- School of Aquatic and Fishery Sciences, University of Washington, Seattle, WA, United States
Marine pathogens present serious challenges to aquaculture, fisheries productivity, and marine conservation requiring novel solutions to identify, control, and mitigate their effects. Several ecological habitats, such as mangroves and wetlands can recycle waste and serve as aquatic filtration systems. While nutrient cycling and other ecosystem services of these habitats have been well-studied, their potential to remove pathogens and mechanisms of filtration remain largely unstudied. Here, we review how mangroves, shellfish beds, seagrasses, and constructed wetlands can reduce pathogen pressure in coastal ecosystems. Mangroves may inhibit bacterial growth through phytochemicals in their leaves and remove viruses through desalination in their roots. Some bivalves remove pathogens by excreting pathogens through their pseudofeces and others concentrate pathogens within their tissues. Seagrasses slow flow rates, increase sedimentation rates and may reduce pathogens through allelopathy. Constructed wetlands decrease pathogens through a combination of mechanical, biological, and chemical filtration mechanisms. Protecting and restoring coastal ecosystems is key to maintaining pathogen filtration capacity, benefiting conservation efforts of threatened host populations, and mitigating large disease outbreaks.
Introduction
Diseases, ubiquitous in the marine environment, are vital for healthy ecosystems but can be damaging when they impact fisheries, aquaculture, and ecosystem engineers (Burge and Hershberger, 2020). Pathogens can influence the structure, function, and stability of food webs (Selakovic et al., 2014) and they are critical to ecosystem dynamics as they play an important role in controlling host population densities and nutrient cycling (Lafferty et al., 2008; Carlson et al., 2020). At the Channel Islands National Park in California, sea urchin pathogens drove a community shift from desolate urchin-barrens toward biodiverse kelp forest assemblages (Behrens and Lafferty, 2004). Pathogens can have a positive role in mitigating and controlling algal blooms (Bigalke et al., 2019). Algicidal bacteria limited the growth of three phytoplankton species, allowing other species to increase in abundance and thus have the potential to shift phytoplankton community structure (Bigalke et al., 2019). In New Zealand, nematode, trematode, and acanthocephalan parasites increased biodiversity on mudflats (Thompson et al., 2005).
High pathogen abundance can cause host population declines with implications for community structure, ecosystem dynamics, and fisheries and aquaculture production (Groner et al., 2016). This can be especially damaging in keystone and economically important species such as the long-spined sea urchin (Diadema antillarum) that declined by almost 98% in the 1980’s in the Caribbean (Behrens and Lafferty, 2004). The decline of this ecologically important grazer decreased herbivory of algae, contributing to coral reef deterioration in the region (Onufryk et al., 2018). Pathogens have the potential to severely compromise fisheries. Epizootic shell disease, caused by bacterial dysbiosis (Groner et al., 2016) (Figure 1A) substantially contributed to the decline of one of the most profitable fisheries in the United States (US): the Southern New England lobster stock (Castro et al., 2012; Hoenig et al., 2017). The disease impact was two-fold in that it reduced the abundance of lobsters, with ovigerous females having somewhat higher mortality rates, and rendered the surviving lobsters unappealing for market sale (Lafferty et al., 2015). Another fishery impacted by disease is the black abalone (Haliotis cracherodii) along the West coast of the US (Raimondi et al., 2002). This population suffered major die-offs since the 1980’s, accounting for total population losses of over 90%. These die-offs were caused by abalone withering syndrome, a chronic condition caused by a Rickettsiales-like organism (WS-RLO) that shrinks the mollusk’s foot, preventing the animal from attaching to substrates (Raimondi et al., 2002) (Figure 1A). While efforts were made to rebuild the population, disease remains a hurdle to restoration and conservation efforts. Similarly, sea star wasting disease, hypothesized to be caused by a densovirus (SSaDV), caused a continental collapse of the sunflower star (Pycnopodia helianthoides), a keystone species, and impacted the abundance of 20 asteroid species along the West coast of the US (Lamb et al., 2017; Harvell et al., 2019). Warming ocean temperatures put additional physiological stress on sea stars, decimating wild populations and leading to unprecedented sea star mortality from Alaska to California (Figure 1A) (Harvell et al., 2019). Warming events, especially El Niño can trigger disease outbreaks such as the novel ulcerative skin disease that reduced ring-tailed damselfish (Stegastees beebei) and king angelfish (Holacanthus passer) populations by 78% and 86%, respectively in the Galapagos (Lamb et al., 2018).
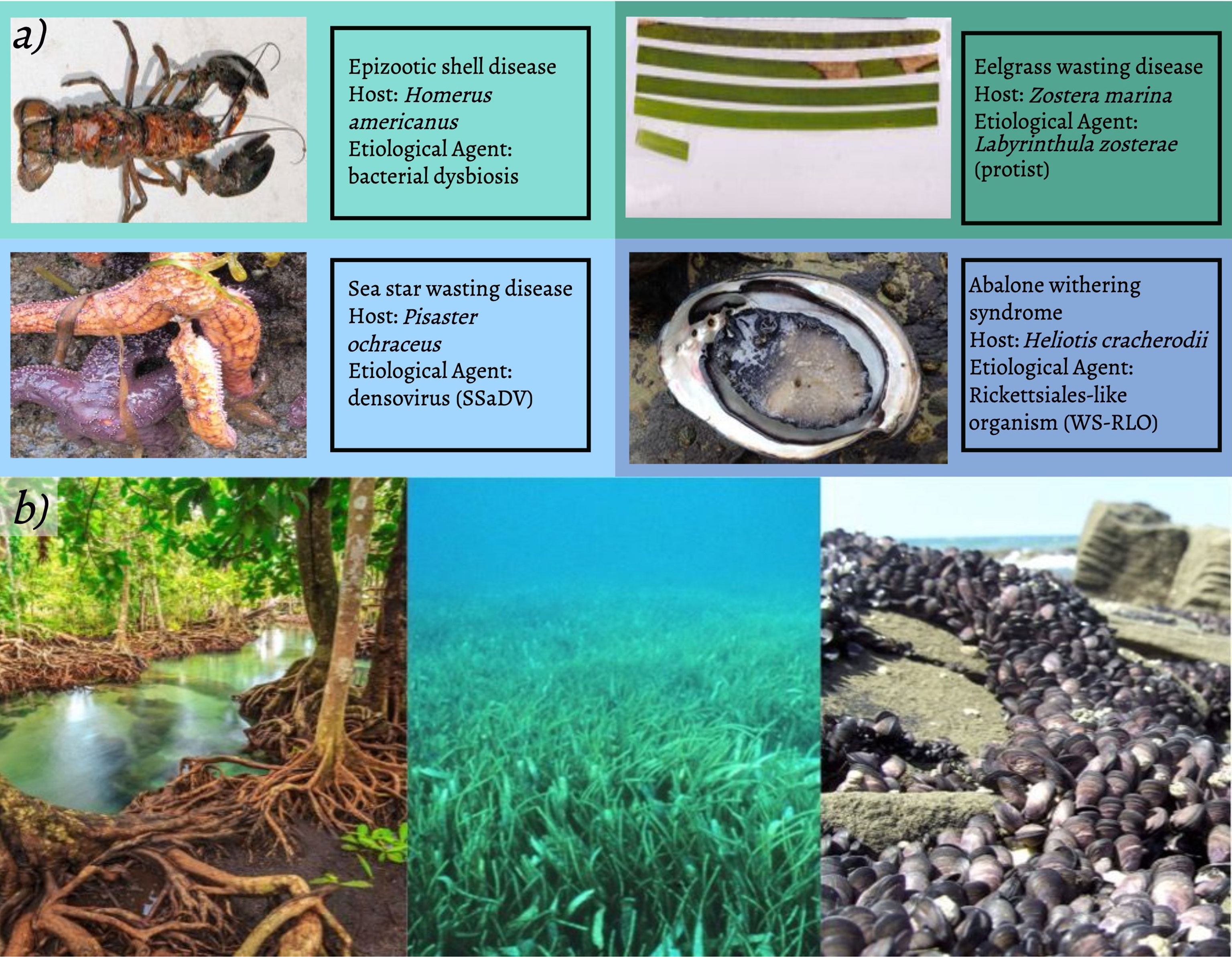
Figure 1 (A) Marine diseases known to severely impact host species including keystone species and ecosystem engineers. (Pictures courtesy of C. Harvell, NOAA Fisheries, AccessScience, O Graham and C Klohmann). Marine ecosystems with pathogen filtration capacity (B). Mangroves (left), seagrasses (middle), and shellfish beds (right). Pictures courtesy of Vecteezy, NOAA Photo Library, and Wikimedia commons.
Large die-offs of seagrass species over the last hundred years have also been attributed to pathogens. Eelgrass wasting disease, likely caused by the etiological agent Labyrinthula zosterae, created a large-scale blight in the Eelgrass (Zostera marina) population along the Atlantic coast of the US in the 1930s that has still not fully recovered (Figure 1A) (Rasmussen, 1977). L. zosterae is causing outbreaks of disease in eelgrass from Europe to western North America (Bockelmann et al., 2013; Groner et al., 2021).
Pathogen pressure is an issue for human health. Humans can experience infections, gastrointestinal and respiratory diseases, and other health effects when exposed to viral and bacterial pathogens in the ocean (Griffin et al., 2003; Graciaa et al., 2018). We must look to innovative, sustainable, and creative solutions to manage ocean pathogens to limit their spread and effects. Filtration is an important ecosystem service to consider for removing and containing pathogens in the coastal environment. Filtration is defined as the reduction of pathogens in the water column by a variety of means including the reduction of water speed (i.e., flow rate), particle interception, biochemical transformation of nutrients and contaminants, absorption of water and nutrients, and sedimentation (Kuehn and Mueller, 2000; “Constructed Treatment Wetlands”, 2004; Brauman et al., 2007; Wu et al., 2016).
Natural filtration in coastal environments can occur by vegetation (i.e., via seagrass, salt marshes and mangroves) and shellfish beds (Burge et al., 2016; J. Lamb et al., 2017). However, these natural filtration services may be in danger as coastal ecosystems are threatened by habitat loss (Halpern et al., 2008), climate change (Harley et al., 2006), and disease (Rasmussen, 1977). The planet has lost about 50% of its wetlands since 1900 (Davidson, 2014) and seagrasses, salt marshes and mangroves are all declining globally (Halpern et al., 2008). Recent estimates indicate that mangroves and seagrass beds are declining annually by 0.13% and 7%, respectively (Evans et al., 2018; Goldberg et al., 2020). Bivalve populations tend to be more intensely managed since they are important source of protein; however, it was estimated that globally, 85% of oysters reefs have been lost (Beck et al., 2011). Since the 1970’s mussel (Mytilus edulis) populations declined by more than 60% in the gulf of Maine, USA (Sorte et al., 2017) and mussel bed communities declined by 58.9% along the West coast of the United States (Smith et al., 2006). Bivalve populations have declined in Europe but are increasing in Asia (Wijsman et al., 2019).
New research seeks to bolster natural filtration systems to decrease pathogen pressure and improve human health. Investing in natural filtration systems is an affordable and low-maintenance option when compared to wastewater treatment facilities, which cost between $250,000-$10,000,000 USD for installation in the United States (“How Much Does an Industrial Water Treatment System Cost?”, 2017). Restoring and protecting wetlands can sustainably reduce pathogen pressure in coastal ecosystems by supplementing existing wastewater treatment strategies. In terrestrial systems, forest cover and proximity to forested areas can decrease childhood diarrheal infections (Herrera et al., 2017), suggesting that conserving forests and riparian vegetation has the potential to limit disease. In Germany, riverbank filtration (water passed through the bank of the river) has been used for over 100 years to purify drinking water, providing approximately 16% of all German drinking water (Kuehn and Mueller, 2000).
Despite recent efforts to better understand pathogen pressure in the ocean (Tracy et al., 2019; Harvell and Lamb, 2020) we still have an incomplete understanding of how these pathogens are controlled in marine systems, and how they will impact marine and human health. So far studies suggest that disease is increasing in certain systems like corals reefs but may be declining in fishes and elasmobranchs (Tracy et al., 2019). It is also unclear whether the systems severely impacted by disease outbreak are lacking a filtration system or have one that has been impaired in some way. Here, we examine the role that coastal marine species, namely (1) mangroves, (2) shellfish beds (mussels and oysters), (3) seagrasses, and (4) constructed wetlands, play in pathogen filtration and the mechanisms that decrease pathogen loads.
Mangroves
Mangrove forests are coastal ecosystems widespread in the intertidal zone in tropical and subtropical areas, covering 136,000 km2 globally (Figure 1B) (Spalding and Maricé, 2021). These forests provide key ecosystem services such as carbon sequestration, nursery habitat for fish, and coastal protection. Mangroves reduce excess nutrients in coastal waters, including nitrogen and phosphorous (Lin and Dushoff, 2004), and are resilient plants capable of exuding and expelling salts, enabling them to thrive in highly saline environments (Spalding and Maricé, 2021). Tropical shrimp farms utilize mangroves’ natural biofiltration properties to efficiently remove excess nutrients (Buhmann and Papenbrock, 2013). They are also the largest store of carbon in the coastal zone and can reduce ocean acidification (Lin and Dushoff, 2004; Sippo et al., 2016). Mangrove trees buffer against acidic water using their roots by metabolizing organic matter from their carbon-rich and oxygen-poor soils and releasing alkaline water in return (Sippo et al., 2016). Mangroves are so efficient at this process that waters within mangrove forests can have a pH as high as 8.1, whereas the seawater measured outside of the forest usually has a pH of 7.3 (Sippo et al., 2016).
Human and fish pathogens are filtered by mangroves. The phytochemicals within mangrove leaves and fungi found in their root sediments may assist in killing bacterial pathogens (Sahoo et al., 2012; Thatoi et al., 2013). Organic solvent extracts of leaves from five mangrove species in the Philippines inhibited growth of pathogenic fish bacteria (Choudhury et al., 2005). These extracts could be used in aquaculture facilities to reduce disease in fish (Choudhury et al., 2005). Mangrove leaf extracts inhibit the growth of human pathogens such as Staphylococcus and Salmonella (Sahoo et al., 2012). Another study inoculated compost with fungi isolated from mangrove sediments and found that plants grown in the fungal mangrove soil had higher disease defenses than plants without the fungi (Ameen and Ali, 2021). Mangroves have also been referred to as “natural wastewater wetlands” (Wu et al., 2008) and considered as secondary wastewater treatment systems in China due to their filtration capabilities (Palacios et al., 2021)Mangrove roots, may reduce viral pathogens as they are efficient desalinators, removing up to 90% of sodium ions from seawater (Kim et al., 2016). The outermost root layer has a negative surface potential, attracting the positively charged sodium ions and repelling the negatively charged chlorine ions. These root potentials trap sodium ions in the first (outer) root layer, enabling fresh water to enter the inner roots (Kim et al., 2016) (Figure 2C). Mangrove desalination may be an important mechanism for pathogen filtration, as salts promote viral absorption due to the their negative charge (Lukasik et al., 2000). This charge is hypothesized to increase hydrophobic interactions between viruses and the roots, which may lead to viral absorption by the mangroves (Lukasik et al., 2000).
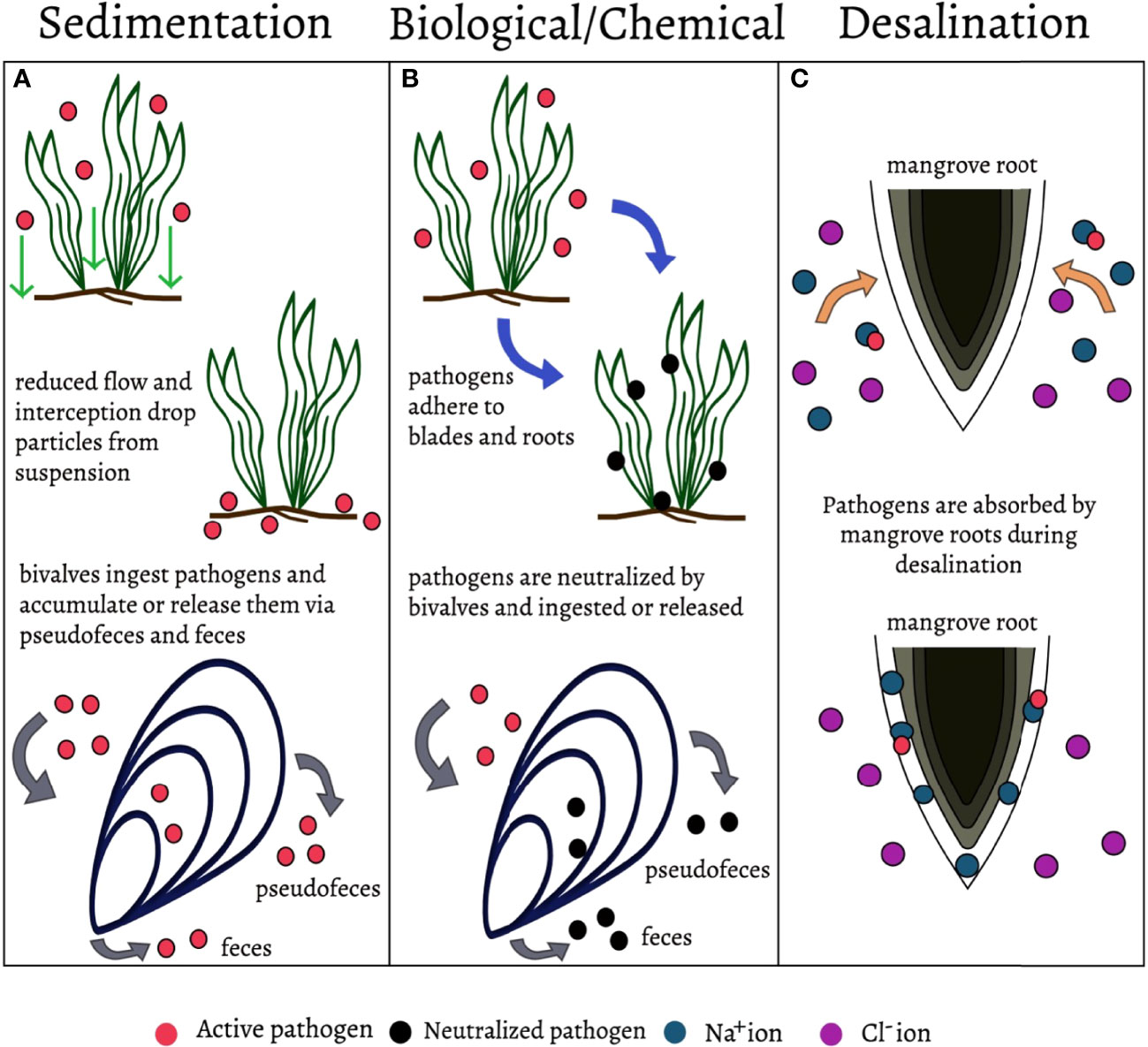
Figure 2 Mechanisms involved in natural pathogen filtration. (A) Sedimentation - The removal of pathogens from suspension. This mechanism does not neutralize pathogens but removes them from the environment, it is utilized in seagrasses, bivalves, and constructed wetlands. (B) Biological/Chemical filtration - the removal of pathogens through adhesion, consumption, and inhibition (allelopathy). Neutralizes pathogens and/or removes them from the environment. Utilized in seagrasses, bivalves, constructed wetlands, and mangroves. (C) Desalination- The removal of sodium ions from salt water. Salts may promote viral absorption due to their negative charge, potentially removing viruses from the seawater. This mechanism is utilized by mangrove roots.
For centuries, mangrove extracts were used to treat a variety of human health issues, and microbial compounds found in mangrove leaves and roots can kill antibiotic-resistant bacteria (Abeysinghe, 2010; Saad et al., 2011; Durai and Radhakrishnan, 2016; Alizadeh Behbahani et al., 2018; Audah, 2020). These services could make mangrove forests very efficient pathogen filtration systems; however, more research is needed to better understand how we can best utilize mangroves to remove pathogens from coastal waters.
Shellfish Beds
Bivalves (Phylum Mollusca) are favorable pathogen filtration specialists due to their high filtration capacity, ubiquity in many ecosystems, tolerance for poor water quality, and abundance. An individual filter-feeding bivalve can filter 10-100 gallons of water a day using their gills (Burge et al., 2016). Bivalves feed by capturing particles from the water column, primarily phyto- and zooplankton, but they can also consume bacteria, viruses, and other organic matter in the process. Bivalves are selective feeders and particle capture depends on size, density and surface chemical compounds (Burge et al., 2016). Some pathogens may be killed within bivalve gills and gut tissue during ingestion and digestion, but most are likely expelled as feces or pseudofeces (Figure 2). These feces and pseudofeces remove pathogens through sedimentation, sinking out of the water column along with attached bacteria and viruses (Burge et al., 2016). For example, eelgrass plants had fewer lesions from wasting disease (Labyrinthula zosterae) when cocultured with oysters (Groner et al., 2018). Bivalves may also inhibit microbial growth through the production of peptides and polypeptides (Zannella et al., 2017). Additionally, mussels are used at aquaculture facilities to reduce bacterial and eukaryotic pathogen abundance in fish farms (Voudanta et al., 2016).
While bivalves may remove pathogens due to their filtration efficiency, they could also store them in their tissues. Some studies found concentrated pathogens within filter-feeder gill tissues, implicating bivalves as reservoirs for pathogens, and in some cases, amplification (Ben-Horin et al., 2015; Harvell and Lamb, 2020). Accumulation occurs when pathogens do not degrade and instead build up inside the bivalve and can lead to transmission to species that consume the filter feeder (Burge et al., 2016). Specifically, clams and mussels can accumulate and transmit Vibrio haemolyticus to humans (Harvell and Lamb, 2020). Mussels off the coast of California have tested positive for Toxoplasma gondii, the causative agent of toxoplasmosis, the same strain known to infect sea otters in the region (Miller et al., 2008). This potential transmission renders bivalves a less sustainable option for natural filtration, as shellfish beds present an infection risk to their predators. However, recent research has shown that oyster aquaculture may reduce pathogens for wild oyster populations as long as aquaculture stocks are harvested before they can spread disease (Ben-Horin et al., 2018).
Bivalves grow in very dense clusters, potentially increasing their filtration ability (Figure 1B). Mussels (Mytilus trossulus) can reach densities of 35 individuals per 100 cm2 in the intertidal zone and up to 90 individuals per 100 cm2 at aquaculture facilities (Kirk et al., 2007). Given the large distribution of bivalves, their filtration efficiency, and high densities, shellfish beds have the potential to reduce pathogen loads in coastal waters (Ben-Horin et al., 2015). Bivalves could also be used to monitor pathogens and have been used to monitor pollutants. In Poznan, Poland, clams are used as bioindicators to assess water quality: In the presence of even small concentrations of pollutants, the clams will close their shells as a defense mechanism to prevent ingesting harmful or lethal items. Sensors glued to clams are triggered when the bivalves close, which initiates a shutoff of the water supply. This biological sensor system provides a remarkable means of monitoring environmental pollutants at very little cost (Micu, 2020). The National Oceanic and Atmospheric Administration (NOAA) used bivalves as environmental indicators by establishing oysters in the Chesapeake Bay, Maryland to monitor environmental contamination. Scientists tested oyster tissues for pharmaceutical products, pesticides, and other contaminants, detecting 98 different contaminants using this ecosystem-based monitoring approach (Apeti et al., 2018). Complementary monitoring methods can be developed with bivalves to filter, monitor and detect pathogens in the natural environment. One example is an oyster fishery in Myanmar that detected pathogenic bacterial in bivalve tissues, illustrating the impacts of agricultural runoff on coastal ecosystems (Littman et al., 2020).
Seagrass Beds
Seagrass beds are another ecosystem hypothesized to remove pathogens and are one of the most important coastal ecosystems, providing $1.9 trillion USD in nutrient cycling services annually (Waycott et al., 2009) (Figure 1B). Additionally, seagrasses act as a “nutrient pump” by absorbing nutrients from the soil and releasing these nutrients through their leaves (Fourqurean, 2002). Seagrasses are estimated to cover 600,000 km2 of the coastal floor from the Arctic circle to the tropics, and some beds are large enough to be seen from space, earning them the name “forests of the sea” (Cullen-Unsworth and Unsworth, 2013).
While seagrasses are well studied, their ability to filter pathogens from the water column was only recently identified. In the tropics, researchers found that seagrass beds are associated with reduced abundances of human, fish, and invertebrate pathogens (Lamb et al., 2017). This reduction of pathogens not only improves human health but also benefits corals that have fewer diseases in seagrass beds (Lamb et al., 2017). To date, little is known about the underlying mechanisms involved in the reduction of these pathogens. However, it is likely that a combination of sedimentation, a decrease in water flow rate, and mechanical and chemical filtration contribute to seagrasses’ reduction of pathogens (S. Wu et al., 2016) (Figures 2A, B). Ocean flow rates are reduced by up to 40% within seagrass meadows, which may lead to pathogen particles dropping out of suspension (Lamb et al., 2017). In the South China Sea researchers found that one seagrass species, Enhalus acroides, trapped pathogens within its beds thus removing bacteria from the water (Deng et al., 2021). Phytochemicals within the seagrass plants may also kill pathogens, but this mechanism is still unclear (Babuselvam et al., 2017). Thalassia hemprichii, likely inhibited pathogens using antimicrobial chemical compounds such as phenol, flavonoid, and tannins (Deng et al., 2021).Eelgrass (Zostera marina), a temperate seagrass that provides $87,000 of ecosystem services per hectare annually (Babuselvam et al., 2017), has been shown to exclude harmful dinoflagellates (Jacobs-Palmer et al., 2020). Researchers discovered significantly lower dinoflagellate abundances inside and around the Z. marina bed, such that Z. marina can form a ‘halo’ of dinoflagellate exclusion, up to 16 meters beyond the extent of the meadow (Jacobs-Palmer et al., 2020). Other studies found reduced algal growth due to seagrass phenolic compounds (DellaGreca et al., 2000) and antilarval and antibacterial properties from ethanol extract from a seagrass species in the South China Sea (Qi et al., 2008). Allelopathic activity in seagrasses, the defensive release of chemicals, and biological interactions of the micro and macro epibionts may also play a role in pathogen filtration services in this important ecosystem (Jacobs-Palmer et al., 2020). The outbreaks of the unicellular protist L. zosterae (Sullivan et al., 2018) in eelgrass beds (M. Bockelmann et al., 2013; Groner et al., 2021) suggest that seagrass pathogens may also accumulate within the beds and that biological filtration may not only apply to pathogens that are non-infectious to seagrass. It is important to note that the decline of seagrass beds worldwide is attributed to both pathogens and anthropogenic factors such as ocean warming and eutrophication. More research is needed to fully understand the vulnerability and potential of these filtration mechanisms (Jacobs-Palmer et al., 2020) (Figure 2).
Pathogen Reduction Using Constructed Wetlands
Constructed wetlands are defined as treatment systems that improve water quality through natural processes involving wetland vegetation, soils, and associated microbial assemblages (“Constructed Treatment Wetlands”, 2004). In the 1950s, constructed wetlands were developed to expand on the services that naturally-occurring wetlands provided, such as improving water quality from stormwater runoff, greywater, and wastewater (Wu et al., 2016). Constructed wetlands can vary in their design but are meant to remove heavy metals, nitrogen, phosphorous, and chemical pollutants from runoff and remove harmful pathogens (Wu et al., 2016).
Constructed wetlands are utilized in a wide variety of industries to filter wastes, such as in textiles, paper milling, agriculture, and mining (Buhmann and Papenbrock, 2013). Recently, constructed wetlands were integrated into the aquaculture industry and have successfully been used to remove nutrients from catfish and rainbow trout aquaculture facilities (Buhmann and Papenbrock, 2013). Vegetation in the constructed wetland removes excess nutrients and also reduce water flow, allowing solids to be taken up by their root systems (Buhmann and Papenbrock, 2013). Constructed wetlands are excellent effluent removal options for land-locked aquaculture facilities because they can be implemented in both fresh and saline environments (Buhmann and Papenbrock, 2013). Additionally, constructed wetlands decrease human pathogens from wastewater without the use of chlorine, which is a more sustainable and less damaging process than traditional chemical practices (Wu et al., 2016). Some constructed wetlands reduce water flow, allowing viruses in suspension to be killed by UV radiation (Wu et al., 2016).Pathogens are removed from constructed wetlands by multiple mechanisms. The reduction of nutrients and pathogens depends on wetland type, size, and design and a combination of physical, chemical, and biological mechanisms (Wu et al., 2016). Some physical factors include mechanical filtration, sedimentation, and absorption of organic matter as well as CW design. Sedimentation, the removal of particles from suspension, is most effective against bacteria and coliforms such as fecal streptococcus due to these pathogens’ larger size and faster settling rate (Wu et al., 2016). Mechanical filtration (i.e., passing the water through a filter), included in some constructed wetlands, can effectively remove pathogenic microorganisms, particularly protozoans (Wu et al., 2016). One study in a constructed wetland (Redder et al., 2010) noted a 100-fold reduction in protozoan pathogens when using mechanical filtration. Mechanical filtration is also effective at removing fecal indicator bacteria such as Enterococcus and E. coli (Wu et al., 2016). Predation activity of nematodes, rotifers, protozoa, bacterivorous bacteria, and phages is also an important factor affecting the bacterial removal in CWs (Wu et al., 2016). Artificial and vegetative oxygenation increase dissolved oxygen in CWs and are correlated with pathogen die-offs, especially bacteria (Wu et al., 2016).The need for sustainable and affordable wastewater treatment systems, such as CWs, will only continue to grow along with the global population and wastewater discharge (Wu et al., 2016). CWs are an excellent example of a sustainable investment that will lower the abundance and risk of pathogens. These systems may also provide some insight into the mechanisms associated with pathogen removal in natural ecosystems.
Discussion
Marine diseases are challenging to manage because pathogen transmission is impacted by water chemistry, currents, and ecological dynamics. We need creative solutions to reduce pathogen pressure to limit catastrophic host population die-offs and other negative downstream impacts. Natural filtration systems are an appealing solution to reduce pathogen loads and supplement wastewater treatment due to (1) their cost compared to artificial filtration systems and/or water treatment plants and (2) because pathogen reduction is not contingent upon pathogen isolation. These benefits make natural filtration systems an excellent investment to reduce novel, zoonotic diseases in marine environments with important consequences for human health (Sutton-Grier and Sandifer, 2019).
Natural pathogen filtration has several inherent limitations. Natural filtration only has the potential to reduce infectious disease when transmission is a rate-limiting step for the epidemiology. Some marine pathogens are so ubiquitous that exposure and transmission are almost guaranteed. In other cases, such as epizootic shell disease in lobsters, many of the bacteria associated with this disease are found on the shell of healthy lobsters, but their role switches from commensal to pathogenic. Natural filtration may be unable to prevent or mitigate the effects of such pathogens on an ecologically significant scale.
In mangroves and seagrasses, filtration mechanisms are not fully understood; likewise, in shellfish beds, it is not clear which pathogens are removed and which pathogens concentrate within bivalve tissues. Similarly, the extent of pathogen removal is still being explored in constructed wetlands. Important aspects of each system need to be explored to take full advantage of their filtration properties, including optimal densities in filtering bivalve populations, the role of microbiomes and epiphytes on seagrasses and mangroves, allelopathic interactions, and climate change on filtration capability. Additionally, it is unclear what the impact of filtration is on the host organism and if it can affect its productivity, physiological performance and/or quality. Future work is needed to examine the interactions between pathogen and host in ecosystems with filtration potential and to answer the following questions: Can natural filters fail due to pathogen overload? How can we test and monitor filtration performance? How often do we need to replace natural filtration systems to maintain pathogen filtration efficiency? What are the impacts of extracellular and intracellular pathogens in filtration performance? These are important questions as we consider the use of natural filtration systems to reduce pathogens in the coastal zone.
Coastal wetland ecosystems create habitats that facilitate high biodiversity, which may lead to lower pathogen pressure (Johnson et al., 2013; Duffy et al., 2015; Rahman et al., 2021). Despite the critical roles these ecosystems play, they are increasingly threatened by climate change, pollution, and anthropogenic activities. It is essential to protect them to maintain the services they provide. Conserving these natural areas may decrease pathogen transmission between wildlife and humans, leading to fewer zoonotic events (Keesing and Ostfeld, 2021). We require further understanding of the mechanisms involved in pathogen filtration, its relation to other ecosystem services, and the role that other ecological factors play in pathogen reduction to implement these systems at their full potential.
Author Contributions
All authors listed have made a substantial, direct, and intellectual contribution to the work and approved it for publication.
Funding
This work was supported by the National Science Foundation Graduate Research Fellowship Program awarded to Corinne Klohmann and the Alfred Sloan Research Fellowship and National Science Foundation-CAREER awarded to Dr. Padilla-Gamiño.
Conflict of Interest
The authors declare that the research was conducted in the absence of any commercial or financial relationships that could be construed as a potential conflict of interest.
Publisher’s Note
All claims expressed in this article are solely those of the authors and do not necessarily represent those of their affiliated organizations, or those of the publisher, the editors and the reviewers. Any product that may be evaluated in this article, or claim that may be made by its manufacturer, is not guaranteed or endorsed by the publisher.
Acknowledgments
We would like to thank Dr. Drew Harvell, Dr. Jennifer Ruesink, Dr. Chelsea Wood, and Dr. Maya Groner for expert feedback on the manuscript. We would also like to thank the members of the Padilla-Gamiño laboratory, Núria Viladrich, Miranda Roethler, and Callum Backstrom for their review of this manuscript.
References
Abeysinghe P. D. (2010). Antibacterial Activity of Some Medicinal Mangroves Against Antibiotic Resistant Pathogenic Bacteria. Indian J. Pharm. Sci. 72 (2), 167–172. doi: 10.4103/0250-474X.65019
Alizadeh Behbahani B., Farideh Tabatabaei Y., Fakhri S., Hamid N., Alireza V., Ali A. (2018). Phytochemical Analysis and Antibacterial Activities Extracts of Mangrove Leaf Against the Growth of Some Pathogenic Bacteria. Microb. Pathog. 114, 225–232. doi: 10.1016/j.micpath.2017.12.004
Ameen F, Ali A. A.-H. (2021). Compost Inoculated With Fungi From a Mangrove Habitat Improved the Growth and Disease Defense of Vegetable Plants. Sustainability 13 (1), 1245. doi: 10.3390/su13010124
Apeti D., Wirth E., Leight A. K., Mason A., Pisarski E. (2018). An Assessment of Contaminants of Emerging Concern in Chesapeake Bay, MD and Charleston Harbor, SC (NOAA Technical Memorandum NOS NCCOS 240: Silver Spring, MD), 104. Available at: https://coastalscience.noaa.gov/project/mussel-watch-program-assessment-chesapeake-bay-charleston-harbor/. doi: 10.25923/p4nc-7m71
Audah K. A. (2020). Antibacterial Screening of Mangrove Extract Library Showed Potential Activity Against Escherichia Coli and Staphylococcus Aureus. Journal of Tropical Life Science.(10),105−111. doi:10.11594/jtls.10.02.03
(2017). How Much Does an Industrial Water Treatment System Cost? ( Samco Tech (blog). Available at: https://www.samcotech.com/how-much-does-an-industrial-water-treatment-system-cost/.
Micu A. (2020). In Poznan, Poland, Eight Clams Get to Decide If People in the City Get Water or Not ( ZME Science (blog). Available at: https://www.zmescience.com/science/poznan-mussel-water-plants-892524/.
EPA(2004). Constructed Treatment Wetlands ( United States Environmental Protection Agency, EPA). Available at: https://www.epa.gov/wetlands/constructed-wetlands.
Babuselvam M., Panneerselvam A., Kanimozhi K., Kavitha G. (2017) Antibacterial Potential of Actinomycetes From Seagrass Against Human and Aquaculture Pathogens. Available at: https://www.semanticscholar.org/paper/Antibacterial-potential-of-actinomycetes-from-human-Babuselvam-Panneerselvam/e2bb6e6ac37c81b256547d86e50f527a1291e06b.
Beck M. W., Brumbaugh R. D., Airoldi L., Carranza A., Coen L. D., Crawford C., et al. (2011). Oyster Reefs at Risk and Recommendations for Conservation, Restoration, and Management. BioScience 61 (2), 107–116. doi: 10.1525/bio.2011.61.2.5
Behrens M., Lafferty K. (2004). Effects of Marine Reserves and Urchin Disease on Southern Californian Rocky Reef Communities. Mar. Ecology-Progress Ser. - Mar. ECOL-PROGR Ser. 279, 129–139. doi: 10.3354/meps279129
Ben-Horin T., Bidegain G., Huey L., Narvaez D. A., Bushek D. (2015). Parasite Transmission Through Suspension Feeding. J. Invertebr. Pathol. 131, 155–176. doi: 10.1016/j.jip.2015.07.006
Ben-Horin T., Burge C. A., Bushek D., Groner M. L., Proestou D. A., Huey L. I., et al. (2018). Intensive Oyster Aquaculture Can Reduce Disease Impacts on Sympatric Wild Oysters. Aquacult. Environ. Interact. 10, 557–567. doi: 10.3354/aei00290
Bigalke A., Meyer N., Alkistis Papanikolopoulou L., Helen Wiltshire K., Pohnert G. (2019). The Algicidal Bacterium Kordia Algicida Shapes a Natural Plankton Community. Appl. Environ. Microbiol. 85 (7), e02779–e02718. doi: 10.1128/AEM.02779-18
Bockelmann A.-C., Tams V., Ploog J., Schubert P. R., Reusch T. B. H. (2013). Quantitative PCR Reveals Strong Spatial and Temporal Variation of the Wasting Disease Pathogen, Labyrinthula Zosterae in Northern European Eelgrass (Zostera Marina) Beds. PloS One 8 (5), e62169. doi: 10.1371/journal.pone.0062169
Brauman K. A., Daily G. C., Duarte T. K., Mooney H. A. (2007). The Nature and Value of Ecosystem Services: An Overview Highlighting Hydrologic Services. Annu. Rev. Environ. Resour. 32 (1), 67–98. doi: 10.1146/annurev.energy.32.031306.102758
Buhmann A., Papenbrock J. (2013). Biofiltering of Aquaculture Effluents by Halophytic Plants: Basic Principles, Current Uses and Future Perspectives. Environ. Exp. Bot. 92, 122–133. doi: 10.1016/j.envexpbot.2012.07.005. Sustainable cultivation and exploitation of halophyte crops in a salinizing world.
Burge C. A., Closek C. J., Friedman C. S., Groner M. L., Jenkins C. M., Shore-Maggio A., et al. (2016). The Use of Filter-Feeders to Manage Disease in a Changing World. Integr. Comp. Biol. 56 (4), 573–587. doi: 10.1093/icb/icw048
Burge C. A., Hershberger P. K. (2020). “Climate Change Can Drive Marine Diseases,” in Marine Disease Ecology (Oxford: Oxford University Press). doi: 10.1093/oso/9780198821632.003.0005
Carlson C. J., Hopkins S., Bell K. C., Doña J., Godfrey S. S., Kwak M. L., et al. (2020). A Global Parasite Conservation Plan. Biol. Conserv. 250, 108596. doi: 10.1016/j.biocon.2020.108596
Castro K. M., Stanley Cobb J., Gomez-Chiarri M., Tlusty M. (2012). Epizootic Shell Disease in American Lobsters Homarus Americanus in Southern New England: Past, Present and Future. Dis. Aquat. Organisms 100 (2), 149–158. doi: 10.3354/dao02507
Choudhury S., Sree A., Mukherjee S., Pattnaik P., Maringanti B. (2005). In Vitro Antibacterial Activity of Extracts of Selected Marine Algae and Mangroves Against Fish Pathogens. Asian Fish. Sci. 18 (2005), 285–294. doi: 10.33997/j.afs.2005.18.3.009
Cullen-Unsworth L., Unsworth R. (2013). Seagrass Meadows, Ecosystem Services, and Sustainability. Environ. Sci. Policy Sustain. Dev. 55, 14–28. doi: 10.1080/00139157.2013.785864
Davidson N. (2014). How Much Wetland Has the World Lost? Long-Term and Recent Trends in Global Wetland Area. Mar. Freshw. Res. 65, 936–941. doi: 10.1071/MF14173
DellaGreca M., Fiorentino A., Isidori M., Monaco P., Zarrelli A. (2000). Antialgal Ent-Labdane Diterpenes From Ruppia Maritima. Phytochemistry 55 (8), 909–913. doi: 10.1016/S0031-9422(00)00253-3
Deng Y., Liu S., Feng J., Wu Y., Mao C. (2021). What Drives Putative Bacterial Pathogens Removal Within Seagrass Meadows? Mar. pollut. Bull. 166, 112229. doi: 10.1016/j.marpolbul.2021.112229
Duffy J. E., Reynolds P. L., Boström C., Coyer J. A., Cusson M., Donadi S., et al. (2015). Biodiversity Mediates Top–down Control in Eelgrass Ecosystems: A Global Comparative-Experimental Approach. Ecol. Lett. 18 (7), 696–705. doi: 10.1111/ele.12448
Durai S., Radhakrishnan M. (2016). Antimicrobial Activity of Mangrove Leaves Against Drug Resistant Pathogens, : International Journal of PharmaTech Research in India Vol. 9. 141–146.
Evans S. M., Griffin K. J., Blick R. A. J., Poore A. G. B., Vergés A. (2018). Seagrass on the Brink: Decline of Threatened Seagrass Posidonia Australis Continues Following Protection. PLoS One 13 (4), e0190370. doi: 10.1371/journal.pone.0190370
Fourqurean J. W. (2002). HEMMINGA, M. A., AND C. M. DUARTE. 2000. Seagrass Ecology. Cambridge University Press. Xi+298 P. US$80. ISBN 0-521-66184-6. Limnol. Oceanogr. 47 (2), 611–611. doi: 10.4319/lo.2002.47.2.0611
Goldberg L., Lagomasino D., Thomas N., Fatoyinbo T. (2020). Global Declines in Human-Driven Mangrove Loss. Global Change Biol. 26 (10), 5844–5855. doi: 10.1111/gcb.15275
Graciaa D. S., Cope J. R., Roberts V. A., Cikesh B. L., Kahler A. M., Vigar M., et al. (2018). Outbreaks Associated With Untreated Recreational Water - United States 2000-2014. MMWR. Morb. Mortal. Wkly. Rep. 67 (25), 701–706. doi: 10.15585/mmwr.mm6725a1
Griffin D. W., Donaldson K. A., Paul J. H., Rose J. B. (2003). Pathogenic Human Viruses in Coastal Waters. Clin. Microbiol. Rev. 16 (1), 129–143. doi: 10.1128/CMR.16.1.129-143.2003
Groner M. L., Burge C. A., Cox R., Rivlin N. D., Turner M., Van Alstyne K. L., et al. (2018). Oysters and Eelgrass: Potential Partners in a High PCO2 Ocean. Ecology 99 (8), 1802–1814. doi: 10.1002/ecy.2393
Groner Ml, Eisenlord M. E., Yoshioka R. M., Fiorenza E. A., Dawkins P. D., Graham O. J., et al. (2021). Warming Sea Surface Temperatures Fuel Summer Epidemics of Eelgrass Wasting Disease. Mar. Ecol. Prog. Ser. 679, 47–58. doi: 10.3354/meps13902
Groner M. L., Maynard J., Breyta R., Carnegie R. B., Dobson A., Friedman C. S., et al. (2016). Managing Marine Disease Emergencies in an Era of Rapid Change. Philos. Trans. R. Soc. B: Biol. Sci. 371 (1689), 20150364. doi: 10.1098/rstb.2015.0364
Halpern B. S., Walbridge S., Selkoe K. A., Kappel C. V., Micheli F., D’Agrosa C., et al. (2008). A Global Map of Human Impact on Marine Ecosystems. Science 319, 948−952. doi: 10.1126/science.1149345
Harley C. D. G., Randall Hughes A., Hultgren K. M., Miner B. G., Sorte C. J. B., Thornber C. S., et al. (2006). The Impacts of Climate Change in Coastal Marine Systems. Ecol. Lett. 9 (2), 228–241. doi: 10.1111/j.1461-0248.2005.00871.x
Harvell C. D., Lamb J. B. (2020). Disease Outbreaks Can Threaten Marine Biodiversity. In Mar. Dis. Ecol. Oxford: Oxford Univ. Press. doi: 10.1093/oso/9780198821632.003.0008
Harvell C. D., Montecino-Latorre D., Caldwell J. M., Burt J. M., Bosley K., Keller A., et al. (2019). Disease Epidemic and a Marine Heat Wave Are Associated With the Continental-Scale Collapse of a Pivotal Predator (Pycnopodia Helianthoides). Sci. Adv, 5. doi: 10.1126/sciadv.aau7042
Herrera D., Ellis A., Fisher B., Golden C. D., Johnson K., Mulligan M., et al. (2017). Upstream Watershed Condition Predicts Rural Children’s Health Across 35 Developing Countries. Nat. Commun. 8 (1), 811. doi: 10.1038/s41467-017-00775-2
Hoenig J. M., Groner M. L., Smith M. W., Vogelbein W. K., Taylor D. M., Landers D. F. Jr., et al. (2017). Impact of Disease on the Survival of Three Commercially Fished Species. Ecol. Appl. 27 (7), 2116–2127. doi: 10.1002/eap.1595
Jacobs-Palmer E., Gallego R., Ramón-Laca A., Kunselman E., Cribari K., Horwith M., et al. (2020). A Halo of Reduced Dinoflagellate Abundances in and Around Eelgrass Beds. PeerJ 8, e8869. doi: 10.7717/peerj.8869
Johnson P. T. J., Preston D. L., Hoverman J. T., Richgels K. L. D. (2013). Biodiversity Decreases Disease Through Predictable Changes in Host Community Competence. Nature 494 (7436), 230–233. doi: 10.1038/nature11883
Keesing F., Ostfeld R. S. (2021). Impacts of Biodiversity and Biodiversity Loss on Zoonotic Diseases. Proc. Natl. Acad. Sci. 118 (17). doi: 10.1073/pnas.2023540118
Kim K., Kim H., Hong Lim J., Joon Lee S. (2016). Development of a Desalination Membrane Bioinspired by Mangrove Roots for Spontaneous Filtration of Sodium Ions. ACS Nano 10 (12), 11428–11433. doi: 10.1021/acsnano.6b07001
Kirk M., Esler D., Sean Boyd W. (2007). Morphology and Density of Mussels on Natural and Aquaculture Structure Habitats: Implications for Sea Duck Predators. Mar. Ecol. Prog. Ser. 346, 179–187. doi: 10.3354/meps07046
Kuehn W., Mueller U. (2000). Riverbank Filtration: An Overview. J. AWWA 92 (12), 60–69. doi: 10.1002/j.1551-8833.2000.tb09071.x
Lafferty K. D., Allesina S., Arim M., Briggs C. J., De Leo G., Dobson A. P., et al. (2008). Parasites in Food Webs: The Ultimate Missing Links. Ecol. Lett. 11 (6), 533–546. doi: 10.1111/j.1461-0248.2008.01174.x
Lafferty K. D., Harvell C. D., Conrad J. M., Friedman C. S., Kent M. L., Kuris A. M., et al. (2015). Infectious Diseases Affect Marine Fisheries and Aquaculture Economics. Annu. Rev. Mar. Sci. 7, 471–496. doi: 10.1146/annurev-marine-010814-015646
Lamb R. W., Smith F., Aued A. W., Salinas-de-León P., Suarez J., Gomez-Chiarri M., et al. (2018). El Niño Drives a Widespread Ulcerative Skin Disease Outbreak in Galapagos Marine Fishes. Sci. Rep. 8 (1), 16602. doi: 10.1038/s41598-018-34929-z
Lamb J., Van de Water J., Bourne D., Altier C., Hein M., Fiorenza E., et al. (2017). Seagrass Ecosystems Reduce Exposure to Bacterial Pathogens of Humans, Fishes, and Invertebrates. Science 355, 731. doi: 10.1126/science.aal1956
Lin B., Dushoff J. (2004). Mangrove Filtration of Anthropogenic Nutrients in the Rio Coco Solo, Panama. Manage. Environ. Quality: Int. J. 15, 131–142. doi: 10.1108/14777830410523071
Littman R. A., Fiorenza E. A., Wenger A. S., Berry K. L. E., van de Water J. A. J. M., Nguyen L., et al. (2020). Coastal Urbanization Influences Human Pathogens and Microdebris Contamination in Seafood. Sci. Total Environ. 736, 139081. doi: 10.1016/j.scitotenv.2020.139081
Lukasik J., Scott T. M., Andryshak D., Farrah S. R. (2000). Influence of Salts on Virus Adsorption to Microporous Filters. Appl. Environ. Microbiol. 66 (7), 2914–2920. doi: 10.1128/AEM.66.7.2914-2920.2000
Miller M. A., Miller W. A., Conrad P. A., James E. R., Melli A. C., Leutenegger C. M., et al. (2008). Type X Toxoplasma Gondii in a Wild Mussel and Terrestrial Carnivores From Coastal California: New Linkages Between Terrestrial Mammals, Runoff and Toxoplasmosis of Sea Otters. Int. J. Parasitol. 38 (11), 1319–1328. doi: 10.1016/j.ijpara.2008.02.005
Onufryk J., Ebersole J., Defilippo J., Beck G. (2018). Diadema Antillarum on St. Croix, USVI: Current Status and Interactions With Herbivorous Fishes. Yale J. Biol. Med. 91, 1. PMID: 30588206 PMCID: PMC6302631
Palacios O. A., Raúl Adame-Gallegos J., Estela Rivera-Chavira B., Nevarez-Moorillon G. V. (2021). Antibiotics, Multidrug-Resistant Bacteria, and Antibiotic Resistance Genes: Indicators of Contamination in Mangroves? Antibiotics 10 (9):1103. doi: 10.3390/antibiotics10091103
Qi S.-H., Zhang S., Qian P.-Y., Wang B.-G. (2008). Antifeedant, Antibacterial, and Antilarval Compounds From the South China Sea Seagrass Enhalus Acoroides51, 5, 441–447. doi: 10.1515/BOT.2008.054
Rahman M. M., Zimmer M., Ahmed I., Donato D., Kanzaki M., Xu M. (2021). Co-Benefits of Protecting Mangroves for Biodiversity Conservation and Carbon Storage. Nat. Commun. 12 (1), 3875. doi: 10.1038/s41467-021-24207-4
Raimondi P. T., Miner C., Ambrose R., Engle J. M., Minchinton T. E. (2002). Continued Declines of Black Abalone Along the Coast of California: Are Mass Mortalities Related to El Niño Events? Mar. Ecology-Progress Ser. - Mar. ECOL-PROGR Ser. 242, 143–152. doi: 10.3354/meps242143
Redder A., Dürr M., Daeschlein G., Baeder-Bederski O., Koch C., Müller R., et al. (2010). Constructed Wetlands–Are They Safe in Reducing Protozoan Parasites? Int. J. Hyg. Environ. Health 213 (1), 72–77. doi: 10.1016/j.ijheh.2009.12.001
Saad S., Muhammad T., Susanti D., Qaralleh H., Afifah Binti Abdul Rahim N. (2011). Antimicrobial Activity of Mangrove Plant (Lumnitzera Littorea). Asian Pacific J. Trop. Med. 4 (7), 523–525. doi: 10.1016/S1995-7645(11)60138-7
Sahoo G., Mulla N. S. S., Ansari Z. A., Mohandass C. (2012). Antibacterial Activity of Mangrove Leaf Extracts Against Human Pathogens. Indian J. Pharm. Sci. 74 (4), 348–351. doi: 10.4103/0250-474X.107068
Selakovic S., de Ruiter P. C., Heesterbeek H. (2014). Infectious Disease Agents Mediate Interaction in Food Webs and Ecosystems. Proc. R. Soc. B: Biol. Sci. 281 (1777), 20132709. doi: 10.1098/rspb.2013.2709
Sippo J. Z., Maher D. T., Tait D. R., Holloway C., Santos I. R. (2016). Are Mangroves Drivers or Buffers of Coastal Acidification? Insights From Alkalinity and Dissolved Inorganic Carbon Export Estimates Across a Latitudinal Transect. Global Biogeochem. Cycles 30 (5), 753–766. doi: 10.1002/2015GB005324
Smith J. R., Fong P., Ambrose R. F. (2006). Dramatic Declines in Mussel Bed Community Diversity: Response to Climate Change? Ecology 87 (5), 1153–1161. doi: 10.1890/0012-9658(2006)87[1153:DDIMBC]2.0.CO;2
Sorte C. J. B., Davidson V. E., Franklin M. C., Benes K. M., Doellman M. M., Etter R. J., et al. (2017). Long-Term Declines in an Intertidal Foundation Species Parallel Shifts in Community Composition. Global Change Biol. 23 (1), 341–352. doi: 10.1111/gcb.13425
Spalding M. D., Maricé L. (2021). The State of the World’s Mangroves 2021 ( Global Mangrove Alliance).
Sullivan B. K., Trevathan-Tackett S. M., Neuhauser S., Govers L. L. (2018). Review: Host-Pathogen Dynamics of Seagrass Diseases Under Future Global Change. Mar. pollut. Bulletin Securing Future Seagrass 134, 75–88. doi: 10.1016/j.marpolbul.2017.09.030
Sutton-Grier A. E., Sandifer P. A. (2019). Conservation of Wetlands and Other Coastal Ecosystems: A Commentary on Their Value to Protect Biodiversity, Reduce Disaster Impacts, and Promote Human Health and Well-Being. Wetlands 39 (6), 1295–1302. doi: 10.1007/s13157-018-1039-0
Thatoi H., Behera B. C., Mishra R. R. (2013). Ecological Role and Biotechnological Potential of Mangrove Fungi: A Review. Mycology 4 (1), 54–71. doi: 10.1080/21501203.2013.785448
Thompson R. M., Mouritsen K. N., Poulin R. (2005). Importance of Parasites and Their Life Cycle Characteristics in Determining the Structure of a Large Marine Food Web. J. Anim. Ecol. 74 (1), 77–85. doi: 10.1111/j.1365-2656.2004.00899.x
Tracy A. M., Pielmeier M. L., Yoshioka R. M., Heron S. F., Harvell C. D. (2019). Increases and Decreases in Marine Disease Reports in an Era of Global Change. Proc. R. Soc. B: Biol. Sci. 286 (1912), 20191718. doi: 10.1098/rspb.2019.1718
Voudanta E., Ar Kormas K., Monchy S., Delegrange A., Vincent D., Genitsaris S., et al. (2016). Mussel Biofiltration Effects on Attached Bacteria and Unicellular Eukaryotes in Fish-Rearing Seawater. PeerJ 4, e1829. doi: 10.7717/peerj.1829
Waycott M., Duarte C. M., Carruthers T. J. B., Orth R. J., Dennison W. C., Olyarnik S., et al. (2009). Accelerating Loss of Seagrasses Across the Globe Threatens Coastal Ecosystems. Proc. Natl. Acad. Sci. 106 (30), 12377–12381. doi: 10.1073/pnas.0905620106
Wijsman J. W. M., Troost K., Fang J., Roncarati A. (2019). “Global Production of Marine Bivalves. Trends and Challenges,” in Goods and Services of Marine Bivalves. Eds. Smaal A. C., Ferreira J. G., Grant J., Petersen J. K., Strand Ø (Cham: Springer International Publishing), 7–26. doi: 10.1007/978-3-319-96776-9_2
Wu S., Carvalho P. N., Müller J. A., Remony Manoj V., Dong R. (2016). Sanitation in Constructed Wetlands: A Review on the Removal of Human Pathogens and Fecal Indicators. Sci. Total Environ. 541, 8–22. doi: 10.1016/j.scitotenv.2015.09.047
Wu Y., Chung A., Tam N. F. Y., Pi N., Wong M. H. (2008). Constructed Mangrove Wetland as Secondary Treatment System for Municipal Wastewater. Ecol. Eng. 34 (2), 137–146. doi: 10.1016/j.ecoleng.2008.07.010
Keywords: parasite, disease ecology, coastal ecosystems and habitats, health, water quality, zoonotic
Citation: Klohmann CA and Padilla-Gamiño JL (2022) Pathogen Filtration: An Untapped Ecosystem Service. Front. Mar. Sci. 9:921451. doi: 10.3389/fmars.2022.921451
Received: 15 April 2022; Accepted: 14 June 2022;
Published: 11 July 2022.
Edited by:
Shan He, Ningbo University, ChinaReviewed by:
Jenny R. Hillman, The University of Auckland, New ZealandLydia Jeanne Baker, University of Miami, United States
Yanchen Sun, The University of Tennessee, Knoxville, United States
Copyright © 2022 Klohmann and Padilla-Gamiño. This is an open-access article distributed under the terms of the Creative Commons Attribution License (CC BY). The use, distribution or reproduction in other forums is permitted, provided the original author(s) and the copyright owner(s) are credited and that the original publication in this journal is cited, in accordance with accepted academic practice. No use, distribution or reproduction is permitted which does not comply with these terms.
*Correspondence: C. A. Klohmann, Y2FrMjY4QHV3LmVkdQ==
†These authors have contributed equally to this work and share first authorship