- ViStA Lab, Birla Institute of Technology and Science (BITS), Pilani–KK Birla Goa Campus, Goa, India
Our oceans have been under immense stress due to the deposition and accumulation of marine debris, of which 80% are plastics. Of these, microplastics which are small plastic fragments measuring less than five mm, have been a real bane to the marine fauna, especially the ornamental fishes inhabiting coral reef regions. The multibillion-dollar marine ornamental fish trade depends on these fishes caught from coral reefs. It has often been found that these small fishes are severely affected due to the choking of their gills with microplastics as they are natural filter feeders. To curb the load of plastics in the oceans, and especially in the waters around coral reefs, this study aims to develop a small-scale solution, which could later be scaled up by increasing the size and number of each unit as required. The system has been inspired by the filter-feeding mechanism of manta rays as a basic model for the filtration module. The use of a bacteria named Ideonella sakaiensis, which has been known to decompose plastics inherently is the second level of ideation that has gone into the design. The whole system has been envisaged as a floating system, where the filtration units would be submerged under water and the design of the platform over water would have a provision for self-sustenance, apart from the obvious role in maintaining buoyancy. Each filtration unit has been designed to possess multiple layers of sieving, vortexing and cross-flow filtration with a batch of I. sakaiensis at the end of the unit. To maintain a unidirectional flow of water, a fan would be placed at the end of these filtration units. This system can be a solution to the accumulation of plastics in a localized environment and can be scaled up in terms of size and the number of units to cover a greater area and volume to reduce the menace of plastic pollution. This could be a unique and cost-effective answer to the loss of marine fauna, more specifically ornamental fishes to the curse of microplastics.
Introduction
Microplastics in the Ocean and Their Filtration
The ocean is home to several species of aquatic plants and animals where the dynamic nature of the ocean currents creates a balance which is essential for the survival of the vast abundance of species in the oceans. Ocean flora and fauna have adapted to this dynamic ecosystem courtesy different adaptations and correlations. However, the accumulation of plastic contaminants of various sizes have caused a significant disruption in this ecosystem (O’Brine and Thompson, 2010; Lusher, 2015). Plastic particles with sizes smaller than five millimetres, generally referred to as microplastics (Cauwemberghe et al., 2013) are one of the major contaminants in oceans. These tiny plastic granules are a run-off of several products ranging from cleansers and cosmetics to paint sources (Browne, 2015). The problem with these microplastics is that they are persistent and non-biodegradable – implying a long life in the aquatic environment once they have entered into it (Nelms et al., 2018). The result of this has been the accumulation of microplastics in alarming concentrations in almost all water bodies (Barnes et al., 2009; McCormick et al., 2016; Napper et al., 2021).
Microplastics also attract and adsorb toxins (such as DDT) from the surrounding marine environment, making their threat potentially hazardous to animals at a higher trophic level in the food chain. On account of their size, these plastics are available for intake to a vast spectrum of aquatic organisms, and ultimately remain as persistent deposits in cells and tissues of these organisms (Farrell and Nelson, 2013). The potential threat of being passed on to higher organisms becomes even more evident with the recent discoveries of microplastics in human blood (Leslie et al., 2022). In the case of fishes, a commercially significant activity ranging to the scale of billions of dollars, there has been multiple reports of bio-accumulation wherein inhibition of acetylcholinesterase activity, reduced activity in the form of decreased swimming and predatory performances, changes in endocrine biochemistry and histological changes in gills have been reported as some of the effects (Karami et al., 2016; Choi et al., 2018; Wen et al., 2018).
Filtration Mechanism in Manta Rays
In the quest for an effective solution to the microplastics problem, it was natural to look into the vast repository of the ocean and derive inspiration from the same. An exhaustive search into the filter feeding organisms and their mechanisms drew attention to the case of the manta rays, which are classic examples of filter feeders. These elasmobranchs can reach upto 23 feet in width and support their big mass by feeding on small zooplankton, microcrustaceans, and mesoplankton (50 to 500 μm). Manta rays swim with open mouths, capturing their food and expelling seawater through the gill slits (Paig-Tran et al., 2011).
The efficient filtration mechanism consists of long, parallel arrays of leaf-like filter lobes, akin to an innovative gill raker (Paig-Tran et al., 2013). Water carrying the food particles flows in through the mouth, is directed over the filters, to be expelled through the filter pores. The flow of water over the lobes is such that it hits the filters both in the forward and reverse directions – this is largely due to the orientation of the filter lobes within the cavity. The solid particles encountering the filter lobes ricochet away and flow separation happens behind the leading edge of each filter lobe, causing a vortex within each pore. Water can pass through the filter lobes with little resistance, but the small particles that encounter the swirling eddies are unable to turn that sharply. This filter-feeding apparatus of the manta ray enables it to capture tiny particles of food smaller than its filter pores and ricochet the particles back into the ray’s buccal cavity (Divi et al., 2018).
This process of filter feeding, described as a ricochet separation mechanism, can be a unique solid-fluid separation model which resists clogging as well as adaptability to systems with high flow rates. Instead of forming a film or a coat over the filter, the captured particles are concentrated above making redundant the need for costly and time-consuming cleaning mechanisms. This mechanism could be translated into a model which could capture plastic particles from the water and concentrate them in a chamber that may be ideally designed to be located downstream to the filter. Since the design is also imbibed with the advantage of making interventions redundant, the next step to the process should be the degradation of the accumulated plastic debris.
Ideonella sakaiensis and Plastic Degradation
The search for a biological system which could degrade plastic debris led to the concept of plastic degradation by Ideonella sakaiensis, a Gram negative, aerobic, rod-shaped bacterium belonging to the genus Ideonella and family Comamonadaceae (Yoshida et al., 2021). Originally isolated from a sediment sample taken outside of a plastic bottle recycling facility in Sakai, Japan, I. sakaiensis has piqued the interest of the scientific fraternity due to its unique ability of degrading the plastic polyethylene terephthalate (PET). Interestingly, the bacterium also uses PET as a sole carbon and energy source for growth, thereby making it an excellent choice for growth in environments with high concentration of accumulated plastics. I. sakaiensis grows at a pH range of 5.5 to 9.0 and a temperature of 15–42°C on PET surfaces in a community with other I. sakaiensis cells by adhering to the PET and other cells with thin appendages. As an added advantage, I. sakaiensis was found to have significant halotolerance with regard to the marine salinity (Yoshida et al., 2021).
The enzyme PET Hydrolase or PETase (present in the bacterium) degrades PET into mono(2-hydroxyethyl) terephthalic acid (MHET), a heterodimer composed of terephthalic acid (TPA) and ethylene glycol. The I. sakaiensis PETase functions by hydrolyzing the ester bonds present in PET with high specificity (Supplementary Figure 1). The resulting MHET is then degraded into its two monomeric constituents by a lipid-anchored MHET hydrolase enzyme, or MHETase, on the bacterial cell’s outer membrane.
Of the products, ethylene glycol is readily taken up and used by I. sakaiensis and many other bacteria. On the other hand, terephthalic acid which is more resistant to degradation is imported into the cell via the terephthalic acid transporter protein. Once in the cell, the aromatic terephthalic acid molecule is oxidized by terephthalic acid-1,2-dioxygenase and 1,2-dihydroxy-3,5-cyclohexadiene-1,4-dicarboxylate dehydrogenase into a catechol intermediate. The catechol ring is then cleaved by PCA 3,4-dioxygenase before the compound is integrated into other metabolic pathways (e.g. TCA cycle), thereby leaving no harmful or unsafe products in the ecosystem.
In other words, both molecules derived from the PET are used by the cell to produce energy and to use in its own metabolic processes. Eventually, the assimilated carbon may be mineralized to carbon dioxide and released into the atmosphere. Under experimental conditions, the complete degradation of a test PET film was achieved after six weeks at a temperature of 30°C.
A Design Concept to Address Microplastics Degradation
This article aims to capitalise on the dual premise of filtration of microplastics using a mechanism inspired by the ricochet separation in manta rays and also the further degradation of these trapped microplastics with the aid of I. sakaiensis. The dual mechanism can be used to develop a small-scale solution for alleviating the problem of microplastics being deposited in the ocean. The basic design and workflow of this filtration system has been highlighted in Figure 1.
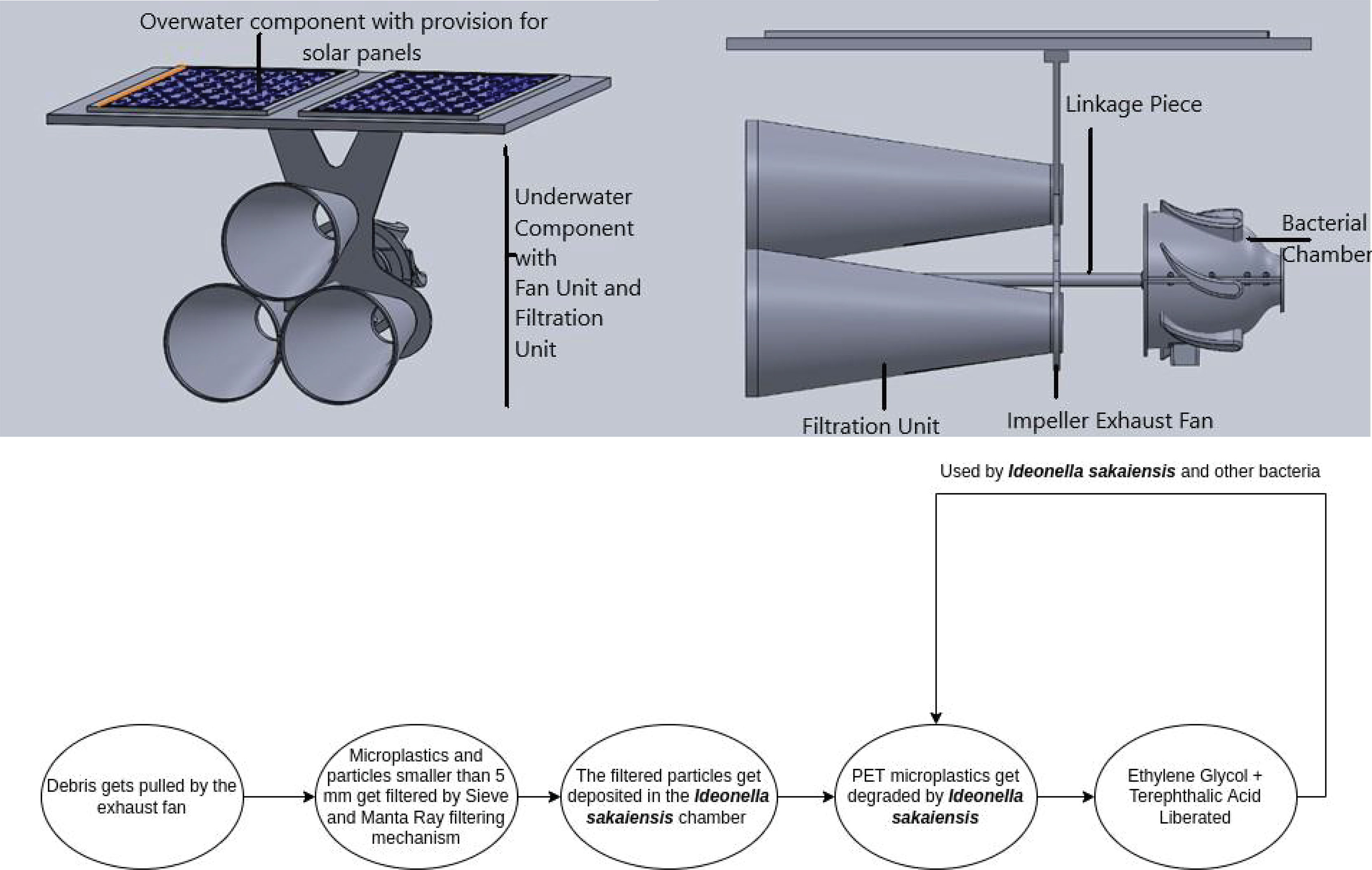
Figure 1 (Top) Model of the filtration unit inspired by the ricochet separation mechanism of manta rays, left: frontal view, right: lateral view (Bottom) Process flow through the filtration unit.
The design of the filtration unit has an underwater component and a component which is meant to be floating over water. The underwater component has two major units – the filtration unit and the fan unit. The major design consideration for the assembly is the buoyancy condition – material, weight and moment balancing is essential. Scalability and deployability are also considered. All components are modelled in Solidworks 2021.
The underwater component consists of a filtration unit (x3), linkage piece, impeller exhaust fan and a bacterial chamber. The conical filtration units are modelled to maximize water entry as well as cross filtration. To increase the rate of plastic filtration and decomposition, 3 such units are stacked and held in a pyramid formation by the linkage piece. This piece connects the filtering unit to the floating component or the surface plate (welded) which houses the power source, viz. the solar panels powering the exhaust fan. The suction and cross filtrational flow is created by this exhaust fan (concept model), covering all 3 filtration units and enabling unidirectional flow through them. A storage battery is conceptualized as well on the surface plate.
Aerodynamic filter fins, inspired and modelled on the filter feeding apparatus of manta rays are arranged in a filter lobe array on the inside and along the length of the conical slope of each filtration unit. These create flow outlets and conditions for bulk of the water and large particles to exit by cross filtration and particle ricocheting (Divi et al., 2018). Microplastic rich water then can enter the Ideonella chamber (posterior end of the filtration units), where the microplastics deposit for filtration and decomposition. To make sure that I. sakaiensis doesn’t end up decomposing the filtration unit, the filtration units are made using fiberglass. This system can be a model for deployment in coral reefs where microplastic pollution has caused considerable harm. The self-sustainability of the system allows for the inexpensive maintenance and life of the same. The dynamic modelling for the filtration unit is underway, and would be soon highlighted for practical purposes.
Data Availability Statement
The original contributions presented in the study are included in the article/Supplementary Material. Further inquiries can be directed to the corresponding author.
Author Contributions
PS contributed to research and ideation. SB was responsible for the analysis and scripting of the manuscript. All authors contributed to the article and approved the submitted version.
Conflict of Interest
The authors declare that the research was conducted in the absence of any commercial or financial relationships that could be construed as a potential conflict of interest.
Publisher’s Note
All claims expressed in this article are solely those of the authors and do not necessarily represent those of their affiliated organizations, or those of the publisher, the editors and the reviewers. Any product that may be evaluated in this article, or claim that may be made by its manufacturer, is not guaranteed or endorsed by the publisher.
Acknowledgments
The authors sincerely acknowledge the help rendered by Suhas Badadal, BITS, Pilani – KK Birla Goa Campus for the CAD designing of the filtration unit and its description.
Supplementary Material
The Supplementary Material for this article can be found online at: https://www.frontiersin.org/articles/10.3389/fmars.2022.919743/full#supplementary-material
References
Barnes D. K., Galgani F., Thompson R. C., Barlaz M. (2009). Accumulation and Fragmentation of Plastic Debris in Global Environments. Philos. T. R. Soc B. 364, 1985–1998. doi: 10.1098/rstb.2008.0205
Browne M. A. (2015). “Sources and Pathways of Microplastic to Habitats,” in Marine Anthropogenic Litter. Eds. Bergmann M., Gutow L., Klages &M. (Berlin: Springer), 229–244. doi: 10.1007/978-3-319-16510-3_9
Cauwemberghe L. V., Vanreusel A., Mees J., Janssen C. R. (2013). Microplastic Pollution in Deep Sea Sediments. Environ. Pollut. 182, 495–499. doi: 10.1016/j.envpol.2013.08.013
Choi J. S., Jung Y.-J., Hong N.-H., Hong S. H., Park J.-W. (2018). Toxicological Effects of Irregularly Shaped and Spherical Microplastics in a Marine Teleost, the Sheepshead Minnow (Cyprinodon Variegatus). Mar. Pollut. Bull. 129, 231–240. doi: 10.1016/j.marpolbul.2018.02.039
Divi R. V., Strother J. A., Paig-Tran E. W. M. (2018). Manta Rays Feed Using Ricochet Separation, a Novel Nonclogging Filtration Mechanism. Sci. Adv. 4, 9533. doi: 10.1126/sciadv.aat9533
Farrell P., Nelson K. (2013). Trophic Level Transfer of Microplastic: Mytilus Edulis (L.) to Carcinus Maenas (L.). Environ. Pollut. 177, 1–3. doi: 10.1016/j.envpol.2013.01.046
Karami A., Romano N., Galloway T., Hamzah H. (2016). Virgin Microplastics Cause Toxicity and Modulate the Impacts of Phenanthrene on Biomarker Responses in African Catfish (Clarias Gariepinus). Environ. Res. 151, 58–70. doi: 10.1016/j.envres.2016.07.024
Leslie H. A., van Velzen M. J. M., Brandsma S. H., Vethaaka D., Garcia-Vallejo J. J., Lamoree M. H. (2022). Discovery and Quantification of Plastic Particle Pollution in Human Blood. Environ. Int. 163, 107199. doi: 10.1016/j.envint.2022.107199
Lusher A. (2015). “Microplastics in the Marine Environment: Distribution, Interactions and Effects,” in Marine Anthropogenic Litter (Cham: Springer), 245–307. doi: 10.1007/978-3-319-16510-3_10
McCormick A. R., Hoellein T. J., London M. G., Hittie J., Scott J. W., Kelly J. J. (2016). Microplastic in Surface Waters of Urban Rivers: Concentration, Sources, and Associated Bacterial Assemblages. Ecosphere 7, e01556. doi: 10.1002/ecs2.1556
Napper I. E, Baroth A., Barrett A. C., Bhola S., Chowdhury G. W., Davies B. F.R., et al. (2021). The Abundance and Characteristics of Microplastics in Surface Water in the Transboundary Ganges River. Environ. Pollut. 274, 116348. doi: 10.1016/j.envpol.2020.116348
Nelms S. E., Galloway T. S., Godley B. J., Jarvis D. S., Lindeque P. K. (2018). Investigating Microplastic Trophic Transfer in Marine Top Predators. Environ. Pollut. 238, 999–1007. doi: 10.1016/j.envpol.2018.02.016
O’Brine T., Thompson R. C. (2010). Degradation of Plastic Carrier Bags in the Marine Environment. Mar. Pollut. Bull. 60, 2279–2283. doi: 10.1016/j.marpolbul.2010.08.005
Paig-Tran E. W. M., Bizzarro J. J., Strother J. A., Summers A. P. (2011). Bottles as Models: Predicting the Effects of Varying Swimming Speed and Morphology on Size Selectivity and Filtering Efficiency in Fishes. J. Exp. Biol. 214, 1643–1654. doi: 10.1242/jeb.048702
Paig-Tran E. W. M., Kleinteich T., Summers A. P. (2013). The Filter Pads and Filtration Mechanisms of the Devil Rays: Variation at Macro and Microscopic Scales. J. Morphol. 274, 1026–1043. doi: 10.1002/jmor.20160
Wen B., Zhang N., Jin S.-R., Chen Z.-Z., Gao J.-Z., Liu Y., et al. (2018). Microplastics Have a More Profound Impact Than Elevated Temperatures on the Predatory Performance, Digestion and Energy Metabolism of an Amazonian Cichlid. Aquat. Toxicol. 195, 67–76. doi: 10.1016/j.aquatox.2017.12.010
Keywords: microplastics, ideonella sakaiensis, manta ray, pollution, biological magnification
Citation: Sankrityayan P and Biswas S (2022) Plastic Filtration and Decomposition According to Ricochet Filtering Mechanism Using Ideonella sakaiensis. Front. Mar. Sci. 9:919743. doi: 10.3389/fmars.2022.919743
Received: 13 April 2022; Accepted: 20 May 2022;
Published: 23 June 2022.
Edited by:
Archana Sinha, Central Inland Fisheries Research Institute (ICAR), IndiaReviewed by:
Gayatri Tripathi, Central Institute of Fisheries Education (ICAR), IndiaCopyright © 2022 Sankrityayan and Biswas. This is an open-access article distributed under the terms of the Creative Commons Attribution License (CC BY). The use, distribution or reproduction in other forums is permitted, provided the original author(s) and the copyright owner(s) are credited and that the original publication in this journal is cited, in accordance with accepted academic practice. No use, distribution or reproduction is permitted which does not comply with these terms.
*Correspondence: Prakhar Sankrityayan, ZjIwMTcwMDQ3QGdvYS5iaXRzLXBpbGFuaS5hYy5pbg==