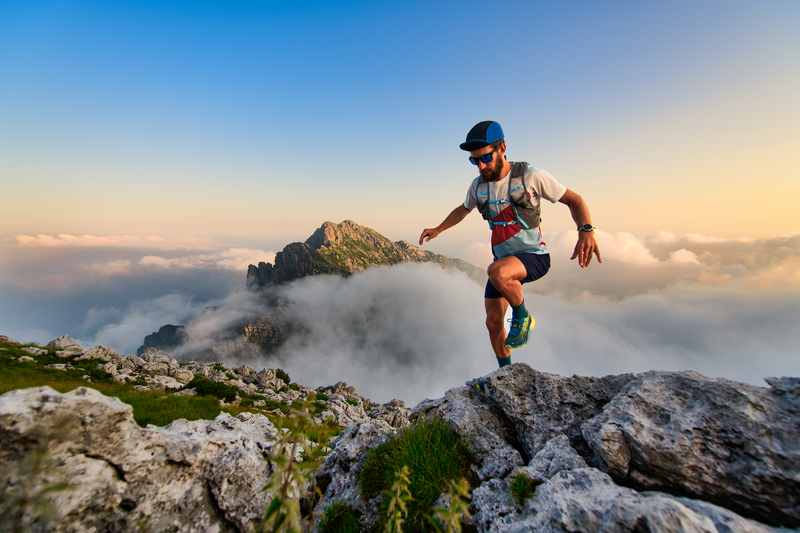
95% of researchers rate our articles as excellent or good
Learn more about the work of our research integrity team to safeguard the quality of each article we publish.
Find out more
ORIGINAL RESEARCH article
Front. Mar. Sci. , 28 July 2022
Sec. Ocean Solutions
Volume 9 - 2022 | https://doi.org/10.3389/fmars.2022.920480
This article is part of the Research Topic Nature-Based Solutions for Natural Hazards and Climate Change View all 17 articles
Nature-based strategies, such as wave attenuation by tidal marshes, are increasingly proposed as a complement to mitigate the risks of failure of engineered flood defense structures such as levees. However, recent analysis of historic coastal storms revealed smaller dike breach dimensions if there were natural, high tidal marshes in front of the dikes. Since tidal marshes naturally only experience weak flow velocities (~0-0.3 ms-1 during normal spring tides), we lack direct observations on the stability of tidal marsh sediments and vegetation under extreme flow velocities (order of several ms-1) as may occur when a dike behind a marsh breaches. As a first approximation, the stability of a tidal marsh sediment bed and winter-state vegetation under high flow velocities were tested in a flume. Marsh monoliths were excavated from Phragmites australis marshes in front of a dike along the Scheldt estuary (Dutch-Belgian border area) and installed in a 10 m long flume test section. Both sediment bed and vegetation responses were quantified over 6 experimental runs under high flow velocities up to 1.75 ms-1 and water depth up to 0.35 m for 2 hours. These tests showed that even after a cumulative 12 hours exposure to high flow velocities, erosion was limited to as little as a few millimeters. Manual removal of the aboveground vegetation did not enhance the erosion either. Present findings may be related to the strongly consolidated, clay- and silt-rich sediment and P. australis root system in this experiment. During the flow exposure, the P. australis stems were strongly bent by the water flow, but the majority of all shoots recovered rapidly when the flow had stopped. Although present results may not be blindly extrapolated to all other marsh types, they do provide a strong first indication that marshes can remain stable under high flow conditions, and confirm the potential of well-developed tidal marshes as a valuable extra natural barrier reducing flood discharges towards the hinterland, following a dike breach. These outcomes promote the consideration to implement tidal marshes as part of the overall flood defense and to rethink dike strengthening in the future.
Low-lying coastal and estuarine areas are increasingly exposed to flood risks as a result of climate change induced sea level rise, increasing storminess, associated storm surges, and land subsidence (Hallegatte et al., 2013; Tessler et al., 2015; Nicholls et al., 2021). Potential impacts in case of floods increase as coastal populations continue to expand (Neumann et al., 2015; Paprotny et al., 2018). This all results in a growing need for climate-resilient flood risk mitigation strategies (Hinkel et al., 2014; Morris et al., 2020; McEvoy et al., 2021). In addition to engineered flood defense structures, such as dikes, the conservation or creation of natural habitats such as tidal marshes and mangroves in front of flood defense structures, can provide additional nature-based flood risk mitigation, by reducing storm impacts on engineered structures (Vuik et al., 2016; Vuik et al., 2018; Zhu et al., 2020a), while at the same time providing ecological benefits such as increased biodiversity, water purification and carbon sequestration (Cheong et al., 2013; Temmerman et al., 2013; Teuchies et al., 2013; Schoonees et al., 2019; Smith et al., 2020). However, uncertainty remains about the functionality of natural habitats as buffers against flood risks under extreme storm conditions.
Relying only on earthen dikes or levees as flood defense structures is risky, as past storm events have shown that dikes can fail and may breach, with dramatic consequences for the communities living in the lowlands behind the dikes. For instance, dike breaching caused the death of more than 1800 people during the North Sea storm in 1953 in the Netherlands (Kabat et al., 2009), more than 1500 deaths due to Hurricane Katrina in 2005 in New Orleans, USA (Day et al., 2007), and displaced more than 100 000 people due to cyclone Aila in 2009 in Bangladesh (Auerbach et al., 2015). Dike breaches result from a structural failure of the dike, i.e., when hydrodynamic forces on the dike exceed the structural strength of the dike. During a storm surge, the hydrodynamic stress generated by high water levels, waves and tidal currents might reach this critical threshold, through mechanisms including dike overtopping by waves or flow, seepage (piping) through the dike, and dike erosion as a result (Vorogushyn et al., 2010; Danka and Zhang, 2015). In NW-Europe, dikes are often constructed of an inner core of non-cohesive sandy material, a top layer of cohesive sediment (i.e. clay or silt), and optionally/often a vegetated cover (Morris et al., 2009; van Loon-Steensma and Schelfhout, 2017). Once an initial disturbance of the top layer reaches the inner sandy core, this non-cohesive sediment will erode more easily, potentially leading to a rapidly expanding dike breach (Visser, 1998; Stanczak and Oumeraci, 2012; Peeters et al., 2015). In many embanked regions the land behind the dikes has a lower elevation compared to the sea or estuarine water level during a storm surge. Due to this elevation difference, a dike breach will result in strong flow velocities and deep flooding into the embanked areas.
In addition to improved response strategies like evacuation, the presence of natural tidal marsh habitats in front of dikes can play a role in mitigating the impacts of dike breaching. Recent analysis of historical dike breach events during the North Sea flood in 1953 in the Netherlands (Zhu et al., 2020a), showed that dike breaches were more narrow and more shallow when tidal marshes were present in front of dikes compared to breaches without tidal marshes in front of them. These findings suggest that tidal marshes serve as an extra natural ‘barrier’ that restricts the flow discharge towards the dike breach, thereby limiting breach growth and resulting breach width and depth (Figure 1). Calculations indicated that the reduced dike breach dimensions behind marshes decrease the flood discharge, and thereby the speed of flooding, the flood depth and hence the potential damage behind the breached dikes (Zhu et al., 2020a). As a result, evacuation procedures will be facilitated. As such, this study showed a new mechanism of nature-based flood risk mitigation by tidal marshes in front of dikes, in addition to the previously shown function of marshes for attenuation of storm waves, currents, surge levels and erosion (e.g. Möller et al., 2014; Spencer et al., 2015; Stark et al., 2015; Carus et al., 2016; Schoutens et al., 2019). Gaining in depth understanding of this new mechanism is highly valuable, as it may inspire novel nature-based flood designs and new integrated flood risk strategies (Zhu et al., 2020a).
Figure 1 Hypothetical explanation of the protective function of tidal marshes in front of a dike breach. An aerial image of two neighboring dike breaches (white circles) at the former Haringvliet estuary (the Netherlands) during the North Sea flood in 1953 (A). Illustration of how flow velocities and water volume differ in case of a dike breach with tidal marsh (left) and without tidal marsh in front of the dike (right) (B). Credit: Figure adapted from Zhu et al. (2020a).
However, key questions remain, as there is a lack of direct observations so far on the stability of tidal marshes under the high flow velocities that may be expected over a marsh towards a dike breach (Figure 1). In the exceptional case of a dike breach during storm surge conditions, flow velocities over a marsh towards a dike breach may reach up to several ms-1. Direct measurements of such situations are lacking, but estimations for the extreme storm surge and dike breach conditions in 1953 in the Netherlands (Figure 1) indicate that the storm surge level was up to 2.6 m above the marsh surface elevation, for which corresponding flow velocities (assuming critical flow conditions) may have reached almost 5 ms-1 in dike breaches (based on Zhu et al., 2020a). Flow velocities on a marsh right in front of a dike breach are expected to be lower, due to spreading of the flow over a larger width and due to drag, but may still be in the order of several ms-1. This is much more extreme than the normal tidal conditions under which marsh sediment beds and vegetation naturally develop ~0-0.3 ms-1 (Bouma et al., 2005a; Temmerman et al., 2012; Schoutens et al., 2019; Schoutens et al., 2020). Therefore, a crucial question is how stable a marsh can be under such high flow conditions, and hence whether it may serve as an extra natural barrier restricting the flow discharge towards the inundated land behind the dike breach (Figure 1). Or in other words, can we rely on the additional strength provided by the tidal marsh to the overall flood defense in reducing the flood risk i.e., preventing or limiting the breach to grow in depth and width?In general, marsh vegetation and the high intertidal elevation of marshes (i.e. reducing water depth) cause drag to the flow and reduce flow velocities (Carus et al., 2016; Schoutens et al., 2019) and wave heights (Möller et al., 2014; Silinski et al., 2016b; Schoutens et al., 2019). As a consequence of attenuating hydrodynamic forces from waves and currents, tidal marshes have the capacity to trap sediments and organic particles and as such build up elevation and strength (Brooks et al., 2021). Tidal marsh sediments typically have a high fraction of silt and clay particles in combination with a variety of small organic compounds, which increases the sediment cohesiveness (Grabowski et al., 2011; Winterwerp et al., 2012). Apart from the small-scale sediment composition, marsh sediments consist of a larger scale network of roots and rhizomes which forms an adhesive between sediments, sediment aggregates and organic compounds (Gyssels et al., 2005; Brooks et al., 2021; Chirol et al., 2021). Belowground plant structures in combination with the cohesive sediments reinforce the structural shear strength of the sediment bed (Shepard et al., 2011; Bouma et al., 2014). Previous flume experiments with simulated storm waves, have confirmed strong resistance of tidal marsh sediments to erosion (Möller et al., 2014; Spencer et al., 2016; Möller et al., 2019). Nevertheless, it is unknown how the tidal marsh vegetation and sediment bed will respond to the high flow conditions during a dike breach event. Moreover, storm surges in NW Europe are typically strongest in the winter season (Masselink et al., 2016; Hansen et al., 2019) when the aboveground plant shoots on tidal marshes die off, and their hydrodynamic attenuation capacity is reduced (Schoutens et al., 2019; Schulze et al., 2019). To understand the effect of high flow velocities on the stability of a tidal marsh with winter-state vegetation, measurements of marsh stability under such conditions are needed.In this study, we performed flume experiments with tidal marsh monoliths (1.2 m long x 0.8 m wide x 0.4 m high) extracted from the field, exposing them to very high flow velocities in the flume facility to explore the stability of tidal marshes. We studied (1) the resilience of the vegetation in its winter state in combination with (2) the erosion resistance of the sediment. The results of this study will be discussed in light of a new aspect of the nature-based shoreline protection function of tidal marshes, i.e. whether in case of a dike breach, tidal marshes could persist as an extra natural barrier, restricting the flow discharge towards the low land behind the breached dike, mitigate the impacts of a flood and reduce the flood risk.
This flume experiment was conducted in the Mesodrome flume facility at the University of Antwerp (Belgium) (Figure 2A). The flume consists of a 10 m long, 2.0 m wide and up to 1.5 m deep test section and has a maximum pump capacity of 0.6 m3 s-1. To generate very high flow velocities the width of the test section was reduced to 0.8 m. Within the test section, 8 monoliths were placed to create a marsh of 0.8 m wide and 9.6 m long (Figure 2B).
Figure 2 Overview of the flume dimensions and experimental setup (A). Schematic top view and side view of the experimental setup in the flume (B). The flow is generated by a pump that forces water through a collimator into the 0.80 m wide test section. Uniform flow was created by removal of the aboveground vegetation in the first meters of the test section.
The monoliths were excavated from tidal marshes in the Scheldt estuary (Figures 3A, B, 51.35 N, 4.23 E) as sediment blocks of 0.8 m by 1.2 m in surface area and 0.4 m depth, with vegetation growing on top. The monoliths were excavated on January 20 and 21, 2021, from brackish tidal marshes dominated by a mature Phragmites australis (Cav.) Trin. Ex Steud. Vegetation (Figures 3C, D) which can grow up to 4 meter high (Supplementary Figure 1).
Figure 3 Aerial picture showing the Scheldt estuary from the mouth to the city of Antwerp (A). The monolith extraction took place within the marshes along the Hedwige- Prosperpolder (HPP) at the Dutch-Belgian border (yellow line) (B) within a mature, high marsh location adjacent to the dike (C, D). Red arrows indicate the extraction location in the different figures.
Extraction was done by digging a pit, as such creating a vertical cliff in the sediment; then pushing a metal plate horizontally into the cliff at a depth of 0.4 m under the horizontal sediment surface (Supplementary Figure 2A); next a rectangular mold of 0.8 by 1.2 m in surface area and 0.4 m high was placed on top of the sediment surface and pushed down gently until it made contact with the metal plate (Supplementary Figure 2B). The marsh monolith, contained within a “box” created by the plate and the mold, was lifted and placed on a pallet covered with a thin horizontal, perforated multiplex board (i.e. to support the sediment block, to prevent cracks and to allow a little bit of drainage during the flume experiments). The mold was removed and vertical multiplex boards were attached around the monolith for transport by a truck (Figure 3D and Supplementary Figure 2C). After placing the monoliths in the flume with a crane, the protective vertical boards were removed from around the monoliths and they were positioned along the 10 m test section (Supplementary Figure 2D).
Aboveground biomass of the first 5.6 meters of the monoliths (at the leading edge of the test section) was removed to create a zone without vegetation for the incoming flow before that entered the zone with vegetation remaining along a length of 4 m at the end of the test section (Figure 2B). The remaining shoots were cut at a height just below the wooden beams crossing the flume (Figure 2A) to exclude interference of bending of the shoots with the beams. The beams were needed to keep the setup in place and withstand the hydrodynamic forces. As such the remaining vegetation stems were max. 1 m high. Small gaps in between the different monoliths and at the side edges were filled with sediment to ensure that the sediment bed was continuous across the flume test section. Before the monoliths were exposed to flow velocities, the flume was filled up with water to the sediment surface to let the sediment in the monoliths settle for two days. A first set of 8 monoliths was used as a pilot experiment to find out the desired settings (i.e. combination of water level and flume pump rate) to create maximum possible flow velocities with this flume setup. Next, the second set of 8 monoliths was installed in the flume to have undisturbed monoliths before starting the measurements of tidal marsh stability.
The experiments were conducted as six separate runs of 2 hours. Flow velocity and water depths remained constant during each run, but progressively increased from 1.00 to 1.75 ms-1 and from 0.15 to 0.35 m with each new run (Table 1). Flow conditions were defined by the Reynolds number (Re):
Table 1 Overview of the six experimental runs with varying vegetation cover (m), water depth (d, cm), water surface slope (%), flow velocities (vin, ms-1), Reynolds (Re) and Froude (Fr) numbers within the vegetation test section.
with vin as depth averaged flow velocity (ms-1), R as hydraulic radius which is defined as the cross-sectional area, A (i.e. for rectangular flumes: A = w.d with w being the flume width which was 0.8 m), divided by the wet perimeter of the flow (i.e. for rectangular flumes: 2d + w) and v being the kinematic viscosity (10-6 m2s-1 for water); and the Froude number (Fr):
with g as the gravitational acceleration (9.81 ms-2) and D as the hydraulic depth which is defined as A/w, which in a rectangular flume is equal to the water depth, d.
The effect of aboveground vegetation cover on the sediment stability was tested by starting with two runs with the original vegetation cover present over 4 m of length of the test section (run 1 and 2), followed by consecutively manual removal (clipping) of 2 m of vegetation (run 3 and 4) and ending without vegetation cover (run 5 and 6). Flow velocities were measured every run with an electromagnetic flow meter (EMF, Valeport model 801, Totnes, UK) in the middle of the flume width and along a vertical depth gradient with an interval of 5 cm. The flow was measured 4.0 m and 0.8 m before the end of the test section (Figure 2B). Flume-wall effects on the flow were regularly checked by expanding the measurement of flow velocities over a cross-sectional grid. At the same positions, water depth was measured to calculate the slope of the water surface.
Site-specific sediment composition was quantified on six surficial sediment samples after removing the top layer of plant litter. Samples from the sediment bed were taken with a Kopecky ring (5.0 cm in diameter and 5.1 cm high and five replicates) and used to determine dry bulk density (after drying at 70°C for 72 h). Next, six mixed scrape samples of the top 2 cm were used to perform volumetric grain size analyses with a Mastersizer 2000 (Malvern) based on laser diffraction after a combined H2O2 and HCl treatment to remove organic compounds and disperse aggregates. Organic matter content was determined with the loss on ignition method, i.e., by ashing the samples for 4 hours at 550°C in a muffle furnace (Heiri et al., 2001). Shear strength was estimated based on four replicates with a pocket shear vane tester (Eijkelkamp, NL) for the surface sediment and a field inspection shear vane tester (Eijkelkamp, NL) at 10 cm depth. Penetration resistance of the sediment was measured on four replicates with a penetrologger (Eijkelkamp, NL) with a 1 cm depth interval, and an average of the upper 10 cm was calculated. All samples were taken in close approximation of the monolith extraction site, i.e. within 1-2 meters.
The marsh was covered with a homogenous P. australis vegetation in winter-state, i.e. the aboveground biomass consisted of dead, leafless stems, and leaf litter was lying on the sediment bed in between the standing stems (Supplementary Figure 3). The reed vegetation was characterized in the field in the same week as the monolith extraction (end of January 2021). Shoot densities were counted at three replicate 0.40 x 0.40 m square plots before all aboveground biomass was harvested and dried at 70°C for 72 h to quantify the aboveground biomass. Shoot lengths and basal shoot diameters were measured on 20 shoots, which were harvested to measure biomechanical properties, i.e. the flexural stiffness and Young’s modulus. For the latter, the basal 20 cm of the shoots were used to perform three-point bending tests with a universal testing device (Instron 5942, precision ± 0.5%). For more details on the methods to quantify the biomechanical properties we refer to Schoutens et al. (2021). Belowground biomass was quantified from five replicate sediment cores of 0.10 m diameter sampled up to 0.40 m depth (i.e. the same depth as the monoliths), which were sampled at the location of monolith extraction. The cores were frozen and cut into slices (0-2.5 cm; 2.5-5.0 cm; 5.0-10 cm; 10-20 cm; 20-30 cm; 30-40 cm). For each segment, the sediment was washed out and the remaining belowground biomass was dried (at 70°C for 72 h) and weighed.
Within the vegetated test section, 20 shoots were monitored during the first two runs (i.e. 4 meters of vegetation cover). In the third and fourth experimental run, 12 remaining shoots were monitored. In the fifth and sixth run, all vegetation was removed. The bending of the shoots in response to the high flow velocities was quantified in six categories indicating the shoot bending angle compared to the initial situation before the experimental runs. The categories ranged from shoots that did not suffer any damage or reconfiguration (< 5° bending angle) up to heavily bent shoots (> 35°) and broken shoots (i.e. flushed away or clearly broken shoots). The measurements were done at different moments in time, i.e. during the experimental run, directly after the run when the flow was stopped and after one (or three for run 4) day(s) of recovery.
Bed level changes (by erosion or sedimentation) were quantified by measuring the elevation of the sediment bed before and after every experimental run. Pin measurements [following a Sedimentation Erosion Bar, SEB, approach, see Nolte et al. (2013)] were performed along a grid over the 4 m long and 0.8 m wide vegetated part of the test section with a 5 cm interval in the direction parallel to the flume length and a 10 cm interval perpendicular to the flume length, revealing a total of 444 point measurements.
Tidal marsh monoliths were exposed to six consecutive runs of two hours each, with water depths ranging from 0.15 to 0.35 m and depth-averaged flow velocities ranging from approximately 1.00 to 1.75 ms-1 (Table 1). Flow velocities increased and the water surface slope decreased from runs 1 and 2 (with vegetation over 4 m of the flume length) to runs 3 and 4 (vegetation partially removed and remaining present over 2 m) and runs 5 and 6 (without vegetation cover) (Table 1). The combined effect of the flume side walls and the rearrangement of the plant shoots (in response to the flow) towards the center of the flume caused variations of flow velocities over the width of the flume with a standard deviation of 0.1 – 0.2 ms-1. Flow conditions during all runs were estimated to be sub-critical to nearly critical (estimated Froude numbers between 0.81-0.98) and highly turbulent (Reynolds numbers > 105) (Table 1). The sediment was characterized by a high silt fraction (~72%; 2-63 µm) and clay fraction (~17%; < 2 µm), around 20% of organic matter, and relatively high values of shear strength and penetration resistance (Tables 2A, B). P. australis vegetation in winter has a more modest aboveground biomass compared to the summer situation i.e., smaller, thinner and more flexible shoots (Table 3). The majority of belowground biomass was found between 10 and 30 cm depth. At a depth below 30 cm, belowground biomass decreased (Figure 4). The sediment bed is covered with a layer of litter (e.g. old leaves) under which a superficial network of fine roots can be found (Figure 8 and Supplementary Figure 3).
Table 2 Overview of the sediment characteristics (A) at the extraction site presented as the mean, standard deviation (SD) and sample size (N). (B) Field studies with recording of shear vane shear strength measurements near the sediment surface in mature tidal marshes.
Table 3 Aboveground and belowground properties of reed vegetation (left) at the monolith extraction sites presented as the mean, standard deviation (SD) and sample size (N) and (right) compared to peak biomass measurements in literature (mean ± SD).
Figure 4 Belowground biomass up to 40 cm depth represented as boxplots with outliers shown as black dots.
Figure 8 Sediment surface after the last experimental run with the emerging shallow subsurface mat of fine roots. Note that above-ground shoots have been manually clipped.
Vegetation of P. australis in its winter state was able to cope with short-term very high flow velocities. The response of the reed vegetation and the sediment bed dynamics after an experimental run were the cumulative result of all previous experimental runs. During the two hour runs, the shoots were bent heavily in the direction of the flow, but recovered rapidly after the flow was stopped (Figure 5). Even after four consecutive runs, total damage remained limited as less than 17% of the sampled shoots were broken and less than 17% were bent more than 35°.
Figure 5 Percentage of the reed shoots (n = 20 for run 1 and 2; n = 12 for run 3 and 4) that show a response in terms of breaking and bending angle when exposed to high flow velocities. The response was quantified in six response categories (broken shoots, and 5 shoot bending angles) and was measured at three moments in time (during the flow, directly after the flow stopped, 1 day later after runs 1-3 and 3 days later after final run 4).
Over the entire period of the six experimental runs (i.e., 12 hours cumulated exposure time), vertical erosion was limited to a median (± standard deviation) cumulative total erosion of 6.7 ± 2.4 mm only. Over individual runs (2 hours), the median erosion was maximum 2.5 ± 2.5 mm (for run 2) and less than 1.0 mm for all other runs. The first run removed part of the litter and organic debris that was initially covering the sediment surface (Figure 6 and Supplementary Figure 3). In the sections where aboveground vegetation was removed, even more litter was removed in the run directly after removal (i.e. in the 4.0 – 2.0 m and 2.0 – 0.0 m distance from the end of the test section in run 3 and run 5 respectively). The presence or absence of standing P. australis shoots had no effect on the bed elevation changes (e.g. ANOVA comparing the vegetated section with the non-vegetated section in run 4: F1,387 = 0.171, p = 0.68) and no systematic spatial patterns were found throughout the six runs (Figure 6). Although there is a general trend of slight erosion, at some locations sediment accretion was also observed (Figures 6, 7). Apart from the general trend of limited erosion, outliers of several centimeters of erosion and deposition were observed throughout the entire experiment, as a result of translocated sediment aggregates (Figure 7). After the 5th and 6th run, i.e. respectively after 10 h and 12 h of cumulative high flow velocities, first signs of uprooting appeared and revealed a shallow subsurface mat of fine roots (Figure 8).
Figure 6 Top view of the flume showing the spatial interpolation of the elevation changes measured during the experimental runs (Run 1 - 6). The elevation changes were calculated as the differences in surface elevation before and after each experimental run. The contours are based on a raster of 444 pin measurements (see methods). White spaces represent missing data. Gray bars represent areas where the space between the pins was 10 cm instead of 5 cm (because of obstruction of the wooden beams supporting the flume construction, see Figure 2).
Figure 7 Boxplots summarizing the sediment bed elevation changes as a result of the different experimental runs with outliers represented as black dots. The data were split between the first two meters of the test section (having a vegetation cover in runs 1-2, and no vegetation in runs 3-6) and the back-end two meters (with vegetation in runs 1-4, no vegetation in runs 5-6). Presence or absence of vegetation is indicated by green or brown colors, respectively.
In face of global climate change, nature-based shoreline and flood protection strategies are increasingly proposed as an adaptive measure to increase the climate-resilience of traditional, engineered flood protection structures. A recent analysis of historic dike breaches highlighted that tidal marshes, when present in front of dikes, can limit the breach-depth in a dike, and hence serve as an extra natural barrier limiting flooding in case of a dike breach (Zhu et al., 2020a). To gain more insights in the actual robustness of these nature-based solutions, we exposed for the first time extended marsh-lengths to very high flow conditions as are likely to happen when a dike breaches behind the marsh. Our flume tests revealed that both the marsh sediment and marsh vegetation show a high resistance against erosion by extended periods of very high flow velocities.
The results in this study suggest that the reduction of aboveground biomass in winter, reduces the experienced drag which then promotes the resistance of the vegetation against high flow velocities. We tested the winter state stability of P. australis vegetation, which is a typical dominant species in the high intertidal zone of brackish tidal marshes in NW European estuaries and in many other brackish and freshwater tidal estuaries worldwide (Srivastava et al., 2014). We found a high capacity of P. australis to withstand high flow velocities, as most of the aboveground stems (83%) did not break, of which 66.5% had a less than 25° bending angle at the end of all flume runs (Figure 5). At first sight, this finding of high resistance of P. australis to high flow velocities may be contrasting with previous studies, showing that P. australis vegetation has a lower tolerance to strong hydrodynamic forces, in comparison to the pioneer marsh species that grow on lower intertidal elevations in the brackish parts of NW European estuaries (Coops et al., 1996; Asaeda et al., 2005). Yet, this apparent contradiction between our findings and previous studies may be explained by the following hypotheses. Firstly, these previous studies focused on the growth of P. australis under average hydrodynamic conditions during a whole growing season instead of short-term extremes in winter conditions. The extreme flow conditions generated on the tidal marsh platform close to a dike breach are expected to last for only a limited time period i.e. usually only one or two high tides that coincide with the storm surge event with a marsh inundation depth of several meters and inundation time of two to four hours per high tide (Stark et al., 2015; Smolders et al., 2020; Zhu et al., 2020a). Once the storm surge has passed, normal tidal conditions prevail again, with very shallow water depths (<0.3 m during high spring tides and even no flooding during neap tides) and weak flow velocities (<0.3 ms-1) typically found for the high intertidal marshes investigated here (Bouma et al., 2005a; Temmerman et al., 2012). Hence, the capacity of P. australis shoots to cope with high flow velocities is only required for a limited time period in the order of several hours, while previous studies assessed the growth response of P. australis to hydrodynamic conditions over a whole growing season (many months) (Coops et al., 1996; Asaeda et al., 2005). Secondly, P. australis drops its leaves in winter, which might further contribute to its higher capacity to withstand high flow velocities, as compared to the previous studies on P. australis in summer condition. The vulnerability of tidal marsh vegetation to experience stress from hydrodynamic forces is known to be dependent on plant traits that generate high drag forces on the shoots, i.e. plants with high biomass, tall shoots, and stiff stems experience higher drag forces under given hydrodynamic conditions (Bouma et al., 2005b; Schoutens et al., 2020). It should be mentioned that to fit the flume setup of this experiment, P. australis shoots were shortened, which could have promoted the resistance during the flow and the recovery process after the flow i.e., shorter shoots experience less drag force during the flow and less downward force during recovery. Nevertheless, compared to values reported in literature for typical P. australis summer vegetation, the vegetation from which monoliths were extracted for this experiment had a lower shoot biomass, and stems were thinner and smaller when monolith excavation took place at the start of the winter season in January 2021 (Table 3). Moreover, compared to the stiff shoots reported for P. australis in summer (Table 3), our results suggest that the remaining winter shoots have a higher flexibility. This might be because the stiffest shoots brake and get washed away and only the slightly more flexible shoots remain in winter, which is in line with studies that monitored shoot stiffness of P. australis over an entire season (Zhu et al., 2020b).
P. australis winter vegetation in front of a dike can handle short-term high flow velocities, i.e. a cumulative 12 hours in this experiment, without losing much of the shoot biomass (<17% of stems). This finding indicates that after a dike breach has been repaired, the marsh has a high chance to continue providing its shoreline protection function through vegetation-induced attenuation of waves, currents and erosion (Möller et al., 2014; Spencer et al., 2015; Carus et al., 2016; Schoutens et al., 2019; Sheng et al., 2021; Zhang et al., 2022). Furthermore, through survival of the marsh vegetation, the marsh can also sustain its capacity to accumulate suspended sediments that are supplied during regular tidal inundations (Temmerman et al., 2003; Silinski et al., 2016a) and as such, to build up elevation in balance with long-term sea level rise (Temmerman et al., 2004). Studies have demonstrated that tidal marshes in front of dikes, that grow vertically in balance with sea level rise, are very effective in sustaining their nature-based mitigation of waves, and reduction of wave loads on the dikes, under future scenarios of sea level rise (Vuik et al., 2019; Zhu et al., 2020a). Our findings indicate that dike breaching behind marshes, after breaches are repaired, will not compromise this long-term nature-based shoreline protection function of the marshes.
The sediment surface in this experiment was highly resistant against erosion by high flow velocities (Figures 5, 6). Apart from some outliers, the elevation changes ranged predominantly between – 5 and + 5 mm. Here we note that this range of elevation change is not much more than the measurement accuracy of the SEB method (1.5 mm) (van Wijnen and Bakker, 2001; Nolte et al., 2013). Studies on the sediment stability of tidal marshes against vertical erosion under storm surge conditions confirm the highly stable nature of tidal marsh sediments, both found in flume studies mimicking storm conditions (Möller et al., 2014; Spencer et al., 2016) and field assessments after storms (Pennings et al., 2021). Nevertheless, there are also field studies reporting considerable erosion of marshes after very severe storms, such as in freshwater marshes on the Mississippi deltaic plain after the severe 2005 hurricane season (Howes et al., 2010). Moreover, the generated flow velocities in our study go beyond the flow conditions that may be expected on a marsh during a storm surge (Bennett et al., 2020). Nevertheless, the sediment bed remained stable which indicates that the shear strength of the sediment in our experiment was higher than the exerted shear stress (De Smit et al., 2021). In the subsequent paragraphs, we discuss one by one a total of four possible explanations for the observed extremely high erosion resistance of the sediment bed.
Firstly, sediment composition is known to be a key determinant for the susceptibility to erosion of sediment surfaces (Lo et al., 2017; De Battisti et al., 2019; Evans et al., 2021). Thus our finding of high sediment bed resistance to vertical erosion is likely to also be determined by case-specific sediment properties. The grain size distribution of the mineral sediment fraction plays a major role. Erosion resistance of marsh sediments is known to increase with decreasing grain size and associated increasing cohesiveness (Christiansen et al., 2000; Feagin et al., 2009; Lo et al., 2017). Tidal marshes that are situated in front of a dike are often situated relatively high in the tidal frame, being sheltered from the incoming hydrodynamics at the shoreward marsh edge and experiencing relatively shallow flooding at high spring tides, allowing fine sediments to settle. This may explain the high proportions of small sediment fractions, i.e. silt and, to a lesser extent, clay, in the tidal marsh monoliths used in our experiment (Table 2A). The high silt and clay content promotes sediment cohesion and is most likely the first reason why our sediment had such a high resistance against erosion.
Secondly, high elevated, mature marshes in front of a dike, like in our case, are typically characterized by strongly consolidated sediments (Tempest et al., 2015), which increases the erosion resistance of the sediment surface (Watts et al., 2003). This is in line with our penetration resistance measurements that were comparable with values found in NW European salt marshes ranging around 200 kPa in the upper sediment layers (e.g. Are et al., 2002; van de Vijsel et al., 2020). Dry bulk densities in this study indicate a soil texture that is favorable for root growth and water drainage (Bradley and Morris, 1990). Although bulk densities are often higher in deeper soil layers, i.e. more compacted, bulk densities in the top few centimeters in this study where in line with values measured in other, natural marshes along NW European marshes, ranging between 0.50 – 0.65 g.cm-3 (Crooks and Pye, 2000; Watts et al., 2003; Tempest et al., 2015; Schulte Ostermann et al., 2021).
Thirdly, organic matter content has a positive impact on the erosion resistance of minerogenic tidal marsh sediments and consists of small organic substances and larger belowground root biomass. Our measurements of Loss on Ignition combine these two components and revealed a relatively high fraction of organic matter in the sediments (20.03 ± 0.88%), compared to values in literature ranging between 6-20% for natural mature marshes (Crooks and Pye, 2000; Watts et al., 2003; Tempest et al., 2015; Gillen et al., 2021). In addition to clay particles, small organic substances increase the sediment cohesiveness by forming an adhesive between sediment particles, creating bigger sediment aggregates. Nevertheless, in marshes dominated by organic material (i.e. 80-90% of the sediment fraction), the sediment properties will be different with a lower bulk density and less consolidation (Brooks et al., 2021) which reduces the sediment stability and increases potential erosion processes (Chambers et al., 2019; Himmelstein et al., 2021).
Fourthly, the presence of vegetation can increase the stability of the sediment by (i) reducing hydrodynamic forces due to friction between the aboveground shoots and the moving water and (ii) by providing structural rigidity for sediments and aggregates through the belowground root system (Vannoppen et al., 2015; Cahoon et al., 2020). The sediment surface stability under the short-term high flow velocities in this study was not affected by the presence or absence of aboveground biomass (Figures 6, 7). Rapid removal of the dead organic litter and debris covering the sediment surface was observed after the first experimental runs. In natural marsh conditions, however, the larger scales (i.e., less boundary conditions and larger surface areas) might result in a redistribution of this organic matter rather than complete removal as observed in this flume experiment, hence providing a local shielding function for the sediment surface. Although logistical restrictions did not allow to install fresh, undisturbed monoliths for every single run, the elevation changes in run 2-6 might be more similar to the observations after run 1 in which there was a redistribution of organic matter rather than a removal. During the high flow velocities, P. australis shoots were completely bent over (Figure 5), hence the friction with the water column was reduced. Although not directly tested, the high fraction of organic matter and the high portion of root biomass at the sediment surface might have had an important contribution to the sediment stability. That is, a high fraction of root biomass near the sediment surface (Figure 4) might cause a decrease in bulk density by creating pores and voids between the sediment particles (Brain et al., 2012; Chen et al., 2012; Jafari et al., 2019). Root networks change the sediment characteristics both through the presence of dead and living roots which then function as a structural framework for the sediment aggregates, promote the formation of pores and enhance drainage capacity, hence increasing the erosion resistance of the sediment surface (Gyssels et al., 2005; Grabowski et al., 2011; Evans et al., 2021). Only in the two highest flow conditions (after 10-12 h of cumulative flow exposure), uprooting of fine roots covering the entire sediment surface was observed (Figure 8). In addition, when roots get exposed at the sediment surface, they will cause local turbulence, which may result in scour features (Bouma et al., 2009; Schoutens et al., 2021). This might suggest that when high flow velocities would continue for a much longer period of time, the top layer of sediment may get damaged or removed, exposing the subsurface sediment layers with lower belowground biomass. Moreover, vertical variations in the shape of the roots, e.g. from dense fine roots in the upper sediment layers towards sparser thicker roots in deeper layers (Gillen et al., 2021) or reduced belowground biomass with increasing depth (Howes et al., 2010), can alter the shear strength too. Nevertheless, subsurface shear vane tests indicated highly stable sediments limiting the erosion risk (Table 2A), even when the top layer of sediment including the dense root network is gone.
Despite the fact that we simulated maximal, near to critical flow conditions in our flume experiment, with depth averaged flow velocities up to 1.75 ms-1 (Table 1), we recognize that the water depths in our experiment were, for practical reasons, limited to maximum 0.35 m. Under extreme storm surge conditions, when water depths on marshes can be as much as 1.5 to 2.5 m (e.g. Stark et al., 2015; Zhu et al., 2020a), it may be well possible that maximum flow velocities over a marsh nearby a dike breach reach up to several ms-1. Therefore, the present flume experiment needs to be regarded as a first test, which reveals promising results on tidal marsh stability and motivates further testing under larger water depths and higher flow velocities.
Current findings are based on one specific tidal marsh characterized by fine, cohesive sediments with a high shear strength and a monospecific P. australis vegetation. Although we argue that there are marshes with similar characteristics, we recognize that many other types of vegetated marshes exist e.g., minerogenic and organogenic marshes. Although this study provides insights in the stability of tidal marshes under high flow velocities, further research would be needed to confirm our findings for a wider range of hydrodynamic conditions and variety of marsh types.
Further research should focus on increasing the water depth and the flow velocities to simulate more extreme storm surge conditions. Field experiments with in situ, controlled dike breaches could be an option, however they remain logistically challenging (Peeters et al., 2019, Peeters et al., 2015; Wu et al., 2011). Furthermore, such controlled dike breach experiments are usually conducted during calm or moderate weather conditions and during normal tidal conditions, when water depths and flow velocities over marshes are small compared to extreme storm surge conditions. Finding suitable test locations with low elevated marshes in front of a dike is challenging, as the opportunities for in situ dike breach experiments are very limited. Lowering the marsh platform or lowering the dike could be an option to simulate the water depths and flow velocities expected during a dike breach. Experiments in large and deep flume facilities could be an alternative to control the water depth and flow velocity without taking into account the natural tidal cycling.Apart from experiments with more extreme flow conditions, exploring the sediment stability of different types of vegetated marshes is advised. Several studies point towards the role of plant traits in stabilizing the sediment based on the structure of the root network (Howes et al., 2010; Jafari et al., 2019; Gillen et al., 2021). In this study, P. australis formed dense, shallow mats of roots which could benefit the sediment stability compared to species that only develop roots in the deeper sediment layers, suggesting that the type of roots and their structure might play an important role in the sediment stability (Feagin et al., 2009; De Battisti et al., 2019). Other studies emphasize the effect of sediment composition, such as the decreasing sediment stability in function of an increasing fraction of organic substances (Gillen et al., 2021) or an increasing fraction of coarser, sandy sediments (Schoutens et al., 2019; Evans et al., 2021). Moreover, climate induced environmental changes might alter the stability of tidal marshes, i.e. increased stress from inundation by sea level rise and increased hydrodynamic forces from storms could alter the sediment dynamics (Schuerch et al., 2018; Jiang et al., 2020) and the growth of tidal marsh vegetation (Kirwan and Guntenspergen, 2012). Therefore, testing the sediment stability and stabilizing effect of different vegetation types in different environmental settings (e.g. climate) will contribute to the generality of our findings and could improve our understanding of how the stability of vegetated marshes under extreme flow velocities could change in the future. In the first place, we advise to test vegetated marshes which typically occur in front of dikes, such as salt marsh species (e.g. Elymus sp. or Spartina sp.), freshwater marsh species and tropical systems such as mangroves.In the present study, we focus on a mature, high elevated marsh adjacent to the dike. However, the response to extreme flow velocities might be different in marshes with a more complex geomorphology created by features such as creeks (Symonds and Collins, 2004). Moreover, young, low-lying developing marshes are expected to have different, less stable sediment characteristics (Evans et al., 2021), which is especially the case when tidal marshes are (re)created, e.g. in managed realignment projects (Tempest et al., 2015; Van Putte et al., 2020). Implementations of nature-based shoreline protection by tidal marshes has increased over the past decades and will further increase in the future. Many projects involve new marsh development on low elevated, initially bare flats in front of the embankment, where sediment accretion might be relatively fast (Vandenbruwaene et al., 2011; Oosterlee et al., 2018), resulting in less consolidated sediments, low bulk densities, poor drainage and hence lower shear strengths (Watts et al., 2003; Van Putte et al., 2020). Experience from existing tidal marsh (re)creation projects suggests that it might take several years before marshes develop with sediments that have enough strength to withstand high shear stresses (Fearnley, 2008; Kadiri et al., 2011; Tempest et al., 2015; Sha et al., 2018) as can be expected on the marsh platform in front of a dike breach. In this respect, further research is needed on the rates of development of marsh sediment strength, as marshes develop from young pioneer marshes to older established marshes.
Our results indicate that conservation, restoration and creation of tidal marshes in front of engineered flood protection structures such as dikes, provides an extra natural ‘barrier’ that can remain stable under high flow velocities, and as such may reduce flood depths and damage in case of breaching of the engineered dike. Note that this extra barrier-function requires stable high marshes which need time to develop, but which have the capacity to adapt and develop with changing environmental conditions such as sea level rise. Hence, instead of constructing dikes directly adjacent to the mean low water level, thereby embanking and losing pre-existing tidal marshes and diminishing the chance of new seaward tidal marsh establishment, we stress the benefits of providing enough space for tidal marshes in front of dikes. In areas where coastal development by building of dikes is ongoing, such as in Tianjin (China), Jakarta (Indonesia), or Port Harcourt (Nigeria) (Martín-Antón et al., 2016; Sengupta et al., 2018; Zhang et al., 2020), land reclamation often leads to degradation of many of the ecosystem functions and can bring extra costs that are often not accounted for in the reclamation project (Wang et al., 2010; Tan et al., 2020). Therefore, preserving part of the tidal marshes in front of newly constructed dikes is strongly advised.
In cases where no tidal marshes are present, the creation of suitable conditions for marsh development is recommended (Van Loon-steensma and Slim, 2013). At locations with enough space shoreward of the dikes (e.g. along shallow bays or lagoons), tidal marsh establishment can be facilitated by creating shallow, sheltered conditions by sediment nourishment and building (temporal) barriers to reduce hydrodynamics and capture sediments (Hofstede, 2003; Dao et al., 2018; Vuik et al., 2019; Baptist et al., 2021; Hu et al., 2021). Furthermore, active planting of marsh plants may eventually further enhance the chances of tidal marsh establishment (Tagliapietra et al., 2018).
At locations where space is limited shoreward of dikes (e.g. embanked estuaries with narrow shipping channels), it is worth to explore areas where there is space for more landward building of a new dike and breaching of the existing dike, so-called managed coastal realignment. Managed realignment creates a sheltered environment, suitable for tidal marsh development between the breached and the new dike. This managed realignment strategy has been implemented in several places over the last decades (Esteves and Williams, 2017; van den Hoven et al., 2022), for instance in Belgium where a total of 2500 ha of tidal marshes will be (re)created by 2030 (Temmerman et al., 2013). In places where managed realignment is not a desirable option, one could think about double-dike systems with transitional polders (i.e., see Figure 7 in Zhu et al., 2020). This implies that after a high marsh has established, the land can be converted back to its former (often agricultural) function, while still offering the benefits of an elevated foreshore. However, as stated before, it is important to account for the time needed to develop stable marsh sediments. This means that if we think nature-based solutions are a promising option to cope with sea-level rise, we must plan well in advance in order to initiate the development of new marshes as part of any kind of nature-based flood protection program.
The raw data supporting the conclusions of this article will be made available by the authors, without undue reservation.
KS: Preparation of the experiment, performing the measurements, data analysis and visualization, interpretation of results, writing - review & editing. MS: Performing the measurements, data analysis, writing. MB: Experimental design, interpretation of results, review & editing. KH: Experimental design, interpretation of results, review & editing. TB: Provisioning of facilities, experimental design, performing the measurements, interpretation of results, review & editing. SA: Experimental design. PH: Experimental design. JL-S: Experimental design, interpretation of results, writing - review & editing. PM: Provisioning of facilities, experimental design. JS: Provisioning of facilities, preparation of the experiment, experimental design, interpretation of results, writing - review & editing, PP: Provisioning of facilities, preparation of the experiment, experimental design, interpretation of results, writing - review & editing. ST: Provisioning of facilities, preparation of the experiment, experimental design, interpretation of results, writing - review & editing, supervision. All authors contributed to the article and approved the submitted version.
The Mesodrome flume facility was funded through AnaEE-Flanders projects I001921N and G0H4117N. We would like to thank the funders of this research, i.e. Interreg Polder2C’s consortium funding the Living lab Hedwige-Prosperpolder project and personal funding from the Research Foundation Flanders, Belgium (FWO, PhD fellowship for fundamental research K. Schoutens, 1116319 N). JS is grateful to the Antwerp University Research Fund (BOF; Project no. 43171). SM, MB, KV, TB, SA., PH, JL-S. are part of the project The Hedwige-Prosper Polder as a future-oriented experiment in managed realignment: integrating saltmarshes in water safety (with project number 17589) of the research programme ’Living Labs in the Dutch Delta’ which is (partly) financed by the Dutch Research Council (NWO).
The authors declare that the research was conducted in the absence of any commercial or financial relationships that could be construed as a potential conflict of interest.
All claims expressed in this article are solely those of the authors and do not necessarily represent those of their affiliated organizations, or those of the publisher, the editors and the reviewers. Any product that may be evaluated in this article, or claim that may be made by its manufacturer, is not guaranteed or endorsed by the publisher.
Thank you Geert Vanwesenbeeck (Jan De Nul NV) and Baecke B.V. for the logistical support in excavating the monoliths and placing them safely in the Mesodrome flume facility. We thank Lennart Van IJzerloo for performing the biomechanical measurements at NIOZ, Yerseke. Thank you to all volunteers and especially Heleen Keirsebelik, Ignace Pelckmans and Dimitri Van Pelt for the logistical support by preparing the flume set-up.
The Supplementary Material for this article can be found online at: https://www.frontiersin.org/articles/10.3389/fmars.2022.920480/full#supplementary-material
Ameen A. D., Kolker A. S., Taylor C. M. (2017). Vegetation and Shear Strength in a Delta-Splay Mouth Bar. Wetlands 37, 1159–1168. doi: 10.1007/s13157-017-0948-7
Are D., Kemp G. P., Giustina F. D., Day J. W., Scarton F. (2002). A Portable, Electrically-Driven Dutch Cone Penetrometer for Geotechnical Measurements in Soft Estuarine Sediments. J. Coast. Res. 18, 372–378.
Asaeda T., Fujino T., Manatunge J. (2005). Morphological Adaptations of Emergent Plants to Water Flow: A Case Study With Typha Angustifolia, Zizania Latifolia and Phragmites Australis. Freshw. Biol. 50, 1991–2001. doi: 10.1111/j.1365-2427.2005.01445.x
Auerbach L. W., Goodbred S. L., Mondal D. R., Wilson C. A., Ahmed K. R., Roy K., et al. (2015). Flood Risk of Natural and Embanked Landscapes on the Ganges-Brahmaputra Tidal Delta Plain. Nat. Clim. Change 5, 153–157. doi: 10.1038/nclimate2472
Baptist M. J., Dankers P., Cleveringa J., Sittoni L., Willemsen P. W. J. M., Van Puijenbroek M. E. B., et al. (2021). Salt Marsh Construction as a Nature-Based Solution in an Estuarine Social-Ecological System. Nature-Based. Solut. 1, 100005. doi: 10.1016/j.nbsj.2021.100005
Bennett W. G., van Veelen T. J., Fairchild T. P., Griffin J. N., Karunarathna H. (2020). Computational Modelling of the Impacts of Saltmarsh Management Interventions on Hydrodynamics of a Small Macro-Tidal Estuary. J. Mar. Sci. Eng. 8,1–30. doi: 10.3390/JMSE8050373
Bouma T. J., De Vries M. B., Low E., Kusters L., Herman P. M. J., Tánczos I. C., et al. (2005a). Flow Hydrodynamics on a Mudflat and in Salt Marsh Vegetation: Identifying General Relationships for Habitat Characterisations. Hydrobiologia 540, 259–274. doi: 10.1007/s10750-004-7149-0
Bouma T. J., De Vries M. B., Low E., Peralta G., Tánczos I. C., van de Koppel J., et al. (2005b). Trade-Offs Related to Ecosystem Engineering: A Case Study on Stiffness of Emerging Macrophytes. Ecology 86, 2187–2199. doi: 10.1890/04-1588
Bouma T. J., Friedrichs M., Klaassen P., van Wesenbeeck B. K., Brun F. G., Temmerman S., et al. (2009). Effects of Shoot Stiffness, Shoot Size and Current Velocity on Scouring Sediment From Around Seedlings and Propagules. Mar. Ecol. Prog. Ser. 388, 293–297. doi: 10.3354/meps08130
Bouma T. J., van Belzen J., Balke T., Zhu Z., Airoldi L., Blight A. J., et al. (2014). Identifying Knowledge Gaps Hampering Application of Intertidal Habitats in Coastal Protection: Opportunities & Steps to Take. Coast. Eng. 87, 147–157. doi: 10.1016/j.coastaleng.2013.11.014
Bradley P., Morris J. (1990). Physical Characteristics of Salt Marsh Sediments: Ecological Implications. Mar. Ecol. Prog. Ser. 61, 245–252. doi: 10.3354/meps061245
Brain M. J., Long A. J., Woodroffe S. A., Petley D. N., Milledge D. G., Parnell A. C. (2012). Modelling the Effects of Sediment Compaction on Salt Marsh Reconstructions of Recent Sea-Level Rise. Earth Planet. Sci. Lett. 345–348, 180–193. doi: 10.1016/j.epsl.2012.06.045
Brooks H., Möller I., Carr S., Chirol C., Christie E., Evans B., et al. (2021). Resistance of Salt Marsh Substrates to Near-Instantaneous Hydrodynamic Forcing. Earth Surf. Process. Landforms. 88, 67–88. doi: 10.1002/esp.4912
Cahoon D. R., McKee K. L., Morris J. T. (2020). How Plants Influence Resilience of Salt Marsh and Mangrove Wetlands to Sea-Level Rise. Estuaries Coasts. 44, 883–898. doi: 10.1007/s12237-020-00834-w
Carus J., Paul M., Schröder B. (2016). Vegetation as Self-Adaptive Coastal Protection: Reduction of Current Velocity and Morphologic Plasticity of a Brackish Marsh Pioneer. Ecol. Evol. 6, 1579–1589. doi: 10.1002/ece3.1904
Chambers L. G., Steinmuller H. E., Breithaupt J. L. (2019). Toward a Mechanistic Understanding of “Peat Collapse” and its Potential Contribution to Coastal Wetland Loss. Ecology 100, 1–15. doi: 10.1002/ecy.2720
Chen Y., Thompson C. E. L., Collins M. B. (2012). Saltmarsh Creek Bank Stability: Biostabilisation and Consolidation With Depth. Cont. Shelf Res. 35, 64–74. doi: 10.1016/j.csr.2011.12.009
Cheong S.-M., Silliman B., Wong P. P., van Wesenbeeck B., Kim C.-K., Guannel G. (2013). Coastal Adaptation With Ecological Engineering. Nat. Clim. Change 3, 787–791. doi: 10.1038/nclimate1854
Chirol C., Spencer K. L., Carr S. J., Möller I., Evans B., Lynch J., et al. (2021). Effect of Vegetation Cover and Sediment Type on 3D Subsurface Structure and Shear Strength in Saltmarshes. Earth Surf. Process. Landforms. 46, 2279–2297. doi: 10.1002/esp.5174
Christiansen T., Wiberg P. L., Milligan T. G. (2000). Flow and Sediment Transport on a Tidal Salt Marsh. Estuaries Coasts 50, 315–331. doi: 10.1006/ecss.2000.0548
Coops H., Van der Velde G., Van der G., (1996). Effects of waves on helophyte stands : mechanical characteristics of stems of Phragmites australis and Scirpus lacustris. Aquat. Bot. 53, 175–185. 10.1016/0304-3770(96)01026-1
Crooks S., Pye K. (2000). Sedimentological Controls on the Erosion and Morphology of Saltmarshes: Implications for Flood Defense and Habitat Recreation. Geol. Soc Spec. Publ. 175, 207–222. doi: 10.1144/GSL.SP.2000.175.01.16
Danka J., Zhang L. (2015). Dike Failure Mechanisms and Breaching Parameters. J. Geotech. Geoenvironmental. Eng. 141, 841–846. doi: 10.1061/(ASCE)GT.1943-5606.0001335
Dao T., Stive M. J. F., Hofland B., Mai T. (2018). Wave Damping Due to Wooden Fences Along Mangrove Coasts. J. Coast. Res. 34, 1317. doi: 10.2112/jcoastres-d-18-00015.1
Day J. W., Boesch D. F., Clairain E. J., Kemp G. P., Laska S. D., Mitsch W. J., et al. (2007). Restoration of the Mississippi Delta: Lessons From Hurricanes Katrina and Rita. Science 80,1679–1684. doi: 10.1126/science.1137030
De Battisti D., Fowler M. S., Jenkins S. R., Skov M. W., Rossi M., Bouma T. J., et al. (2019). Intraspecific Root Trait Variability Along Environmental Gradients Affects Salt Marsh Resistance to Lateral Erosion. Front. Ecol. Evol. 7. doi: 10.3389/fevo.2019.00150
De Smit J. C., Kleinhans M. G., Gerkema T., Bouma T. J. (2021). Quantifying Natural Sediment Erodibility Using a Mobile Oscillatory. Estuar. Coast. Shelf Sci. 262, 107574. doi: 10.1016/j.ecss.2021.107574
Esteves L. S., Williams J. J. (2017). Managed Realignment in Europe: A Synthesis of Methods, Achievements and Challenges. Living Shorelines. Sci. Manage. Nature-based. Coast. Prot., 157–182.
Evans B. R., Brooks H., Chirol C., Kirkham M. K., Möller I., Royse K., et al. (2021). Vegetation Interactions With Geotechnical Properties and Erodibility of Salt Marsh Sediments. Estuar. Coast. Shelf Sci. 265, 107713. doi: 10.1016/j.ecss.2021.107713
Feagin R. a, Lozada-Bernard S. M., Ravens T. M., Möller I., Yeager K. M., Baird A. H. (2009). Does Vegetation Prevent Wave Erosion of Salt Marsh Edges? Proc. Natl. Acad. Sci. U. S. A. 106, 10109–10113. doi: 10.1073/pnas.0901297106
Fearnley S. (2008). The Soil Physical and Chemical Properties of Restored and Natural Back-Barrier Salt Marsh on Isles Dernieres, Louisiana. J. Coast. Res. 24, 84–94. doi: 10.2112/05-0620.1
Gillen M. N., Messerschmidt T. C., Kirwan M. L. (2021). Biophysical Controls of Marsh Soil Shear Strength Along an Estuarine Salinity Gradient. Earth Surf. Dyn. 9, 413–421. doi: 10.5194/esurf-9-413-2021
Grabowski R. C., Droppo I. G., Wharton G. (2011). Erodibility of Cohesive Sediment: The Importance of Sediment Properties. Earth-Science. Rev. 105, 101–120. doi: 10.1016/j.earscirev.2011.01.008
Gyssels G., Poesen J., Bochet E., Li Y. (2005). Impact of Plant Roots on the Resistance of Soils to Erosion by Water: A Review. Prog. Phys. Geogr. 29, 189–217. doi: 10.1191/0309133305pp443ra
Hallegatte S., Green C., Nicholls R. J., Corfee-Morlot J. (2013). Future Flood Losses in Major Coastal Cities. Nat. Clim. Change 3, 802–806. doi: 10.1038/nclimate1979
Hansen F., Kruschke T., Greatbatch R. J., Weisheimer A. (2019). Factors Influencing the Seasonal Predictability of Northern Hemisphere Severe Winter Storms. Geophys. Res. Lett. 46, 365–373. doi: 10.1029/2018GL079415
Heiri O., Lotter A. F., Lemcke G. (2001). Loss on Ignition as a Method for Estimating Organic and Carbonate Content in Sediments: Reproducibility and Comparability of Results. J. Paleolimnol. 25, 101–110. doi: 10.1016/0009-2541(93)90140-E
Himmelstein J., Vinent O. D., Temmerman S., Kirwan M. L. (2021). Mechanisms of Pond Expansion in a Rapidly Submerging Marsh. Front. Mar. Sci. 8. doi: 10.3389/fmars.2021.704768
Hinkel J., Lincke D., Vafeidis A. T., Perrette M., Nicholls R. J., Tol R. S. J. J., et al. (2014). Coastal Flood Damage and Adaptation Costs Under 21st Century Sea-Level Rise. Proc. Natl. Acad. Sci. U. S. A. 111, 3292–3297. doi: 10.1073/pnas.1222469111
Hofstede J. L. A. (2003). Integrated Management of Artificially Created Salt Marshes in the Wadden Sea of Schleswig-Holstein, Germany. Wetl. Ecol. Manage. 11, 183–194. doi: 10.1023/A:1024248127037
Howes N. C., FitzGerald D. M., Hughes Z. J., Georgiou I. Y., Kulp M. A., Miner M. D., et al. (2010). Hurricane-Induced Failure of Low Salinity Wetlands. Proc. Natl. Acad. Sci. U. S. A. 107, 14014–14019. doi: 10.1073/pnas.0914582107
Hu Z., Borsje B. W., Belzen J., Willemsen P. W. J. M. (2021). Mechanistic Modeling of Marsh Seedling Establishment Provides a Positive Outlook for Coastal Wetland Restoration Under Global Climate Change Geophysical Research Letters. Geophys. Res. Lett. 48, 1–12. doi: 10.1029/2021GL095596
Jafari N. H., Harris B. D., Cadigan J. A., Day J. W., Sasser C. E., Kemp G. P., et al. (2019). Wetland Shear Strength With Emphasis on the Impact of Nutrients, Sediments, and Sea Level Rise. Estuar. Coast. Shelf Sci. 229, 1–27. doi: 10.1016/j.ecss.2019.106394
Jiang L., Gerkema T., Idier D., Slangen A. B. A., Soetaert K. (2020). Effects of Sea-Level Rise on Tides and Sediment Dynamics in a Dutch Tidal Bay. Ocean. Sci. 16, 307–321. doi: 10.5194/os-16-307-2020
Kabat P., Fresco L. O., Stive M. J. F., Veerman C. P., Van Alphen J. S. L. J., Parmet B. W. A. H., et al. (2009). Dutch Coasts in Transition. Nat. Geosci. 2, 450–452. doi: 10.1038/ngeo572
Kadiri M., Spencer K. L., Heppell C. M., Fletcher P. (2011). Sediment Characteristics of a Restored Saltmarsh and Mudflat in a Managed Realignment Scheme in Southeast England. Hydrobiologia 672, 79–89. doi: 10.1007/s10750-011-0755-8
Kirwan M. L., Guntenspergen G. R. (2012). Feedbacks Between Inundation , Root Production , and Shoot Growth in a Rapidly Submerging Brackish Marsh. J. Ecol. 100, 764–770. doi: 10.1111/j.1365-2745.2012.01957.x
Lo V. B., Bouma T. J., van Belzen J., Van Colen C., Airoldi L. (2017). Interactive Effects of Vegetation and Sediment Properties on Erosion of Salt Marshes in the Northern Adriatic Sea. Mar. Environ. Res. 131, 32–42. doi: 10.1016/j.marenvres.2017.09.006
Martín-Antón M., Negro V., Del Campo J. M., López-Gutiérrez J. S., Esteban M. D. (2016). Review of Coastal Land Reclamation Situation in the World. J. Coast. Res. 1, 667–671. doi: 10.2112/SI75-133.1
Masselink G., Scott T., Poate T., Russell P., Davidson M., Conley D. (2016). The Extreme 2013/2014 Winter Storms: Hydrodynamic Forcing and Coastal Response Along the Southwest Coast of England. Earth Surf. Process. Landforms. 41, 378–391. doi: 10.1002/esp.3836
McEvoy S., Haasnoot M., Biesbroek R. (2021). How are European Countries Planning for Sea Level Rise? Ocean. Coast. Manage. 203, 105512. doi: 10.1016/j.ocecoaman.2020.105512
Möller I., Bouma T. J., Brendel M., Brooks H., Cao H., Carr S., et al. (2019). “Response of Ecologically-Mediated Shallow Intertidal Shore Transitions to Extreme Hydrodynamic Forcing (RESIST),” in Presentation at Hydralab Symposium(Bucharest), 1–9. Hydralab.eu
Möller I., Kudella M., Rupprecht F., Spencer T., Paul M., van Wesenbeeck B. K., et al. (2014). Wave Attenuation Over Coastal Salt Marshes Under Storm Surge Conditions. Nat. Geosci. 7, 727–731. doi: 10.1038/ngeo2251
Morris R. L., Boxshall A., Swearer S. E. (2020). Climate-Resilient Coasts Require Diverse Defence Solutions. Nat. Clim. Change 10, 485–487. doi: 10.1038/s41558-020-0798-9
Morris M., Hassan M., Kortenhaus A., Visser P. (2009). Breaching Processes: A State of the Art Review (HR Wallingford, UK: FLOODsite Publ), 70.
Neumann B., Vafeidis A. T., Zimmermann J., Nicholls R. J. (2015). Future Coastal Population Growth and Exposure to Sea-Level Rise and Coastal Flooding - A Global Assessment. PLoS One 10, 1–34. doi: 10.1371/journal.pone.0118571
Nicholls R. J., Lincke D., Hinkel J., Brown S., Vafeidis A. T., Meyssignac B., et al. (2021). A Global Analysis of Subsidence, Relative Sea-Level Change and Coastal Flood Exposure. Nat. Climate Change. 11, 338–342. doi: 10.1038/s41558-021-00993-z
Nolte S., Koppenaal E. C., Esselink P., Dijkema K. S., Schuerch M., De Groot A. V., et al. (2013). Measuring Sedimentation in Tidal Marshes: A Review on Methods and Their Applicability in Biogeomorphological Studies. J. Coast. Conserv. 17, 301–325. doi: 10.1007/s11852-013-0238-3
Oosterlee L., Cox T. J. S., Vandenbruwaene W., Maris T., Temmerman S., Meire P. (2018). Tidal Marsh Restoration Design Affects Feedbacks Between Inundation and Elevation Change. Estuaries Coasts 41, 613–625. doi: 10.1007/s12237-017-0314-2
Paprotny D., Sebastian A., Morales-Nápoles O., Jonkman S. N. (2018). Trends in Flood Losses in Europe Over the Past 150 Years. Nat. Commun. 9, 1–12. doi: 10.1038/s41467-018-04253-1
Peeters P., Visser K. P., Mostaert F. (2019). “Large-Scale Dike Breach Experiments in Belgium,” in Data Report Wijmeers & Outlook Future Experiments. Version 3.0. FHR Reports, 00_089_3(Antwerp, Belgium: Flanders Hydraulics Research).
Peeters P., Zhao G., De Vos L., Visser P. J. (2015). Large-Scale Dike Breaching Experiments at Lillo in Belgium. Scour. Eros. - Proc. 7th Int. Conf. Scour. Erosion. ICSE. 2014, 289–297. doi: 10.1201/b17703-36
Pennings S. C., Glazner R. M., Hughes Z. J., Kominoski J. S., Armitage A. R. (2021). Effects of Mangrove Cover on Coastal Erosion During a Hurricane in Texas, USA. Ecology 102, 1–8. doi: 10.1002/ecy.3309
Schoonees T., Gijón Mancheño A., Scheres B., Bouma T. J., Silva R., Schlurmann T., et al. (2019). Hard Structures for Coastal Protection, Towards Greener Designs. Estuaries Coasts 42, 1709–1729. doi: 10.1007/s12237-019-00551-z
Schoutens K., Heuner M., Fuchs E., Minden V., Schulte-Ostermann T., Belliard J. P., et al. (2020). Nature-Based Shoreline Protection by Tidal Marsh Plants Depends on Trade-Offs Between Avoidance and Attenuation of Hydrodynamic Forces. Estuar. Coast. Shelf Sci. 236, 11. doi: 10.1016/j.ecss.2020.106645
Schoutens K., Heuner M., Minden V., Schulte Ostermann T., Silinski A., Belliard J.-P., et al. (2019). How Effective are Tidal Marshes as Nature-Based Shoreline Protection Throughout Seasons? Limnol. Oceanogr. 64, 1750–1762. doi: 10.1002/lno.11149
Schoutens K., Reents S., Nolte S., Evans B., Paul M., Kudella M., et al. (2021). Survival of the Thickest ? Impacts of Extreme Wave-Forcing on Marsh Seedlings are Mediated by Species Morphology. Limnol. Oceanogr., 66, 1–16. doi: 10.1002/lno.11850
Schuerch M., Dolch T., Bisgwa J., Vafeidis A. T. (2018). Changing Sediment Dynamics of a Mature Backbarrier Salt Marsh in Response to Sea-Level Rise and Storm Events. Front. Mar. Sci. 5. doi: 10.3389/fmars.2018.00155
Schulte Ostermann T., Kleyer M., Heuner M., Fuchs E., Temmerman S., Schoutens K., et al. (2021). Hydrodynamics Affect Plant Traits in Estuarine Ecotones With Impact on Carbon Sequestration Potentials. Estuar. Coast. Shelf Sci. 259, 107464. doi: 10.1016/j.ecss.2021.107464
Schulze D., Rupprecht F., Nolte S., Jensen K. (2019). Seasonal and Spatial Within − Marsh Differences of Biophysical Plant Properties : Implications for Wave Attenuation Capacity of Salt Marshes. Aquat. Sci. 81, 1–11. doi: 10.1007/s00027-019-0660-1
Sengupta D., Chen R., Meadows M. E. (2018). Building Beyond Land: An Overview of Coastal Land Reclamation in 16 Global Megacities. Appl. Geogr. 90, 229–238. doi: 10.1016/j.apgeog.2017.12.015
Sha X., Xu K., Bentley S. J., Robichaux P. A. (2018). Characterization and Modeling of Sediment Settling, Consolidation, and Suspension to Optimize Coastal Louisiana Restoration. Estuar. Coast. Shelf Sci. 203, 137–147. doi: 10.1016/j.ecss.2018.02.008
Sheng Y. P., Rivera-Nieves A. A., Zou R., Paramygin V. A., Angelini C., Sharp S. J. (2021). Invasive Phragmites Provides Superior Wave and Surge Damage Protection Relative to Native Plants During Storms. Environ. Res. Lett. 16: 1–12. doi: 10.1088/1748-9326/abf288
Shepard C. C., Crain C. M., Beck M. W. (2011). The Protective Role of Coastal Marshes: A Systematic Review and Meta-Analysis. PLoS One 6, 1–11. doi: 10.1371/journal.pone.0027374
Silinski A., Fransen E., Bouma T. J., Meire P., Temmerman S. (2016a). Unravelling the Controls of Lateral Expansion and Elevation Change of Pioneer Tidal Marshes. Geomorphology 274, 106–115. doi: 10.1016/j.geomorph.2016.09.006
Silinski A., Heuner M., Troch P., Puijalon S., Bouma T. J., Schoelynck J., et al. (2016b). Effects of Contrasting Wave Conditions on Scour and Drag on Pioneer Tidal Marsh Plants. Geomorphology 255, 49–62. doi: 10.1016/j.geomorph.2015.11.021
Smith C. S., Rudd M. E., Gittman R. K., Melvin E. C., Patterson V. S., Renzi J. J., et al. (2020). Coming to Terms With Living Shorelines: A Scoping Review of Novel Restoration Strategies for Shoreline Protection. Front. Mar. Sci. 7. doi: 10.3389/fmars.2020.00434
Smolders S., Teles M. J., Leroy A., Maximova T., Meire P., Temmerman S. (2020). Modeling Storm Surge Attenuation by an Integrated Nature-Based and Engineered Flood Defense System in the Scheldt Estuary (Belgium). J. Mar. Sci. Eng. 8, 2–28. doi: 10.3390/jmse8010027
Spencer T., Brooks S. M., Evans B. R., Tempest J. A., Möller I. (2015). Southern North Sea Storm Surge Event of 5 December 2013 : Water Levels, Waves and Coastal Impacts. Earth Sci. Rev. 146, 120–145. doi: 10.1016/j.earscirev.2015.04.002
Spencer T., Möller I., Rupprecht F., Bouma T. J., van Wesenbeeck B. K., Kudella M., et al. (2016). Salt Marsh Surface Survives True-to-Scale Simulated Storm Surges. Earth Surf. Process. Landforms. 41, 543–552. doi: 10.1002/esp.3867
Srivastava J., Kalra S. J. S., Naraian R. (2014). Environmental Perspectives of Phragmites Australis (Cav.) Trin. Ex. Steudel. Appl. Water Sci. 4, 193–202. doi: 10.1007/s13201-013-0142-x
Stanczak G., Oumeraci H. (2012). Modeling Sea Dike Breaching Induced by Breaking Wave Impact-Laboratory Experiments and Computational Model. Coast. Eng. 59, 28–37. doi: 10.1016/j.coastaleng.2011.07.001
Stark J., Van Oyen T., Meire P., Temmerman S. (2015). Observations of Tidal and Storm Surge Attenuation in a Large Tidal Marsh. Limnol. Oceanogr. 60, 1371–1381. doi: 10.1002/lno.10104
Symonds A. M., Collins M. B. (2004). Sediment Dynamics Associated With Managed Realignment; Freiston Shore. Wash. UK. Coast. Eng. 4, 1–13. doi: 10.1142/9789812701916
Tagliapietra D., Baldan D., Barausse A., Buosi A., Curiel D., Guarneri I., et al. (2018). “Protecting and Restoring the Salt Marshes and Seagrasses in the Lagoon of Venice,” in Management and Restoration of Mediterranean Coastal Lagoons in Europe. Càtedra d'Ecosistemes litorals Mediterrànis i LIFE Pletera, Italy
Tan L., Ge Z., Zhou X., Li S., Li X., Tang J. (2020). Conversion of Coastal Wetlands, Riparian Wetlands, and Peatlands Increases Greenhouse Gas Emissions: A Global Meta-Analysis. Glob. Change Biol. 26, 1638–1653. doi: 10.1111/gcb.14933
Temmerman S., Govers G., Wartel S., Meire P. (2003). Spatial and Temporal Factors Controlling Short-Term Sedimentation in a Salt and Freshwater Tidal Marsh, Scheldt Estuary, Belgium, SW Netherlands. Earth Surf. Process. Landforms. 28, 739–755. doi: 10.1002/esp.495
Temmerman S., Govers G., Wartel S., Meire P. (2004). Modelling Estuarine Variations in Tidal Marsh Sedimentation: Response to Changing Sea Level and Suspended Sediment Concentrations. Mar. Geol. 212, 1–19. doi: 10.1016/j.margeo.2004.10.021
Temmerman S., Meire P., Bouma T. J., Herman P. M. J., Ysebaert T., De Vriend H. J. (2013). Ecosystem-Based Coastal Defence in the Face of Global Change. Nature 504, 79–83. doi: 10.1038/nature12859
Temmerman S., Moonen P., Schoelynck J., Govers G., Bouma T. J. (2012). Impact of Vegetation Die-Off on Spatial Flow Patterns Over a Tidal Marsh. Geophys. Res. Lett. 39, 1–5. doi: 10.1029/2011GL050502
Tempest J. A., Harvey G. L., Spencer K. L. (2015). Modified Sediments and Subsurface Hydrology in Natural and Recreated Salt Marshes and Implications for Delivery of Ecosystem Services. Hydrol. Process. 29, 2346–2357. doi: 10.1002/hyp.10368
Tessler Z. D., Vörösmarty C. J., Grossberg M., Gladkova I., Aizenman H., Syvitski J. P. M., et al. (2015). Profiling Risk and Sustainability in Coastal Deltas of the World. Science 80, 638–643. doi: 10.1126/science.aab3574
Teuchies J., Vandenbruwaene W., Carpentier R., Bervoets L., Temmerman S., Wang C., et al. (2013). Estuaries as Filters: The Role of Tidal Marshes in Trace Metal Removal. PLoS One 8, 1–11. doi: 10.1371/journal.pone.0070381
Vandenbruwaene W., Maris T., Cox T. J. S., Cahoon D. R., Meire P., Temmerman S. (2011). Sedimentation and Response to Sea-Level Rise of a Restored Marsh With Reduced Tidal Exchange: Comparison With a Natural Tidal Marsh. Geomorphology 130, 115–126. doi: 10.1016/j.geomorph.2011.03.004
van den Hoven K., Kroeze C., van Loon-Steensma J. M. (2022). Characteristics of Realigned Dikes in Coastal Europe: Overview and Opportunities for Nature-Based Flood Protection. Ocean. Coast. Manage. 222, 106116. doi: 10.1016/j.ocecoaman.2022.106116
van de Vijsel R. C., van Belzen J., Bouma T. J., van der Wal D., Cusseddu V., Purkis S. J., et al. (2020). Estuarine Biofilm Patterns: Modern Analogues for Precambrian Self-Organization. Earth Surf. Process. Landforms. 45, 1141–1154. doi: 10.1002/esp.4783
van Loon-Steensma J. M., Schelfhout H. A. (2017). Wide Green Dikes: A Sustainable Adaptation Option With Benefits for Both Nature and Landscape Values? Land. Use Policy 63, 528–538. doi: 10.1016/j.landusepol.2017.02.002
Van Loon-steensma J. M., Slim P. A. (2013). The Impact of Erosion Protection by Stone Dams on Salt- Marsh Vegetation on Two Wadden Sea Barrier Islands. J. Coast. Res. 29, 783–796. doi: 10.2112/JCOASTRES-D-12-00123.1
Vannoppen W., Vanmaercke M., De Baets S., Poesen J. (2015). A Review of the Mechanical Effects of Plant Roots on Concentrated Flow Erosion Rates. Earth-Science. Rev. 150, 666–678. doi: 10.1016/j.earscirev.2015.08.011
Van Putte N., Temmerman S., Verreydt G., Seuntjens P., Maris T., Heyndrickx M., et al. (2020). Groundwater Dynamics in a Restored Tidal Marsh are Limited by Historical Soil Compaction. Estuar. Coast. Shelf Sci. 244, 106101. doi: 10.1016/j.ecss.2019.02.006
van Wijnen H. J., Bakker J. P. (2001). Long-Term Surface Elevation Change in Salt Marshes: A Prediction of Marsh Response to Future Sea-Level Rise. Estuar. Coast. Shelf Sci. 52, 381–390. doi: 10.1006/ecss.2000.0744
Vorogushyn S., Merz B., Lindenschmidt K. E., Apel H. (2010). A New Methodology for Flood Hazard Assessment Considering Dike Breaches. Water Resour. Res. 46, 1–17. doi: 10.1029/2009WR008475
Vuik V., Borsje B. W., Willemsen P. W. J. M., Jonkman S. N. (2019). Salt Marshes for Flood Risk Reduction: Quantifying Long-Term Effectiveness and Life-Cycle Costs. Ocean. Coast. Manage. 171, 96–110. doi: 10.1016/j.ocecoaman.2019.01.010
Vuik V., Jonkman S. N., Borsje B. W., Suzuki T. (2016). Nature-Based Flood Protection: The Efficiency of Vegetated Foreshores for Reducing Wave Loads on Coastal Dikes. Coast. Eng. 116, 42–56. doi: 10.1016/j.coastaleng.2016.06.001
Vuik V., van Vuren S., Borsje B. W., van Wesenbeeck B. K., Jonkman S. N. (2018). Assessing Safety of Nature-Based Flood Defenses: Dealing With Extremes and Uncertainties. Coast. Eng. 139, 47–64. doi: 10.1016/j.coastaleng.2018.05.002
Wang X., Chen W., Zhang L., Jin D., Lu C. (2010). Estimating the Ecosystem Service Losses From Proposed Land Reclamation Projects: A Case Study in Xiamen. Ecol. Econ. 69, 2549–2556. doi: 10.1016/j.ecolecon.2010.07.031
Watts C. W., Tolhurst T. J., Black K. S., Whitmore A. P. (2003). In Situ Measurements of Erosion Shear Stress and Geotechnical Shear Strength of the Intertidal Sediments of the Experimental Managed Realignment Scheme at Tollesbury, Essex, UK. Estuar. Coast. Shelf Sci. 58, 611–620. doi: 10.1016/S0272-7714(03)00139-2
Wilson C. A., Hughes Z. J., FitzGerald D. M. (2012). The Effects of Crab Bioturbation on Mid-Atlantic Saltmarsh Tidal Creek Extension: Geotechnical and Geochemical Changes. Estuar. Coast. Shelf Sci. 106, 33–44. doi: 10.1016/j.ecss.2012.04.019
Winterwerp J. C., van Kesteren W. G. M., van Prooijen B., Jacobs W. (2012). A Conceptual Framework for Shear Flow–Induced Erosion of Soft Cohesive Sediment Beds. J. Geophys. Res. Ocean. 117, 1–17. doi: 10.1029/2012JC008072
Wu W., Altinakar M., Al-Riffai M., Bergman N., Bradford S., Cao Z., et al. (2011). Earthen Embankment Breaching. J. Hydraul. Eng. 137, 1549–1564. doi: 10.1061/(asce)hy.1943-7900.0000498
Zhang Y., Chen R., Wang Y. (2020). Tendency of Land Reclamation in Coastal Areas of Shanghai From 1998 to 2015. Land. Use Policy 91, 104370. doi: 10.1016/j.landusepol.2019.104370
Zhang W., Ge Z., Li S., Tan L., Zhou K., Li Y., et al. (2022). The Role of Seasonal Vegetation Properties in Determining the Wave Attenuation Capacity of Coastal Marshes : Implications for Building Natural Defenses. Ecol. Eng. 175, 106494. doi: 10.1016/j.ecoleng.2021.106494
Zhu Z., Vuik V., Visser P. J., Soens T., van Wesenbeeck B., van de Koppel J., et al. (2020a). Historic Storms and the Hidden Value of Coastal Wetlands for Nature-Based Flood Defence. Nat. Sustain. 3, 853–862. doi: 10.1038/s41893-020-0556-z
Keywords: sediment stability, flow velocity, flood risk, dike breach, nature-based adaptation
Citation: Schoutens K, Stoorvogel M, van den Berg M, van den Hoven K, Bouma TJ, Aarninkhof S, Herman PMJ, van Loon-Steensma JM, Meire P, Schoelynck J, Peeters P and Temmerman S (2022) Stability of a Tidal Marsh Under Very High Flow Velocities and Implications for Nature-Based Flood Defense. Front. Mar. Sci. 9:920480. doi: 10.3389/fmars.2022.920480
Received: 14 April 2022; Accepted: 03 June 2022;
Published: 28 July 2022.
Edited by:
Borja Gonzalez Reguero, University of California, Santa Cruz, United StatesReviewed by:
Patrick Biber, University of Southern Mississippi, United StatesCopyright © 2022 Schoutens, Stoorvogel, van den Berg, van den Hoven, Bouma, Aarninkhof, Herman, van Loon-Steensma, Meire, Schoelynck, Peeters and Temmerman. This is an open-access article distributed under the terms of the Creative Commons Attribution License (CC BY). The use, distribution or reproduction in other forums is permitted, provided the original author(s) and the copyright owner(s) are credited and that the original publication in this journal is cited, in accordance with accepted academic practice. No use, distribution or reproduction is permitted which does not comply with these terms.
*Correspondence: Ken Schoutens, a2VuLnNjaG91dGVuc0B1YW50d2VycGVuLmJl
Disclaimer: All claims expressed in this article are solely those of the authors and do not necessarily represent those of their affiliated organizations, or those of the publisher, the editors and the reviewers. Any product that may be evaluated in this article or claim that may be made by its manufacturer is not guaranteed or endorsed by the publisher.
Research integrity at Frontiers
Learn more about the work of our research integrity team to safeguard the quality of each article we publish.