- 1Department of Biological Sciences, University of Bergen, Bergen, Norway
- 2Integrative Fish Biology Group, Ocean and Environment Department, NORCE Norwegian Research Center, Bergen, Norway
- 3Radboud Institute for Biological and Environmental Studies, Nijmegen, Netherlands
The successful transfer of farmed post-smolt Atlantic salmon (Salmo salar) depends on proper stress responses and cognitive functions during the early seawater (SW) phase. However, with increasing summer oceanic temperatures, these processes may become a challenge, implicating allostasis and welfare. Therefore, we examined the effect of post-smolt transfer from 10°C SW to elevated temperatures (13°C, 16°C, and 18°C) on plasma cortisol and telencephalic genes modulating cognition (neurod, bdnf, pcna, and c-fos) and stress-axis regulation (crf, crfbp, mr, gr1, gr2, and hsd11b2). Fish were sampled at i) 1 day following transfer, ii) 45 days of acclimation, and iii) 45 days and 1 h after an acute challenge test (ACT) using confinement stress. Fish transferred to 13°C retained stress responses, elevating levels of cortisol, crf, mr, gr2, c-fos, and bdnf and maintaining levels of neurod and pcna. Contrastingly, although cortisol increased at 16°C, telencephalic genes reverted to an inhibition of stress responses, increasing crfbp and gr1 complemented with dampened bdnf, neurod, and c-fos responses. However, transferring post-smolts to 18°C showed the most adverse effects, having absent stress responses (cortisol and c-fos), elevated crfbp, and a suppression of hsd11b2 and neurod. The hsd11b2 downregulation implies low cortisol inhibition in line with absent modulations in corticosteroid receptors and stress responses. These results suggest that the transfer to 16°C and 18°C inhibits the normal reactive response of post-smolts. Following acclimation (45 days), cortisol levels were basal for all groups; however, post-smolts at 16°C and 18°C maintained a telencephalic inhibition of key regulatory genes (crf, mr, gr2, and hsd11b2), alongside a lower mr/gr1 ratio, an indicator of chronic allostatic load. Moreover, neural plasticity (neurod and pcna) was suppressed at 16°C and 18°C, suggesting impacts of elevated allostatic loads with potentially inferior cognitive capacities. Despite maintaining similar plasma cortisol responses to ACTs, post-smolts at 16°C and 18°C elevated neural activation (c-fos) to stress, implying greater challenges, with the 18°C group also elevating the level of bdnf. In summary, the telencephalon shows that post-smolts transferred to 16°C and 18°C continue to struggle with the thermal allostatic loads even after acclimation, which is not revealed by plasma cortisol levels, grounding the importance of telencephalic measures in identifying environmental thresholds and hidden challenges.
1 Introduction
Salmon are highly plastic to their environment as illustrated by their diverse life history strategies (Bourret et al., 2016). This is achieved physiological adjustments and brain plasticity to maintain homeostasis and adapt to challenges (Ebbesson and Braithwaite, 2012; Salvanes et al., 2013). The brain’s involvement in coping with stress determines how individuals deal with stressors, at both functional and structural levels. The consequences of these physiological and behavioral stress responses can be adaptive on short and long term (allostasis) but may also become maladaptive if complete adaptation is not met [allostatic (over)load] (Korte et al., 2007). In this case, chronic persistent allostatic load may alter neural plasticity and neurogenesis, predisposing fish vulnerable to stress-related dysfunctions (Korte et al., 2007; Romero et al., 2009). The model of allostasis has been applied to fish, showing that elevated allostatic load decreases cognitive functions (Grassie et al., 2013; Manuel et al., 2014a), alters stress feedback between body and brain (Madaro et al., 2015; Gorissen and Flik, 2016; Madaro et al., 2016; Samaras et al., 2018), and impairs immunity (Yada and Tort, 2016) and reproduction (Schreck, 2010). However, mildly increased allostatic loads, such as chronic mild stressors, may be difficult to detect in protected environments with sufficient energy supplies. Therefore, acute challenge tests (ACTs) have been optimized to subject fish to an added stressor, allowing to detect these underlying physiological differences that would otherwise go unnoticed (Grassie et al., 2013; Madaro et al., 2015; Samaras et al., 2018). Altogether, maintaining proper brain function is key for allostasis, as when the animal’s normal reactive scope is overwhelmed, the ability to respond to additional challenges deteriorates, leading to impaired welfare (Romero et al., 2009; Ebbesson and Braithwaite, 2012; Schreck and Tort, 2016; McCormick and Romero, 2017).
Climate change–driven thermal allostatic loads in aquatic habitats are ever-increasing, altering life history strategies of salmon stocks (Friedland, 1998; Vargas–Chacoff et al., 2018; Nicola et al., 2018 and Nielsen et al., 2013). Furthermore, the increasing use of year–round land–based rearing strategies may expose farmed Atlantic salmon (Salmo salar) post–smolts to elevated ambient temperatures during summer and autumn sea cage transfers, to which fish may lack the necessary acclimation history (Friedland, 1998). As elevated temperatures have pervasive and considerable physiological consequences in Atlantic salmon (Gonçalves et al., 2006; Gallant et al., 2017; Vargas–Chacoff et al., 2018), this may impact stress responses and capacities to handle stressors as upper thermal limits are approached, inherently raising welfare concern (Flik et al., 2006; Olsvik et al., 2013; Madaro et al., 2018; Beemelmanns et al., 2021). Moreover, the first few months after post–smolt sea cage transfer are considered the most vulnerable for the salmon lifecycle, during which fish must resist a variety of other stressors [i.e., confinement, handling, and transport (Handeland et al., 2003; Jarungsriapisit et al., 2016)]. As a result, the successful transfer of post–smolts relies on adaptation, appropriate stress responses, and cognitive functions to promote allostasis and stress resilience during this critical period.
Activation of the stress response releases cortisol to re–establish homeostasis, effects that are sustained through corticosteroid receptors (CRs), including type I mineralocorticoid receptor (mr) and type II glucocorticoid receptors (gr1 and gr2) (Wendelaar Bonga, 1997; Mommsen et al., 1999). In most fish species, mr and gr2 have high affinity to cortisol and regulate baseline functions, whereas gr1 has low cortisol affinity and is activated by high cortisol levels (Stolte et al., 2008; Sørensen et al., 2013). When stress becomes chronic (overload), CR signaling may become suppressed to protect neuronal integrity (Madaro et al., 2015; Madaro et al., 2016). Given the importance of CRs in regulating neural functions, improper regulation can induce receptor bioavailability changes that shift the mr/gr1 ratio, which can impose significant constraints to stress responses, neural plasticity, and neurogenesis, pivotal for allostasis (De Kloet et al., 2005; Sørensen et al., 2011; Sørensen et al., 2013; Madaro et al., 2015; Madaro et al., 2016). Further, 11β–Hydroxysteroid dehydrogenase type 2 (HSD11B2) is an important safety mechanism here by regulating cortisol levels via conversion to inactive cortisone (Alderman and Vijayan, 2012; Mifsud and Reul, 2018). A recent study in European seabass (Dicentrarchus labrax) revealed thermally induced changes to telencephalic mr and gr1 (Goikoetxea et al., 2021). In particular, MR is central for processes of learning and memory in the telencephalon (homolog to hippocampus and amygdala) (Karst et al., 2005), and suppressed levels of these can negatively impact cognitive functions (De Kloet et al., 2005; Samaras et al., 2018). The neural activation of the stress axis is also tightly regulated by the corticotropin–releasing factor (crf) system, which stimulates adrenocorticotropic hormone (acth) and cortisol release from the pituitary gland and head kidney, respectively (Flik et al., 2006; Gorissen and Flik, 2016). As the actions of crf are inhibited by crf–binding protein (crfbp), the interplay between these components is critical for stress response reactivity during and after stress (Fryer et al., 1984; Baker et al., 1996; Rotllant et al., 2000; Huising et al., 2004; Manuel et al., 2014b). Although temporally differentiated, recent evidence suggests a modulation of the crf system to coincide with reductions in neural proliferation in response to thermal challenges, implicating a role of this system in the regulation of neural processes (Yuan et al., 2020). Taken together, as increased stress commonly manifests as altered telencephalic transcript levels of crf, crfbp, hsd11b2, mr, gr1, and gr2, monitoring these provides a promising approach to study allostasis, which can optionally be linked to shifts in telencephalic functions such as neural plasticity (Sørensen et al., 2013). Further, because of the Atlantic Salmon Genome Tetraploidy (Lien et al., 2016), paralog genes may allow differential regulation to stressors for promoting plasticity (Macqueen and Johnston, 2014). Therefore, paralog–specific analysis of these telencephalic regulatory genes was implemented.
Temperature modulations in fish behavior have previously been noted (Bartolini et al., 2015; Toni et al., 2019). However, knowledge is still lacking to how neural mechanisms underpin these changes in Atlantic salmon. Proper cognitive functioning of the fish telencephalon relies on neural plasticity and neurogenesis for learning, memory, and decision–making, whereby stress can either stimulate or inhibit these processes depending on severity and duration (Ebbesson and Braithwaite, 2012; Sørensen et al., 2013). Therefore, the modulation in these key neural components can signal shifts to cognitive capacities and flexibility to environmental challenges, illustrating their importance for stress resilience (Ebbesson and Braithwaite, 2012; Shors et al., 2012; Grassie et al., 2013; Salvanes et al., 2013). Neurogenic differentiation factor (neurod) is involved in the initiation and regulation of neural differentiation, providing a reliable indicator for neuroplasticity (Ebbesson and Braithwaite, 2012), whereas proliferating cell nuclear antigen (pcna) is a marker for neurogenesis (Sørensen et al., 2011; Sadoul et al., 2018). Conversely, brain–derived neurotrophic factor (bdnf) is involved in synapse refinement and promotes neurogenesis and cell survival, which allow rapid modifications in coping strategies and behavioral phenotypes (McMillan et al., 2004; Manuel et al., 2014a; Vindas et al., 2017; Mes et al., 2018). In contrast, the immediate early gene (IEG) c–fos is rapidly expressed in neurons in response to stimuli and indicates neural activity (Kovács, 1998; Pavlidis et al., 2015). The use of c–fos transcript abundance in tandem with plasma cortisol profiles provides a refined approach to address differences in stress response capacities (Mes et al., 2018). Collectively, this demonstrates the potential of these neural markers in evaluating the impact of the environmental challenges on post–smolt resilience and allostasis (Sørensen et al., 2011; Sadoul et al., 2018).
With the ongoing impacts of global warming reporting considerable welfare concerns in Atlantic salmon along with the increasing year-round rearing strategies in aquaculture (Olsvik et al., 2013; Beemelmanns et al., 2021), this study aims to address the influence of elevated temperatures on post–smolt stress responses and telencephalic functions, which are predicted to profoundly impact allostasis, stress resilience, and welfare during the key early seawater (SW) phase. In particular, during summer months, post–smolts can be exposed to rapid elevations in temperatures due to transfer strategies between (semi–) closed containment systems (s–CCSs) and sea cages. Therefore, to mimic common industry practices, we exposed SW–acclimated post–smolts at 10°C directly to 13°C, 16°C, and 18°C, sampled at 1 day post–transfer and after 45 days of acclimation, and then subjected the fish to an ACT using a confinement stressor as an added challenge. To monitor the impacts of stress, circulating plasma cortisol concentrations and transcript levels of telencephalic stress [crf (α and β), crfbp, mr, gr2 (α and β), gr1 (α and β), and hsd11b2 (α and β)] and neural (neurod, bdnf, pcna, and c–fos) markers were analyzed in tandem.
2 Material and Methods
2.1 Fish
All experimental procedures were approved by Norwegian Food Safety Authority (Identification number 8017). On May 11, 2016, Atlantic salmon smolts (99 ± 0.61 g and of 21.4 ± 0.2 cm) were brought from Kjærelva smolt facility (Lerøy Vest Ltd.) to the Industry Laboratory at the High Technology Center in Bergen, Norway. Fish were randomly distributed into eight 500–L and 1–m3 square tanks with flow–through freshwater (FW) at 10°C, and biomass adjustments resulted in 5.4 kg m−3. Fish were left to acclimate to the new conditions for 7 days, followed by salinity adjustment to 15‰ on May 18, to 25‰ on May 23, and full–strength SW (34‰) on May 29, after which fish were left to acclimate to new conditions until initiation of trials.
2.2 Experimental Design
On August 17, four experimental temperature treatments (10°C, 13°C, 16°C, and 18°C), with each treatment containing two randomly selected replicate tanks, were initiated. The four treatments included adjusting water temperature to 13°C, 16°C, and 18°C within 30 min, with a control group maintained at 10°C. Throughout the trials, fish were exposed to constant light and fed continuously with a commercial dry diet (EWOS Microboost 30) using automatic feeders, with temperature and oxygen (>80%) saturation of each tank’s water outlet monitored continuously.
2.3 Tissue Sampling
Samplings (n = 12 per treatment) were conducted at both 1 day post–rapid elevation in temperature to represent the transfer phase referred to as Transfer (1d). The post–smolts were sampled next after 45 days of acclimation [Acclimation (45d)], which was immediately followed by ACTs and sampling 1 h post–stress [ACT (1h)]. Both the Acclimation (45d) and ACT (1h) samplings were conducted in tandem, with 24 fish being quickly netted and 12 fish immediately anesthetized for tissues. The remaining 12 fish were subjected to the ACT by novel confinement stress for 15 min in a 15–L tank within a 150–L holding tank (receiving water from the original treatment). After the 15–min stress, fish were then released into the holding tank for a 45–min recovery period prior to sampling.
For each sampling, fish were starved for a 15–h period and euthanized by receiving a lethal dose (100 mg L−1) of NaCO3–buffered MS222, then immediately bled from the caudal vessel using a heparinized syringe, followed by centrifugation (10 min at 4°C and 4,000 rpm) for plasma collection, and stored at −80°C until cortisol analysis. Plasma samples were attained for all sampling points at Transfer (1d), Acclimation (45d), and ACT (1h). Whole–brain samples were collected at Transfer (1d), Acclimation (45d), and ACT (1h) and preserved in RNAlater (Ambion, Foster City, CA, USA) for 24 h at 8°C prior to storing at −80°C until RNA extraction and analysis of gene expression.
2.4 Plasma Cortisol Analysis
Plasma cortisol concentrations were measured (n = 12 per treatment) for all samplings at Transfer (1d), Acclimation (45d), and ACT (1h) using a custom Enzyme-Linked Immunosorbent Assay (ELISA) analysis previously validated in Atlantic salmon (Madaro et al., 2015; Madaro et al., 2016).
2.5 Extraction of RNA, cDNA Synthesis, and Quantitative Real–Time PCR
Total RNA was extracted from whole telencephalon (n = 12 per treatment) at Transfer (1d), Acclimation (Macqueen and Johnston, 2014), and ACT (1h) by the phenol–chloroform method using TRI Reagent® (Sigma, St. Louis, MO, USA) (Simms et al., 1993). RNA purity was measured using a Nanodrop® ND–1000 UV–Vis Spectrophotometer (NanoDrop Technologies, Wilmington, DE, USA), whereas the concentration was determined using Qubit™3 Instrument (Thermo Fisher Scientific, Eugene, Oregon). Sufficient quality for samples was by assuring RNA integrity values of between 8 and 10 on the Agilent 2100 Bioanalyzer using RNA 6000 Nano LabChip® kit (Agilent technologies, Palo Alto, CA, USA). RNA samples that did not pass quality control measures were removed [see Supplemental Table 1 for final RNA sample (n)]. cDNA synthesis was reverse transcribed using oligo (dT) 20 primers and SuperScript III kit (Invitrogen, Carlsbad, CA, USA) according to the manufacturer’s guidelines. RT–qPCR was conducted using gene–specific primers (Table 1) and SYBR Green Master Mix (Applied Biosystems, Foster City, CA, USA) on the CFX–96 Real–Time PCR detection system platform (Bio–Rad Laboratories, Inc., CA, USA). For each RT–qPCR reaction, 3 µl of cDNA, 0.25 µl of forward primer (10 µmol L−1), 0.25 µl of reverse primer (10 µmol L−1), 3.25 µl of diethylpyrocarbonate (DEPC) – treated dH2O, and 6.25 µl of SYBR Green Master Mix were used to reach a final reaction volume of 13 µl. Melt–curve analysis of primer sets ensured amplification of only a single product with no detectable primer–dimer artifacts. Primer amplification efficiencies (E) were generated by running a two–fold dilution series (1:10, 1:20, 1:40, 1:80, 1:160, 1:320, and 1:640) (cDNA pool) in triplicates to ensure optimal primer efficiency (1.8–2.2) (Table 1). The E were determined by the slope of the regression line a plot of log cDNA dilution versus threshold cycle (Ct) using the formula E = 10−1/slope. A 1:20 dilution was selected for assaying telencephalon cDNA samples. Normalized efficiency corrected relative gene expression was calculated according to (Pfaffl, 2004) using the endogenous reference gene elongation factor 1a (ef1a) (for validation, see Supplemental Table 1). For each qPCR plate, a duplicate No Template Control (NTC) was used to ensure no contamination. Plate inter–calibration was achieved using a duplicate pool run on each plate, from which a correction factor was calculated for each gene–specific plate.
2.6 Statistical Analysis
For all data, normality of distributions and homogeneity of variance were tested using the D’Agostino–Pearson test and the Brown–Forsythe test, respectively, followed by one–way ANOVA tests and Tukey’s post hoc multiple comparisons (Supplemental Table 2). If data did not pass the normality or homogeneity tests, even with data transformations, then the non–parametric Kruskal–Wallis test followed by Dunn’s post hoc test was used. Significance level was set at p < 0.05. Random tank effects were tested using χ2 tests (p < 0.05), and no differences between replicates were found. Outliers were identified using Whisker’s boxplots followed by ROUT1% outlier analysis. Statistical comparisons were made between temperature treatments within each sampling point (indicated by lower case letters). Further, to address the influence of an added confinement stressor (ACT), the non–stressed state [Acclimation (45d)] and stressed fish [ACT (1h)] were statistically compared within each temperature treatment to estimate the effect of stress on the expression of target genes (indicated by uppercase letters). Effect sizes (ω2) were calculated to estimate the relative magnitude differences between gene expression (see Supplemental Table 2). Statistical analyses described above were performed using GraphPad Prism 8 (version 8.3.0). All data are shown as means ± SEM.
To assess inter–relationships between gene expression levels and whole–body cortisol content, data were subjected to principal component analysis (PCA) (Oblimin with Kaiser normalization) using IBM SPSS Statistics 21 (IBM, Armonk, NY, USA). The number of components to retain was based on visual inspection of the scree plot and eigenvalue (>1). Kayer–Meyer–Olkin (KMO) measure of sampling adequacy and Bartlett’s test of sphericity were run to ensure that data obey analysis criteria. Missing samples of data were excluded list–wise, with component loading cutoff point set at −0.400 or 0.400.
3 Results
3.1 Plasma Cortisol
One–day following the abrupt transfer to higher temperatures, post–smolts transferred to 1°C and 16°C displayed significantly elevated plasma cortisol concentrations compared to fish at 18°C and the 10°C control group (Figure 1). Moreover, the magnitude of differences (or effect size, ω2) in cortisol responses was relatively strong within the Transfer (1d) sampling compared to the Acclimation (45d) and ACT (1h) samplings (Supplemental Table 2). Consistently, following Acclimation (45d), plasma cortisol concentrations were low in all groups, with no detectable differences between temperature treatments. Conversely, in response to the ACTs, all temperature groups responded with large ω2 (Supplemental Table 2) and significantly elevated the circulating cortisol concentrations compared to the initial pre–ACT conditions, however, no difference in the magnitude of this response was observed between treatments (Figure 1).
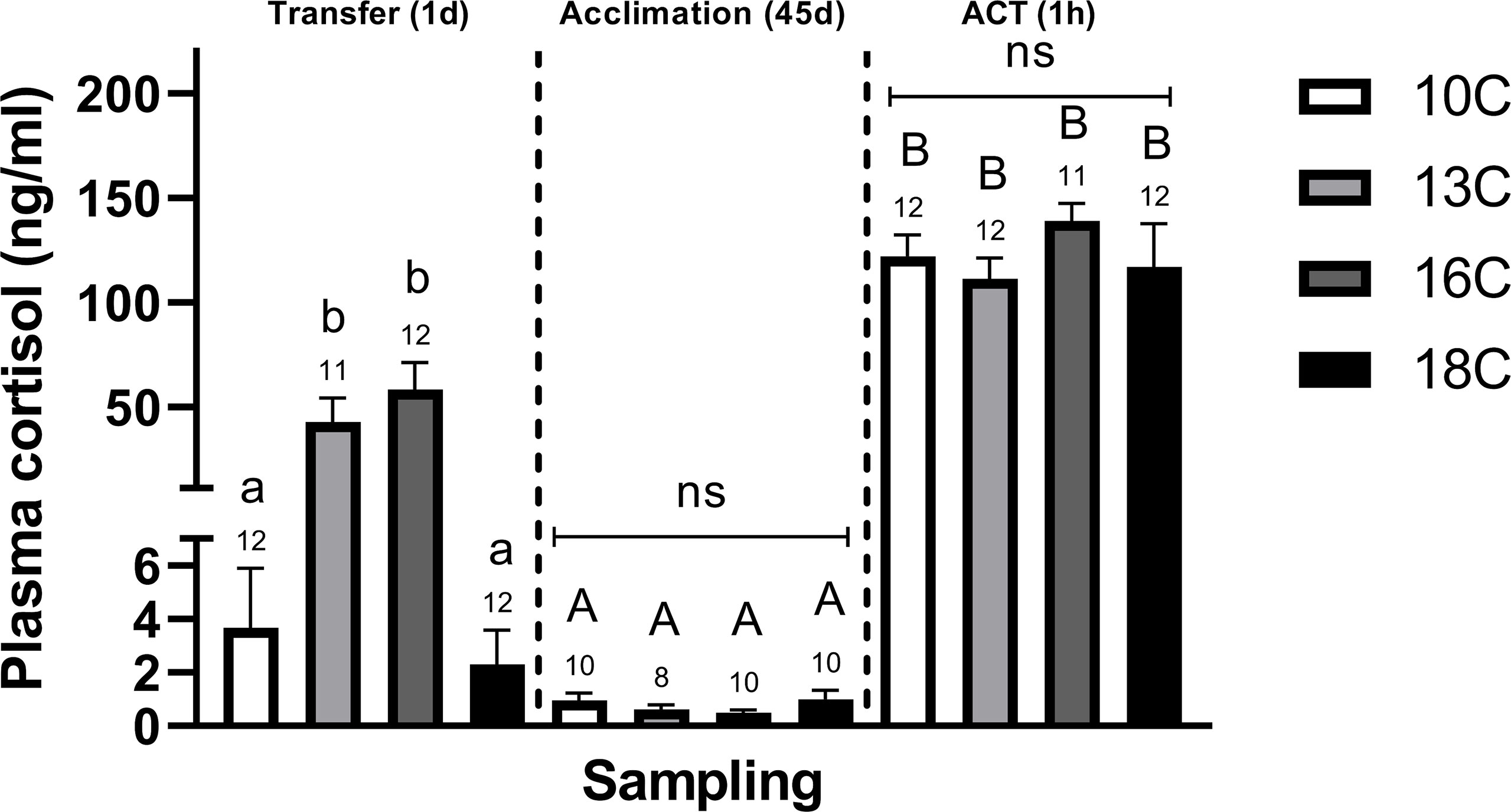
Figure 1 Differences in plasma cortisol levels in post–smolts after Transfer (1d), Acclimation (45d), and the ACT (1h). Values are presented as averages ± SEM of each treatment, with sample (n) indicated above each treatment bar of the figure. Uppercase letters indicate significant difference within a treatment between the Acclimation (45d) (non–stressed) and ACT (1h) (stressed), whereas lowercase letters indicate a significant difference between temperature treatments within a sampling. A significant difference was held at P < 0.05.
3.2 Telencephalon
3.2.1 Transfer (1d)
Stress. At Transfer (1d), both telencephalic crf paralogs (α and β) were significantly elevated in post–smolts transferred to 13°C compared to the 10°C group (Figures 2A, B), whereas only crfα (Figure 2A) showed elevation compared to the 16°C and 18°C groups. Conversely, only post–smolts transferred to 16°C and 18°C displayed significantly higher crfbp compared to the 10°C group (Figure 2C). Both hsd11b2 (α and β) paralogs were significantly lower in post–smolts transferred to 18°C compared to the 10°C and 16°C treatments (Figures 2D, E). Conversely, post–smolts transferred to 13°C had significantly higher mr (Figure 2F) and gr2α (Figure 2I) compared to fish at 10°C and 18°C, whereas gr1α (Figure 2G) was elevated in the 16°C group compared to the 10°C group. This differential regulation in CRs significantly elevated the mr/gr1β ratio (Figure 3B) in post–smolts transferred 13°C compared to the 10°C, 16°C, and 18°C groups but lowered the mr/gr1α (Figure 3A) ratio in fish transferred to 16°C compared to the 10°C group. However, the magnitude of differences revealed to be larger for the mr/gr1β ratio increased at 13°C compared to the low mr/gr1α ratio seen at 16°C (Supplemental Table 2A)
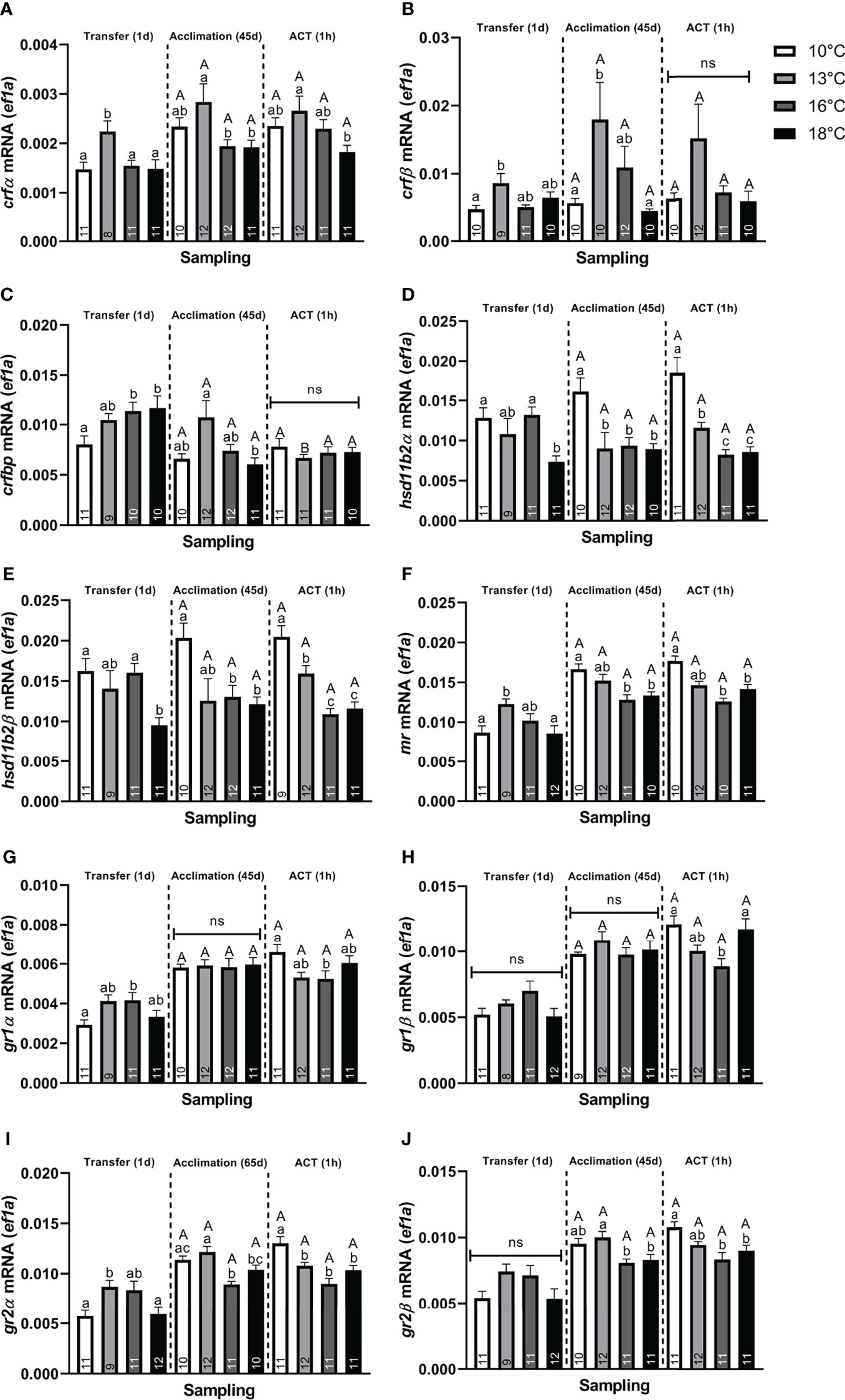
Figure 2 Changes in whole telencephalic expression of (A) corticotropin releasing factor α (crfα), (B) corticotropin releasing factor β (crfβ), (C) corticotropin releasing factor binding protein (crfbp), (D) hydroxysteroid 11–β dehydrogenase 2α (hsd11b2α), (E) hydroxysteroid 11–β dehydrogenase 2β (hsd11b2β), (F) mineralocorticoid receptor (mr), (G) glucocorticoid receptor 1α (gr1α), (H) glucocorticoid receptor 1β (gr1β), (I) glucocorticoid receptor 2α (gr2α), and (J) glucocorticoid receptor 2β (gr2β) in post–smolts at Transfer (1d), Acclimation (45d), and the ACT (1h). Values are presented as averages ± SEM of each treatment, with sample (n) indicated within each treatment bar of the figure. Uppercase letters indicate significant difference within a treatment between the Acclimation (45d) (non–stressed) and ACT (1h) (stressed), whereas lowercase letters signal a significant difference between temperature treatments within a sampling. A significant difference was held at P < 0.05.
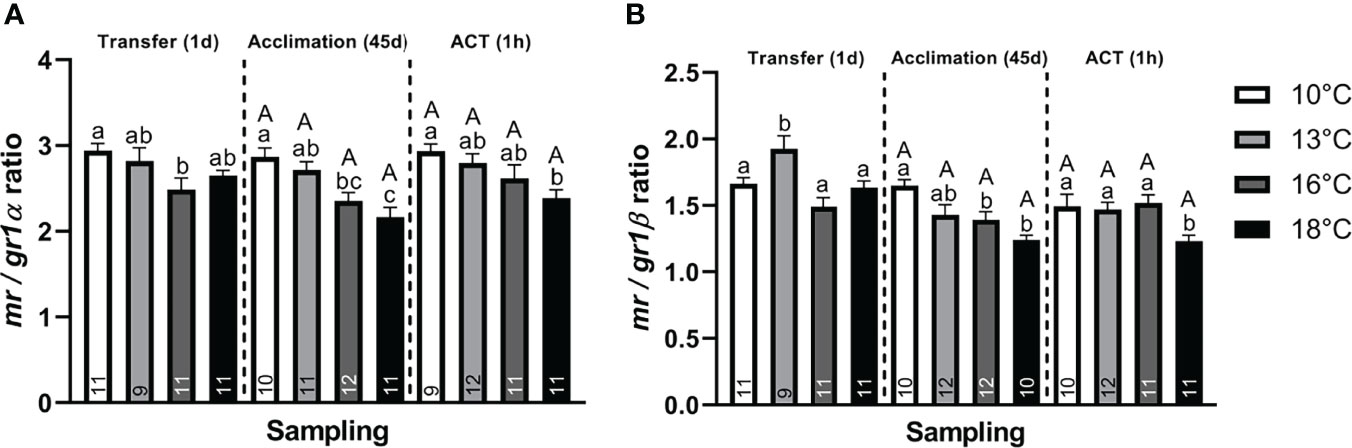
Figure 3 Change in the CR ratio for (A) mr/gr1α and (B) mr/gr1β of whole telencephalon at Transfer (1d), Acclimation (45d), and ACT (1h). Values are presented as averages ± SEM of each treatment, with sample (n) indicated within each treatment bar of the figure. Uppercase letters indicate significant difference within a treatment between the Acclimation (45d) (non–stressed) and ACT (1h) (stressed), whereas lowercase letters indicate a significant difference between temperature treatments within a sampling. A significant difference was held true at P < 0.05.
Neuroplasticity. Neural markers were also affected, with neurod (Figure 4A), showing significantly lower values in post–smolts transferred to 18°C compared to the 10°C and 13°C groups, whereas transfer to 16°C significantly lowered neurod levels compared to the 13°C group. Conversely, bdnf (Figure 4C) was significantly elevated in post–smolts transferred to 13°C compared to the 10°C group, whereas no differential regulation was observed in post–smolts exposed to the higher temperatures, 16°C and 18°C. The induction of c–fos (Figure 4D) was significantly elevated in post–smolts transferred to 13°C and 16°C, but not for fish at 18°C, compared to the 10°C group. Moreover, post–smolts transferred to 13°C showed a significantly higher c–fos response compared to the 16°C and 18°C groups. The expression of pcna was still unaffected at 1 day following the temperature transfer (Figure 4B). The magnitude of these differences seen in neural markers showed to be largest for neurod and c–fos (Supplemental Table 2A).
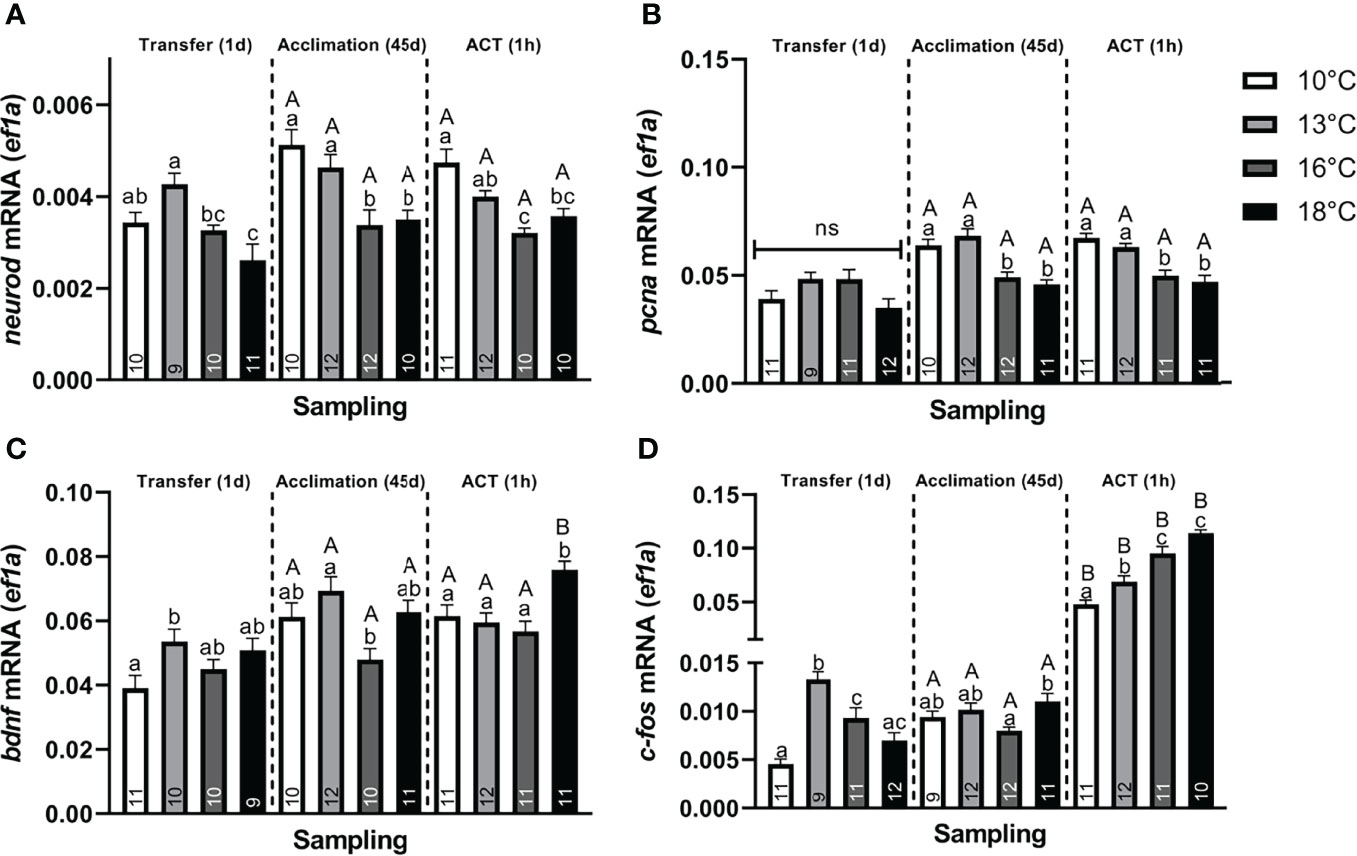
Figure 4 Changes in whole telencephalic expression of (A) neural differentiation factor D (neurod), (B) proliferating nuclear antigen (pcna), (C) brain–derived neurotrophic factor (bdnf), and (D) c–fos in post–smolts at Transfer (1d), Acclimation (45d), and ACT (1h). Values are presented as averages ± SEM of each treatment, with sample (n) indicated within each treatment bar of the figure. Uppercase letters indicate significant difference within a treatment between the Acclimation (45d) (non–stressed) and ACT (1h) (stressed), whereas lowercase letters indicate a significant difference between temperature treatments within a sampling. A significant difference was held at P < 0.05.
3.2.2 Acclimation (45d)
Stress. Following Acclimation (45d), post–smolts at 13°C showed significantly higher crf (α and β) (Figures 2A, B) and crfbp (Figure 2C) compared to fish at 18°C. Further, crfα (Figure 2A) was also higher in post–smolts acclimated to 13°C compared to the 16°C group, whereas the other paralog, crfβ (Figure 2B), showed elevation against the 10°C group. Conversely, hsd11b2 (α and β) was significantly lower in post–smolts acclimated to 16°C and 18°C compared to the 10°C group (Figures 2D, E), with hsd11b2α (Figure 2D) also showing lower levels in the 13°C group.
The CRs were also differentially regulated, with mr (Figure 2F) being significantly lower in post–smolts acclimated to 16°C and 18°C compared to the 10°C group, with both gr2 paralogs (Figures 2I, J) also showing lower levels at 16°C and 18°C compared to the 13°C group. Further, gr2α (Figure 2I) was also lower at 16°C compared to the 10°C group. In contrast, the expression of both gr1 paralogs (Figures 2G, H) was unaffected by the prevailing acclimation temperature at a steady state. This suppression in mr decreased both paralog–specific mr/gr1 ratios (Figures 3A, B) in post–smolts acclimated to 16°C and 18°C compared to the 10°C group, whereas the mr/gr1α ratio (Figure 3A) also displayed lower values in the 18°C group compared to the 13°C group. The magnitude of these differences seen in stress–related genes showed to be strong for gr2α, whereas a moderate difference prevailed for mr and both paralog–specific mr/gr1 ratios (Supplemental Table 2B).
Neuroplasticity. Post–smolts acclimated to 16°C and 18°C had significantly lower neurod (Figure 4A) and pcna (Figure 4B) compared to the 10°C and 13°C groups, whose genes showed a moderate and strong difference in magnitude, respectively (Supplemental Table 2B). Further, bdnf (Figure 4C) was significantly lower in post–smolts acclimated to 16°C compared to the 13°C group, whereas c–fos (Figure 4D) was elevated in post–smolts acclimated 18°C compared to the 16°C group.
3.2.3 ACT (1h)
Stress. The telencephalic expression of crf, hsd11b2, mr, gr2, and gr1 did not respond to the ACT when compared to the initial pre–ACT state [Acclimation (45d)] (Figures 2A, B, D–J). crfbp significantly decreased only in the stressed 13°C group compared to their respective pre–ACT state, resulting in similar levels between all stressed temperature treatments (Figure 2C). The crfα showed significantly lower expression in stressed post–smolts at 18°C compared to 13°C (Figure 2A). Conversely, hsd11b2, mr, and gr2 showed significantly lower expression in stressed post–smolts at 16°C and 18°C compared to the 10°C group (Figures 2D–F, I, J).
Further, although gr1 (Figures 2G, H) did not respond to the ACT, a differential regulation was still observed when comparing between the stressed temperature treatments. Both gr1 paralogs (α and β) showed significantly lower expression in the 16°C group compared to the 10°C group (Figures 2G, H), whereas gr1β was additionally lower compared to the 18°C group (Figure 2H). Moreover, a low mr/gr1 ratio was maintained in the stressed 18°C group compared to the 10°C group (Figures 3A, B), whereas the mr/gr1β was also lower in stressed post–smolts at 18°C compared to the stressed 13°C and 16°C groups (Figure 3B). The magnitude of these differences seen for stress–related genes when challenged by the ACTs was strongest for mr, gr2α, and hsd11b2 (α and β), whereas a moderate difference prevailed for gr2β (Supplemental Table 2C).
Neuroplasticity. In response to ACTs, no differences in neurod and pcna (Figures 4A, B) were observed between the pre–ACT and the stressed state. Therein, neurod (Figure 4A) was maintained low in stressed post–smolt at 16°C and 18°C compared to the 10°C group, with stressed post–smolts at 16°C also showing lower levels compared to 13°C group. The suppression in pcna (Figure 4B) was also maintained in stressed post–smolts at 16°C and 18°C compared to 10°C and 13°C. In contrast, bdnf (Figure 4C) significantly increased in stressed post–smolts at 18°C compared to both the respective pre–ACT state and stressed temperature groups at 10°C, 13°C, and 16°C. The c–fos levels were significantly increased in all stressed acclimation treatments compared to their initial pre–ACT state (Figure 4D). Moreover, c–fos levels were significantly and sequentially higher at each temperature increase from 10°C and 13°C, with 16°C and 18°C groups having the highest levels (Figure 4D). The magnitude of these differences in neural markers seen in stressed post–smolts was strong for neurod, pcna, and c–fos, whereas a moderate response prevailed for bdnf (Supplemental Table 2C).
3.2.4 Principal Component Analysis
We performed PCA separately for samplings Transfer (1d), Acclimation (45d), and the ACT (1h) to investigate the inter–relationship between the different stress– and neural–related genes and whole–body cortisol. The KMO test was moderate for Transfer (1d) (KMO = 0.831), Acclimation (45d) (KMO = 0.683), and ACT (1h) (KMO = 0.757), with the Bartlett’s test of sphericity indicating sufficiently large correlation between items for PCA [Transfer (1d): χ2 = 1,101.169, df = 105, P < 0.001, Acclimation (45d): χ2 = 544.605, df = 105, P < 0.001, ACT (45d): χ2 = 496.217, df = 105, P < 0.001). For the PCA on the Transfer (1d), dataset 3 components were extracted, explaining 82.9% of the variance (Table 2A), with neural plasticity markers neurod and pcna loading with stress–related genes hsd11b2 (α and β), gr1 (α and β), gr2 (α and β), mr, and crfbp into component 1, crf (α and β) and bdnf into component 2, and cortisol and c–fos loading into component 3. When temperature acclimated (45d), five components were extracted, making up 80.4% of the variance that showed greater gene separation among the PCs (Table 2B). Neural markers neurod and pcna loaded with stress–related genes gr2β, mr, and crfβ into component 1, bdnf showed to negatively load with hsd11b2 (α and β) into component 2, c–fos, gr1 (α and β), and gr2α loaded more strongly into component 3 compared to that seen for mr, gr2β, and bdnf, cortisol and crf (α and β) that loaded into component 4, while crfβ loaded together with crfbp into component 5. Following exposure to the added confinement stress, ACT (1h) (Table 2C), four components were extracted, making up 76.9% of the variance, with neurod, bdnf, gr1 (α and β), gr2 (α and β), and mr loading together into component 1, whereas neurod, pcna, and hsd11b2 (α and β) showed negative loading with c–fos and bdnf into component 2, crf and crfbp loading together into component 3, and cortisol loading alone in component 4.
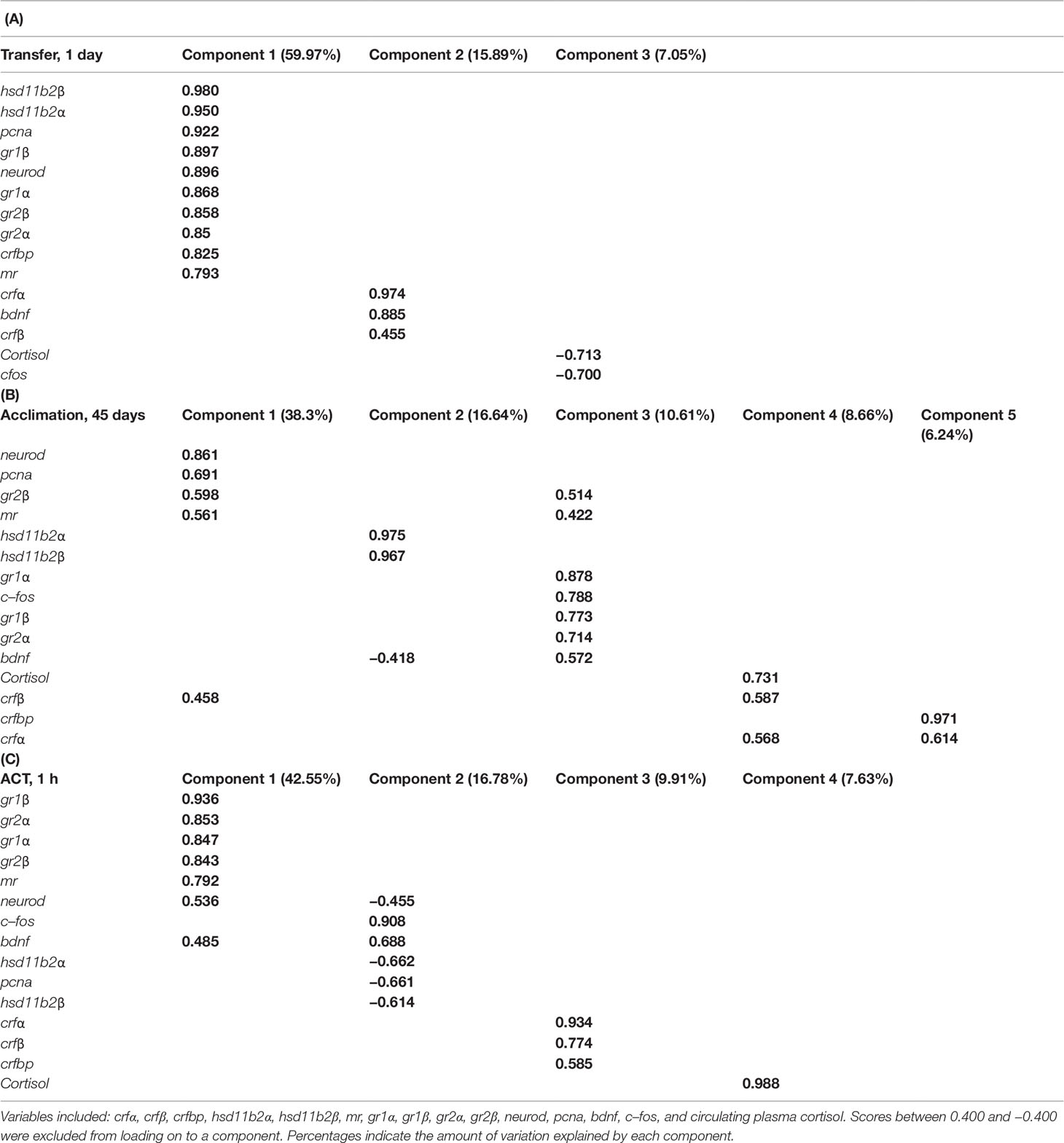
Table 2 Variables loaded onto components in principal component analysis (PCA) within samplings: (A) Transfer (1d), (B) Acclimation (45d), and (C) ACT (1h).
4 Discussion
The inter–relationship between the stress axis, neural activation, and cognitive plasticity for stress resilience is well documented and provides important underlying information as to the degree of stress being experienced (Sørensen et al., 2011; Sørensen et al., 2012; Sørensen et al., 2013; Manuel et al., 2014b; Madaro et al., 2015). Still, it is well noted that both early life experiences and circadian rhythm can differentially influence stress responses and brain plasticity, therefore, when interpreting changes in these parameters, such implications should be kept in mind (Auperin and Geslin, 2008; Ebbesson and Braithwaite, 2012; Sánchez–Vázquez et al., 2019). Further, it is important to clarify that the work done here in interpreting relative transcript abundance does not always translate to the protein abundances. This is due to differences in protein turnover rates and a multitude of regulatory possibilities at transcriptional, translational, and post–translational levels (Maier et al., 2009), therefore, caution is advised when interpreting these results. Our findings reveal that transfer to elevated temperatures may contain welfare concerns for post–smolt Atlantic salmon, with fish transferred to 16°C and 18°C, experiencing largest challenges and allostatic loads. These groups displayed a suppression in stress responses to the abrupt temperature transfer, whereas after acclimation, although cortisol responses to ACTs were maintained similar, neural activation (c–fos) to ACTs was elevated with increasing acclimation temperature, alongside an upregulation in bdnf at 18°C. Moreover, telencephalic CR profiles were disturbed, showing an elevation in gr1α and suppression in hsd11b2 when initially transferred to 16°C and 18°C, respectively, whereas a suppression in both mr and hsd11b2 persisted following acclimation to these temperatures. This lowered the mr/gr1α ratio when initially transferred to 16°C, whereas, following acclimation, both paralog–specific mr/gr1 ratios were lowered at 16°C and 18°C. Together with the suppression in hsd11b2 seen at elevated temperatures, suggesting lower inhibition of cortisol action, these CR–related disturbances could be the reason of stress–induced disparities in receptor stimulation when exposed to 16°C and 18°C. Consistently, this coincided with a suppression in telencephalic neurod and pcna at 16°C and 18°C after acclimation, suggesting impacts on telencephalic neural processes. Taken together, post–smolt transfer to 16°C and 18°C cues improper stress responses and telencephalic functions, which could propose for inferior underlying cognitive capabilities and elevated stress loads, and has potential implications for post–smolt welfare. However, it should be noted that no mortalities were observed during the trials, reasoning that fish still tolerate these temperatures at least within the time frame studied.
4.1 Temperature Transfer
Rapid thermal changes can have considerable homeostatic impacts in ectotherms, which, depending on severity, can either inhibit or maintain a capacity to mount a proper response to the challenge (Ebbesson and Braithwaite, 2012). If the stressor severity becomes overwhelming (allostatic overload), then this may detrimentally impact physiology by suppressing functions and reducing capacities to manage added challenges (Ebbesson and Braithwaite, 2012; Madaro et al., 2015; Samaras et al., 2018).
4.1.1 Telencephalic Regulation
Temperature modifications to behavior and circulating cortisol profiles have been evidenced in various teleost species (Lankford et al., 2003; Davis, 2004; Madaro et al., 2018), however, few studies have addressed the potential mechanisms behind these divergences and their implication on key neural processes of the telencephalon. Our findings show that post–smolts abruptly transferred from 10°C to warmer temperatures, 13°C and 16°C, maintained elevated plasma cortisol concentrations, whereas fish transferred to 18°C lacked a cortisol response altogether (Figure 1). Similarly, telencephalic c–fos (Figure 4D) was elevated in the 13°C and 16°C groups, with post–smolts transferred to 13°C having the strongest response. This implies that the stress response is still well retained when transferred to 13°C, while a suppression begins to manifest at 16°C and becomes absent at 18°C. Similar cortisol responses are demonstrated in chronically stressed Atlantic salmon (Madaro et al., 2015). Consistent with this notion, post–smolts transferred to 16°C and 18°C upregulated telencephalic crfbp (Figure 2C), suggesting a downregulation in the crf system, a typical indicator of a suppressed stress response (Manuel et al., 2014b; Gorissen and Flik, 2016; Samaras et al., 2018). In contrast, crf (Figures 2A, B) was elevated in post–smolts transferred to 13°C, supporting a still active crf system that aligns to the largest stress responses seen at this transfer temperature (Gorissen and Flik, 2016). Taken together, these findings suggest impacted stress response capacities in post–smolts when transferred to 16°C and 18°C, with the latter group resembling physiological indices coherent to chronically stressed fish (Madaro et al., 2015; Samaras et al., 2018), that could suggest allostatic loads are beginning to surpass the animal’s reactive scope (Romero et al., 2009).
This differential regulation in stress responses also coincided with alterations to CR profiles, with the 13°C group displaying elevated mr and gr2α (Figures 2F, I), the 16°C group showing an upregulation in gr1α (Figure 2G), whereas, surprisingly, CRs were less affected in post–smolts transferred to 18°C. Moreover, this differential regulation in CRs was associated with an elevated mr/gr1β ratio (Figure 3B) in post–smolts transferred to 13°C, whereas fish at 16°C had a lower mr/gr1α ratio (Figure 3A). Collectively, these CR changes could cue an upregulation in mr– and gr1–related functions when transferred to 13°C and 16°C, respectively. Elevated mr at 13°C may also imply that stress conditions are still tolerable, considering the sensitivity of this receptor to cortisol stimulation (Stolte et al., 2008), suggesting that the beneficial physiological effects of mr–related signaling may still be retained to cope with stress loads at this transfer temperature (Karst et al., 2005). Conversely, larger gr1 activation, as suggested by elevated gr1α at 16°C (Figure 2G), is a common indice of elevated cortisol stimulation that could represent a larger demand to suppress tissue functions and prevent overstimulation (Stolte et al., 2008), reasoning elevated challenges. Alternatively, the decrease in mr/gr1 ratio (Figure 3A) seen at 16°C may also propose an attempt to reduce mr bioavailability, perhaps to limit larger disparities in receptor stimulation as stress loads accumulate. In contrast, fish transferred to 18°C, having a suppressed CRF system (increased crfbp) (Figure 2C) and absent stress responses (cortisol and c–fos) (Figures 1, 4D), may explain the limited modulations in CRs as cortisol–related stimulation is readily retained low, thereby lowering the demand to regulate receptor functions. In support, the downregulation in hsd11b2 at 18°C (Figures 2D, E) could propose a lower need to inhibit cortisol action (Alikhani–Koopaei et al., 2004; Alderman and Vijayan, 2012). In summary, these telencephalic indices in stress–related genes at 18°C also potentially suggest a convergence toward a reduced reactive scope, with the 16°C group showing an intermediate response (Romero et al., 2009). Contrastingly, post–smolts going from 10°C to 13°C seemingly still retain proper stress responses and telencephalic capacities, cueing manageable stress loads.
4.1.2 Regulation of Neural Plasticity and Neurogenesis
Cognition plays key roles in fish stress resilience, in which mild or severe challenges can evoke rapid changes in neural plasticity that modulate cognitive functions either detrimentally or beneficially (Ebbesson and Braithwaite, 2012). Post–smolts elevated bdnf when rapidly transferred to 13°C, in line with our recent findings observing a similar response in fish when exposed from 13°C down to 7°C and 4°C (Tang et al., 2022). This supports the importance of BDNF in managing rapid onsets in stress (Vindas et al., 2017). Elevated bdnf may help resist the adverse effects of cortisol overstimulation on neural networks to preserve neuronal integrity, thus presenting a surprisingly robust response (Vindas et al., 2017). Consistently, bdnf has also shown to promote rapid shifts in behavior to benefit stress resilience under large allostatic loads, as reported for inhibitory behavioral phenotypes (Soliman et al., 2010; Manuel et al., 2014a). Nevertheless, although alteration of bdnf is robust, it also implies a larger demand to resist more adverse stress loads, logically suggesting that rapid temperature elevations are stressful for post–smolts. Surprisingly, in line with the stress response and CR patterns (see Section 4.1.1.), bdnf showed a limited differential regulation in post–smolts transferred to 16°C and 18°C (Figure 4C) despite facing a greater thermal challenge. This could suggest greater challenges in line with the downregulation in stress response capacities seen to begin at 16°C and worse at 18°C (see Section 4.1.1.). Alternatively, it cannot be ruled out that suppressed stress functions at 16°C and 18°C may lessen the need to regulate bdnf to protect neuronal circuits, e.g., if cortisol–related stimulation is retained low. However, because allostatic overloads are well recognized to hampering neural functions (Korte et al., 2007; Romero et al., 2009), this still remains a viable alternative for this limited bdnf regulation seen at 16°C and 18°C (Figure 4C). In support, post–smolts transferred to 16°C and, especially, 18°C also displayed a suppression in neurod (Figure 4A), suggesting a declining pool of differentiating neurons and reduced neural plasticity. Because of the importance of neurod in memory and learning (Salvanes et al., 2013; Grassie et al., 2013), this could argue an inferior cognitive capacity in post–smolts to manage the prevailing stress loads when transferred to 16°C and 18°C. This starkly contrasts post–smolts transferred to 13°C, which still maintained or elevated these neural plasticity markers (Figures 4A–C). Taken together, these findings suggest that the transfer of post–smolts to 13°C shows indices of superior cognitive capacities compared to 16°C and 18°C, which, in part, may be due to larger impacts of stress loads on telencephalic neural processes at these higher temperatures.
Stress acts through the actions of CRs to modify neural plasticity, with prior reports demonstrating stimulatory effects of mr, such as for neurod (Van Weert et al., 2017; van Weert et al., 2019) and bdnf (Suri and Vaidya, 2013), whereas, in contrast, gr1 being inhibitory (Suri and Vaidya, 2013; Pavlidis et al., 2015; Chen et al., 2017). In line with this, our findings reveal stress–related elevation in mr and the mr/gr1β ratio (Figures 2F, 3B) when transferring post–smolts to 13°C that coincidently maintained neurod (Figure 4A) and elevated bdnf (Figure 4C). However, it should not be ruled out that crf may also play roles in neural regulation, for instance, bdnf grouped strongly with crf in PC2 of the PCA (Table 2A). Nevertheless, these effects inversely mirrored that seen in neural plasticity for post–smolts transferred to 16°C, having elevated gr1α (Figure 2G), and suppressed hsd11b2 at 18°C (Figures 2D, E). In particular, the downregulation in hsd11b2 (Figures 2D, E) at 18°C coincided with the strongest suppression in neurod (Figure 4A), supporting prior evidence that hsd11b2 plays important functions in maintaining proper neural development (Alderman and Vijayan, 2012). Conforming to the inter–relationship between stress–related genes and neural plasticity, the PCA results of Transfer (1d) revealed a strong loading of neural plasticity markers with these regulatory genes in the same component (PC1) that explained a large portion of the variance (59.97%) (Table 2A). Conversely, cortisol and c–fos grouped together in component 3, revealing a negative loading compared to the other components, perhaps supporting a similar but opposing trend when compared neural plasticity and their regulatory genes (Table 2A). Still, although these findings point to the importance of these stress–related genes in regulating neural plasticity, further mechanistic studies are required, considering the complexity of these neural pathways. In summary, these data suggest that post–smolt transfer from 10°C to 16°C and 18°C may impede vital telencephalic functions, whereas transfer to 13°C revealed to be more favorable in sustaining stress and telencephalic responses needed to promote stress resilience and adaptation to the challenge.
4.2 Acclimation (45d) and Responses to ACTs (1h)
Following long–term exposure to the thermal stressor, if the accumulation of challenges is still tolerable, then neural adjustments become crucial for sustaining homeostasis and progressing challenge adaptation to manage persisting allostatic loads (Ebbesson and Braithwaite, 2012). Therefore, differences in these neural adaptations can indicate varying stress loads and impeded welfare, especially if neural adjustments become suppressed.
4.2.1 Telencephalic Regulation
When acclimated, cortisol concentrations were basal, indicating recovery from the initial temperature transfer period (Figure 1). Further, in response to the ACTs, all treatments responded with elevated cortisol levels, however, no effect of temperature was observed on the circulating levels (Figure 1). This starkly contrasts our previous work (Tang et al., 2022) and others (Madaro et al., 2018), demonstrating a suppression in cortisol responses when acclimating post–smolts to temperatures below 13°C. However, the present findings are consistent with a prior study in Atlantic salmon, similarly reporting limited modulation in stress–related cortisol release above 12°C (Madaro et al., 2018). Contrasting the cortisol responses, the telencephalic induction of c–fos (Figure 4D) to the ACTs increased with increasing acclimation temperature, suggesting larger neural activation to stress at elevated temperatures. As the induction of c–fos is noted for its dependence on stress/stimulus strength (Kovács, 1998) and elevation during both acute and chronic stresses (Pavlidis et al., 2015), this could imply that post–smolts experience greater stress loads when challenged at elevated temperatures, 16°C and 18°C (Figure 4D), despite not translating to greater circulating cortisol levels. Therefore, these findings demonstrate c–fos as a relevant stress marker, which, when used in tandem with cortisol, should be considered in future stress studies.
A dual elevation in both crf and crfbp (Figures 2A–C) was observed in post–smolts acclimated to 13°C. However, in line with the limited differences in cortisol levels at a steady state, no clear differential regulation between the acclimation treatments was detected for crf and crfbp, suggesting that the CRF system at least within the telencephalon was unaffected by prevailing acclimation temperature at a steady state. Conversely, in response to the ACTs, crfbp (Figure 2C) was downregulated in post–smolts acclimated to 13°C, whereas the elevated levels of crfα (Figure 2A) were still maintained. This lower abundance of crfbp when stressed at 13°C could serve to enhance the telencephalic activation of the CRF system to manage neural capacities (Gorissen and Flik, 2016). However, as no variation in the cortisol levels (Figure 1) were observed when stressed, the reason behind upregulating this system in the telencephalon requires further study. In contrast, post–smolts acclimated to 16°C and 18°C showed a direct suppression in gr2 (Figures 2I, J), mr (Figure 2F), and lower mr/gr1 ratio (Figures 3A, B). This may suggest that fish acclimated to 16°C and 18°C retain perturbations and disparities in stimulation of mr– and gr2–related functions, which are suppressed as a protective mechanism (Madaro et al., 2015). Moreover, the lower mr/gr1 ratio at 16°C and 18°C (Figures 3A, B) may be seen as attempt to downplay mr–related functions or alternatively enhance the bioavailability of gr1 for stimulation to suppress and preserve telencephalic neural processes from elevated stress loads (Stolte et al., 2008). Further, the hsd11b2 (Figures 2D, E) became co–suppressed with mr (Figure 2F) at 16°C and 18°C, which supports prior work describing a tight regulatory link of hsd11b2 over mr–related action (Alikhani–Koopaei et al., 2004). Thus, the suppression in hsd11b2 at 16°C and 18°C may reflect a repercussion to the stress–related suppression in mr, inherently reducing the demand to maintain elevated hsd11b2 (Alderman and Vijayan, 2012). Taken together, these perturbations in stress–related genes, mr, gr2, and hsd11b2, when acclimated to 16°C and 18°C, cue greater disparities in telencephalic regulation, suggesting a continuation of challenges even after acclimation. Further, considering the importance of mr and gr2 in stimulating neural capacities (Karst et al., 2005), perturbations in these may inadvertently inflict disruptions to telencephalic processes, such as neural plasticity and neurogenesis, and implicate welfare.
4.2.2 Regulation of Neural Plasticity and Neurogenesis
Consistent with the seen perturbations in CRs (Section 4.2.1.), acclimating post–smolts to 16°C and 18°C suppressed telencephalic neurod (Figure 4A) and pcna (Figure 4B), suggesting stress–related impacts on neural differentiation and neurogenesis (Sørensen et al., 2011; Sørensen et al., 2012). Such inhibition of neural functions can impair cognition, due to the well noted roles of these processes in memory and learning (Grassie et al., 2013; Alfonso et al., 2019). Further, these hampered indices of neural plasticity at 16°C and 18°C aligned with the largest c–fos induction when stressed by the ACTs (Figure 4D) (Section 4.2.1.) (Kovács, 1998), which may suggest thermal–related disruptions in telencephalic functions. In support, post–smolts acclimated to 18°C also elevated bdnf (Figure 4C) when challenged by the ACT, cueing a larger demand to protect neuronal circuits from cortisol or shift behavioral phenotypes to adjust to added stress loads, suggesting elevated stress loads to added challenges (Manuel et al., 2014a; Vindas et al., 2017; Linz et al., 2019).
It is noteworthy that the levels of neurod and pcna ran anti–parallel to the stress–induced telencephalic induction of c–fos to ACTs at 16°C and 18°C (Figures 4A, B, D). Although still warranting further study, prior reports in mammals demonstrate the importance of c–fos for synaptic plasticity of learning and memory processes (Guzowski et al., 2001; Fleischmann et al., 2003). Therefore, it is tempting to speculate whether the larger activation of c–fos could relate to attempts to support neural plasticity if dysfunctions in these processes begin emerging in post–smolts at elevated temperatures. Still, it should not be ruled out that the larger induction of telencephalic c–fos may also represent alternative mechanisms to activate other neural pathways to satisfy telencephalic requirements and abilities when challenged at these more stressful temperatures. For example, the importance of c–fos activation is also noted for hippocampal–dependent spatial and fear memory (Katche and Medina, 2017) and could align to the elevation in bndf when stressed at 18°C, which is specifically noted for roles in fear avoidance in fish (Manuel et al., 2014a). Consistently, when stressed, both neurod and pcna negatively loaded with c–fos and bdnf into PC2, suggesting opposing response effects (Table 2C). Still, because of our rudimentary understanding over IEG pathways in fish, further mechanistic study is required.
Reminiscent to the initial transfer phase (Section 4.1.2.), the suppression in pcna and neurod (Figures 4A, B) when acclimated to 16°C and 18°C coincided with the suppression in gr2 (α and β) and mr and lower mr/gr1 ratio (Figures 2F, I ,J, 3A, B), providing further support to the consensus that stress acts through CRs to modify neural processes (Sørensen et al., 2013; Sadoul et al., 2018). This is also supported by the PCAs showing that mr and gr2β loaded strongly with neural markers neurod and pcna in PC1 (Table 2B). This is consistent with prior evidence in mammals and fish, demonstrating a tight coupling of mr over neurod potentiation, with this study also pointing to the importance of gr2 (van Weert et al., 2019; Alfonso et al., 2019). Moreover, it is not inconceivable that similar regulatory mechanisms may also exist for pcna in the fishes (Le Menuet and Lombès, 2014). The importance of hsd11b2 for neural processes should also not be undermined as these findings reveal post–smolts acclimated to 16°C and 18°C to having suppressed telencephalic hsd11b2 (α and β) (Figures 2D, E), coinciding with both suppressed mr and gr2 (Figures 2F, I, J) and neural plasticity (neurod and pcna) (Figures 4A, B). This supports reports localizing this enzyme in the neurogenic regions of the telencephalon that argues an importance in limiting discrepancies in CR activity to prevent untimely neural differentiation (Alderman and Vijayan, 2012). In support, hsd11b2, pcna, and neurod loaded negatively together in PC2 of stressed post–smolts (Table 2C). Nevertheless, it still should not be ruled out that the lower mr/gr1 ratio (Figures 3A, B) at elevated temperatures may also progress the physiological bioavailability GR1 over neural functions (De Kloet et al., 2005; Sørensen et al., 2013; Odaka et al., 2017). In line, studies support the importance of full GR activation for IEG induction (Gutièrrez–Mecinas et al., 2011; Mifsud and Reul, 2018), which may alternatively account for the larger induction of c–fos (Figure 4D) at elevated temperatures. In line with this, although not seen when stressed, c–fos loaded strongly with gr1 (α and β) and gr2α in PC3 in non–stressed acclimated fish (Table 2B). Still, due to the complexity of these regulatory pathways, further studies are warranted.
In summary, acclimation to 16°C and 18°C lowered the telencephalic expression of genes related to the CRF system (crf αβ and crfbp) while suppressing mr and gr2 (α and β) and lowered the mr/gr1 ratio. These coincided with downregulation in neural plasticity markers neurod and pcna while elevating c–fos induction to added stress at 16°C and 18°C. Together, these indices suggest that challenges are sustained in post–smolts when acclimated to 16°C and 18°C, which may impede proper neural development and have consequences for welfare. Therefore, further investigation is warranted on these temperature–related functional and neuro–anatomical effects and their consequences for cognitive functions.
5 Conclusions
This study shows that temperature elevations to 16°C and 18°C impair post–smolt stress responses and telencephalic functions, which may contain long–lasting challenges and elevated allostatic loads. The rapid transfer from 10°C to 16°C and 18°C revealed dampened telencephalic responses for c–fos, which, at 18°C, also presented as suppressed cortisol concentrations. These could cue stress–related reductions in stress response capabilities to handle challenges when exposed to these thermal transfers. Further, post–smolt transfer at 16°C contained elevated gr1α and a lower mr/gr1α ratio, proposing larger stress loads. However, abrupt transfer to 18°C showed the most adverse indices, indicating suppressed neural plasticity in addition to the absent stress responses (cortisol, c–fos), which commonly mimic physiological indices of chronic stress. On the other hand, the abrupt transfer of post–smolts to 13°C still robustly retained telencephalic capacities, which were even enhanced toward the thermal challenge, indicating that stress loads can be dealt with physiologically. Following acclimation, the regulation of telencephalic CRs converged to a direct suppression in mr and gr2 (α and β) and a lower mr/gr1 ratio in post–smolts at 16°C and 18°C. This implies that stress–related disparities in CR functions hold standfast even after apparent acclimation to these temperatures, supporting long–lasting stress–related effects at 16°C and 18°C. Moreover, these coincided with a suppression in neurod and pcna, suggesting a reduction in neural plasticity and neurogenesis, respectively, that plausibly imply inferior adaptation and cognitive abilities. Consistently, the induction of c–fos to ACTs was elevated at 16°C and 18°C, suggesting larger stress loads, with fish acclimated to 18°C, additionally showing elevating the level of bdnf, implying an added level of challenge. Taken together, our data suggest that post–smolt transfer from 10°C to 16°C and 18°C should be avoided due to indices of larger stress–related dysfunctions in the telencephalon that may cue compromised cognitive functions that are vital for stress resilience and welfare.
Data Availability Statement
The original contributions presented in the study are included in the article/Supplementary Material. Further inquiries can be directed to the corresponding author.
Ethics Statement
The animal study was reviewed and approved by Norwegian Food Safety Authority.
Author Contributions
TP performed conceptualization, and data acquisition, analysis and interpretation, followed by producing the original manuscript draft and revision. SS was involved in the revision of the manuscript. GN were involved in conception of design and acquisition of data, manuscript revision. NT was involved in conception of the design, and manuscript revision. GM was involved in acquisition of data and manuscript revision. EE was involved in conceptualization, and data analysis and interpretation and revision of manuscript. All authors contributed to the article and approved the submitted version.
Funding
This study was funded by the Norwegian Research Council (Grant/Award Number: 237856/O30) apart of the Centre for Research–based Innovation, CtrlAQUA. Additional funding was provided by Norwegian Research Centre AS (NORCE) and University of Bergen.
Conflict of Interest
The authors declare that the research was conducted in the absence of any commercial or financial relationships that could be construed as a potential conflict of interest.
Publisher’s Note
All claims expressed in this article are solely those of the authors and do not necessarily represent those of their affiliated organizations, or those of the publisher, the editors and the reviewers. Any product that may be evaluated in this article, or claim that may be made by its manufacturer, is not guaranteed or endorsed by the publisher.
Acknowledgments
We would like to give special thanks to Egor Gaidukov and Valentina Tronci for their valuable contribution and participation in experimental work and data acquisition.
Supplementary Material
The Supplementary Material for this article can be found online at: https://www.frontiersin.org/articles/10.3389/fmars.2022.926136/full#supplementary-material
References
Alderman S. L., Vijayan M. M. (2012). 11β–Hydroxysteroid Dehydrogenase Type 2 in Zebrafish Brain: A Functional Role in Hypothalamus–Pituitary–Interrenal Axis Regulation. J. Endocrinol. doi: 10.1530/JOE-12-0379
Alfonso S., Sadoul B., Gesto M., Joassard L., Chatain B., Geffroy B., et al. (2019). Coping Styles in European Sea Bass: The Link Between Boldness, Stress Response and Neurogenesis. Physiol. Behav. 207, 76–85. doi: 10.1016/j.physbeh.2019.04.020
Alikhani–Koopaei R., Fouladkou F., Frey F. J., Frey B. M. (2004). Epigenetic Regulation of 11β–Hydroxysteroid Dehydrogenase Type 2 Expression. J. Clin. Invest. 114(8), 1146–1157. doi: 10.1172/JCI21647
Auperin B., Geslin M. (2008). Plasma Cortisol Response to Stress in Juvenile Rainbow Trout is Influenced by Their Life History During Early Development and by Egg Cortisol Content. Gen. Comp. Endocrinol. 158 (3), 234–239. doi: 10.1016/j.ygcen.2008.07.002
Baker B. I., Bird D. J., Buckingham J. C. (1996). In the Trout, CRH and AVT Synergize to Stimulate ACTH Release. Regul. Pept., 67(3), 207–210. doi: 10.1016/S0167-0115(96)00130-9
Bartolini T., Butail S., Porfiri M. (2015). Temperature Influences Sociality and Activity of Freshwater Fish. Environ. Biol. Fishes. 98 (3), 825–832. doi: 10.1007/s10641-014-0318-8
Beemelmanns A., Zanuzzo F. S., Xue X., Sandrelli R. M., Rise M. L., Gamperl A. K. (2021). The Transcriptomic Responses of Atlantic Salmon (Salmo Salar) to High Temperature Stress Alone, and in Combination With Moderate Hypoxia. BMC Genomics 22, 1–33. doi: 10.1186/s12864-021-07464-x
Bourret S. L., Caudill C. C., Keefer M. L. (2016). Diversity of Juvenile Chinook Salmon Life History Pathways. Rev. Fish. Biol. Fish. 26 (3), 375–403. doi: 10.1007/s11160-016-9432-3
Chen H., Lombès M., Le Menuet D. (2017). Glucocorticoid Receptor Represses Brain–Derived Neurotrophic Factor Expression in Neuron–Like Cells. Mol. Brain. 10, 12. doi: 10.1186/s13041-017-0295-x
Davis K. B. (2004). Temperature Affects Physiological Stress Responses to Acute Confinement in Sunshine Bass (Morone Chrysops×Morone Saxatilis). Comp. Biochem. Physiol. – A Mol. Integr. Physiol. 139(4), 433–440. doi: 10.1016/j.cbpb.2004.09.012
De Kloet E. R., Joëls M., Holsboer F. (2005). Stress and the Brain: From Adaptation to Disease. Nat. Rev. Neurosci. 6: 463–475. doi: 10.1038/nrn1683
Ebbesson L. O. E., Braithwaite V. A. (2012). Environmental Effects on Fish Neural Plasticity and Cognition. J. Fish. Biol. 81 (7), 2151–2174. doi: 10.1111/j.1095-8649.2012.03486.x
Fleischmann A., Hvalby O., Jensen V., Strekalova T., Zacher C., Layer L. E., et al. (2003). Impaired Long–Term Memory and NR2A–type NMDA Receptor–Dependent Synaptic Plasticity in Mice Lacking C–Fos in the CNS. J. Neurosci. 23 (27), 9116–9122. doi: 10.1523/JNEUROSCI.23-27-09116.2003
Flik G., Klaren P. H. M., Van Den Burg E. H., Metz J. R., Huising M. O. (2006). CRF and Stress in Fish. Gen. Comp. Endocrinol. 146(1): 36–44 doi: 10.1016/j.ygcen.2005.11.005
Friedland K. D. (1998). Ocean Climate Influences on Critical Atlantic Salmon (Salmo Salar) Life History Events. Can. J. Fish. Aquat. Sci. 55 (SUPPL.1), 119–130. doi: 10.1139/d98-003
Fryer J., Lederis K., Rivier J. (1984). Cortisol Inhibits the ACTH–releasing Activity of Urotensin I, CRF and Sauvagine Observed With Superfused Goldfish Pituitary Cells. Peptides. 5(5): 925–930, doi: 10.1016/0196-9781(84)90118-9
Gallant M. J., LeBlanc S., MacCormack T. J., Currie S. (2017). Physiological Responses to a Short–Term, Environmentally Realistic, Acute Heat Stress in Atlantic Salmon, Salmo Salar. Facets. 2 (1), 330–341. doi: 10.1139/facets-2016-0053
Goikoetxea A., Sadoul B., Blondeau–Bidet E., Aerts J., Blanc M. O., Parrinello H, et al. (2021). Genetic Pathways Underpinning Hormonal Stress Responses in Fish Exposed to Short– and Long–Term Warm Ocean Temperatures. Ecol. Indic. 120, 106937. doi: 10.1016/j.ecolind.2020.106937
Gonçalves J., Carraça S., Damasceno–Oliveira A., Fernández–Durán B., Diaz J., Wilson J., et al. (2006). Effect of Reduction in Water Salinity on Osmoregulation and Survival of Large Atlantic Salmon Held at High Water Temperature. N Am. J. Aquac. 68 (4), 324–329. doi: 10.1577/A05-056.1
Gorissen M., Flik G. (2016). The Endocrinology of the Stress Response in Fish: An Adaptation–Physiological View. Fish. Physiol. 35, 75–111. doi: 10.1016/B978-0-12-802728-8.00003-5
Grassie C., Braithwaite V. A., Nilsson J., Nilsen T. O., Teien H. C., Handeland S. O., et al. (2013). Aluminum Exposure Impacts Brain Plasticity and Behavior in Atlantic Salmon (Salmo Salar). J. Exp. Biol. 216 (16), 3148–3155. doi: 10.1242/jeb.083550
Gutièrrez–Mecinas M., Trollope A. F., Collins A., Morfett H., Hesketh S. A., Kersanté F, et al. (2011). Long–lasting Behavioral Responses to Stress Involve a Direct Interaction of Glucocorticoid Receptors With ERK1/2–MSK1–Elk–1 Signaling. Proc. Natl. Acad. Sci. U.S.A. 108 (33), 13806–13811. doi: 10.1073/pnas.1104383108
Guzowski J. F., Setlow B., Wagner E. K., McGaugh J. L. (2001). Experience–dependent Gene Expression in the Rat Hippocampus After Spatial Learning: A Comparison of the Immediate–Early Genes Arc, C–Fos, and Zif268. J. Neurosci. 21 (14), 5089–5098. doi: 10.1523/JNEUROSCI.21-14-05089.2001
Handeland S. O., Björnsson B. T., Arnesen A. M., Stefansson S. O. (2003). Seawater Adaptation and Growth of Post–Smolt Atlantic Salmon (Salmo Salar) of Wild and Farmed Strains. Aquaculture 220 (1–4), 367–384. doi: 10.1016/S0044-8486(02)00508-2
Huising M. O., Metz J. R., van Schooten C., Taverne–Thiele A. J., Hermsen T., Verburg–van Kemenade B. M. L., et al. (2004). Structural Characterisation of a Cyprinid (Cyprinus Carpio L.) CRH, CRH–BP and CRH–R1, and the Role of These Proteins in the Acute Stress Response. J. Mol. Endocrinol. 32 (3), 627–648. doi: 10.1677/jme.0.0320627
Jarungsriapisit J., Moore L. J., Taranger G. L., Nilsen T. O., Morton H. C., Fiksdal I. U., et al. (2016). Atlantic Salmon (Salmo Salar L.) Post–Smolts Challenged Two or Nine Weeks After Seawater–Transfer Show Differences in Their Susceptibility to Salmonid Alphavirus Subtype 3 (SAV3). Virol. J. 13 (1), 1–14. doi: 10.1186/s12985–016–0520–8
Karst H., Berger S., Turiault M., Tronche F., Schütz G., Joëls M. (2005). Mineralocorticoid Receptors are Indispensable for Nongenomic Modulation of Hippocampal Glutamate Transmission by Corticosterone. Proc. Natl. Acad. Sci. U.S.A. 102 (52), 19204–19207. doi: 10.1073/pnas.0507572102
Katche C., Medina J. H. (2017). Requirement of an Early Activation of BDNF/c–Fos Cascade in the Retrosplenial Cortex for the Persistence of a Long–Lasting Aversive Memory. Cereb Cortex. 27 (2), 1060–1067. doi: 10.1093/cercor/bhv284
Kiilerich P., Kristiansen K., Madsen S. S. (2007). Hormone Receptors in Gills of Smolting Atlantic Salmon, Salmo Salar: Expression of Growth Hormone, Prolactin, Mineralocorticoid and Glucocorticoid Receptors and 11β–Hydroxysteroid Dehydrogenase Type 2. Gen. Comp. Endocrinol. 152 (2–3), 295–303. doi: 10.1016/j.ygcen.2006.12.018
Korte S. M., Olivier B., Koolhaas J. M. (2007). A New Animal Welfare Concept Based on Allostasis. Physiol. Behav. 92 (3), 422–428 doi: 10.1016/j.physbeh.2006.10.018
Kovács K. J. (1998). C–Fos as a Transcription Factor: A Stressful (Re)View From a Functional Map. Neurochem. Int. 33 (4), 287–297. doi: 10.1016/S0197-0186(98)00023-0
Lai F., Royan M. R., Gomes A. S., Espe M., Aksnes A., Norberg B., et al. (2021). The Stress Response in Atlantic Salmon (Salmo Salar L.): Identification and Functional Characterization of the Corticotropin–Releasing Factor (Crf) Paralogs. Gen. Comp. Endocrinol. 313. 113894. doi: 10.1016/j.ygcen.2021.113894
Lankford S. E., Adams T. E., Cech J. J. (2003). Time of Day and Water Temperature Modify the Physiological Stress Response in Green Sturgeon, Acipenser Medirostris. Comp. Biochem. Physiol. – A Mol. Integr. Physiol. 135 (2), 291–302. doi: 10.1016/S1095-6433(03)00075-8
Le Menuet D., Lombès M. (2014). The Neuronal Mineralocorticoid Receptor: From Cell Survival to Neurogenesis. Steroids 91, 11–19. doi: 10.1016/j.steroids.2014.05.018
Lien S., Koop B. F., Sandve S. R., Miller J. R., Kent M. P., Nome T., et al. (2016). The Atlantic Salmon Genome Provides Insights Into Rediploidization. Nature 533 (7602), 200–205. doi: 10.1038/nature17164
Linz R., Puhlmann L. M. C., Apostolakou F., Mantzou E., Papassotiriou I., Chrousos G. P., et al. (2019). Acute Psychosocial Stress Increases Serum BDNF Levels: an Antagonistic Relation to Cortisol But No Group Differences After Mental Training. Neuropsychopharmacology 44 (10), 1797–1804. doi: 10.1016/j.steroids.2014.05.018
Macqueen D. J., Johnston I. A. (2014). A Well–Constrained Estimate for the Timing of the Salmonid Whole Genome Duplication Reveals Major Decoupling From Species Diversification. Proc. R Soc. B Biol. Sci. 281 (1778), 20132881. doi: 10.1098/rspb.2013.2881
Madaro A., Folkedal O., Maiolo S., Alvanopoulou M., Olsen R. E. (2018). Effects of Acclimation Temperature on Cortisol and Oxygen Consumption in Atlantic Salmon (Salmo Salar) Post–Smolt Exposed to Acute Stress. Aquaculture. 497: 331–335. doi: 10.1016/j.aquaculture.2018.07.056
Madaro A., Olsen R. E., Kristiansen T. S., Ebbesson L. O. E., Flik G., Gorissen M. (2016). A Comparative Study of the Response to Repeated Chasing Stress in Atlantic Salmon (Salmo Salar L.) Parr and Post–Smolts. Comp. Biochem. Physiol. –Part A Mol. Integr. Physiol. 192, 7–16. doi: 10.1016/j.cbpa.2015.11.005
Madaro A., Olsen R. E., Kristiansen T. S., Ebbesson L. O. E., Nilsen T. O., Flik G., et al. (2015). Stress in Atlantic Salmon: Response to Unpredictable Chronic Stress. J. Exp. Biol. 218 (16), 2538–2550. doi: 10.1242/jeb.120535
Maier T., Güell M., Serrano L. (2009). Correlation of mRNA and Protein in Complex Biological Samples. FEBS Lett. 583 (24), 3966–3973. doi: 10.1016/j.febslet.2009.10.036
Manuel R., Gorissen M., Zethof J., Ebbesson L. O. E., Van De Vis H., Flik G., et al. (2014a). Unpredictable Chronic Stress Decreases Inhibitory Avoidance Learning in Tuebingen Long–Fin Zebrafish: Stronger Effects in the Resting Phase Than in the Active Phase. J. Exp. Biol. 217 (21), 3919–3928. doi: 10.1242/jeb.109736
Manuel R., Metz J. R., Flik G., Vale W. W., Huising M. O. (2014b). Corticotropin–releasing Factor–Binding Protein (CRF–BP) Inhibits CRF– and Urotensin–I–mediated Activation of CRF Receptor–1 and –2 in Common Carp. Gen. Comp. Endocrinol. 202, 69–75. doi: 10.1016/j.ygcen.2014.04.010
McCormick S. D., Romero L. M. (2017). Conservation Endocrinology. Bioscience. 67 (5), 429–442. doi: 10.1093/biosci/bix026
McMillan P. J., Wilkinson C. W., Greenup L., Raskind M. A., Peskind E. R., Leverenz J. B. (2004). Chronic Cortisol Exposure Promotes the Development of a GABAergic Phenotype in the Primate Hippocampus. J. Neurochem. 91 (4), 843–851. doi: 10.1111/j.1471-4159.2004.02760.x
Mes D., Palstra A. P., Henkel C. V., Mayer I., Vindas M. A. (2020). Swimming Exercise Enhances Brain Plasticity in Fish. R Soc. Open Sci. 7, 191640. doi: 10.1098/rsos.191640
Mes D., von Krogh K., Gorissen M., Mayer I., Vindas M. A. (2018). Neurobiology of Wild and Hatchery–Reared Atlantic Salmon: How Nurture Drives Neuroplasticity. Front. Behav. Neurosci. 12, 1–12. doi: 10.3389/fnbeh.2018.00210
Mifsud K. R., Reul J. M. H. M. (2018). Mineralocorticoid and Glucocorticoid Receptor–Mediated Control of Genomic Responses to Stress in the Brain. Stress. 21 (5), 389–402. doi: 10.1080/10253890.2018.1456526
Mommsen T. P., Vijayan M. M., Moon T. W. (1999). Cortisol in Teleosts: Dynamics, Mechanisms of Action, and Metabolic Regulation. Rev. Fish. Biol. Fisheries. 9: 211–268. doi: 10.1023/A:1008924418720
Nicola G. G., Elvira B., Jonsson B., Ayllón D., Almodóvar A. (2018). Local and Global Climatic Drivers of Atlantic Salmon Decline in Southern Europe. Fish. Res. 198, 78–85. doi: 10.1016/j.fishres.2017.10.012
Nielsen J. L., Ruggerone G. T., Zimmerman C. E. (2013). Adaptive Strategies and Life History Characteristics in a Warming Climate: Salmon in the Arctic? Environ. Biol. Fishes 96 (10–11), 1187–1226. doi: 10.1007/s10641-012-0082-6
Nilsen T. O., Ebbesson L. O. E., Kiilerich P., Björnsson B. T., Madsen S. S., McCormick S. D., et al. (2008). Endocrine Systems in Juvenile Anadromous and Landlocked Atlantic Salmon (Salmo Salar): Seasonal Development and Seawater Acclimation. Gen. Comp. Endocrinol. 155 (3), 762–772. doi: 10.1016/j.ygcen.2007.08.006
Odaka H., Adachi N., Numakawa T. (2017). Impact of Glucocorticoid on Neurogenesis. Neural Regener. Res. 12 (7), 1028–1035. doi: 10.4103/1673-5374.211174
Olsvik P. A., Lie K. K., Jordal A. E. O., Nilsen T. O., Hordvik I. (2005). Evaluation of Potential Reference Genes in Real–Time RT–PCR Studies of Atlantic Salmon. BMC Mol. Biol. 6, 21. doi: 10.1186/1471-2199-6-21
Olsvik P. A., Vikeså V., Lie K. K., Hevrøy E. M. (2013). Transcriptional Responses to Temperature and Low Oxygen Stress in Atlantic Salmon Studied With Next–Generation Sequencing Technology. BMC Genomics. 14: 817. doi: 10.1186/1471-2164-14-817
Pavlidis M., Theodoridi A., Tsalafouta A. (2015). Neuroendocrine Regulation of the Stress Response in Adult Zebrafish, Danio Rerio. Prog. Neuro–Psychopharmacol. Biol. Psychiatry 60, 121–131. doi: 10.1016/j.pnpbp.2015.02.014
Romero L. M., Dickens M. J., Cyr N. E. (2009). The Reactive Scope Model – A New Model Integrating Homeostasis, Allostasis, and Stress. Horm. Behav. 55 (3), 375–389. doi: 10.1016/j.yhbeh.2008.12.009
Rotllant J., Balm P. H. M., Ruane N. M., Pérez–Sánchez J., Wendelaar–Bonga S. E., Tort L. (2000). Pituitary Proopiomelanocortin–Derived Peptides and Hypothalamus–Pituitary–Interrenal Axis Activity in Gilthead Sea Bream (Sparus Aurata) During Prolonged Crowding Stress: Differential Regulation of Adrenocorticotropin Hormone and α–Melanocyte–Stimulating. Gen. Comp. Endocrinol. 119 (2), 152–163. doi: 10.1006/gcen.2000.7508
Sørensen C., Bohlin L. C., Øverli Ø, Nilsson G. E. (2011). Cortisol Reduces Cell Proliferation in the Telencephalon of Rainbow Trout (Oncorhynchus Mykiss). Physiol. Behav. 102 (5), 518–523. doi: 10.1016/j.physbeh.2010.12.023
Sørensen C., Johansen I. B., Øverli Ø. (2013). Neural Plasticity and Stress Coping in Teleost Fishes. Gen. Comp. Endocrinol. 181, 25–34. doi:10.1016/j.ygcen.2012.12.003
Sørensen C., Nilsson G. E., Summers C. H., Øverli Ø. (2012). Social Stress Reduces Forebrain Cell Proliferation in Rainbow Trout (Oncorhynchus Mykiss). Behav. Brain Res. 227 (2): 311–318. doi: 10.1016/j.bbr.2011.01.041
Sadoul B., Alfonso S., Bessa E., Bouchareb A., Blondeau–Bidet E., Clair P., et al. (2018). Enhanced Brain Expression of Genes Related to Cell Proliferation and Neural Differentiation is Associated With Cortisol Receptor Expression in Fishes. Gen. Comp. Endocrinol. 267, 76–81. doi: 10.1016/j.ygcen.2018.06.001
Salvanes A. G. V., Moberg O., Ebbesson L. O. E., Nilsen T. O., Jensen K. H., Braithwaite V. A. (2013). Environmental Enrichment Promotes Neural Plasticity and Cognitive Ability in Fish. Proc. R Soc. B Biol. Sci. 280 (1767), 13. doi: 10.1098/rspb.2013.1331
Samaras A., Santo C. E., Papandroulakis N., Mitrizakis N., Pavlidis M., Höglund E., et al. (2018). Allostatic Load and Stress Physiology in European Seabass (Dicentrarchus Labrax L.) and Gilthead Seabream (Sparus Aurata L.). Front. Endocrinol. (Lausanne). 9. doi: 10.3389/fendo.2018.00451
Sánchez–Vázquez F. J., López–Olmeda J. F., Vera L. M., Migaud H., López–Patiño M. A., Míguez J. M. (2019). Environmental Cycles, Melatonin, and Circadian Control of Stress Response in Fish. Front. Endocrinol. (Lausanne) 10, 1–18. doi: 10.3389/fendo.2019.00279
Schreck C. B. (2010). Stress and Fish Reproduction: The Roles of Allostasis and Hormesis. Gen. Comp. Endocrinol. 165 (3), 549–556. doi: 10.1016/j.ygcen.2009.07.004
Schreck C. B., Tort L. (2016). The Concept of Stress in Fish. Fish. Physiol. 35, 1–34. doi: 10.1016/B978–0–12–802728–8.00001–1
Shors T. J., Anderson M. L., Curlik D. M., Nokia M. S. (2012). Use it or Lose it: How Neurogenesis Keeps the Brain Fit for Learning. Behav. Brain Res. [Internet]. 227 (2), 450–458. doi: 10.1016/j.bbr.2011.04.023
Simms D., Cizdziel P., Chomczynski P. (1993). Trizol: A New Reagent for Optimal Single–Step Isolation of RNA. Focus, 15 (4), 99–102.
Soliman F., Glatt C. E., Bath K. G., Levita L., Jones R. M., Pattwel S. S., et al. (2010). A Genetic Variant BDNF Polymorphism Alters Extinction Learning in Both Mouse and Human. Science 80). 863–866. doi: 10.1126/science.1181886
Stolte E. H., de Mazon A. F., Leon–Koosterziel K. M., Jesiak M., Bury N. R., Sturm A., et al. (2008). Corticosteroid Receptors Involved in Stress Regulation in Common Carp, Cyprinus Carpio. J. Endocrinol. 198, 403–417. doi: 10.1677/JOE-08-0100
Suri D., Vaidya V. A. (2013). Glucocorticoid Regulation of Brain–Derived Neurotrophic Factor: Relevance to Hippocampal Structural and Functional Plasticity. Neuroscience. 239,196–213. doi: 10.1016/j.neuroscience.2012.08.065
Tang P. A., Stefansson S. O., Nilsen T. O., Gharbi N., Lai F., Tronci V., et al. (2022). Exposure to Cold Temperatures Differentially Modulates Neural Plasticity and Stress Responses in Post–Smolt Atlantic Salmon (Salmo Salar). Aquaculture, 560, 738458. doi: 10.1016/j.aquaculture.2022.738458
Toni M., Angiulli E., Miccoli G., Cioni C., Alleva E., Frabetti F., et al. (2019). Environmental Temperature Variation Affects Brain Protein Expression and Cognitive Abilities in Adult Zebrafish (Danio Rerio): A Proteomic and Behavioural Study. J. Proteomics 204. 103396. doi: 10.1016/j.jprot.2019.103396
Van Weert L. T. C. M., Buurstede J. C., Mahfouz A., Braakhuis P. S. M., Polman J. A. E., Sips H. C. M., et al. (2017). NeuroD Factors Discriminate Mineralocorticoid From Glucocorticoid Receptor DNA Binding in the Male Rat Brain. Endocrinology. 158 (5), 1511–1522. doi: 10.1210/en.2016-1422
van Weert L. T. C. M., Buurstede J. C., Sips H. C. M., Mol I. M., Puri T., Damsteegt R., et al. (2019). Mechanistic Insights in Neurod Potentiation of Mineralocorticoid Receptor Signaling. Int. J. Mol. Sci. 20 (7), 1575. doi: 10.3390/ijms20071575
Vargas–Chacoff L., Regish A. M., Weinstock A., McCormick S. D. (2018). Effects of Elevated Temperature on Osmoregulation and Stress Responses in Atlantic Salmon Salmo Salar Smolts in Fresh Water and Seawater. J. Fish. Biol. 93 (3), 550–559. doi: 10.1111/jfb.13683
Vindas M. A., Gorissen M., Höglund E., Flik G., Tronci V., Damsgård B., et al. (2017). How do Individuals Cope With Stress? Behavioural, Physiological and Neuronal Differences Between Proactive and Reactive Coping Styles in Fish. J. Exp. Biol. 220 (8), 1524–1532. doi: 10.1242/jeb.153213
Wendelaar Bonga S. E. (1997). The Stress Response in Fish. Physiological Reviews. 77(3), 591–625. doi: 10.1152/physrev.1997.77.3.591
Yada T., Tort L. (2016). Stress and Disease Resistance: Immune System and Immunoendocrine Interactions. Fish. Physiol. 35, 365–403. doi: 10.1016/B978-0-12-802728-8.00010-2
Keywords: Temperature, Post-smolts, Stress, Pallium, Neural plasticity, Allostasis, Welfare
Citation: Tang PA, Gharbi N, Nilsen TO, Gorissen M, Stefansson SO and Ebbesson LOE (2022) Increased Thermal Challenges Differentially Modulate Neural Plasticity and Stress Responses in Post-Smolt Atlantic Salmon (Salmo salar). Front. Mar. Sci. 9:926136. doi: 10.3389/fmars.2022.926136
Received: 22 April 2022; Accepted: 17 June 2022;
Published: 22 July 2022.
Edited by:
Ayelén Melisa Blanco, University of Vigo, SpainReviewed by:
Anthony K Gamperl, Memorial University of Newfoundland, CanadaIan Bouyoucos, University of Manitoba, Canada
Copyright © 2022 Tang, Gharbi, Nilsen, Gorissen, Stefansson and Ebbesson. This is an open-access article distributed under the terms of the Creative Commons Attribution License (CC BY). The use, distribution or reproduction in other forums is permitted, provided the original author(s) and the copyright owner(s) are credited and that the original publication in this journal is cited, in accordance with accepted academic practice. No use, distribution or reproduction is permitted which does not comply with these terms.
*Correspondence: P. A. Tang, cHRhbkBub3JjZXJlc2VhcmNoLm5v