food and environmental samples by different techniques: A comparison. Radiat. Prot. Dosimetry 97 (4), 325–328. doi: 10.1093/oxfordjournals.rpd.a006681
Garcia-Tenorio R., Rozmaric M., Harms A., Godoy J. M.D.O., Barsanti M., Schirone A., et al. (2020). From radiometry to chronology of a marine sediment core: A 210Pb dating interlaboratory comparison exercise organised by the IAEA. Mar. Pollut. Bull. 159, 111490. doi: 10.1016/j.marpolbul.2020.111490
Geibert W., Stimac I., Rutgers van der Loeff M. M., Kuhn G. (2019). Dating deep-sea sediments with 230Th excess using a constant rate of supply model. Paleoceanography Paleoclimatology 34 (12), 1895–1912. doi: 10.1029/2019PA003663
Geraldi N. R., Ortega A., Serrano O., Macreadie P. I., Lovelock C. E., Krause-Jensen D., et al. (2019). Fingerprinting blue carbon: Rationale and tools to determine the source of organic carbon in marine depositional environments. Front. Mar. Sci. 6. doi: 10.3389/fmars.2019.00263
Geraldi N. R., Díaz-Rúa R., Shea L. A., Duarte C. M. (2020). Performance of extraction methods for extracellular DNA from sediments across marine habitats. Environ. DNA 2 (1), 91–98. doi: 10.1002/edn3.48
Gilbey J., Carvalho G., Castilho R., Coscia I., Coulson M. W., Dahle G., et al. (2021). Life in a drop: Sampling environmental DNA for marine fishery management and ecosystem monitoring. Mar. Policy 124, 104331. doi: 10.1016/j.marpol.2020.104331
Glaser B., Haumaier L., Guggenberger G., Zech W. (1998). Black carbon in soils: The use of benzenecarboxylic acids as specific markers. Org. Geochem. 29 (4), 811–819. doi: 10.1016/S0146-6380(98)00194-6
Goidts E., Van Wesemael B., Crucifix M. (2009). Magnitude and sources of uncertainties in soil organic carbon (SOC) stock assessments at various scales. Eur. J. Soil Sci. 60 (5), 723–739. doi: 10.1111/j.1365-2389.2009.01157.x
Gordon E. S., Goni M. A. (2003). Sources and distribution of terrigenous organic matter delivered by the Atchafalaya river to sediments in the northern Gulf of Mexico. Geochim. Cosmochim. Acta 67 (13), 2359–2375. doi: 10.1016/S0016-7037(02)01412-6
Gregg R., Elias J. L., Alonso I., Crosher I. E., Muto P., Morecroft M. D. (2021). Carbon storage and sequestration by habitat: A review of the evidence (second edition) Natural England Research Report NERR094. in Natural England research report.
Grey E. K., Bernatchez L., Cassey P., Deiner K., Deveney M., Howland K. L., et al. (2018). Effects of sampling effort on biodiversity patterns estimated from environmental DNA metabarcoding surveys. Sci. Rep. 8 (1), 2–11. doi: 10.1038/s41598-018-27048-2
Gulliver P., Cook G. T., MacKenzie A. B., Naysmith P., Anderson R. (2001). Transport of Sellafield-derived 14C from the Irish Sea through the North Channel. Radiocarbon 43 (2B), 869–877. doi: 10.1017/S0033822200041539
Guo L., Semiletov I., Gustafsson Ö., Ingri J., Andersson P., Dudarev O., White D. (2004). Characterization of Siberian Arctic coastal sediments: Implications for terrestrial organic carbon export. Global Biogeochem. Cycles 18 (1), GB1036. doi: 10.1029/2003gb002087
Guo L., White D. M., Xu C., Santschi P. H. (2009). Chemical and isotopic composition of high-molecular-weight dissolved organic matter from the Mississippi River plume. Mar. Chem. 114 (3–4), 63–71. doi: 10.1016/j.marchem.2009.04.002
Gustafsson Ö., Bucheli T. D., Kukulska Z., Andersson M., Largeau C., Rouzaud J. N., et al. (2001). Evaluation of a protocol for the quantification of black carbon in sediments. Global Biogeochem. Cycles 15 (4), 881–890. doi: 10.1029/2000GB001380
Haghipour N., Ausín B., Usman M. O., Ishikawa N., Wacker L., Welte C., et al. (2019). Compound- specific radiocarbon analysis by elemental analyzer–accelerator mass spectrometry: Precision and limitations. Anal. Chem. 91, 2042–2049. doi: 10.1021/acs.analchem.8b04491
Hajdas I., Ascough P., Garnett M. H., Fallon S. J., Pearson C. L., Quarta G., et al. (2021). Radiocarbon dating. Nat. Rev. Methods Primers 1, 1–26. doi: 10.1038/s43586-021-00058-7
Hammes K., Schmidt M. W.I., Smernik R. J., Currie L. A., Ball W. P., Nguyen T. H., et al. (2007). Comparison of quantification methods to measure fire-derived (black-elemental) carbon in soils and sediments using reference materials from soil, water, sediment and the atmosphere. Global Biogeochem. Cycles 21 (3),GB3016. doi: 10.1029/2006GB002914
Hansell D. A. (2013). Recalcitrant dissolved organic carbon fractions. Annu. Rev. Mar. Sci. 5, 421–425. doi: 10.1146/annurev-marine-120710-100757
Harris D., Horwáth W. R., Van Kessel C. (2001). Acid fumigation of soils to remove carbonates prior to total organic carbon or carbon-13 isotopic analysis. Soil Sci. Soc. America J. 65, 1853–1856. doi: 10.2136/sssaj2001.1853
Harrison J. B., Sunday J. M., Rogers S. M. (2019). Predicting the fate of eDNA in the environment and implications for studying biodiversity. Proc. R. Soc. B: Biol. Sci. 286 (1915), 20191409. doi: 10.1098/rspb.2019.1409
Hassen N. E. H., Reguigui N., Helali M. A., Mejjad N., Laissaoui A., Benkdad A., et al. (2020). Evaluating the historical sedimentation patterns in two different Mediterranean deep environments (Sardinia and Sicily Channels). Mediterr. Mar. Sci. 20 (3), 542–548. doi: 10.12681/mms.19558.
Heaton T., Blaauw M., Blackwell P., Ramsey C. B., Reimer P., Scott E. (2020). The IntCal20 approach to radiocarbon calibration curve construction: A new methodology using bayesian splines and errors-in-Variables. Radiocarbon 62 (4), 821–863. doi: 10.1017/RDC.2020.46
Henderson G. M., Anderson R. F. (2003). The U-series toolbox for paleoceanography. Uranium-series Geochem. 52, 493–531. doi: 10.1515/9781501509308-017
Hiddink J. G., van de Welde S. J., McConnaughey R. A., de Broger E., O'Neill F. G. (2021). ‘Quantifying the carbon benefits of ending bottom trawling’. Available at: https://figshare.com/articles/preprint/Quantifying_the_carbon_benefits_of_ending_bottom_trawling/16722808.
Hogg A. G., Heaton T. J., Hua Q., Palmer J. G., Turney C. S., Southon J., et al. (2020). SHCal20 southern hemisphere calibration, 0–55,000 years cal BP. Radiocarbon 62 (4), 759–778. doi: 10.1017/RDC.2020.59
Howard J., Hoyt S., Isensee K., Telszewski M., Pidgeon E. (eds.) (2014). Coastal Blue Carbon: Methods for assessing carbon stocks and emissions factors in mangroves, tidal salt marshes, and seagrasses.Arlington, Virginia, USA:Conservation International, Intergovernmental Oceanographic Commission of UNESCO, International Union for Conservation of Nature.
Hua Q., Barbetti M., Rakowski A. Z. (2013). Atmospheric radiocarbon for the period 1950–2010. Radiocarbon 55 (4), 2059–2072. doi: 10.2458/azu_js_rc.v55i2.16177
Humphreys M. P., Achterberg E. P., Hopkins J. E., Chowdhury M. Z. H., Griffiths A. M., Hartman S. E., et al. (2019). Mechanisms for a nutrient-conserving carbon pump in a seasonally stratified, temperate continental shelf sea. Prog. Oceanogr. 177, 101961. doi: 10.1016/j.pocean.2018.05.001
Hunt C., Demšar U., Dove D., Smeaton C., Cooper R., Austin W. E. N. (2020). Quantifying marine sedimentary carbon: A new spatial analysis approach using seafloor acoustics, imagery, and ground-truthing data in Scotland. Front. Mar. Sci. 7, 588. doi: 10.3389/fmars.2020.00588
IAEA (2010). Analytical methodology for the determination of radium isotopes in environmental samples (Vienna, Austria: IAEA).
IPCC (2008). ‘IPCC guidelines for national greenhouse gas inventories–a primer.’. Ed. Eggleston H. S., et al. (Japan: National Greenhouse Gas Inventories Programme).
IPCC (2013). 2013 Supplement to the 2006 IPCC guidelines for national greenhouse gas inventories: Wetlands. Ed. Hiraishi T., Krug T., Tanabe K., Srivastava N., Baasansuren J., Fukuda M., et al. (Switzerland: IPCC).
Jablonski S. A., Mecray E. L., Munson J. M., Blackwood D. S. (2002). Geochemical sediment analysis procedures. Available at: https://pubs.usgs.gov/of/2002/of02-371/. (Accessed August 2022)
Jenkins C. (2005). ‘Summary of the on CALCULATION methods used in dbSEABED’. Ed. Buczkowski B. J., Reid J. A., Jenkins C. J., Reid J. M., Williams S. J., Flocks J. G. (Gulf of Mexico and Caribbean(Puerto Rico and U.S. Virgin Islands):Offshore Surficial Sediment Data Release: U.S. Geological Survey DataSeries 146.
Jenkins C. (2018). Sediment accumulation rates for the Mississippi Delta region: A time-interval synthesis. J. Sediment. Res. 88 (2), 301–309. doi: 10.2110/jsr.2018.15
Jo T., Minamoto T. (2021). Complex interactions between environmental DNA (eDNA) state and water chemistries on eDNA persistence suggested by meta-analyses. Mol. Ecol. Resour. 21 (5), 1490–1503. doi: 10.1111/1755-0998.13354
Kaloustian J., Pauli A. M., Pastor J. (2001). Kinetic study of the thermal decompositions of biopolymers extracted from various plants. J. Therm. Anal. Calorim. 63, 7–20. doi: 10.1023/A:1010199831895
Kershaw P. (1986). Radiocarbon dating of Irish Sea sediments. Estuar. Coast. Shelf Sci. 23 (2), 295–303. doi: 10.1016/0272-7714(86)90029-6
Kharbush J. J., Close H. G., Van Mooy B. A. S., Arnosti C., Smittenberg R. H., Le Moigne F. A. C., et al. (2020). Particulate organic carbon deconstructed: Molecular and chemical composition of particulate organic carbon in the ocean. Front. Mar. Sci. 7. doi: 10.3389/fmars.2020.00518
Kleber M., Bourg I. C., Coward E. K., Hansel C. M., Myneni S. C. B., Nunan N. (2021). Dynamic interactions at the mineral–organic matter interface. Nat. Rev. Earth Environ. 2, 402–421. doi: 10.1038/s43017-021-00162-y
Kokubu Y., Rothäusler E., Filippi J. B., Durieux E. D. H., Komatsu T. (2019). Revealing the deposition of macrophytes transported offshore: Evidence of their long-distance dispersal and seasonal aggregation to the deep sea. Sci. Rep. 9 (1), 4331. doi: 10.1038/s41598-019-39982-w
Komada T. (2008). Carbonate removal from coastal sediments for the determination of organic carbon and its isotopic signatures, δ13C and Δ14C: Comparison of fumigation and direct acidification by hydrochloric acid. Limnol. Oceanogr. Methods 6(6), 254–262. doi: 10.4319/lom.2008.6.254
Krause-Jensen D., Duarte C. M. (2016). Substantial role of macroalgae in marine carbon sequestration. Nat. Geosci. 9 (10), 737–742. doi: 10.1038/ngeo2790
Krause-Jensen D., Lavery P., Serrano O., Marba N., Masque P., Duarte C. M. (2018). Sequestration of macroalgal carbon: The elephant in the blue carbon room. Biol. Lett. 14 (6),20180236. doi: 10.1098/rsbl.2018.0236
Kristensen E. (1990). Characterization of biogenic organic matter by stepwise thermogravimetry (STG). Biogeochemistry 9), 135–159. doi: 10.1007/BF00692169
Kühn W., Pätsch J., Thomas H., Borges A. V., Schiettecatte L.-S., Bozec Y., et al. (2010). Nitrogen and carbon cycling in the North Sea and exchange with the North Atlantic-a model study, part II: Carbon budget and fluxes. Cont. Shelf Res. 30 (16), 1701–1716. doi: 10.1016/j.csr.2010.07.001
Kumar M., Boski T., González-Vila F. J., de la Rosa J. M., González-Pérez J. A. (2020). Discerning natural and anthropogenic organic matter inputs to salt marsh sediments of Ria Formosa lagoon (South Portugal). Environ. Sci. pollut. Res. 27 (23), 28962–28985. doi: 10.1007/s11356-020-09235-9
Kutschera W. (2013). Applications of accelerator mass spectrometry. Int. J. Mass Spectrom. 349, 203–218. doi: 10.1016/j.ijms.2013.05.023
Lafratta A., Serrano O., Masqué P., Mateo M. A., Fernandes M., Gaylard S., et al. (2020). Challenges to select suitable habitats and demonstrate 'additionality' in Blue Carbon projects: A seagrass case study. Ocean Coast. Manage. 197, 105295. doi: 10.1016/j.ocecoaman.2020.105295
Lallias D., Hiddink J. G., Fonseca V. G., Gaspar J. M., Sung W., Neill S. P., et al. (2015). Environmental metabarcoding reveals heterogeneous drivers of microbial eukaryote diversity in contrasting estuarine ecosystems. ISME J. 9 (5), 1208–1221. doi: 10.1038/ismej.2014.213
Lalonde K., Mucci A., Ouellet A., Gélinas Y. (2012). Preservation of organic matter in sediments promoted by iron. Nature 483 (7388), 198–200. doi: 10.1038/nature10855
Lamb P. D., Hunter E., Pinnegar J. K., Creer S., Davies R. G., Taylor M. I. (2019). How quantitative is metabarcoding: A meta-analytical approach. Mol. Ecol. 28 (2), 420–430. doi: 10.1111/mec.14920
Landenmark H. K. E., Forgan D. H., Cockell C. S. (2015). An estimate of the total DNA in the biosphere. PloS Biol. 13 (6), 1–10. doi: 10.1371/journal.pbio.1002168
LaRowe D. E., Arndt S., Bradley J. A., Estes E. R., Hoarfrost A., Lang S. Q., et al. (2020). The fate of organic carbon in marine sediments - new insights from recent data and analysis. Earth-Sci. Rev. 204, 103146. doi: 10.1016/j.earscirev.2020.103146
Legge O., Johnson M., Hicks N., Jickells T., Diesing M., Aldridge J., et al. (2020). Carbon on the northwest European shelf: Contemporary budget and future influences. Front. Mar. Sci. 7, 143. doi: 10.3389/fmars.2020.00143
Leonard A., Castle S., Burr G. S., Lange T., Thomas J. (2013). A wet oxidation method for AMS radiocarbon analysis of dissolved organic carbon in water. Radiocarbon 55, 545–552. doi: 10.1017/S0033822200057672
Libby W. F., Anderson E. C., Arnold J. R. (1949). Age determination by radiocarbon content — world- wide assay of natural radiocarbon. Science 109, 227–228. doi: 10.1126/science.109.2827.227
Litherland A., Zhao X. L., Kieser W. (2011). Mass spectrometry with accelerators. Mass Spectrom. Rev. 30, 1037–1072. doi: 10.1002/mas.20311
Loh P. S., Reeves A. D., Harvey S. M., Overnell J., Miller J. A. E. J. (2008). The fate of terrestrial organic matter in two Scottish sea lochs. Estuar. Coast. Shelf Sci. 76 (3), 566–579. doi: 10.1016/j.ecss.2007.07.023
Lopez-Capel E., Bol R., Manning D. A. C. (2005). Application of simultaneous thermal analysis mass spectrometry and stable carbon isotope analysis in a carbon sequestration study. Rapid Commun. Mass Spectrom. 19 (22), 3192–3198. doi: 10.1002/rcm.2145
Lougheed B. C., Metcalfe B., Ninnemann U. S., Wacker L. (2018). Moving beyond the age–depth model paradigm in deep-sea palaeoclimate archives: Dual radiocarbon and stable isotope analysis on single foraminifera. Climate Past 14 (4), 515–526. doi: 10.5194/cp-14-515-2018
Lougheed B., Obrochta S. J. (2016). MatCal: open source bayesian 14C age calibration in MatLab. J. Open Res. Software 4, e42. doi: 10.5334/jors.130.
Lovelock C. E., Duarte C. M. (2019). Dimensions of blue carbon and emerging perspectives. Biol. Lett. 15 (3), 20180781. doi: 10.1098/rsbl.2018.0781
Luisetti T., Turner R. K., Andrews J. E., Jickells T. D., Kröger S., Diesing M., et al. (2019). Quantifying and valuing carbon flows and stores in coastal and shelf ecosystems in the UK. Ecosyst. Serv. 35, 67–76. doi: 10.1016/j.ecoser.2018.10.013
Luisetti T., Ferrini S., Grilli G., Jickells T. D., Kennedy H., Kröger S., et al. (2020). Climate action requires new accounting guidance and governance frameworks to manage carbon in shelf seas. Nat. Commun. 11 (1), 4599. doi: 10.1038/s41467-020-18242-w
MacKenzie A. B., Hardie S. M.L., Farmer J. G., Eades L. J., Pulford I. D. (2011). Analytical and sampling constraints in 210Pb dating. Sci. Total Environ. 409 (7), 1298–1304. doi: 10.1016/j.scitotenv.2010.11.040
Macreadie P. I., Anton A., Raven J. A., Beaumont N., Connolly R. M., Friess D. A., et al. (2019). The future of blue carbon science. Nat. Commun. 10 (1), 3998. doi: 10.1038/s41467-019-11693-w
Madsen A. T., Murray A. S., Andersen T. J., Pejrup M., Breuning-Madsen H. (2005). Optically stimulated luminescence dating of young estuarine sediments: A comparison with 210Pb and 137Cs dating. Mar. Geol. 214 (1–3), 251–268. doi: 10.1016/j.margeo.2004.10.034
Manning D. A. C., Lopez-Capel E., Barker S. (2005). Seeing soil carbon: Use of thermal analysis in the characterization of soil C reservoirs of differing stability. Mineral. Mag. 69 (4), 425–435. doi: 10.1180/0026461056940260
Mas J. L., Aparicio P., Galán E., Romero-Baena A., Miras A., Yuste A., Martín D. (2020). Determination of uranium and thorium isotopes in kaolinitic samples by ICP-MS/MS. Appl. Clay Sci. 196, 105736. doi: 10.1016/j.clay.2020.105736
Masiello C. A. (2004). New directions in black carbon organic geochemistry. Mar. Chem. 92, 201–213. doi: 10.1016/j.marchem.2004.06.043
Masiello C. A., Druffel E. R. M., Currie L. A. (2002). Radiocarbon measurements of black carbon in aerosols and ocean sediments. Geochim. Cosmochim. Acta 66 (6), 1025–1036. doi: 10.1016/S0016-7037(01)00831-6
Mauquoy D., Payne R. J., Babeshko K. V., Bartlett R., Boomer I., Bowey H., et al. (2020). Falkland Island peatland development processes and the pervasive presence of fire. Quaternary Sci. Rev. 240, 106391. doi: 10.1016/j.quascirev.2020.106391
Meyers P. A. (1994). Preservation of elemental and isotopic source identification of sedimentary organic matter. Chem. Geol. 114 (3–4), 289–302. doi: 10.1016/0009-2541(94)90059-0
Middelburg J. J. (2018). Reviews and syntheses: To the bottom of carbon processing at the seafloor. Biogeosciences 15 (2), 413–427. doi: 10.5194/bg-15-413-2018
Middelburg J. J., Nieuwenhuize J., Van Breugel P. (1999). Black carbon in marine sediments. Mar. Chem. 65 (3–4), 245–252. doi: 10.1016/S0304-4203(99)00005-5
Molnár M., Hajdas I., Janovics R., Rinyu L., Synal H. A., Veres M., Wacker L. (2013). C-14 analysis of groundwater down to the millilitre level. Nucl. Instrum. Methods Phys. Res. Section B: Beam Interact. Mater. Atoms 294, 573–576. doi: 10.1016/j.nimb.2012.03.038
Morys C., Brüchert V., Bradshaw C. (2021). Impacts of bottom trawling on benthic biogeochemistry in muddy sediments: Removal of surface sediment using an experimental field study. Mar. Environ. Res. 169, 105384. doi: 10.1016/j.marenvres.2021.105384
Najjar R. G., Herrmann M., Alexander R., Boyer E. W., Burdige D. J., Butman D., et al. (2018). Carbon budget of tidal wetlands, estuaries, and shelf waters of eastern North America. Global Biogeochem. Cycles 32 (3), 389–416. doi: 10.1002/2017GB005790
Natali C., Bianchini G., Carlino P. (2020). Thermal stability of soil carbon pools: Inferences on soil nature and evolution. Thermochim. Acta 683, 178478. doi: 10.1016/j.tca.2019.178478
Nieuwenhuize J., Maas Y. E., Middelburg J. J. (1994). Rapid analysis of organic carbon and nitrogen in particulate materials. Mar. Chem. 45 (3), 217–224. doi: 10.1016/0304-4203(94)90005-1
Oberle F. K. J., Storlazzi C. D., Hanebuth T. J. J. (2016b). What a drag: Quantifying the global impact of chronic bottom trawling on continental shelf sediment. J. Mar. Syst. 159, 109–119. doi: 10.1016/j.jmarsys.2015.12.007
Oberle F. K. J., Swarzenski P. W., Reddy C. M., Nelson R. K., Baasch B., Hanebuth T. J. J. (2016a). Deciphering the lithological consequences of bottom trawling to sedimentary habitats on the shelf. J. Mar. Syst. 159, 120–131. doi: 10.1016/j.jmarsys.2015.12.008
Ortega A., Geraldi N. R., Alam I., Kamau A. A., Acinas S. G., Logares R., et al. (2019). Important contribution of macroalgae to oceanic carbon sequestration. Nat. Geosci. 12 (9), 748–754. doi: 10.1038/s41561-019-0421-8
Ortega A., Geraldi N. R., Duarte C. M. (2020). Environmental DNA identifies marine macrophyte contributions to blue carbon sediments. Limnol. Oceanogr. 65 (12), 3139–3149. doi: 10.1002/lno.11579
Oudghiri F., García-Morales J. L., Rodríguez-Barroso M. R. (2015). Novel use of TGA-FTIR technique to predict the pollution degree in marine sediments. Infrared Phys. Technol. 72, 52–57. doi: 10.1016/j.infrared.2015.07.006
Palanques A., Puig P., Guillén J., Demestre M., Martín J. (2014). Effects of bottom trawling on the Ebro continental shelf sedimentary system (NW Mediterranean). Cont. Shelf Res. 72, 83–98. doi: 10.1016/J.CSR.2013.10.008
Pappa F. K., Tsabaris C., Patiris D. L., Eleftheriou G., Ioannidou A., Androulakaki E. G., et al. (2019). Temporal investigation of radionuclides and heavy metals in a coastal mining area at Ierissos Gulf, Greece. Environ. Sci. pollut. Res. 26 (26), 27457–27469. doi: 10.1007/s11356-019-05921-5
Paradis S., Pusceddu A., Masqué P., Puig P., Moccia D., Russo T., Iacono Lo C., et al. (2019). Organic matter contents and degradation in a highly trawled area during fresh particle inputs (Gulf of Castellammare, southwestern Mediterranean). Biogeosciences 16 (21), 4307–4320. doi: 10.5194/bg-16-4307-2019
Paradis S., Goñi M., Masqué P., Durán R., Arjona-Camas M., Palanques A., et al. (2021). Persistence of biogeochemical alterations of deep-sea sediments by bottom trawling. Geophys.ical Res. Lett. 48, e2020GL091279. doi: 10.1029/2020GL091279
Pawlowski J., Bruce K., Panksep K., Aguirre F. I., Amalfitano S., Apothéloz-Perret-Gentil L., et al. (2022). Environmental DNA metabarcoding for benthic monitoring: A review of sediment sampling and DNA extraction methods. Sci. Total Environ. 818, 151783. doi: 10.1016/j.scitotenv.2021.151783
Perdue E. M., Koprivnjak J. F. (2007). Using the C/N ratio to estimate terrigenous inputs of organic matter to aquatic environments. Estuar. Coast. Shelf Sci. 73 (1–2), 65–72. doi: 10.1016/j.ecss.2006.12.021
Pessarrodona A., Moore P. J., Sayer M. D. J., Smale D. A. (2018). Carbon assimilation and transfer through kelp forests in the NE Atlantic is diminished under a warmer ocean climate. Global Change Biol. 24 (9), 4386–4398. doi: 10.1111/gcb.14303
Phillips S. C., Johnson J. E., Miranda E., Disenhof C. (2011). Improving CHN measurements in carbonate-rich marine sediments. Limnol. Oceanogr. 9, 194–203. doi: 10.4319/lom.2011.9.194
Pusceddu A., Bianchelli S., Martín J., Puig P., Palanques A., Masqué P., Danovaro R. (2014). Chronic and intensive bottom trawling impairs deep-sea biodiversity and ecosystem functioning. Proc. Natl. Acad. Sci. U. S. A. 111 (24), 8861–8866. doi: 10.1073/pnas.1405454111
Queirós A. M., Stephens N., Widdicombe S., Tait K., McCoy S. J., Ingels J., et al. (2019). Connected macroalgal-sediment systems: Blue carbon and food webs in the deep coastal ocean. Ecol. Monogr. 89 (3), 1–21. doi: 10.1002/ecm.1366
Ramsey C. B. (1995). Radiocarbon calibration and analysis of stratigraphy: The OxCal program. Radiocarbon 37, 425–430. doi: 10.1017/S0033822200030903
Ramsey C. B. (2009). Bayesian analysis of radiocarbon dates. Radiocarbon 51 (1), 337–360. doi: 10.1017/s0033822200033865
Ramsey C. B., Heaton T. J., Schlolaut G., Staff R. A., Bryant C. L., Brauer A., et al. (2020). Reanalysis of the atmospheric radiocarbon calibration record from Lake Suigetsu, Japan. Radiocarbon 62 (4), 989–999. doi: 10.1017/RDC.2020.18
Ratnasingham S., Hebert P. D. N. (2007). BOLD: The barcode of life data system: Barcoding. Mol. Ecol. Notes 7 (3), 355–364. doi: 10.1111/j.1471-8286.2007.01678.x
Reef R., Atwood T. B., Samper-Villarreal J., Adame M. F., Sampayo E. M., Lovelock C. E. (2017). Using eDNA to determine the source of organic carbon in seagrass meadows. Limnol. Oceanogr. 62 (3), 1254–1265. doi: 10.1002/lno.10499
Reimer P. (2020). Composition and consequences of the IntCal20 radiocarbon calibration curve. Quaternary Res. 96, 22–27. doi: 10.1017/qua.2020.42
Reiss H., Wieking G., Kröncke I. (2007). Microphytobenthos of the Dogger Bank: A comparison between shallow and deep areas using phytopigment composition of the sediment. Mar. Biol. 150, 1061–1071. doi: 10.1007/s00227-006-0423-0
Ren P., Liu Y., Shi X., Sun S., Fan D., Wang X. (2019). Sources and sink of black carbon in Arctic Ocean sediments. Sci. Total Environ. 689, 912–920. doi: 10.1016/j.scitotenv.2019.06.437
Rijnsdorp A. D., Depestele J., Molenaar P., Eigaard O. R., Ivanović A., O’Neill F. G. (2021). Sediment mobilization by bottom trawls: A model approach applied to the Dutch North Sea beam trawl fishery. ICES J. Mar. Sci. 78 (5), 1574–1586. doi: 10.1093/icesjms/fsab029
Robbins J. A. (1978). “‘Geochemical and geophysical applications of radioactive lead’,” in The biogeochemistry of lead in the environment. Ed. Nriagu J. O. (Amsterdam: North-Holland Biomedical Press), 285–393.
Roberts C. M., O’Leary B. C., Mccauley D. J., Cury P. M., Duarte C. M., Lubchenco J., et al. (2017). Marine reserves can mitigate and promote adaptation to climate change. Proc. Natl. Acad. Sci. United States America 114 (24), 6167–6175. doi: 10.1073/pnas.1701262114
Robinson L. F., Belshaw N. S., Henderson G. M. (2004). U and Th concentrations and isotope ratios in modern carbonates and waters from the Bahamas. Geochim. Cosmochim. Acta 68 (8), 1777–1789. doi: 10.1016/j.gca.2003.10.005
Russo T., Parisi A., Cataudella S. (2011). New insights in interpolating fishing tracks from VMS data for different métiers. Fish. Res. 108 (1), 184–194. doi: 10.1016/j.fishres.2010.12.020
Sadler P. M. (1981). Sediment accumulation rates and the completeness of stratigraphic sections. J. Geol. 89.5, 569–584. doi: 10.1086/628623
Sahu S. K., Ajmal P. Y., Bhangare R. C., Tiwari M., Pandit G. G. (2014). Natural radioactivity assessment of a phosphate fertilizer plant area. J. Radiat. Res. Appl. Sci. 7 (1), 123–128. doi: 10.1016/j.jrras.2014.01.001
Sala E., Mayorga J., Bradley D., Cabral R. B., Atwood T. B., Auber A., et al. (2021). Protecting the global ocean for biodiversity, food and climate. Nature. 592, 397–402. doi: 10.1038/s41586-021-03371-z
Sanches L. F., Guenet B., dos Anjos Cristiano Marino N., de Assis Esteves F. (2021). Exploring the drivers controlling the priming effect and its magnitude in aquatic systems. J. Geophys.ical Res.: Biogeosci. 126, e2020JG006201. doi: 10.1029/2020JG006201
Sañé E., Martín J., Puig P., Palanques A. (2013). Organic biomarkers in deep-sea regions affected by bottom trawling: Pigments, fatty acids, amino acids and carbohydrates in surface sediments from the La Fonera (Palamós) canyon, NW Mediterranean Sea. Biogeosciences 10 (12), 8093–8108. doi: 10.5194/bg-10-8093-2013
Santos G. M., Granato-Souza D., Barbosa A. C., Oelkers R., Andreu-Hayles L. (2020). Radiocarbon analysis confirms annual periodicity in cedrela odorata tree rings from the equatorial Amazon. Quaternary Geochronol. 58, 101079. doi: 10.1016/j.quageo.2020.101079
Santos I. R., Burdige D. J., Jennerjahn T. C., Bouillon S., Cabral A., Serrano O., et al. (2021). The renaissance of Odum’s outwelling hypothesis in “Blue Carbon” science. Estuar. Coast. Shelf Sci. 255, 107361. doi: 10.1016/j.ecss.2021.107361
Santschi P. H., Rowe G. T. (2008). Radiocarbon-derived sedimentation rates in the Gulf of Mexico. Deep Sea Res. Part II: Top. Stud. Oceanogr. 55 (24–26), 2572–2576. doi: 10.1016/j.dsr2.2008.07.005
Schmidt S., Howa H., Diallo A., Martín J., Cremer M., Duros P., et al. (2014). Recent sediment transport and deposition in the Cap-Ferret Canyon, South-East Margin of Bay of Biscay. Deep-Sea Res. Part II: Top. Stud. Oceanogr. 104, 134–144. doi: 10.1016/j.dsr2.2013.06.004
Serpetti N., Heath M., Rose M., Witte U. (2012). High resolution mapping of sediment organic matter from acoustic reflectance data. Hydrobiologia 680 (1), 265–284. doi: 10.1007/s10750-011-0937-4
Sikes E. L., Uhle M. E., Nodder S. D., Howard M. E. (2009). Sources of organic matter in a coastal marine environment: Evidence from n-alkanes and their δ13C distributions in the Hauraki Gulf, New Zealand. Mar. Chem. 113 (3–4), 149–163. doi: 10.1016/j.marchem.2008.12.003
Silburn B. E., Sivyer D. B., Kroeger S., Parker R., Mason C., Nelson P., et al. (2017). ‘Shelf sea biogeochemistry sediment characterisation’ (UK: British Oceanographic Data Centre - Natural Environment Research Council).
Smeaton C., Austin W. E. N. (2017). Sources, sinks, and subsidies: Terrestrial carbon storage in mid-latitude fjords. J. Geophys.ical Res.: Biogeosci. 122 (11), 2754–2768. doi: 10.1002/2017JG003952
Smeaton C., Austin W. E. (2022). Quality not quantity: Prioritizing the management of sedimentary organic matter across continental shelf seas. Geophys.ical Res. Lett. 49, e2021GL097481. doi: 10.1029/2021GL097481
Smeaton C., Cui X., Bianchi T. S., Cage A. G., Howe J. A., Austin W. E. N. (2021c). The evolution of a coastal carbon store over the last millennium. Quaternary Sci. Rev. 266, 107081. doi: 10.1016/j.quascirev.2021.107081
Smeaton C., Hunt C. A., Turrell W. R., Austin W. E. N. (2021a). Marine sedimentary carbon stocks of the United Kingdom’s Exclusive Economic Zone. Front. Earth Sci. 9. doi: 10.3389/feart.2021.593324
Smeaton C., Yang H., Austin W. E. N. (2021b). Carbon burial in the mid-latitude fjords of Scotland. Mar. Geol. 441, 106618. doi: 10.1016/j.margeo.2021.106618
Soetaert K., Herman P. M. J., Middelburg J. J. (1996). A model of early diagenetic processes from the shelf to abyssal depths. Geochim. Cosmochim. Acta 60 (6), 1019–1040. doi: 10.1016/0016-7037(96)00013-0
Sparkes R. B., Selver A. D., Gustafsson Ö., Semiletov I. P., Haghipour N., Wacker L., et al. (2016). Macromolecular composition of terrestrial and marine organic matter in sediments across the East Siberian Arctic Shelf. Cryosphere 10 (5), 2485–2500. doi: 10.5194/tc-10-2485-2016
Stevenson M. A., Abbott G. D. (2019). Exploring the composition of macromolecular organic matter in arctic ocean sediments under a changing sea ice gradient. J. Anal. Appl. Pyrolysis 140, 102–111. doi: 10.1016/j.jaap.2019.02.006
Subha Anand S., Rengarajan R., Shenoy D., Gauns M., Naqvi S. W. A. (2017). POC export fluxes in the Arabian Sea and the Bay of Bengal: A simultaneous 234Th/238U and 210Po/210Pb study. Mar. Chem. 198, 70–87. doi: 10.1016/j.marchem.2017.11.005
Synal H.-A., Jacob S., Suter M. (2000). The PSI/ETH small radiocarbon dating system. Nucl. Instrum. Methods Phys. Res. Section B: Beam Interact. Mater. Atom 2, 1–7.
Tao S., Eglinton T. I., Montluçon D. B., McIntyre C., Zhao M. (2015). Pre-aged soil organic carbon as a major component of the Yellow River suspended load: Regional significance and global relevance. Earth Planet. Sci. Lett. 414, 77–86. doi: 10.1016/j.epsl.2015.01.004
Thompson C. E. L., Williams M. E., Amoudry L., Hull T., Reynolds S., Panto A., Fones G. R. (2019). Benthic controls of resuspension in UK shelf seas: Implications for resuspension frequency. Cont. Shelf Res. 185, 3–15. doi: 10.1016/j.csr.2017.12.005
Thornton S. F., McManus J. (1994). Application of organic carbon and nitrogen stable isotope and C/N ratios as source indicators of organic matter provenance in estuarine systems: Evidence from the Tay Estuary, Scotland. Estuar. Coast. Shelf Sci. 38 (3), 219–233. doi: 10.1006/ecss.1994.1015
Tolosa I., Bayona J. M., Albaigés J. (1996). Aliphatic and polycyclic aromatic hydrocarbons and sulfur/oxygen derivatives in northwestern Mediterranean sediments: Spatial and temporal variability, fluxes, and budgets. Environ. Sci. Technol. 30 (8), 2495–2503. doi: 10.1021/es950647x
Trevathan-Tackett S. M. (2016). Dynamics of refractory carbon in seagrass meadows. (PhD Thesis,University of Technology Sydney:Sydney Australia).
Trevathan-Tackett S. M., Kelleway J., Macreadie P. I., Beardall J., Ralph P., Bellgrove A. (2015). Comparison of marine macrophytes for their contributions to blue carbon sequestration. Ecology 96 (11), 3043–3057. doi: 10.1890/15-0149.1
Trevathan-Tackett S. M., Macreadie P. I., Sanderman J., Baldock J., Howes J. M., Ralph P. J. (2017). A global assessment of the chemical recalcitrance of seagrass tissues: Implications for long-term carbon sequestration. Front. Plant Sci. 8. doi: 10.3389/fpls.2017.00925
van Nugteren P., Moodley L., Brummer G.-J., Heip C. H. R., Herman P. M. J., Middelburg J. J. (2009). Seafloor ecosystem functioning: The importance of organic matter priming. Mar. Biol. 156, 2277–2287. doi: 10.1007/s00227-009-1255-5
Vaughn D. R., Bianchi T. S., Shields M. R., Kenney W. F., Osborne T. Z. (2021). Blue carbon soil stock development and estimates within northern Florida wetlands. Front. Earth Sci. 9. doi: 10.3389/feart.2021.552721
Verardo D. J., Froelich P. N., McIntyre A. (1990). Determination of organic carbon and nitrogen in marine sediments using the Carlo Erba NA-1500 analyzer. Res. Part A. Oceanogr. Res. Papers 37 (1), 157–165. doi: 10.1016/0198-0149(90)90034-S
Wacker L., Lippold J., Molnár M., Schulz H. (2013). Towards radiocarbon dating of single foraminifera with a gas ion source. Nucl. Instrum. Methods Phys. Res. Section B 294,307–310. doi: 10.1016/j.nimb.2012.08.038
Wagner S., Brandes J., Spencer R. G.M., Ma K., Rosengard S. Z., Moura J. M. S., Stubbins A. (2019). Isotopic composition of oceanic dissolved black carbon reveals non-riverine source. Nat. Commun. 10 (1), 1–8. doi: 10.1038/s41467-019-13111-7
Wakelin S. L., Holt J. T., Blackford J. C., Allen J. I., Butenschön M., Artioli Y. (2012). Modeling the carbon fluxes of the northwest European continental shelf: Validation and budgets. J. Geophys.ical Res.: Oceans 117 (5), 1–17. doi: 10.1029/2011JC007402
Watson S. C. L., Preston J., Beaumont N. J., Watson G. J. (2020). Assessing the natural capital value of water quality and climate regulation in temperate marine systems using a EUNIS biotope classification approach. Sci. Total Environ. 744, 140688. doi: 10.1016/j.scitotenv.2020.140688
Welte C., Wacker L., Hattendorf B., Christl M., Fohlmeister J., Breitenbach S. F.M., et al. (2016). Laser ablation - accelerator mass spectrometry: An approach for rapid radiocarbon analyses of carbonate archives at high spatial resolution. Anal. Chem. 88 (17), 8570–8576. doi: 10.1021/acs.analchem.6b01659
Wernberg T., Filbee-Dexter K. (2018). Grazers extend blue carbon transfer by slowing sinking speeds of kelp detritus. Sci. Rep. 8 (1), 1–7. doi: 10.1038/s41598-018-34721-z
Wilson R. J., Speirs D. C., Sabatino A., Heath M. R. (2018). A synthetic map of the north-west European Shelf sedimentary environment for applications in marine science. Earth System Sci. Data 10 (1), 109–130. doi: 10.5194/essd-10-109-2018
Yates M. C., Fraser D. J., Derry A. M. (2019). Meta-analysis supports further refinement of eDNA for monitoring aquatic species-specific abundance in nature. Environ. DNA 1 (1), 5–13. doi: 10.1002/edn3.7
Zhao Y., Wu F., Fang X., Yang Y. (2015). Topsoil C/N ratios in the Qilian Mountains area: Implications for the use of subaqueous sediment C/N ratios in paleo-environmental reconstructions to indicate organic sources. Palaeogeogr. Palaeoclimatol. Palaeoecol. 426, 1–9. doi: 10.1016/j.palaeo.2015.02.038
Keywords: particulate organic carbon (POC), marine sediment analysis, carbon stock, carbon sequestration, carbon provenance, carbon vulnerability, carbon management
Citation: Graves CA, Benson L, Aldridge J, Austin WEN, Dal Molin F, Fonseca VG, Hicks N, Hynes C, Kröger S, Lamb PD, Mason C, Powell C, Smeaton C, Wexler SK, Woulds C and Parker R (2022) Sedimentary carbon on the continental shelf: Emerging capabilities and research priorities for Blue Carbon. Front. Mar. Sci. 9:926215. doi: 10.3389/fmars.2022.926215
Received: 22 April 2022; Accepted: 08 August 2022;
Published: 08 September 2022.
Edited by:
Tim Rixen, Leibniz Centre for Tropical Marine Research (LG), GermanyReviewed by:
Markus Diesing, Geological Survey of Norway, NorwayNicole Burdanowitz, University of Hamburg, Germany
Copyright © 2022 Graves, Benson, Aldridge, Austin, Dal Molin, Fonseca, Hicks, Hynes, Kröger, Lamb, Mason, Powell, Smeaton, Wexler, Woulds and Parker. This is an open-access article distributed under the terms of the Creative Commons Attribution License (CC BY). The use, distribution or reproduction in other forums is permitted, provided the original author(s) and the copyright owner(s) are credited and that the original publication in this journal is cited, in accordance with accepted academic practice. No use, distribution or reproduction is permitted which does not comply with these terms.
*Correspondence: Carolyn A. Graves, Y2Fyb2x5bi5ncmF2ZXNAY2VmYXMuY28udWs=