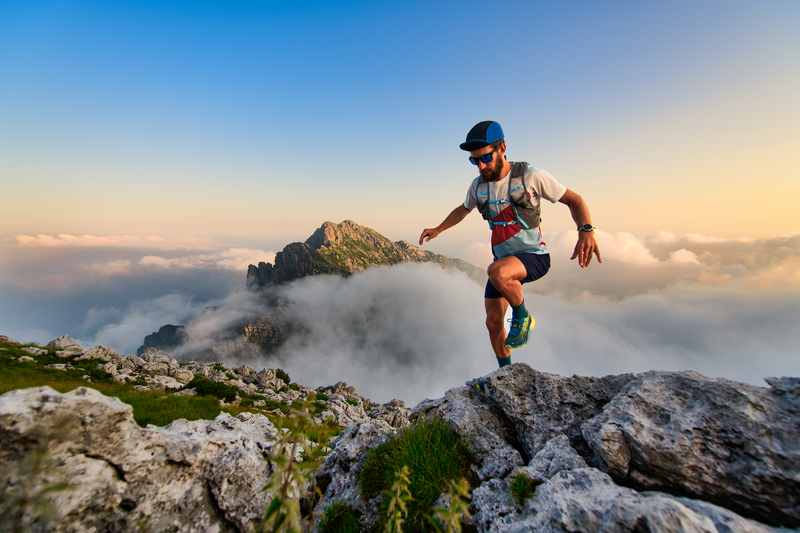
95% of researchers rate our articles as excellent or good
Learn more about the work of our research integrity team to safeguard the quality of each article we publish.
Find out more
ORIGINAL RESEARCH article
Front. Mar. Sci. , 27 July 2022
Sec. Marine Biogeochemistry
Volume 9 - 2022 | https://doi.org/10.3389/fmars.2022.924452
This article is part of the Research Topic Biogeochemistry and Genomics of Silicification and Silicifiers, volume II View all 11 articles
In today’s oceans, diatoms are abundant and diverse primary producers distinguished by their silica shells. Although molecular clocks suggest that diatoms arose as much as 250 million years ago (Ma), the earliest known diatom fossils date from 190 Ma, leading to the suggestion that early diatoms were at best lightly silicified. By the Cretaceous Period, large circular (in cross section) diatoms with highly silicified frustules thrived in surface oceans, only later to be joined by species with elongated and thinner frustules, as well as lower SiO2 content. Decreased Si availability in surface oceans has been proposed as a principal driver of diatom evolution. Here, we investigate this through physiological experiments assessing the functional acclimation response of diatoms to reconstructed paleo-seawater. Four diatom species, differing in size and shape, were acclimated to reconstructed paleoenvironments mimicking Mesozoic/Cenozoic concentrations of nutrients in the presence of different Si regimes. When exposed to 500 µM Si, all populations, save for that of Conticribra weissflogii, became more highly silicified; the higher Si content per cell at 500 µM Si coincided with slower growth in small-sized cells. All species except C. weissflogii also showed lower photosynthetic efficiency as well as greater cell volume in comparison with diatoms acclimated to 205 or 25 µM Si. Average cell stoichiometry correlates with cell shape, but not size; pennates, in particular Phaeodactylum tricornutum, showed an acclimatory response to Si regimes, modulating Si use efficiency (the lower the external Si concentrations, the higher the C and N quotas per Si).
Experimental data suggest that in the densely silicified and bigger C. weissflogii grown at higher Si, diffusion of silicic acid across membranes made a larger contribution to Si uptake, saving energy which could be reallocated into growth. In contrast, for less highly silicified and smaller species, high energy costs of Si homeostasis needed to prevent the overaccumulation of intracellular Si limited growth. While our experimental species reacted individualistically to changing silica availability, with distinct levels of plasticity, selective pressure associated with the temporal decline in Si availability may well have favored elongated shapes. Modern, less silicified species are unable to exploit high Si concentrations.
In the modern ocean, diatoms are abundant and diverse primary producers, distinguished by their silica shells, or frustules. Accounting for some 20% of primary production on Earth, diatoms sustain the global food web while being responsible for 240 Tmol of biogenic silica precipitation annually (Loucaides et al., 2012; Vallina et al., 2014; Malviya et al., 2016; Sutton et al., 2018; Vincent and Bowler, 2020; Tréguer et al., 2021). The oldest known diatom fossils occurred in Late Jurassic (ca. 165 million years old, Ma) amber (Girard et al., 2020), and since that time, diatoms have diversified to play a crucial role in ocean ecology (Armbrust, 2009); indeed, their radiation has molded marine ecosystems through time (Finkel and Kotrc, 2010; Kotrc and Knoll, 2015; Cermeño et al., 2015; Medlin, 2015; Knoll and Follows, 2016; Benoiston et al., 2017), affecting both the carbon and silica cycles (Siever, 1992; Ragueneau et al., 2006; Renaudie, 2016; Conley et al., 2017; Tréguer et al., 2018).
In Precambrian oceans, before the evolution of organisms with biomineralized skeletons, the concentration of dissolved silica (DSi) in seawater must have been much higher than today (Siever, 1992; Conley and Carey, 2015; Conley et al., 2017). The Ediacaran/Cambrian radiations of siliceous sponges and radiolarians established biology as a major component of the silica cycle, demonstrably changing the depositional dynamics of silica in the oceans (Maliva et al., 1989; Kidder and Tomescu, 2016) and probably decreasing the seawater DSi concentration (Conley et al., 2017). New biological influences emerged during the Mesozoic Era, as multiple clades of silica biomineralizing protists spread through the oceans (Kotrc and Knoll, 2015; Marron et al., 2016). Diatoms, in particular, are thought to have further drawn down DSi in surface seawater, leading to the low concentration (<30 µM) observed today (Racki and Cordey, 2000; Conley et al., 2017). Changes in hydrothermal fluxes and continental weathering can also have influenced DSi through time (Frings et al., 2016; Conley et al., 2017), but independent perspectives on orogenesis and seafloor spreading suggest that the Mesozoic-Cenozoic DSi decline was largely mediated by biology.
Within diatoms, the diversification of specialized transporters (silicon transporters, SIT) helped to make them the dominant marine DSi utilizers in Cenozoic oceans (Durkin et al., 2016; Fontorbe et al., 2017). As a consequence, competitors for this resource, principally radiolarians and sponges, show declines in test mass and/or environmental distribution (Harper and Knoll, 1975; Maldonado et al., 1999; Lazarus et al., 2009; Hendry et al., 2018). Diatoms themselves show a change in skeletal morphology through time, with a trend toward smaller, more elongated, and less highly silicified frustules toward the present (Finkel et al., 2005; Armbrust, 2009; Finkel and Kotrc, 2010). It is worth noting that factors other than DSi availability may have influenced the evolution of silicifiers (e.g., Finkel et al., 2005; Hendry et al., 2018) and that changing selectivity of preservation may also influence the observed record (Westacott et al., 2021). That noted, could changes in DSi availability have influenced the observed shifts in the fossil record? We hypothesize that decreasing DSi favored smaller and less silicified diatom cells in marine environments. To test this hypothesis, four morphologically distinct modern diatoms (Chaetoceros muelleri, Conticribra weissflogii, Phaeodactylum tricornutum, Cylindrotheca fusiformis) were acclimated to paleo-reconstructed environments according to Ratti et al. (2011), modified to mimic Mesozoic to modern changes in DSi concentration. As nutrient limitation is known to affect silica deposition and dissolution in diatoms (Takeda, 1998; Hutchins and Bruland, 1998; Boyle, 1998; De La Rocha et al., 2000; Mosseri et al., 2008; Finkel et al., 2010; Bucciarelli et al., 2010; Cohen et al., 2017; Meyerink et al., 2017; Panagiotopoulos et al., 2020), experiments using reconstructed seawater that include interpreted variation in the bioavailability of N, Fe, Zn, and Mo (Ratti et al., 2011; Giordano et al., 2018) better approximate ancient marine environments and their interactions with organisms. Growth, photosynthetic efficiency, organic and inorganic composition, and frustule morphology were assessed.
Two centric diatom species, Chaetoceros muelleri (CCAP 1010/3, https://www.ccap.ac.uk/) and Conticribra weissflogii (Thalassiosira weissflogii in earlier literature; DCG 0320, https://bccm.belspo.be/about-us/bccm-dcg), and two raphid pennate diatoms characterized by thin frustules, Cylindrotheca fusiformis (NEPCC417) and Phaeodactylum tricornutum (DCG 0981), were acclimated for at least 10 generations to three newly designed growth media combining the Mesozoic/Cenozoic concentration of nutrients (as already published by Ratti et al., 2011; Table 1) and the presence of different Si regimes (Table 2) in AMCONA medium (Fanesi et al., 2014) buffered with 10 mM Tris–HCl, pH 8.0.
Table 1 Mesozoic/Cenozoic concentration of nutrients. Ratti et al., 2011.
Table 2 Silicic acid concentrations added to the Mesozoic/Cenozoic medium to mimic the progressive DSi depletion through geologic time.
There are varying estimates for DSi levels in ancient oceans. Siever (1992) proposed that diatom evolution gradually reduced seawater DSi from some 1000 µM to its present level of<30 µM in most surface waters. In contrast, Conley et al. (2017) estimated that DSi concentrations of 500 µM in pre-diatom oceans fell rapidly to near-modern levels as diatoms began their radiation. Most recently, Trower et al. (2021) used Si isotope ratios to argue that DSi levels could have been as low as 150 µM in Paleozoic oceans. Regardless of the starting point, all conclude that diatom radiation reduced DSi concentrations in surface seawater. The DSi values used in our experiments were chosen to explore this range of estimated changes through time.
Acclimated batch cultures were established in 500ml flasks filled with 200 ml of medium and maintained in a culture chamber at 18°C, illuminated with cool white fluorescent lamps at 60 µmol m-2 s-1 and 12:12-h light–dark cycles. All experiments were carried out in the exponential growth phase.
Cell number was measured using a CASY TT Cell Counter (Innovatis AG, Reutlingen, Germany). Aliquots of 100 μl of culture were diluted in 10 ml of an electrolyte solution (CASY TON; Innovatis AG). Cells were pumped into the cell counter through a 150µm capillary at a constant flow, and the number of cells was determined through the enumeration of events measured as change in conductivity. The same instrument was also used to measure the cellular size as the volume of electrolyte solution displaced by the passage of cells through a measuring pore (Palmucci et al., 2011). All determinations were carried out on samples from three distinct cultures. Specific growth rates, μ (1), were derived from daily counts of exponentially growing cells, carried out on a minimum of three distinct cultures for each treatment.
where t is time (d), N0 is the initial cell density (cell· ml-1) at time 0, and Nt is the cell density at the considered time t.
Algae were centrifuged at 1500 g for 5 min. Then, pigments were extracted from the pellet in 2 ml of 100% (v/v) methanol (Ritchie, 2006); the extracts were stored in the dark, at -20°C overnight. The colorless pellet was then separated from the supernatant by centrifugation at 13,000 g for 5 min. The absorbance of the supernatant was evaluated spectrophotometrically (Beckman DU 640 Spectrophotometer, Beckman Coulter) in a range from 750 to 350 nm (scan speed 0.5 nm). The absorbance (Abs) at wavelengths of 664, 630, and 470 nm was used for pigment quantification of chlorophyll a, chlorophyll c1 + c2, and carotenoids, respectively. Methanol absorbance was used as blank, and absorbance at 730 nm was subtracted to all measurements.
Chlorophyll (Chl) concentrations were calculated according to Ritchie, 2006 equations:
Carotenoids’ concentrations were calculated according to Wellburn, 1994 equations:
All results were expressed as pg of pigment per cell (Figure S2).
In vivo variable fluorescence of photosystem II (PSII) chlorophyll a (P680) was analyzed using a Dual Pulse Amplitude Modulation (PAM) 100 fluorimeter (Heinz Walz GmbH, Effeltrich, Germany). Samples of 107 cells were collected by centrifugation at 1500 g for 5 min, resuspended in 2 ml of fresh growth medium, and dark-adapted for 10 min. Subsequently, samples were transferred into a glass cuvette for the PAM analysis under continuous stirring. The measuring light (40 µmol photons m-2 s-1) was turned on to determine the F0 value, the minimum value for chlorophyll fluorescence. Then, a saturation pulse (10,000 µmol photons m-2 s-1, 600 ms) was applied to saturate all centers and allowed to measure the Fm value, the maximum value of fluorescence. The maximum quantum efficiency of PSII, Fv/Fm, was then calculated as follows:
Fv/Fm represents a robust indicator of the maximum quantum yield of PSII photochemistry (Misra et al., 2012). All parameters were obtained using the Dual PAM v1.8 software (Walz GmbH, Effeltrich, Germany).
Cellular C and N contents were determined using an elemental analyzer (ECS 4010, Costech Italy) from 0.1 to 1 mg of dry cells washed twice with an ammonium formate solution isosmotic to the culturing media and dried at 80°C (Giordano et al., 2018). Sulfanilamide (C:N:S = 6:2:1) was used in a standard curve for quantification (Giordano et al., 2018). Data acquisition and analysis were performed with the software EAS Clarity (Costech Analytical Technologies Inc., Milano, Italy; organic composition). All measurements were carried out on three biological replicas.
Similarly prepared samples (0.5–1 mg of dry weight) were analyzed by an elemental analyzer (ECS 4010, Costech Italy) connected to the ID Micro EA isotope ratio mass spectrometer (Compact Science Systems, Lymedale Business Centre, Newcastle-Under-Lyme, United Kingdom) to obtain carbon and nitrogen stable isotope (δ13C and δ15N) ratios. Urea was the isotopic standard reference showing δ13C = −36.6‰ and δ15N = -2.2‰; its replicates were used to normalize isotopic values of algal biomass. Two blank samples (empty aluminum capsules) were analyzed at the start of each analysis to verify that the CO2 and N2 backgrounds were low, and urea standards were also analyzed after every six samples to monitor instrument performance. Data acquisition and analysis were performed with the software EA IsoDelta (Compact Science Systems, Lymedale Business Centre, Newcastle-Under-Lyme, United Kingdom). All the measurements were carried out on three biological replicas.
The absolute abundance of silicon and elements other than C and N was measured using a total reflection X-ray fluorescence spectrometer (S2 PICOFOX, Bruker AXS Microanalysis GmbH, Berlin, Germany) according to Giordano et al. (2018). Sampled diatoms were washed twice with an ammonium formate solution isosmotic to the culturing media and resuspended in 250 µl of dH2O. A solution of 0.1 g l-1 Ga (Sigma-Aldrich, St. Luis, MO, USA) in 5% HNO3 was added as internal standard to a final concentration of 0.5 μl l-1. The suspension was carefully vortexed, and an aliquot of 10 µl was deposed on a plastic sample holder, dried on a heating plate, and measured for 1,000 s. Spectral deconvolution and quantification of elemental abundances were performed by the Spectra 6.1 software (Bruker AXS Microanalysis GmbH, Berlin, Germany).
Diatoms collected during the exponential phase were washed twice with a 0.5M solution of ammonium formate and used to prepare samples for analysis of FTIR spectroscopy. Aliquots of 50 µl of cell suspension were transferred to a silicon window and dried at 80°C (Domenighini and Giordano, 2009). FTIR spectra on whole cells were acquired with a Tensor 27 FTIR spectrometer (Bruker Optics, Ettlingen, Germany). Bands were assigned to cellular pools as described by Giordano et al. (2001), and the relative abundances of lipids, carbohydrates, proteins, and silica were calculated via band integrals of deconvolved spectra, with OPUS 6.5 software (Bruker Optics GmbH, Ettlingen, Germany). Considering that silica absorbance (∼1,075 cm-1) masks some of the typical carbohydrate bands in diatoms, only the integrated value at ∼1,150 cm-1 was used as proxy for carbohydrates (Palmucci et al., 2011). Semiquantification of carbohydrates and lipids was obtained by comparing the total protein content measured by a quantitative method (see below) with the FTIR absorbance ratio between the pool of interest and that of proteins according to Palmucci et al. (2011); the three macromolecular pools expressed in arbitrary units were normalized to the corresponding 500 µM of Si pool.
Protein content was measured according to the Lowry method described by Peterson (1977) on diatoms collected by centrifugation (13,000 g for 5 min) during the exponential phase. A volume of 500 µl 1% sodium dodecyl sulfate (SDS) and 0.1 mol l-1 of NaOH were added to the pellet to facilitate membrane disruption and solubilization of proteins. The tubes were vortexed and then incubated at room temperature for 10 min. A volume of 500 µl of reagent A (25% H2O, 25% SDS 10%, 25% NaOH 0.8M, 25% CTC reagent) was added, and the samples were vortexed and let to sit at room temperature for 10 min. A volume of 250 µl of reagent B (83.3% H2O, 16.7% Folin and Cicalteau’s phenol reagent) was then added, and samples were immediately vortexed vigorously; finally, they were incubated in the dark for 30 min. Afterward, the sample absorbance was measured in a Beckman DU 640 Spectrophotometer (Beckman Coulter) at 750 nm. Protein contents were calculated by interpolating absorbance data in a standard curve constructed with known concentrations of bovine serum albumin (BSA). All the measurements were carried out on three biological replicas, and results were expressed as pg of proteins per cell (Figure S4).
Diatom frustules were obtained through oxidation of the organic material using hydrogen peroxide, H2O2. Salts of the culture medium were washed out from the cells for three times with deionized water, then 30% H2O2 was added to the cell suspension to a final concentration of about 15%. Samples were dried in oven at 60°C for 1 day (less silicified species, P. tricornutum and C. fusiformis) or three days (more silicified species, C. muelleri and C. weissflogii). Finally, the material was washed four times with deionized water to carefully remove H2O2. Drops of cleaned material were then poured on a cellulose acetate and cellulose nitrate mixture filter (MF-Millipore™, mesh size 0,45 µm) fixed on conductive carbon adhesive discs pasted on the stub and left to dry completely at 50°C. The stub was then sputter-coated with a thin layer of gold–palladium in a Balzer Union evaporator and analyzed by SEM (High Resolution ZEISS – SUPRA 40). Images were obtained at different magnifications to make morphometric measurements of frustule details (raphe and fibulae in C. fusiformis, Reimann et al., 1965; setae and punctae in C. muelleri, Reinke, 1984) from at least 10 frustules per each of the three biological replicas.
Significant differences among the means of dependent variables in different paleoenvironments (independent variable) were tested with a one-way analysis of variance (ANOVA), followed by Tukey’s post-hoc test. Macromolecular pools as dependent variables in response to paleoenvironments and according to diatom species (independent variables) were analyzed by two-way ANOVA, followed by Tukey’s post-hoc test. Comparison of treatment pairs (morphological parameters at 500 and 25 µM Si, Table S2) was achieved with a two-tailed t-test. The level of significance was set at 0.05. GraphPad Prism 8.0.2.263 was used to carry out the tests (GraphPad Software, San Diego, CA, USA).
The four diatom populations were acclimated to reconstructed paleoenvironments. Growth of the smaller species C. muelleri and P. tricornutum was deeply affected by Si availability in the medium (Figure S1); specifically, the higher the DSi concentration, the lower the growth rate (Table 3). In fact, C. muelleri cells grew very slowly when DSi was 500 µM. The decrease in growth rate of these species was accompanied by an increase in cell volume (Table 3). Growth rates of the larger species C. weissflogii and C. fusiformis were similar among the three DSi treatments. High concentrations of silicic acid mimicking a pre-diatom regime resulted in a lower maximum PSII Quantum Yield in dark-adapted cells, Fv/Fm, of C. muelleri, P. tricornutum, and C. fusiformis. In contrast, C. weissflogii did not show any change in photosynthetic efficiency (Table 3).
Table 3 Average ± SD of specific growth rate (μ), cell volume, and Fv/Fm in the four diatoms acclimated to different paleoenvironments (n ≥ 3).
When cells were subjected to different Si regimes during growth, all diatoms grown in the highest DSi condition except for C. weissflogii accumulated more Si per volume unit (Figure 1). The largest variation was observed in pennate diatoms, C. fusiformis and P. tricornutum, showing twice the content when the concentration of external silicic acid reached 500 µM as compared to contents in the other conditions. The same was true when the Si content was expressed on a per cell basis (Table S1).
Figure 1 Si content per volume (fg·µm-3) in the four diatoms acclimated to different paleoenvironments. Data are means of three biological replicas. Error bars show SD. Different letters represent significant differences among conditions in the same species (p < 0.05, one-way ANOVA followed by Tukey’s post-hoc test, results in Table S3).
The P cell quota had a similar trend to that of the Si cell quota (Table S1). Only P. tricornutum also showed significantly higher C, N, and Fe contents in the highest DSi condition (Table S1). In general, the C:N:P:S:Si ratio of the cells was inversely related to the concentration of silicic acid in the growth medium: lower amounts of assimilated C, N, P, and S per unit of cell Si with higher DSi availability (Table 4). Furthermore, the P cell quota increased with increasing DSi availability (Table S1). Only P. tricornutum also showed significantly higher C, N, and Fe contents in the highest DSi condition (Table S1), with the Fe : Si ratio remaining approximately constant across the different Si treatments (Table 4).
Table 4 Cell stoichiometry in relation to Si content (pg·cell-1) of the four diatoms acclimated to different paleoenvironments.
Interestingly, our centric and pennate diatoms show divergent trends in carbon isotopic fractionation across treatments (Figure 2). Centric diatoms significantly increased observed fractionation (more negative δ13C) as DSi decreased, just the opposite of observed trends in our pennate species. Also, a strong correlation (Pearson r = -0.99 in P. tricornutum and -0.96 in C. fusiformis, Figure S3) between the δ13C and intracellular Si content was observed in pennate cells, but not in our centric species.
Figure 2 δ13C values in the four diatoms acclimated to different paleoenvironments. Data are means of three biological replicas. Error bars show SD. Different letters represent significant differences among conditions in the same species (p< 0.05, one-way ANOVA followed by Tukey’s post hoc test, results in Table S3).
In cells of C. muelleri, C. fusiformis, and P. tricornutum, proteins were more abundant in response to higher silicic acid concentrations in the growth medium (Figure 3 and Figure S4). In pennate diatoms, the same trend was observed for the carbohydrate pool. Save for C. weissflogii, all diatoms did not significantly change the lipid pool in response to DSi treatment. Regarding macromolecular ratios, the cellular carbohydrate/lipid ratio showed C reallocation in all species (Table 5). In centric diatoms, the protein-to-carbohydrate ratio was not affected while the protein-to-lipid ratio was significantly higher in response to higher DSi availability (Table 5).
Figure 3 Protein, carbohydrates, and lipid pools in the four diatoms acclimated to different paleoenvironments. Data are means of three replicates. Error bars show SD. Different letters represent significant differences among conditions in the same species (p < 0.05, two-way ANOVA followed by Tukey’s post-hoc test, results in Table S3).
Table 5 Macromolecular pool ratios (arbitrary unit) for the four diatoms acclimated to different paleoenvironments.
Frustule structure shows only limited variation as a function of treatment. In C. muelleri, setae were thicker when grown at high DSi, but not significantly (Figures 4C, D; Table S2). Neither did C. weissflogii show any significant difference in frustule morphology as a function of treatment (Figure 4B). By contrast, the pennate species both showed a significant difference between frustules grown at high versus low DSi (Figure 5 and Table S2). Under high DSi, P. tricornutum frustules were significantly wider and raphes significantly thicker (Figures 5A, C, D), while the raphe in C. fusiformis was significantly wider (Figures 5B, E, F and Table S2).
Figure 4 SEM images of frustules from C. muelleri (A) and C. weissflogii (B) acclimated to 500 µM Si (left images) and 25 µM Si (right images). Details of C. muelleri setae at 500 µM Si (C) and 25 µM Si (D) are shown.
Figure 5 SEM images of frustules from P. tricornutum (A) and C. fusiformis (B) acclimated to 500 µM Si (left images) and 25 µM Si (right images). Details of P. tricornutum and C. fusiformis raphe at 500 µM Si ((C, E) respectively) and 25 µM Si ((D, F) respectively) are shown.
Paleontological and geochemical data support the hypothesis that diatom radiation drove a decline in the DSi of surface seawater that influenced the subsequent evolution of both diatoms and other organisms that form skeletons of silica (Finkel et al., 2005; Rabosky and Sorhannus, 2009; Conley et al., 2017; Hendry et al., 2018). At the same time, other factors, including climate change, orogenesis, and predation pressure, may have influenced both observed paleontological patterns and inferred temporal variation in seawater composition (Falkowski et al., 2004; Zachos et al., 2008; Ratti et al., 2013; Lazarus et al., 2014; Conley et al., 2017; Giordano et al., 2018; Petrucciani et al., 2022). The experiments reported here confirm that whatever other factors inform our understanding of Mesozoic–Cenozoic diatom evolution, declining DSi could have had a direct influence on diatom growth, physiology, and morphogenesis.
Perhaps counterintuitively, our experimental species did not grow better at high DSi concentrations; indeed, just the opposite occurred. All the diatoms in our experiments thrived at the low concentrations of N, Fe, Zn, Mo, and Si characteristic of modern oceans (Table 3 and Figure S1). In modern oceans, regional and seasonal Si limitation (i.e., in the Southern Ocean) is known to downregulate Si uptake and silicification in DSi users, including diatoms (Pinkerton et al., 2021). Nevertheless, diatoms maintain nearly maximal division rates (Olsen and Paasche, 1986). Our data underscore that different species show distinct responses to Si availability. In fact, although growth of the two smaller species, C. muelleri and P. tricornutum, was significantly lower at the highest DSi concentration (Table 3), the acclimation of C. muelleri to 500μM DSi concentration was strongly hampered (Figure S1) as the pennate species reached a stationary phase growth (Figure S1). The drastic reduction in photosynthetic efficiency was consistent with growth limitation (Table 3). It has been suggested that a high silicic acid concentration in the external medium can lead to cytotoxic effects (Marron et al., 2016) through auto-polymerization of intracellular soluble Si which then overaccumulates (Milligan et al., 2004).
Why a high external concentration of DSi should result in a high intracellular Si concentration is unclear. It is known that Si influx makes use of i) energy-dependent transporters for Si uptake (SIT), which have acquired the influx function in response to the scarce availability of Si in oceans, and ii) diffusion (Thamatrakoln et al., 2006; Thamatrakoln and Hildebrand, 2008; Hildebrand et al., 2018). On the other hand, Si efflux is an overlooked and poorly understood process in diatom metabolism. These originally utilized ancestral SITs requiring energy have been hypothesized, as do currently utilized SITs (Martin-Jezequel et al., 2000; Milligan et al., 2004; Thamatrakoln and Hildebrand, 2008; Shrestha and Hildebrand, 2015; Hildebrand et al., 2018). Our data (Table 3 and Figure S1) likely indicate that DSi uptake was not turned off by algal cells; direct damage due to intracellular Si polymerization and/or energy-dependent mechanisms to avoid it (i.e., counterbalancing Si influx with Si efflux) might affect growth as observed for C. muelleri and P. tricornutum. The effect was observed in less silicified and smaller species characterized by a higher S/V ratio and thus incurring a higher cost to maintain Si homeostasis than bigger cells (Table 3, Figure S1). It is particularly intriguing that in P. tricornutum, Fe : Si was the only ratio not modulated by Si availability in the external medium (Table 4). Nevertheless, when DSi was 500 μM, both Si and Fe cell quotas were higher (roughly 17 and 14 times, respectively) than those in modern Si-treated algae (Table S1). This finding may add nuance to the observation that Fe deficiency leads to enhanced Si content (De La Rocha et al., 2000), suggesting that Fe is strongly involved in Si homeostasis. Further studies are needed to clarify how Fe interacts with Si influx/efflux/biomineralization.
The densely silicified and larger (hence, lower S/V ratio, Table 3 and Figure 1) cells of C. weissflogii had a different fate when grown under high DSi conditions (Table 3 and Figure S1). In the presence of higher environmental DSi concentrations, diffusion of silicic acid across membranes is supposed to make a bigger contribution to the Si uptake than it does in lower DSi growth conditions. This could explain why energy is saved and reallocated into C. weissflogii growth, assuming that DSi influx through SITs is less relevant and the efflux is not needed due to the high Si requirement for thicker C. weissflogii frustules. Only in this species did the photosynthetic efficiency remain unchanged by varying DSi levels (Table 3).
Cell composition in terms of macromolecular pools varied among treatments and in a shape-dependent manner. At high [DSi], our pre- to early diatom Si treatment cells of pennate diatoms were most costly (in terms of energy investment associated with the observed organic composition; Gerotto et al., 2020) and palatable (Palmucci et al., 2011; Ratti et al., 2013), preferentially allocating fixed C into carbohydrates and proteins (Figure 3, Table 5) as compared to the same species acclimated to modern Si treatment.
Data suggest that over geologic time, DSi availability has affected the overall C allocation pattern of diatoms (in particular pennates) and, therefore, predation pressure via its influence on palatability for predators. Thus, DSi decline has favored the evolution of energy-saving, less palatable cells, which are more competitive in intraspecific and interspecific interactions (Petrucciani et al., 2022).
Interestingly, Si availability also affected C isotopic fractionation: when DSi concentration was higher, pennate diatoms increased C fractionation (more negative δ13C) (Figure 2). This could be explained by a change in availability of intracellular inorganic carbon among growth regimes: in fact, a lower contribution of inorganic C mobilized from HCO3- to the fixed C in pennates grown in high DSi conditions results in increased discrimination against 13CO2 (Korb et al., 1996; Keller and Morel, 1999; Vuorio et al., 2006). This may well reflect to a downregulation in pennates of CCM (Riebesell et al., 2000) in favor of other mandatory energy-dependent mechanisms such as Si deposition and Si efflux under high DSi conditions (Giordano et al., 2005; Giordano et al., 2017). Therefore, lower photosynthetic efficiencies were reported (Table 3). In any event, the correlation between silicon content and C fractionation observed in these species underscores the deep interaction between the two elements as well as between biomineralization and C fixation (Figure S3). This is somewhat unexpected, since in centric diatoms the uncoupling of silicon compared with carbon and nitrogen metabolisms was reported (Claquin et al., 2002; Suroy et al., 2015). The observed variations in isotopic fractionation also add further nuance to physiological and paleoenvironmental interpretations of organic carbon isotopes in Mesozoic and Cenozoic marine sediments (Hayes et al., 1999).
Greater availability of DSi was associated, as well, with a greater accumulation of Si in both cells (Figure 1, Table S1) and frustules (Figures 4 and 5), as also evident in the literature compilation of Finkel et al (2010). The high Si content was not simply a function of greater cell volume since Si content expressed on a per-volume basis was also higher in the 500µM Si treatment (Figure 1). In addition, a higher Si quota per cell in diatoms is known to be induced by a slower growth rate (Brzezinski et al., 1990; Friedrichs et al., 2013). Both factors (high DSi and low growth rate) were present when C. muelleri and P. tricornutum showed the highest Si content (Table 3 and Figure S1). The change in Si quota was striking in pennate diatoms (Figure 1 and Table S1), as shown by raphe thickening in P. tricornutum frustules (Figures 5A, B). The entire cellular stoichiometry was overturned (Table 4). C. fusiformis showed a similar trend, suggesting that the pennate diatoms acclimated to changing growth conditions by modulating Si use efficiency: the higher the available DSi in the environment, the lower the efficiency in its use (Table 4). In contrast, centric diatoms had a homeostatic behavior regarding their elemental stoichiometry: such strategy did not allow growth in C. muelleri and limited maximal cell density in C. weissflogii (Table 4 and Figure S1).
In conclusion, even with our small sample size, it becomes clear that diatom responses to changing silica bioavailability are commonly individualistic; that is, there may be few parameters for which diatoms universally respond in the same way. The centric and pennate species in our experiments commonly responded in different ways or to different degrees, suggesting that centrics and pennates may show broad and consistent differences in their physiological responses to changing DSi levels.
While we cannot discount climate change and changing preservational selectivity as influences on the observed fossil record of diatoms, the experimental approach used here acclimating algal species to reconstructed paleoenvironments bolsters the case that decreasing [DSi] had an important influence on diatom biomineralization through time. In particular, the observed physiological responses of pennate species may have helped to drive their differential diversification, governing reduced size and silica usage in diatoms as a group.
The original contributions presented in the study are included in the article/supplementary material. Further inquiries can be directed to the corresponding author.
AP carried out all the experiments and analyzed the data. AHK designed the paleo-reconstructed media and provided a geological perspective to the manuscript. AN conceived and designed the project. AP, AHK, and AN wrote the paper. All authors contributed to the article and approved the submitted version.
Research for AP PhD project was partially funded by Cariverona Foundation, Italy.
Special gratitude goes to the late Mario Giordano who encouraged experiments to test geologically inspired hypotheses for observed patterns.
The authors declare that the research was conducted in the absence of any commercial or financial relationships that could be construed as a potential conflict of interest.
All claims expressed in this article are solely those of the authors and do not necessarily represent those of their affiliated organizations, or those of the publisher, the editors and the reviewers. Any product that may be evaluated in this article, or claim that may be made by its manufacturer, is not guaranteed or endorsed by the publisher.
The Supplementary Material for this article can be found online at: https://www.frontiersin.org/articles/10.3389/fmars.2022.924452/full#supplementary-material.
Armbrust E. V. (2009). The life of diatoms in the world’s oceans. Nature 459, 185–192. doi: 10.1038/nature08057
Benoiston A. S., Ibarbalz F. M., Bittner L., Guidi L., Jahn O., Dutkiewicz S., et al. (2017). The evolution of diatoms and their biogeochemical functions. Phil. Trans. R. Soc B 372, 20160397. doi: 10.1098/rstb.2016.0397
Brzezinski M., Olson R., Chisholm S. (1990). Silicon availability and cell-cycle progression in marine diatoms. Mar. Ecol. Prog. Ser. 67, 83–96. doi: 10.3354/meps067083
Bucciarelli E., Pondaven P., Sarthou G. (2010). Effects of an iron-light co-limitation on the elemental composition (Si, c, n) of the marine diatoms Thalassiosira oceanica and Ditylum brightwellii. Biogeosciences 7 (2), 657–669. doi: 10.5194/bg-7-657-2010
Cermeño P., Falkowski P. G., Romero O. E., Schaller M. F., Vallina S. M. (2015). Continental erosion and the Cenozoic rise of marine diatoms. Proc. Natl. Acad. Sci. U.S.A. 112, 4239–4244. doi: 10.1073/pnas.1412883112
Claquin P., Martin-Jézéquel V., Kromkamp J. C., Veldhuis M. J. W., Kraay G. W. (2002). Uncoupling of silicon compared with carbon and nitrogen metabolisms and the role of the cell cycle in continuous cultures of thalassiosira pseudonana (bacillariophyceae) under light, nitrogen, and phosphorus control. J. Phycology 38, 922–930. doi: 10.1046/j.1529-8817.2002.t01-1-01220.x
Cohen N. R., Ellis K. A., Lampe R. H., McNair H., Twining B. S., Maldonado M. T., et al. (2017). Diatom transcriptional and physiological responses to changes in iron bioavailability across ocean provinces. Front. Mar. Sci. 4. doi: 10.3389/fmars.2017.00360
Conley D. J., Carey J. C. (2015). Silica cycling over geologic time. Nat. Geosci 8, 431–432. doi: 10.1038/ngeo2454
Conley D. J., Frings P. J., Fontorbe G., Clymans W., Stadmark J., Hendry K. R., et al. (2017). Biosilicification drives a decline of dissolved si in the oceans through geologic time. Front. Mar. Sci. 4. doi: 10.3389/fmars.2017.00397
De La Rocha C., Hutchins D., Brzezinski M., Zhang Y. (2000). Effects of iron and zinc deficiency on elemental composition and silica production by diatoms. Mar. Ecol. Prog. Ser. 195, 71–79. doi: 10.3354/meps195071
Domenighini A., Giordano M. (2009). Fourier Transform infrared spectroscopy of microalgae as a novel tool for biodiversity studies, species identification, and the assessment of water quality 1. J. phycology 45 (2), 522–531. doi: 10.1111/j.1529-8817.2009.00662.x
Durkin C. A., Koester J. A., Bender S. J., Armbrust E. V. (2016). The evolution of silicon transporters in diatoms. J. phycology 52, 716–731. doi: 10.1111/jpy.12441
Falkowski P. G., Katz M. E., Knoll A. H., Quigg A., Raven J. A., Schofield O., et al. (2004). The evolution of modern eukaryotic phytoplankton. Science 305, 354–360. doi: 10.1126/science.1095964
Fanesi A., Raven J. A., Giordano M. (2014). Growth rate affects the responses in the green alga Tretaselmis suecica to the external perturbation. Plant Cell Environ. 37 (2), 512–519. doi: 10.1111/pce.12176
Finkel Z. V., Katz M. E., Wright J. D., Schofield O. M. E., Falkowski P. G. (2005). Climatically driven macroevolutionary patterns in the size of marine diatoms over the Cenozoic. Proc. Natl. Acad. Sci. 102, 8927–8932. doi: 10.1073/pnas.0409907102
Finkel Z. V., Kotrc B. (2010). Silica use through time: macroevolutionary change in the morphology of the diatom fustule. Geomicrobiology J. 27, 596–608. doi: 10.1080/01490451003702941
Finkel Z. V., Matheson K. A., Regan K. S., Irwin A. J. (2010). Genotypic and phenotypic variation in diatom silicification under paleo-oceanographic conditions: Diatom silicification. Geobiology 8, 433–445. doi: 10.1111/j.1472-4669.2010.00250.x
Fontorbe G., Frings P. J., de la Rocha C. L., Hendry K. R., Carstensen J., Conley D. J. (2017). Enrichment of dissolved silica in the deep equatorial pacific during the Eocene-oligocene: Equatorial pacific DSi enrichment. Paleoceanography 32, 848–863. doi: 10.1002/2017PA003090
Friedrichs L., Hörnig M., Schulze L., Bertram A., Jansen S., Hamm C. (2013). Size and biomechanic properties of diatom frustules influence food uptake by copepods. Mar. Ecol. Prog. Ser. 481, 41–51. doi: 10.3354/meps10227
Frings P. J., Clymans W., Fontorbe G., de la Rocha C. L., Conley D. J. (2016). The continental Si cycle and its impact on the ocean Si isotope budget. Chem. Geology 425, 12–36. doi: 10.1016/j.chemgeo.2016.01.020
Gerotto C., Norici A., Giordano M. (2020). Toward enhanced fixation of CO2 in aquatic biomass: Focus on microalgae. Front. Energy Res. 8. doi: 10.3389/fenrg.2020.00213
Giordano M., Beardall J., Raven J. A. (2005). CO2 concentrating mechanisms in algae: mechanisms, environmental modulation, and evolution. Annu. Rev. Plant Biol. 56, 99–131. doi: 10.1146/annurev.arplant.56.032604.144052
Giordano M., Kansiz M., Heraud P., Beardall J., Wood B., McNaughton D. (2001). Fourier Transform infrared spectroscopy as a novel tool to investigate changes in intracellular macromolecular pools in the marine microalga chaetoceros muellerii (bacillariophyceae). J. Phycology 37, 271–279. doi: 10.1046/j.1529-8817.2001.037002271.x
Giordano M., Norici A., Beardall J. (2017). Impact of inhibitors of amino acid, protein, and RNA synthesis on c allocation in the diatom Chaetoceros muellerii: a FTIR approach. ALGAE 32, 161–170. doi: 10.4490/algae.2017.32.6.6
Giordano M., Olivieri C., Ratti S., Norici A., Raven J. A., Knoll A. H. (2018). A tale of two eras: Phytoplankton composition influenced by oceanic paleochemistry. Geobiology 16, 498–506. doi: 10.1111/gbi.12290
Girard V., Saint Martin S., Buffetaut E., Saint Martin J. P., Néraudeau D., Peyrot D., et al. (2020). Thai Amber: insights into early diatom history? Bull. la Société Géologique France 2020 191 (1), 23. doi: 10.1051/bsgf/2020028
Harper H. E., Knoll A. H. (1975). Silica, diatoms, and Cenozoic radiolarian evolution. Geol 3, 175. doi: 10.1130/0091-7613(1975)3<175:SDACRE>2.0.CO;2
Hayes J. M., Strauss H., Kaufman A. J. (1999). The abundance of 13C in marine organic matter and isotopic fractionation in the global biogeochemical cycle of carbon during the past 800 ma. Chem. Geology 161, 103–125. doi: 10.2475/ajs.289.4.436
Hendry K. R., Marron A. O., Vincent F., Conley D. J., Gehlen M., Ibarbalz F. M., et al. (2018). Competition between silicifiers and non-silicifiers in the past and present ocean and its evolutionary impacts. Front. Mar. Sci. 5. doi: 10.3389/fmars.2018.00022
Hildebrand M., Lerch S. J. L., Shrestha R. P. (2018). Understanding diatom cell wall silicification-moving forward. Front. Mar. Sci. 5. doi: 10.3389/fmars.2018.00125
Hutchins D. A., Bruland K. W. (1998). Iron-limited diatom growth and Si:N uptake ratios in a coastal upwelling regime. Nature 393, 561–564. doi: 10.1038/31203
Keller K., Morel F. (1999). A model of carbon isotopic fractionation and active carbon uptake in phytoplankton. Mar. Ecol. Prog. Ser. 182, 295–298. doi: 10.3354/meps182295
Kidder D. L., Tomescu I. (2016). Biogenic chert and the Ordovician silica cycle. Palaeogeography Palaeoclimatology Palaeoecol. 458, 29–38. doi: 10.1016/j.palaeo.2015.10.013
Knoll A. H., Follows M. J. (2016). A bottom-up perspective on ecosystem changes in mesozoic oceans. Proc. R. Soc B. 283, 20161755. doi: 10.1098/rspb.2016.1755
Korb R., Raven J., Johnston A., Leftley J. (1996). Effects of cell size and specific growth rate on stable carbon isotope discrimination by two species of marine diatom. Mar. Ecol. Prog. Ser. 143, 283–288. doi: 10.3354/meps143283
Kotrc B., Knoll A. H. (2015). A morphospace of planktonic marine diatoms. i. two views of disparity through time. Paleobiology 41, 45–67. doi: 10.1017/pab.2014.4
Lazarus D., Barron J., Renaudie J., Diver P., Türke A. (2014). Cenozoic Planktonic marine diatom diversity and correlation to climate change. PloS One 9, e84857. doi: 10.1371/journal.pone.0084857
Lazarus D. B., Kotrc B., Wulf G., Schmidt D. N. (2009). Radiolarians decreased silicification as an evolutionary response to reduced Cenozoic ocean silica availability. Proc. Natl. Acad. Sci. 106, 9333–9338. doi: 10.1073/pnas.0812979106
Loucaides S., Van Cappellen P., Roubeix V., Moriceau B., Ragueneau O. (2012). Controls on the recycling and preservation of biogenic silica from biomineralization to burial. Silicon 4, 7–22. doi: 10.1007/s12633-011-9092-9
Maldonado M., Carmona M. C., Uriz M. J., Cruzado A. (1999). Decline in mesozoic reef-building sponges explained by silicon limitation. Nature 401, 785–788. doi: 10.1038/44560
Maliva R. G., Knoll A. H., Siever R. (1989). Secular change in chert distribution: a reflection of evolving biological participation in the silica cycle. PALAIOS 4, 519. doi: 10.2307/3514743
Malviya S., Scalco E., Audic S., Vincent F., Veluchamy A., Poulain J., et al. (2016). Insights into global diatom distribution and diversity in the world’s ocean. Proc. Natl. Acad. Sci. U.S.A. 113, E1516–E1525. doi: 10.1073/pnas.1509523113
Marron A. O., Ratcliffe S., Wheeler G. L., Goldstein R. E., King N., Not F., et al. (2016). The evolution of silicon transport in eukaryotes. Mol. Biol. Evol. 33, 3226–3248. doi: 10.1093/molbev/msw209
Martin-Jezequel V., Hildebrand M., Brzezinski M. A. (2000). Silicon metabolism in diatoms: implications for growth. J. Phycol 36, 821–840. doi: 10.1046/j.1529-8817.2000.00019.x
Medlin L. K. (2015). A timescale for diatom evolution based on four molecular markers: reassessment of ghost lineages and major steps defining diatom evolution. Vie Milieu 65 (4), 821–840.
Meyerink S., Ellwood M. J., Maher W. A., Strzepek R. (2017). Iron availability influences silicon isotope fractionation in two southern ocean diatoms (Proboscia inermis and Eucampia antarctica) and a coastal diatom (Thalassiosira pseudonana). Front. Mar. Sci. 4, 217. doi: 10.3389/fmars.2017.00217
Milligan A. J., Varela D. E., Brzezinski M. A., Morel F. M. M. (2004). Dynamics of silicon metabolism and silicon isotopic discrimination in a marine diatomas a function of pCO 2. Limnol. Oceanogr. 49, 322–329. doi: 10.4319/lo.2004.49.2.0322
Misra A. N., Misra M., Singh R. (2012). Chlorophyll fluorescence in plant biology. Biophysics. 49, 322–329. doi: 10.4319/lo.2004.49.2.0322
Monod J. (1949). The growth of bacterial cultures. Annu. Rev. Microbiol. 3, 371–394. doi: 10.1146/annurev.mi.03.100149.002103
Mosseri J., Quéguiner B., Armand L., Cornet-Barthaux V. (2008). Impact of iron on silicon utilization by diatoms in the southern ocean: A case study of Si/N cycle decoupling in a naturally iron-enriched area. Deep Sea Res. Part II: Topical Stud. Oceanography 55, 801–819. doi: 10.1016/j.dsr2.2007.12.003
Olsen S., Paasche E. (1986). Variable kinetics of silicon-limited growth in Thalassiosira pseudonana (Bacillariophyceae) in response to changed chemical composition of the growth medium. Br. Phycological J. 21, 183–190. doi: 10.1080/00071618600650211
Palmucci M., Ratti S., Giordano M. (2011). Ecological and evolutionary implications of carbon allocation in marine phytoplankton as a function of nitrogen availability: a fourier transform infrared spectroscopy approach1: c allocation as a function of n availability. J. Phycology 47, 313–323. doi: 10.1111/j.1529-8817.2011.00963.x
Panagiotopoulos C., Goutx M., Suroy M., Moriceau B. (2020). Phosphorus limitation affects the molecular composition of Thalassiosira weissflogii leading to increased biogenic silica dissolution and high degradation rates of cellular carbohydrates. Organic Geochemistry 148, 104068. doi: 10.1016/j.orggeochem.2020.104068
Peterson G. L. (1977). A simplification of the protein assay method of lowry et al. which is more generally applicable. Analytical Biochem. 83 2, 346–356. doi: 10.1016/0003-2697(77)90043-4
Petrucciani A., Chaerle P., Norici A. (2022). Diatoms versus copepods: could frustule traits have a role in avoiding predation? Front. Mar. Sci. 8. doi: 10.3389/fmars.2021.804960
Pinkerton M. H., Boyd P. W., Deppeler S., Hayward A., Höfer J., Moreau S. (2021). Evidence for the impact of climate change on primary producers in the southern ocean. Front. Ecol. Evol. 9, 592027. doi: 10.3389/fevo.2021.592027
Rabosky D. L., Sorhannus U. (2009). Diversity dynamics of marine planktonic diatoms across the Cenozoic. Nature 457, 183–186. doi: 10.1038/nature07435
Racki G., Cordey F. (2000). Radiolarian palaeoecology and radiolarites: is the present the key to the past? Earth-Science Rev. 52, 83–120. doi: 10.1016/S0012-8252(00)00024-6
Ragueneau O., Schultes S., Bidle K., Claquin P., Moriceau B. (2006). Si And c interactions in the world ocean: Importance of ecological processes and implications for the role of diatoms in the biological pump: Si and c interactions in the ocean. Global Biogeochem. Cycles 20, n/a–n/a. doi: 10.1029/2006GB002688
Ratti S., Knoll A. H., Giordano M. (2011). Did sulfate availability facilitate the evolutionary expansion of chlorophyll a+c phytoplankton in the oceans? sulfate and evolution of chlorophyll a+c phytoplankton. Geobiology 9, 301–312. doi: 10.1111/j.1472-4669.2011.00284.x
Ratti S., Knoll A. H., Giordano M. (2013). Grazers and phytoplankton growth in the oceans: an experimental and evolutionary perspective. PloS One 8, e77349. doi: 10.1371/journal.pone.0077349
Reimann B. E. F., Lewin J. C., Volcani B. E. (1965). Studies on the biochemistry and fine structure of silica shell formation in diatoms: I. the structure of the cell wall of Cylindrotheca fusiformis. J. Cell Biol. 24, 39–55. doi: 10.1083/jcb.24.1.39
Reinke D. C. (1984). Ultrastructure of Chaetoceros muelleri (bacillariophyceae): auxospore, resting spore and vegetative cell morphology. J. Phycology 20, 153–155. doi: 10.1111/j.0022-3646.1984.00153.x
Renaudie J. (2016). Quantifying the Cenozoic marine diatom deposition history: links to the c and Si cycles. Biogeosciences 13, 6003–6014. doi: 10.5194/bg-13-6003
Riebesell U., Burkhardt S., Dauelsberg A., Kroon B. (2000). Carbon isotope fractionation by a marine diatom: dependence on the growth-rate-limiting resource. Mar. Ecol. Prog. Ser. 193, 295–303. doi: 10.3354/meps193295
Ritchie R. J. (2006). Consistent sets of spectrophotometric chlorophyll equation for acetone, methanol, and ethanol solvents. Photosynthesis Res. 89, 27–41. doi: 10.1007/s11100-006-9065-9
Shrestha R. P., Hildebrand M. (2015). Evidence for a regulatory role of diatom silicon transporters in cellular silicon responses. Eukaryot Cell 14, 29–40. doi: 10.1128/EC.00209-14
Siever R. (1992). The silica cycle in the precambrian. Geochimica Cosmochimica Acta 56, 3265–3272. doi: 10.1016/0016-7037(92)90303-Z
Suroy M., Panagiotopoulos C., Boutorh J., Goutx M., Moriceau B. (2015). Degradation of diatom carbohydrates: A case study with n- and Si-stressed thalassiosira weissflogii. J. Exp. Mar. Biol. Ecol. 470, 1–11. doi: 10.1016/j.jembe.2015.04.018
Sutton J. N., André L., Cardinal D., Conley D. J., de Souza G. F., Dean J., et al. (2018). A review of the stable isotope bio-geochemistry of the global silicon cycle and its associated trace elements. Front. Earth Sci. 5, 112. doi: 10.3389/feart.2017.00112
Takeda S. (1998). Influence of iron availability on nutrient consumption ratio of diatoms in oceanic waters. Nature 393, 774–777. doi: 10.1038/31674
Thamatrakoln K., Alverson A. J., Hildebrand M. (2006). Comparative sequence analysis of diatom silicon transporters: toward a mechanistic model of silicon transport. J. Phycology 42, 822–834. doi: 10.1111/j.1529-8817.2006.00233.x
Thamatrakoln K., Hildebrand M. (2008). Silicon uptake in diatoms revisited: a model for saturable and nonsaturable uptake kinetics and the role of silicon transporters. Plant Physiol. 146, 1397–1407. doi: 10.1104/pp.107.107094
Tréguer P., Bowler C., Moriceau B., Dutkiewicz S., Gehlen M., Aumont O., et al. (2018). Influence of diatom diversity on the ocean biological carbon pump. Nat. Geosci 11, 27–37. doi: 10.1038/s41561-017-0028-x
Tréguer P. J., Sutton J. N., Brzezinski M., Charette M. A., Devries T., Dutkiewicz S., et al. (2021). Reviews and syntheses: The biogeochemical cycle of silicon in the modern ocean. Biogeosciences 18, 1269–1289. doi: 10.5194/bg-18-1269-2021
Trower E. J., Strauss J. V., Sperling E. A., Fischer W. W. (2021). Isotopic analyses of Ordovician–Silurian siliceous skeletons indicate silica-depleted Palaeozoic oceans. Geobiology 19, 460–472. doi: 10.1111/gbi.12449
Vallina S. M., Follows M. J., Dutkiewicz S., Montoya J. M., Cermeno P., Loreau M. (2014). Global relationship between phytoplankton diversity and productivity in the ocean. Nat. Commun. 5, 4299. doi: 10.1038/ncomms5299
Vincent F., Bowler C. (2020). Diatoms are selective segregators in global ocean planktonic communities. mSystems 5 (1), e00444–19. doi: 10.1128/mSystems.00444-19
Vuorio K., Meili M., Sarvala J. (2006). Taxon-specific variation in the stable isotopic signatures (δ13C and δ15N) of lake phytoplankton. Freshw. Biol. 51, 807–822. doi: 10.1111/j.1365-2427.2006.01529.x
Wellburn A. R. (1994). The spectral determination of chlorophylls a and b, as well as total carotenoids, using various solvents with spectrophotometers of different resolution. J. Plant Physiol. 1443, 307–313. doi: 10.1016/S0176-1617(11)81192-2
Westacott S., Planavsky N. J., Zhao M. Y., Hull P. M. (2021). Revisiting the sedimentary record of the rise of diatoms. PNAS 118 (27), e2103517118. doi: 10.1073/pnas.2103517118
Keywords: Si decline, diatom, paleo-reconstructed environments, algal physiology, selective pressure
Citation: Petrucciani A, Knoll AH and Norici A (2022) Si decline and diatom evolution: Insights from physiological experiments. Front. Mar. Sci. 9:924452. doi: 10.3389/fmars.2022.924452
Received: 20 April 2022; Accepted: 01 July 2022;
Published: 27 July 2022.
Edited by:
Brivaela Moriceau, UMR6539 Laboratoire des Sciences de L’environnement Marin (LEMAR), FranceReviewed by:
Anne Willem Omta, Massachusetts Institute of Technology, United StatesCopyright © 2022 Petrucciani, Knoll and Norici. This is an open-access article distributed under the terms of the Creative Commons Attribution License (CC BY). The use, distribution or reproduction in other forums is permitted, provided the original author(s) and the copyright owner(s) are credited and that the original publication in this journal is cited, in accordance with accepted academic practice. No use, distribution or reproduction is permitted which does not comply with these terms.
*Correspondence: Alessandra Norici, YS5ub3JpY2lAdW5pdnBtLml0
Disclaimer: All claims expressed in this article are solely those of the authors and do not necessarily represent those of their affiliated organizations, or those of the publisher, the editors and the reviewers. Any product that may be evaluated in this article or claim that may be made by its manufacturer is not guaranteed or endorsed by the publisher.
Research integrity at Frontiers
Learn more about the work of our research integrity team to safeguard the quality of each article we publish.