- 1National Marine Science Centre, Southern Cross University, Coffs Harbour, NSW, Australia
- 2Research Department, Sydney Institute of Marine Science, Mosman NSW, Australia
- 3School of Geosciences, University of Sydney, Sydney, NSW, Australia
The current coral reefs crisis is motivating a number of innovative projects attempting to leverage new mechanisms to avoid coral bleaching, reduce coral mortality and restore damaged reefs. Shading the reef, through seawater atomised fogging, is one tool in development to reduce levels of irradiance and temperature. To evaluate the potential viability of this concept, here we review 91 years (1930–2021) of published research looking at the effects of different levels of shade and light on coral reefs. We summarised the types of studies, places, coral species used, common responses variable measured, and types of shades used among studies. We discuss issues related to reef scale shading applicability, different methods used to measure light, standardisation methods and most importantly the positive and negative effects of shading corals.
Introduction
The effects of shade and light on corals have been studied for at least 91 years (Yonge and Nicholls, 1930; Kawaguti, 1937; Wethey and Porter, 1976). Early on, this research was aimed at understanding coral biology and patterns of species distributions (Coles and Jokiel, 1978; Falkowski and Dubinsky, 1981; Roth et al., 1982; Dinesen, 1983). Later, shading research was used to comprehend the effects of ultraviolet radiation (UVR) on corals due to ozone depletion (Shick et al., 1996; Lesser, 2000). Shading was also used to elucidate different effects of light on coral growth (Rinkevich and Loya, 1984; Rocha et al., 2013b), which resulted in health improvements of corals grown for the aquarium trade (Rocha et al., 2013a). More recently, shading research has also been used to understand coral distribution patterns and physiology among mesophotic and shallow reefs (Tamir et al., 2019; Ben-Zvi et al., 2021; Lesser et al., 2021), and to describe how low light environments may act as refugia for corals against climate change (Kellogg et al., 2020; Stewart et al., 2021).
After the severe mass bleaching of coral around the world in 1998, models predicted such events will increase in frequency and magnitude during the next 20 years (Hoegh-Guldberg, 1999). Those predictions were correct, with mass coral bleaching events being consecutively documented on the Great Barrier Reef during 2001–2002, 2005–2006, 2008–2011, 2016 and 2017 (Australian Institute of Marine Science, 2021) and in the Caribbean during 2005, 2010–2011, 2015 and 2017 (Muñiz-Castillo et al., 2019). The 2016 episode is considered the worst ever recorded (Hughes et al., 2017) and it is confirmed that coral bleaching events are now more frequent, becoming stronger and producing cumulative effects (Grottoli et al., 2014; Hughes et al., 2017; Hughes et al., 2018). Furthermore, global sea surface temperatures are predicted to rise under all emissions scenarios (including the lowest plausible) until at least mid-century (IPCC, 2021), further increasing the frequency and intensity of coral bleaching events. Thus, new scientific approaches and technological solutions are needed to preserve the remaining coral reefs around the world (Hughes et al., 2010). Consequently, a diverse suite of interventions to pause coral reef decline is being evaluated and tested around the world (Bay et al., 2019; Harrison et al., 2019; Condie et al., 2021; Tollefson, 2021).
Shading of corals is included among a list of unconventional conservation strategies to address temperature and carbon dioxide as global stressors of marine ecosystems (Rau et al., 2012). Besides heat stress, the severity of coral bleaching damage is also correlated with light intensity, thus shading corals from direct sunlight can reduce the bleaching effect of elevated ocean temperatures (Hoegh-Guldberg, 1999). Reduced coral bleaching at 10m depth is attributed to lower levels of light rather than lower temperatures (Baird et al., 2018). Regardless of whether the source of shade is natural or artificial, shading reduces light availability on coral reefs (Coles and Jokiel, 1978; Anthony and Hoegh-Guldberg, 2003; Skirving et al., 2017). Shaded corals subject to high temperature stress will, therefore, be less likely to bleach than unshaded corals under the same stress (Baker et al., 2008). Recent research is finding positive effects of reduced light habitats (e.g., corals growing under mangrove shade) (Yates et al., 2014; Kellogg et al., 2020; Stewart et al., 2021) and other shading sources (e.g., also including turbidity, clouds and artificial shades). A number of authors suggest that such natural shading could help conserve corals under climate change scenarios (Cacciapaglia and van Woesik, 2016; Bellworthy and Fine, 2017; Coelho et al., 2017; Gonzalez-Espinosa and Donner, 2021), but the percentage cover of such natural shading only impacts a tiny fraction of coral reefs around the world. However, some other research is investigating and reporting negative effects of reduced light (e.g., turbidity) in coral reefs (Juhi et al., 2021; López-Londoño et al., 2021). Furthermore, thermal coral bleaching in the absence of light and photosynthesis has been also been experimentally demonstrated indicating there are other possible unelucidated bleaching mechanisms (Tolleter et al., 2013).
While there are challenges associated with the scale of coral reefs, the effects of shading on corals are currently being assessed as a tool to mitigate bleaching events. Currently, the shading of coral reefs over large spatial scales seems far-fetched and are yet to be tested (Condie et al., 2021). Recent advances in cloud brightening and artificial fogging technololgy, however, could make shading entire reefs a reality (Harrison et al., 2019). More recently, field trials testing the concepts of fogging, cloud and sky brightening technologies to cool and shade the reef were tested in Australia (Tollefson, 2021). Some promising preliminary results of those trials showed that prototypes using different nozzle technologies (effervescent and impact pin nozzles) can produce sufficient numbers of sub-micron droplets of seawater per second to create a seawater aerosol fog plume with the right light scattering properties to potentially shade sites on coral reefs in low wind conditions (Harrison et al., 2019; Baker et al., 2021).
To help assess the feasibility of large-scale shading endeavours to reduce the risk of coral bleaching, we reviewed empirical studies looking at the influence of shade and light variation on corals. Specifically, we evaluated the question – what is the minimum amount of shade required to protect coral reefs against bleaching events? Additionally, we reviewed commonly measured variable responses that should be considered to demonstrate the effects of shade on corals under climate change scenarios. Overall, this review summarises the available literature to gather evidence to test if shading coral reefs may effectively help reduce coral mortality during mass bleaching events.
Methods
Using the following publication search engines: Google Scholar, ResearchGate, Scopus, and Science Direct, we used different combinations of keywords to find available literature related to the effects of shade and/or different types of irradiance levels (from natural or artificial light) on corals. These keywords included: shade + shading + light + corals + bleaching + climate change + thermal effects + UV radiation + irradiance + visible light + clouds + fogging, etc. We searched and extracted information for around three months and stopped searching on the 18th October 2021. Although our search was comprehensive, we acknowledge that we still may have missed some published works in journals not indexed by the publication search engines as well as information contained in grey literature.
The targeted information extracted from the publications included: type of shade, irradiance spectrum, irradiance levels, irradiance measurement tool, reported irradiance units, type of light (natural or artificial), studied species, place of study (in situ, ex situ), response variables measured, standardisation method, consideration of clouds, country, year published, duration of the study, recovery period and result highlights. Studies looking at the effects of light/shade only on the zooxanthellae or other coral reef organisms (e.g. sea anemones, crustose coralline algae) were not included in our analyses. Studies that included more than one experiment with clear separated results were treated as independent studies in the analyses (e.g. Toller et al., 2001, Coelho et al., 2017; Rosic et al., 2020). For studies that reported very detailed irradiance levels, such as hourly levels among several spectrum ranges (UV, UVB and PAR) and along a depth gradient (e.g. Lesser, 2000), only the maximum value per depth and spectrum was considered in the comparative analysis. Studies with length duration in hours (<1 day, e.g. 6 h, 12 h) were counted as one-day duration.
Overall Description of Studies
In total, we reviewed 143 independent studies/experiments extracted from 124 peer-reviewed articles, one scientific report, one PhD, Master and honour thesis respectively, and two conference proceedings. More than half of the experiments were conducted using coral species from two families,
Pocilloporidae (32%) and Acroporidae (26%). Specifically, most of the experiments used Stylophora pistillata (32%), Pocillopora damicornis (26%), Acropora millepora (11%) and Acropora muricata (8%) (Figure 1). A recent review looking at coral heat-stress experiments found similar results, most coral knowledge is based on the study of three main families (Acroporidae, Pocilloporidae and Poritidae) (McLachlan et al., 2020). Duration of studies varied from less than a day to up to 960 days (median ± SD = 65.5 ± 127 d). Only four studies provided information related to recovery period after bleaching.
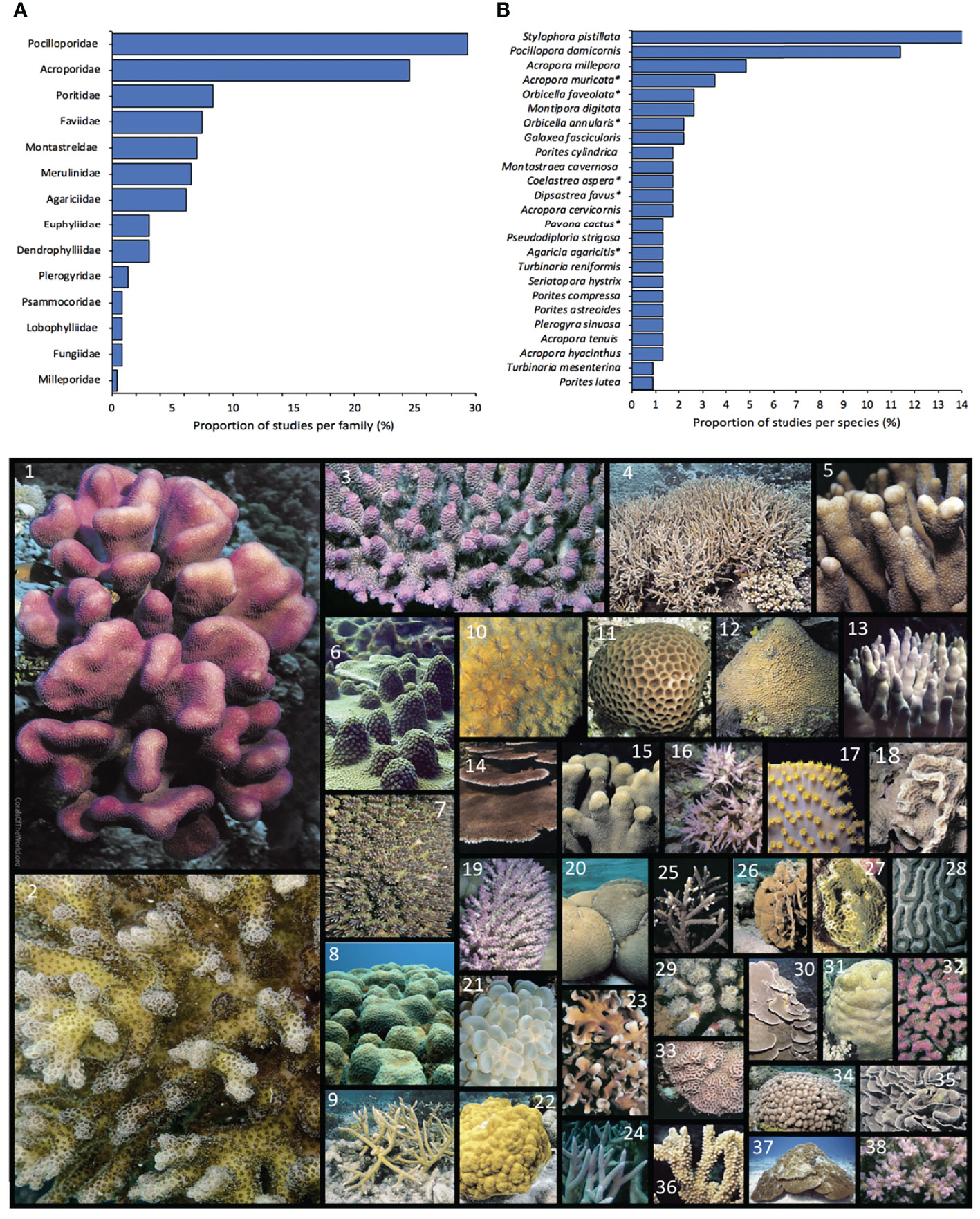
Figure 1 Main coral families (A) and species (B) used on studies looking at the effects of shade and light on corals. Figure below: The dimensions of the coral picture is proportional to the number of studies per species. Species are enumerated from most to least studied respectively. 1: Stylophora pistillata, picture: J. Maragos; 2: Pocillopora damicornis, pic: R. Kelley; 3: Acropora millepora, pic: J. Veron; 4: Acropora muricata*, pic: J. Veron; 5: Montipora digitata, pic: J. Veron; 6: Orbicella faveolata*, pic: E. Weil; 7: Galaxea fascicularis, pic: J. Veron; 8: Orbicella annularis*, pic: E. Weil; 9: Acropora cervicornis, pic: J. Veron; 10: Dipsastrea favus*, pic: J. Veron; 11: Coelastrea aspera*, pic: G Paulay; 12: Monstastraea cavernosa, pic: J. Veron; 13: Porites cylindrica, pic: E. Lovell; 14: Acropora hyacinthus, pic: E Turak; 15: Porites compressa, pic: C. Hunter; 16: Seriatopora hystrix, pic: J. Veron; 17: Turbinaria reniformis, pic: J. Veron; 18: Agaricia agaricites*, pic: J. Veron; 19: Acropora tenuis, pic: J. Veron; 20: Pseudodiploria strigosa*, pic: J. Veron; 21: Plerogyra sinuososa, pic: J. Veron; 22: Porites astreoides, pic: J. Veron; 23: Pavona cactus*, pic: J. Veron; 24: Acropora intermedia, pic: E Turak; 25: Acropora pulchra, pic: J. Veron; 26: Agaricia tenuifolia, pic: J. Veron; 27: Agaricia humilis*, pic: J. Veron; 28: Colpophyllia natans, pic: J. Veron; 29: Fimbriaphyllia paradivisa*, pic: J. Veron; 30: Montipora monasteriata, pic: J Veron; 31: Platygira sinensis, pic: G Allen; 32: Pocillopora verrucosa, pic: J. Veron; 33: Goniastrea pectinata, pic; E. Lovell; 34: Porites furcata, pic: J. Veron; 35: Turbinaria mesenterina, pic: J. Veron; 36: Montipora capitata, pic: J. Veron; 38: Pocillopora acuta*, pic: J. Veron. All pictures were downloaded and slightly modified from Corals of the World Veron et al., 2021. *updated species name based on World Register of Marine Species it might differ with analised studies. Species representing <0.5% of studies were not included.
Most studies were carried out in the field (in situ, 36%), followed by ex situ (laboratory, aquaria and mesocosm) experiments under sunlight (33%), with artificial light (25%), with sun and artificial light (2.5%), with non-specified type of light (2.8%) and a mix of in situ/ex situ (0.7%). More than half of the studies (53%) were undertaken in Australia, Israel, Hawaii and Monaco (Figure 2). Studies ranged from 1930 until 2021, with an overall increasing tendency and at least one study every year since 2000. There were peaks in the number of studies following years with major coral bleaching events (except in 2008 and 2018) (Figure 2).
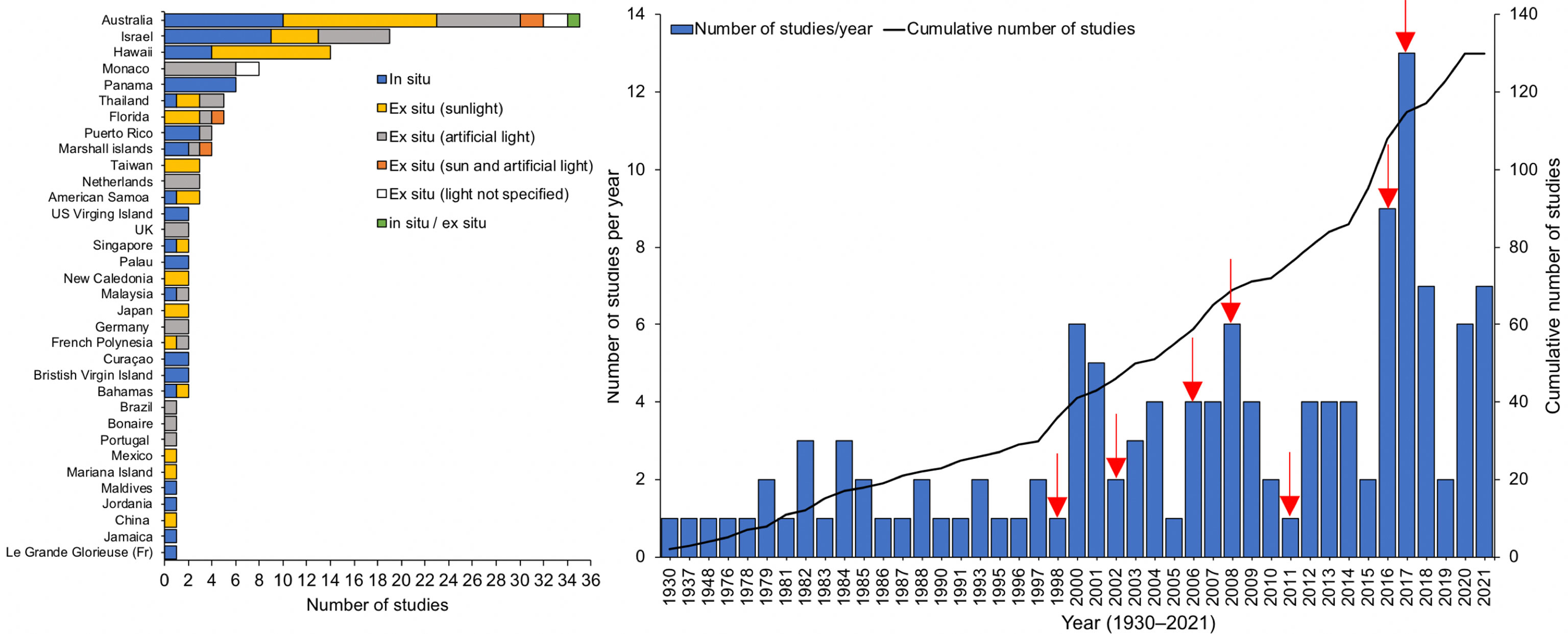
Figure 2 Left: Studies per country, referring to the place (reef or laboratory) and source of light used (natural or artificial). Right: Number of studies and cumulative number of studies looking at the effects of shade or light on corals, red arrows highlight years where massive bleaching events occurred.
Irradiance Spectrum and Irradiance Levels Across Studies
This review revealed a large variety of factors that directly affect irradiance measurements and may jeopardise accurate normalisation for comparison amongst studies. However, we recognise that some of the differences might be attributed to disparities with funding, access to different technologies among regions and detailed project scope. For example, at least 20 different apparatus were used to measure irradiance, with different principles of operation, calibration, and measurement interval (i.e. every 5, 10, 15, 30 or 60 min respectively, once a week, only at noon, during 12h, 24h or during the whole experimental period). Similarly, we identified 33 different wavelength ranges selected from the irradiance spectrum; wide variety of shade materials (e.g. polyvinyl chloride, polyethylene, acrylic, cotton fabric, plastic), filter characteristics (e.g. 8%, 20%, 40%, 65%, 98% UV or PAR blockage), artificial lights (e.g. metal halide, LED, fluorescent lamps), natural light (sunlight, caves, under corals, under mangroves, under other natural shades), depth (above and below surface, and from the surface to 50m), as well as more than 20 different units used to report the level of irradiance (e.g. μmol photons m-2 s-1, μmol m-2 s-1, μmol quanta m-2 s-1, W m-2, E m-2 h-1, g cal cm-2, kJm-2d-1, lux, Js-1m-2nm-1, micro Einstein m-2s-1) (Table 1). This situation has been previously reported in a global meta-analysis of calcification on coral reefs, in which light/PAR data was excluded due to the variety of measurement methods and reporting units (Davis et al., 2021). A similar example can be found in horticultural science, where quantum flux is recommended as the most appropriate means to report radiation, but differences among instrument calibration, reporting units, growers recommendations and engineering specification are an issue when comparing among studies (Thimijan and Heins, 1983; Sipos et al., 2020).
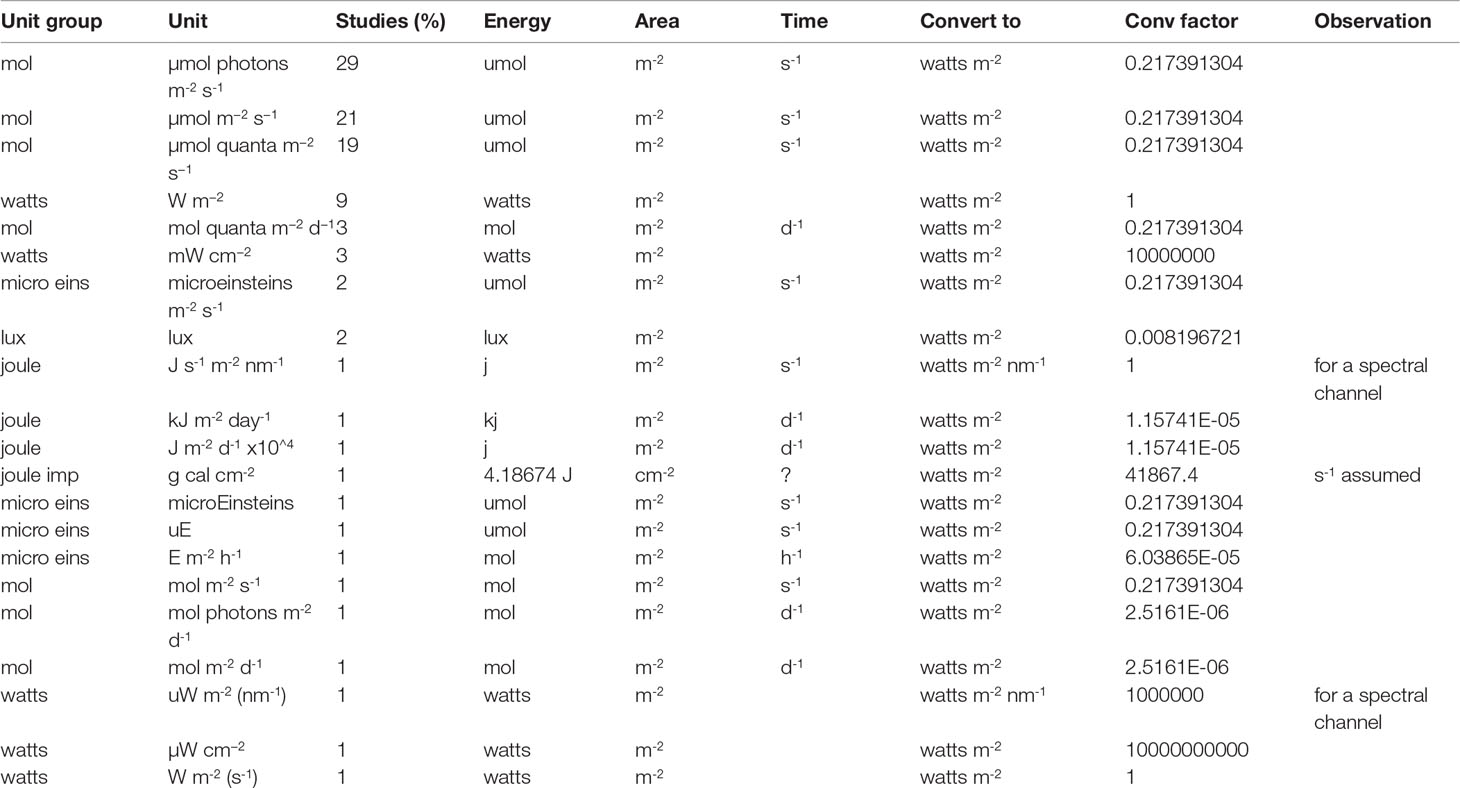
Table 1 Different irradiance units observed among reviewed studies, with a calculated conversion factor to transform all units to preferred watts m-2.
The level of detail when reporting irradiance varied substantially among publications. While some studies provided very detailed irradiance levels at several depths (e.g. Gleason and Wellington, 1995; Lesser, 2000; Anthony et al., 2004), others did not provide any sort of irradiance information, simply using categories such as high light versus low light, sunlight vs shade, shallow vs deep (e.g. Morse et al., 1988; Titlyanov et al., 2001; Fabricius et al., 2004). Most studies (41%) measured the irradiance levels on the visible light spectrum (PAR, 400–700 nm). However, a quarter of the analysed studies failed to report irradiance spectrum, yet half of those studies stated some sort of irradiance levels (PAR spectrum was assumed in those cases) (Figure 3). Furthermore, 8% of the studies report light or shade levels as a percentage of the ambient irradiance but did not report that ambient irradiance level.As early as 60 years ago scientists recognised that cloud cover affects coral reefs (Odum and Odum, 1955). Cloud cover significantly reduces the availability of light, making light measurements non comparable between sunny and cloudy days (Brakel, 1979). To illustrate the strong effects of clouds and their relevance when measuring light levels, Roth et al. (1982) suggested that when determining light intensity, cloud cover was more important than water depth. The best example available to demonstrate the effect of clouds on corals reefs is the cloudy weather during the summer of 1998, which significantly reduced the amount of sun hours, UVR and visible light over the reefs of the Society Islands (French Polynesia), which perhaps contributed to avoidance of a predicted massive bleaching event (based on SST modelling) (Mumby et al., 2001). Despite the importance of cloud cover, the current review revealed that only 38% of 102 studies that utilised natural light considered the presence of cloud cover when measuring the irradiance levels.
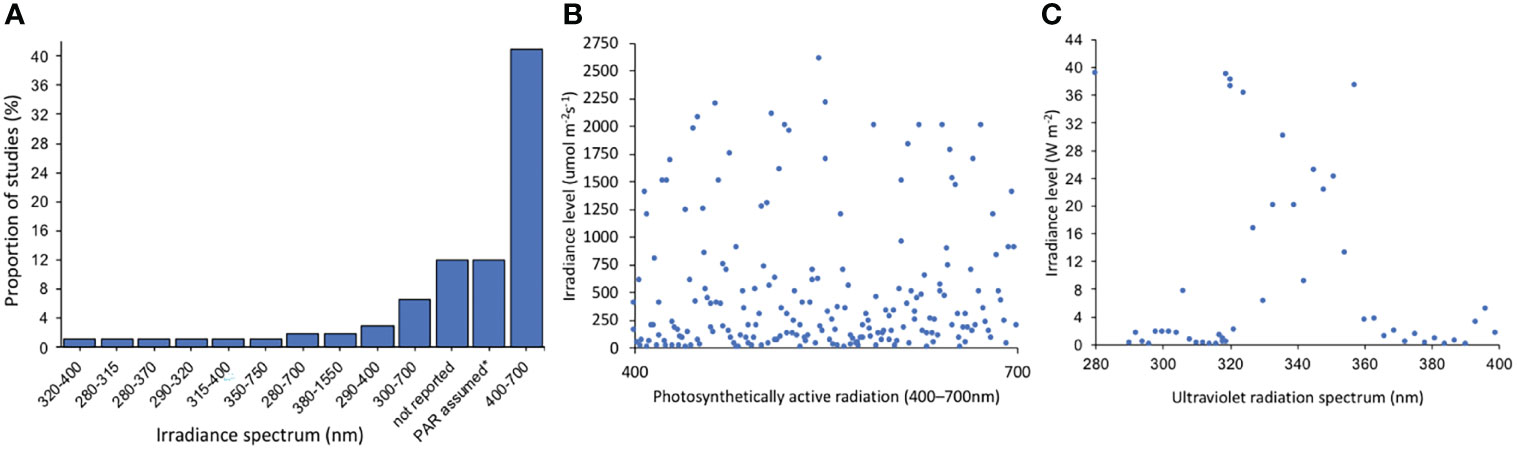
Figure 3 Irradiance spectrum and irradiance levels reported among analysed studies. (A) Proportion of different irradiance spectrum reported by range (nm); (B) Irradiance levels (μmol m-2s-1) specifically for visible light (PAR, 400–700) and (C) Irradiance levels (W m-2) on the ultraviolet radiation spectrum. Note that X axis on graphs b and c are arbitrary for illustration purpose, since most studies report one value along the spectrum range (e.g., PAR: 400–700; UVB: 290–320; UVA: 320–400; UVR: 290–400; UVC: 190–280).
Ultraviolet Radiation Blockage
The effects of UVR on corals reefs has been previously reviewed (Shick et al., 1996; Banaszak and Lesser, 2009). Following this work, however, the publication rate of studies investigating UVR effects on coral reefs has decreased because of the stratospheric ozone restoration during the last decade (Courtial et al., 2017). Most studies indicate that UVR produced a variety of negative effects on corals reefs, such as reduction of respiration, photosynthesis, coral/zooxanthellae growth/calcification, DNA damage, mortality, oxidative stress, and adverse effects on larvae settlement and reproductive functions (Jokiel, 1980; Lesser, 1996; Shick et al., 1996; Banaszak and Lesser, 2009). A few studies have shown positive effects of UVR in coral reefs organisms (e.g. protection against bleaching caused by Vibrio in the Mediterranean and a larger number of planula larvae released by Pocillopora damicornis) (Jokiel and York, 1982; Fine et al., 2002). Blanckaert et al. (2021) found that low levels of UVR increases antioxidant capacity under elevated temperatures and high levels of nitrates.
In general, the high light intensities that are associated with widespread bleaching are also coupled with high temperatures (Brown, 1997; Berkelmans, 2002). UVR and high temperatures worsen the stress effects on corals (Courtial et al., 2017). For instance, in Tahiti, the indicated mean daily UV-B threshold for corals to start bleaching is between 8–10 J cm¯² with temperatures between 29.0–29.5°C, and for a severe bleaching event the mean daily UV-B values may surpass >12 J cm¯² with temperatures above 30°C (Drollet et al., 1996). UV inhibition seems to occur both at low and high light intensities with a high component of short-wave radiation, and with maximum inhibitory effects with <380nm wavelengths (Roth et al., 1982). This must be considered specially for shallow water areas (0–5m), where direct ultraviolet irradiance is significantly higher compared to other parts of the reef (Veal et al., 2009).
Most bleaching events are directly attributed to rising ocean temperatures (Hughes et al., 2017; Hughes et al., 2018), although cold water coral bleaching is also possible (Hoegh-Guldberg and Fine, 2004; González-Espinosa and Donner, 2020). Nonetheless, some bleaching events can occur irrespective of water temperatures (Atwood et al., 1992; Gleason and Wellington, 1993). For instance, doldrum conditions leads to increased water clarity which allows UVR to reach all depths within the photic zone and produce bleaching effects at normal temperatures (Gleason and Wellington, 1993). UVR has been predicted to increase 10% per decade in summer months in the tropics, associated with depletion of ozone and cloud cover decrease (Udelhofen et al., 1999). After an extensive review of the effects of UVR on coral reefs organisms, Banaszak and Lesser (2009) concluded that UVR will only have significant effects in shallow reef environments. For those reason, any potential UVR reduction through artificial shading technologies may be worth pursuing.
Natural and Artificial Shading Effects
Natural Shading
The positive effects of natural shade on light and temperature-stressed corals are well known. Shade-adapted corals have higher chlorophyll and zooxanthellae symbiont densities to capture more light energy compared to corals adapted to high light environments (Falkowski and Dubinsky, 1981; Anthony and Hoegh-Guldberg, 2003), with shaded corals containing up to 7.4 times more chlorophyll than those adapted to high light intensities (Falkowski and Dubinsky, 1981). Shaded microhabitats cause corals to acclimate and photo-adapt to maximise light interception per unit of biomass and per unit mass of photosynthetic pigments (Anthony and Hoegh-Guldberg, 2003).
Natural shaded microhabitats (e.g. crevices, shade produced by the reef matrix or other colonies) provide refuge to corals and reduce bleaching severity during thermal stress episodes (Hoogenboom et al., 2017). Using data from 2,398 Florida reef sites (2005-2015), van Woesik and McCaffrey (2017) concluded that corals under shaded habitat (no light levels provided) and coral genotypes adapted to shaded environments with high productivity and turbidity were less likely to suffer thermal stress during the nearly annual occurrence of bleaching events. Another large study looking at the bleaching susceptibility of 191 species in the Maldives, found a wide range of species in shaded microhabitats with reduced bleaching effect, and therefore, unshaded microhabitats (3 to 5 m depth) are 1.3 times more likely to bleach than corals in shaded microhabitats (Muir et al., 2017). In the warm waters of Palau, no significant bleaching has been observed on reefs naturally shaded by steep natural walls (Fabricius et al., 2004).
Although protection against multiple stressors and long-term buffering are considered essential to properly define a coral refugia (Kavousi and Keppel, 2018), an increasing number of shaded and low light habitats, previously considered non-optimal for coral growth, are becoming progressively recognised as valuable coral refugium under climate change scenarios (Camp et al., 2018). For instance, in the US Virgin Islands, over 30 coral species are thriving in low light (70% PAR attenuation) conditions due to mangrove shading (Yates et al., 2014). Even turbid waters (able to reduce irradiance <250 μmol m-2s-1), that previously were considered inhospitable for corals, are now being considered as potential refuge under current ocean warming predictions (Cacciapaglia and van Woesik, 2016; Teixeira et al., 2019). That is the case of South Atlantic coral reefs, where corals which have high tolerance to turbidity, nutrients and deeper bathymetric distribution, are currently being included among future coral refugia habitats (Mies et al., 2020). In Australia, nearshore turbid areas at the central GBR proved to be a refugia during elevated temperature episodes during a 2016 bleaching event (Morgan et al., 2017). Places with decreased light and temperature are being considered as future refugia for coral reefs (West and Salm, 2003; Kavousi and Keppel, 2018). However, there is substantial evidence that reduced water clarity caused by terrestrial water run-off can cause negative effects on coral reefs (De’ath and Fabricius, 2010; Fabricius et al., 2014; López-Londoño et al., 2021). The effects of terrestrial runoff on coral reefs has been extensively studied and reviewed (Fabricius, 2005; Macdonald et al., 2013; Jones et al., 2020; O’Leary et al., 2021). Overall, it has been estimated that turbidity by suspended solids in the water column has been estimated to account for up to 74–79% of the total annual variation in irradiance, whereas clouds contribute 14–17% and tides 7–10% (Anthony et al., 2004).Corals consume more food in shaded/turbid environments (Anthony and Fabricius, 2000), which could help explain why shaded corals can avoid coral bleaching. It is well known that energy reserves (lipids) are depleted during bleaching (Rodrigues et al., 2008; Grottoli and Rodrigues, 2011). Corals that are able to increase heterotrophy and maintain their energy reserves, are more likely to survive high temperature events (Grottoli et al., 2006; Rodrigues and Grottoli, 2007; Tagliafico et al., 2017). For this reason, energy status (lipid stores) is considered a key predictor variable of coral survivorship over time (Anthony et al., 2007). Similarly, the effects of reduced light have also been studied on mesophotic reefs (deeper than 30 m), this work demonstrates that corals transitioning to heterotrophy (e.g., capturing plankton) due to light limitations deeper than 45 m and zooxanthellae are well adapted to low light conditions (Lesser et al., 2010). In addition, coral features such as: morphology, skeletal scattering of light, tissue thickness and symbiont species can play important role in coral population dynamics on mesophotic reefs (Lesser et al., 2021).
Even though shaded locations have been identified as potential refuges under high temperatures and irradiance scenarios, some very low light conditions have been associated with negative effects on corals. For instance, low light levels can significantly impact growth (Comeau et al., 2014) and some studies found low survival and low recruitment of corals underneath large Acropora hyacinthus shade (Baird and Hughes, 2000). Although at the other end of the range, high levels of light (with high temperatures) may also significantly reduce coral growth (Bahr et al., 2017). Corals may be more susceptible to bleaching under extreme light levels when also subjected to ocean acidification (Suggett et al., 2013).
Artificial Shading
The first experiment using artificial shading to study the effects of temperature and light on corals was carried out in 1978, when Coles and Jokiel (1978) used layers of neutral density screening to achieve 75% and 45% reduction of the full natural light. They demonstrated that high light intensities can aggravate the damage on corals sustained at high temperatures and that the environmental stress history may determine subsequent stress survival of corals. Since then, there has been a growing recognition that shading coral reefs represents a viable option to prevent bleaching, although the issue of how to sufficiently scale-up shading has yet to be resolved.
The recognised potential for shading to mitigate coral bleaching has boosted the number of projects (scientific and community projects) using and testing different types of shades to protect corals (Figure 4). For instance, awnings or umbrella-like devices attached to buoys have been considered to shade and protect corals located on high value reefs (Simpson et al., 2008), and a modification of that idea is currently being used in the Maldives on a community restoration project. Moreover, a study using shade cloth under natural light to achieve two levels of lights (low: 30 and high: 500 μmol photons m-2 s-1), demonstrated that low-light-acclimatised corals presented higher levels of chlorophyll, symbiont density and photosystem II protein than corals in high-light treatments (Jeans et al., 2013). Another project comparing the effectiveness of different engineering technologies to produce shade, concluded that shade cloth was superior at reducing irradiance and seawater temperatures when compared to aeration, airlift and sprinklers (Kramer et al., 2017). Positive effects of artificial shade under laboratory conditions have also demonstrated that non-shaded corals grow slower and show less colour than shaded corals (Coelho et al., 2017). A study using no shade, 28%, 65% and 87% shade respectively, found the largest reduction of zooxanthellae density at the extremes: no shade and 87% shade (Piggot et al., 2009). Overall, most studies used shade levels higher than 50%, highlighting a need to develop more research using lower levels of shade, which will be more feasible for larger scale shading attempts.
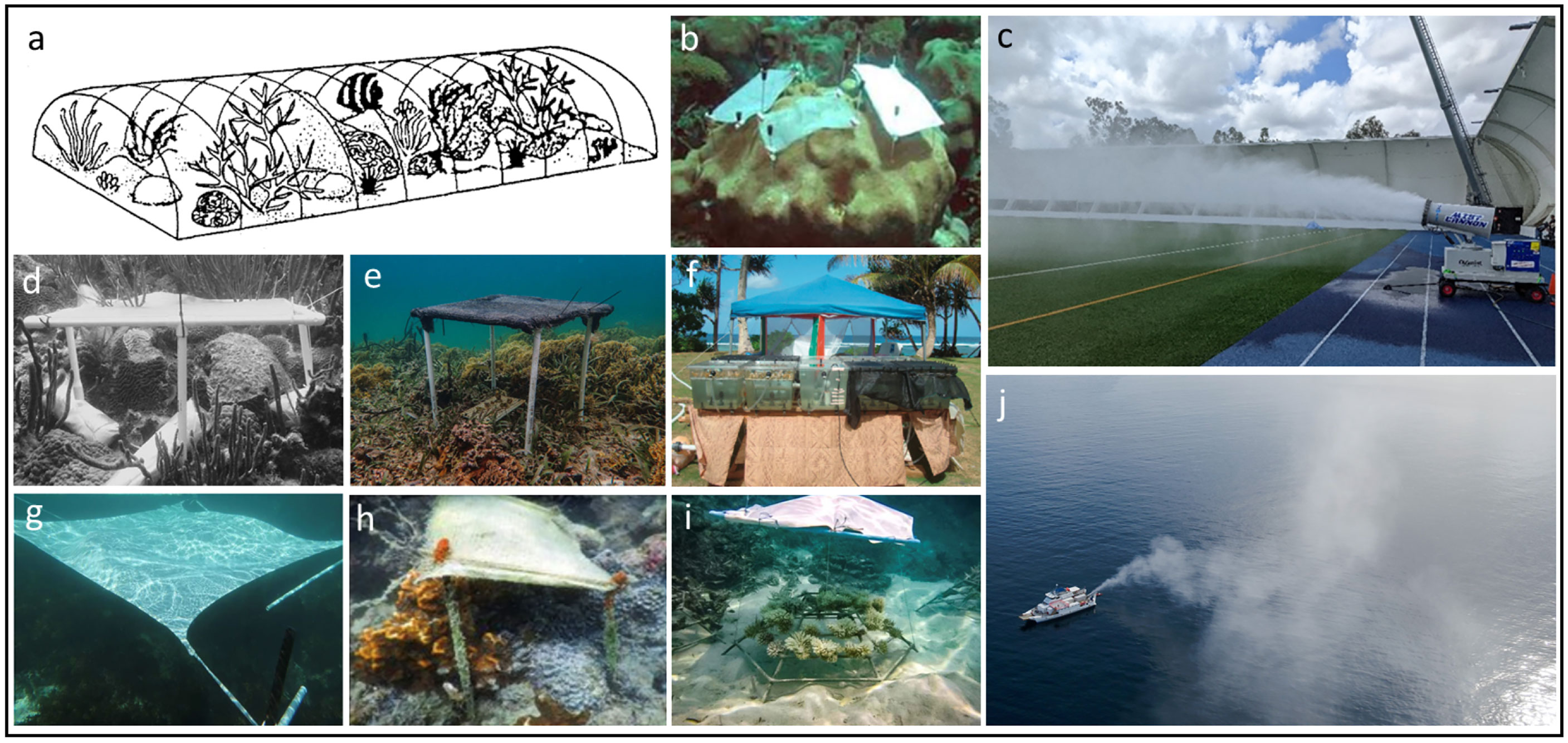
Figure 4 Different types of shade used in diverse coral reefs shading experiments. (A) Diagram showing a 20 m2 black plastic shade deployed for five weeks to investigate the effects of extreme turbidity on coral reefs structure (Rogers, 1979); (B) 13 x 8 cm wire mesh shades deployed for 11 days to investigate the effects of surface temperature and decreasing irradiance on cellular response (Piggot et al., 2009); (C) proof of concept fog cannon (with 100 impact pin nozzle) being tested (October, 2021) for the fogging development, as part of the Reef Restoration and Adaptation Program (Baker et al., 2021, picture: Joel Alroe); (D) smoked plastic on a polyvinyl chloride reducing 20% UVR and 65% PAR to effectively reduce white-plague disease in the Caribbean (Muller and van Woesik, 2009); (E) double layer 40% sun-block shade cloth deployed for 3 months in Panama, looking at the effects of low light environments (i.e. mangroves) as coral refugia (Stewart et al., 2021); (F) Outdoor experiment showing some tanks exposed to sunlight and other tanks covered with knitted black polyethylene fabric shade that reduced 75 and 50% respectively; (G) square-shaped shade sails of 4m2 and 9m2 and >98% UV-blockage certified deployed for 48 days to reduce macroalgal growth on degraded coral reefs; (H) Cotton lined fine white fabric deployed over a year to reduce the progression of coral-killing sponge in Palk Bay, India (Thinesh et al., 2017); (I) shading device utilised in the Maldives to protect bleached corals on a coral propagation project at Maldives (Marine Saver – Reefscapers); (J) cloud and sky brightening field trials at the Great Barrier Reef (March, 2021) testing the V22 cannon with 320 nozzles, as part of the Reef Restoration and Adaptation Program (picture: Brendan Kelaher).
Coral bleaching and mortality caused by instances of extreme low tide combined with high solar radiation are common events (Loya, 1976; Anthony and Kerswell, 2007; Raymundo et al., 2019). A survey of more than 13,000 corals at 14 reefs sites showed significant bleaching at reefs without waves (up to 75%) whereas wave-exposed reefs had < 1% bleaching (Anthony and Kerswell, 2007). Corals as deep as nine metres were bleached due to the increased light stress. Authors concluded that extreme low tidal events can be as damaging as high temperature episodes and that wave exposed sites prevented coral desiccation due to the periodic washing of waves. Thus, shading the reef through seawater fogging could be considered an intervention for reef tidal zone areas for protecting corals during these predictable natural disturbances reported by Anthony and Kerswell (2007). The seawater fog shading may protect the corals against high solar irradiation and the fog plume droplet moisture will reduce desiccation.Besides artificial shading as a tool to prevent coral bleaching, other indirect positive effects have been documented. For instance, shade material that reduces >98% UV has been successfully used to reduce macroalgal overgrowth on degraded coral reefs (Dajka et al., 2021). Cottonlined white fabric shade (selected among wool and silk for effectively reducing up to 30% irradiance, biodegradable nature and least absorbance of heat energy), was useful in reducing the spread of a coral-killing sponge (Thinesh et al., 2017). Furthermore, shade made out of smoke plastic, has been used as a tool to reduce the progression rate of white-plague disease (Muller and Van Woesik, 2009). Similar shading experiments (using polyethylene plastic sheeting) were attempted to reduce black-band disease in corals without success (Muller and Van Woesik, 2011). We note these previous studies were tested in small scale areas (<9m2) only and scalability and practicality of using shade cloth techniques across larger reef areas is still unproven.
Regardless of the shade source (natural or artificial), the reviewed literature demonstrates that different coral species may respond differently (i.e., interspecific, intraspecific and even intracolonial variations) to variable levels of light and stressors (Tilstra et al., 2017; Mizerek et al., 2018; Wangpraseurt et al., 2019; Kuanui et al., 2020; Dobson et al., 2021). Muir et al. (2017) found species identity explained 33.2% of the variation in observed bleaching and identified species lineages of either high or low relative extinction risk. Tilstra et al. (2017) demonstrated intra-individual variability in physiological responses of the scleratinian coral Stylophora pistillata, which may be attributed to differences amongst the spatially distinct clades for this coral species. Overall some genera are considered highly susceptible to bleaching stress, also shown among species variation (Muir et al., 2017).Even though shading technologies are proposed to be used during peak summer (Feb-March, outside of spawning season for the GBR), concerns related to their effects on coral reproduction and recruitment might be raised. If shading technology is considered for use during spawning season, potential impacts on the spawning and recruitment process would need to be considered. Encouragingly, Wallace (1985) observed that that corals recruit successfully under the shade of well-structured reefs, and the overwhelming majority of recruits settle in shaded spots hiding from direct exposure to light (Morse et al., 1988). Laboratory and survey field experiments observed a tendency of coral larvae to settle near shaded margins or edges rather than near to absolute low levels of light (Morse et al., 1988). Thus, shading corals may not threaten reproduction and recruitment processes.
Common Response Variables
Our review identified at least 30 different response variables measured to assess the effects of shade and light on corals. Four of them (symbiont density, skeletal growth, fluorometry normally measured with PAM and chlorophyll levels) were each used in at least 50 studies. Figure 5 shows 23 of those response variables identified. As previously reported by McLachlan et al. (2020), one of the principal challenges in comparing coral studies is the variety of standardisation methods used. Here, we observed at least nine different methods used to standardise coral response variables (Figure 6). Most studies standardise data with respect to surface area (89%), however surface area has been quantified by at least five different methods (i.e., dipping wax, aluminium foil, morphometrics of a cylinder or a circle/core, planimetry of pictures, and 3D modelled area). Further standardisation methods for corals included the use of buoyant weight, dry weight, number of polyps and number of larvae. The wide variety of methods are not directly comparable and create uncertainty when comparing among studies. Encouraging the uniform adoption of preferred standardization methods would be worthwhile.
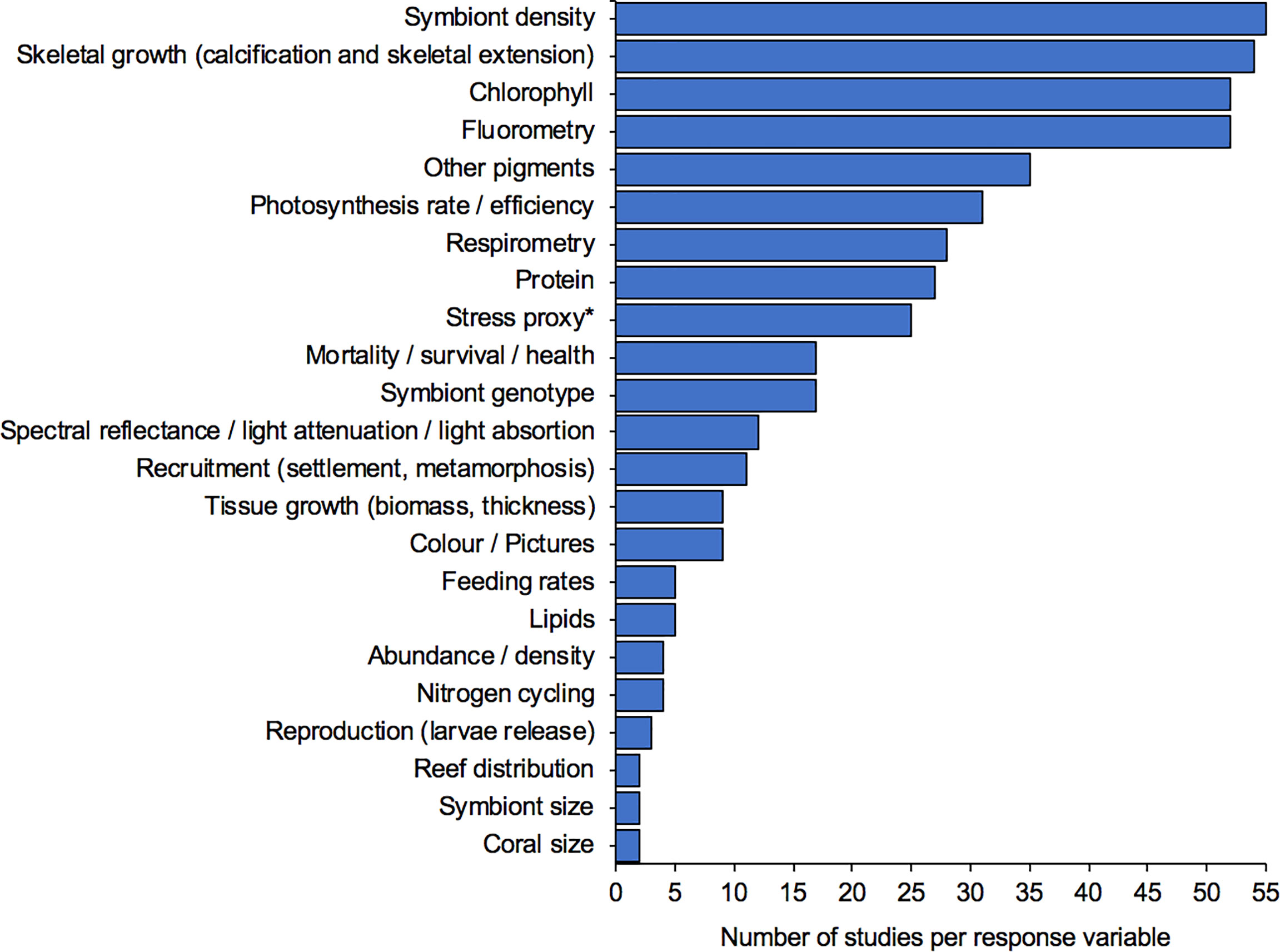
Figure 5 Different response variables investigated on studies looking at the effects of shade/light on coral. *stress proxies include reactive oxygen species (ROS), catalase activity (CAT), lipid peroxidation (LPO), superoxide dismutase (SOD), mycosporine-like amino acids (MAA), Non-enzymatic total antioxidant capacity (TAC), Protein tyrosine nitration (PTN), enzyme linked immunosorbent assays (ELISAs), ascorbate peroxidase (APX). Isolated response variables (<1) were not included (e.g. communication damage, coral surface warming, behaviour, phosphorous excretion, etc.).
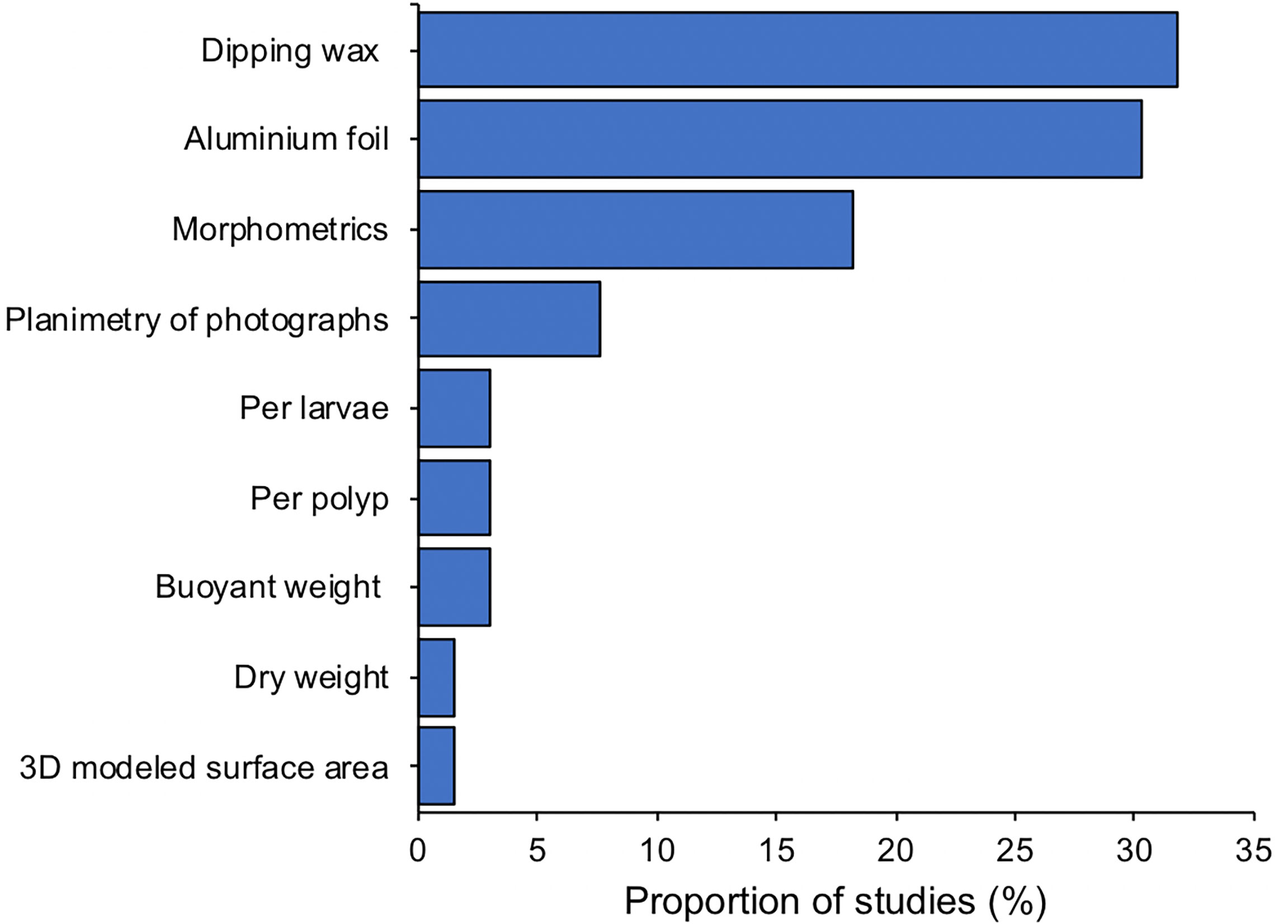
Figure 6 Proportion of standardisation methods used among studies measuring symbiont density and chlorophyll as a response variable.
Coral stress proxies as response variables proliferated following the publication of results based on Mycosporine-like amino acids (MAA) to detect UV sensitivity (Gleason and Wellington, 1995; Jokiel et al., 1997). After that, coral stress proxies have been commonly adopted among studies. Specially the enzymatic antioxidants superoxide dismutase (SOD) and catalase (CAT), regarded as the first line of defense responsible for removing accumulations of potentially toxic reactive oxygen species (ROS) before significant oxidative damage (Baird et al., 2009), are commonly used as indicators of bleaching stress. Among other stress proxies for corals, we identified direct measurements of ROS, non-enzymatic total antioxidant capacity (TAC), protein tyrosine nitration (PTN), enzyme linked immunosorbent assays (ELISAs) and ascorbate peroxidase (APX). Stress proxies and the variety of methods used to measure them (e.g., ROS methods includes intracellular and extracellular), are a topic deserving detailed independent review.
Shade and Recovery Processes of Corals
Implementing coral bleaching interventions following high temperature episodes could be critical to allow corals to reach full recovery (Berg et al., 2020). The coral recovery process consists of newly divided cells forming from the remaining live coral cells subjected to a less stressful environment (Brown et al., 2000), which is considered paramount to the corals’ capacity to survive thermal stress episodes (Rodríguez-Román et al., 2006). Yet, the required time to double the symbiont density of a coral (i.e., Goniastrea aspera) can be around one week (Zamani, 1995). Another study demonstrated that corals can take 3 weeks to recover from 1 week of high temperatures (Berg et al., 2020). Experiments rotating corals that bleached from the side facing the sun (PAR > 1500 μmol m-2 s-1) to the shaded side (PAR < 394 μmol m-2 s-1), showed that bleached corals can quickly recover if they are shaded from direct solar radiation (Brown et al., 2000).
Shading can largely mitigate the negative effects suffered during a high temperature episode (Berg et al., 2020). After bleaching, corals can recover especially if shaded from the sun (Brown et al., 2000). Although just a few studies incorporated recovery period, the evidence shows that reducing irradiance by 50% during the recovery period can decrease coral mortality by 17% (Coles et al., 2018). However, it may be difficult to implement shading technologies on a scale comparable to the geographical footprint of many reefs. A suitable strategy to maximise shading benefits may consider protecting one area (aiming to prevent bleaching completely) and later, move shading infrastructure to a different reef section to assist recovery and reduce coral mortality. Alternating this strategy across reefs and years, may help to increase resilient reefs. It is well understood, that coral thermal stress history is a critical driver of future thermal stress survivorship (Coles and Jokiel, 1978; Grottoli et al., 2014; Hughes et al., 2019). Corals from the year 1970 would suffer mortality after three days at 31°C, but in 2017, comparable corals can survive at least 13 days at that temperature (Coles et al., 2018). Reef systems that experience thermally variable episodes gain more coral-algal resilience than that provided by only heat-resistant symbionts (Oliver and Palumbi, 2011). Thus, some degree of bleaching is tolerable, or even desirable given the trajectory of climate change, so long as most corals survive and recover.
Conclusions
Bleaching events are becoming more severe, more frequent, and more widespread as a result of anthropogenic climate change. Motivated by proposals to use artificial shading as a means of intervention to reduce coral mortality from such events we have reviewed the current state of knowledge with respect to the effects of different levels of shade and light on coral reefs during bleaching stress. Although there are some promising results in the literature, larger scale practical evidence still needs to be verified.
(1) There was a large variability of irradiance units reported in the literature. The most common units were μmol photons m-2s-1 for studies looking at visible light. We suggest watts m-2 as a suitable unit for studies that measure both UVR and visible light, since watts m-2 applies at all frequencies and allows consideration of heat as well as light. We provided conversion factors per available units to facilitate comparisons among studies
(2) Any level of shading from peak sunlight exposure should help to alleviate the effects of light and thermal stress for at least a subset of coral species.
(3) Specifying a shading level that will protect all coral species during a predicted bleaching event cannot be done at present with the current state of knowledge. In particular, different coral species and symbionts appear to respond differently to varying levels of light and stressors.
(4) Reducing the levels of light in the whole spectrum (visible + UVR), but specially achieving a reduction of UVR in shallow waters, would be beneficial to corals during high temperature episodes.
(5) The use of fogging to shade during high irradiance events such as sub-aerial exposure during extreme low tides outside of thermal stress season, such as those described by Anthony and Kerswell (2007), could be particularly effective to protect coral reefs because fog seawater droplets can provide shade and may reduce desiccation at the same time.
(6) As well as during a bleaching event, applying any sort of shading on stressed corals after a coral bleaching event is likely to accelerate recovery and reduce mortality. Although more studies that include recovery period as part of the experiment are required.
(7) Since some studies have shown very low light levels can be deleterious to corals, shading interventions should ideally be tailored to provide appropriate levels of shade only when it is required, during the period of elevated stress and recovery.
Data Availability Statement
The original contributions presented in the study are included in the article. Further inquiries can be directed to the corresponding author.
Author Contributions
AT, PB, BK, DH designed the study. AT, PB, SE searched for the literature. AT extracted all the information. AT and PB analysed the data. All authors contributed to writing the manuscript and approved the final version.
Funding
This work was undertaken for the Reef Restoration and Adaptation Program (Cooling and shading sub-program), funded by the partnership between the Australian Governments Reef Trust and the Great Barrier Reef Foundation.
Conflict of Interest
The authors declare that the research was conducted in the absence of any commercial or financial relationships that could be construed as a potential conflict of interest.
Publisher’s Note
All claims expressed in this article are solely those of the authors and do not necessarily represent those of their affiliated organizations, or those of the publisher, the editors and the reviewers. Any product that may be evaluated in this article, or claim that may be made by its manufacturer, is not guaranteed or endorsed by the publisher.
Acknowledgments
We thank SCU Library Services (Helen Jack and team) for their help on finding old articles. We extend our deepest respect and recognition to all Traditional Owners of the Gumbaynggirr country where this review was written and to all Traditional Owners of the Great Barrier Reef and its Catchments as First Nations Peoples holding the hopes, dreams, traditions and cultures of the Reef.
References
Anthony K. R., Connolly S. R., Hoegh-Guldberg O. (2007). Bleaching, Energetics, and Coral Mortality Risk: Effects of Temperature, Light, and Sediment Regime. Limn. Oceanogr. 52, 716–726. doi: 10.4319/lo.2007.52.2.0716
Anthony K. R., Fabricius K. E. (2000). Shifting Roles of Heterotrophy and Autotrophy in Coral Energetics Under Varying Turbidity. J. Exp. Mar. Biol. Ecol. 252, 221–253. doi: 10.1016/S0022-0981(00)00237-9
Anthony K., Hoegh-Guldberg O. (2003). Variation in Coral Photosynthesis, Respiration and Growth Characteristics in Contrasting Light Microhabitats: An Analogue to Plants in Forest Gaps and Understoreys? Funct. Ecol., 246–259. doi: 10.1046/j.1365-2435.2003.00731.x
Anthony K., Kerswell A. (2007). Coral Mortality Following Extreme Low Tides and High Solar Radiation. Mar. Biol. 151, 1623–1631. doi: 10.1007/s00227-006-0573-0
Anthony K. R., Ridd P. V., Orpin A. R., Larcombe P., Lough J. (2004). Temporal Variation of Light Availability in Coastal Benthic Habitats: Effects of Clouds, Turbidity, and Tides. Limn. Oceanogr. 49, 2201–2211. doi: 10.4319/lo.2004.49.6.2201
Atwood D. K., Hendee J. C., Mendez A. (1992). An Assessment of Global Warming Stress on Caribbean Coral Reef Ecosystems. Bull. Mar. Sc. 51, 118–130.
Australian Institute of Marine Science (2021) Coral Bleaching Events. Available at: https://www.aims.gov.au/docs/research/climate-change/coral-bleaching/bleaching-events.html (Accessed October 15 2021).
Bahr K. D., Jokiel P. L., Rodgers K. (2017). Seasonal and Annual Calcification Rates of the Hawaiian Reef Coral, Montipora Capitata, Under Present and Future Climate Change Scenarios. ICES J. Mar. Sc. 74, 1083–1091. doi: 10.1093/icesjms/fsw078
Baird A. H., Bhagooli R., Ralph P. J., Takahashi S. (2009). Coral Bleaching: The Role of the Host. Trends. Ecol. Evol. 24, 16–20. doi: 10.1016/j.tree.2008.09.005
Baird A., Hughes T. (2000). Competitive Dominance by Tabular Corals: An Experimental Analysis of Recruitment and Survival of Understorey Assemblages. J. Exp. Mar. Biol. Ecol. 251, 117–132. doi: 10.1016/S0022-0981(00)00209-4
Baird M. E., Mongin M., Rizwi F., Bay L. K., Cantin N. E., Soja-Woźniak M., et al. (2018). A Mechanistic Model of Coral Bleaching Due to Temperature-Mediated Light-Driven Reactive Oxygen Build-Up in Zooxanthellae. Ecol. Model. 386, 20–37. doi: 10.1016/j.ecolmodel.2018.07.013
Baker P., Alroe J., Tagliafico A., Ellis S., Harrison L., Hernandez D., et al. (2021). “Fogging Feasibility Stage 1 Report,” in A Report Provided to the Australian Government by the Reef Restoration and Adaptation Program (Townsville: Reef Restoration and Adaptation Program).
Baker A. C., Glynn P. W., Riegl B. (2008). Climate Change and Coral Reef Bleaching: An Ecological Assessment of Long-Term Impacts, Recovery Trends and Future Outlook. Est. Coast. Shelf Sc. 80, 435–471. doi: 10.1016/j.ecss.2008.09.003
Banaszak A. T., Lesser M. P. (2009). Effects of Solar Ultraviolet Radiation on Coral Reef Organisms. Photochem. Photobiol. Sci. 8, 1276–1294. doi: 10.1039/b902763g
Bay L., Rocker M., Boström-Einarsson L., Babcock R., Buerger P., Cleves P., et al. (2019). “Reef Restoration and Adaptation Program: Intervention Technical Summary,” in A Report Provided to the Australian Government by the Reef Restoration and Adaptation Program (Townsville: Reef Restoration and Adaptation Program).
Bellworthy J., Fine M. (2017). Beyond Peak Summer Temperatures, Branching Corals in the Gulf of Aqaba are Resilient to Thermal Stress But Sensitive to High Light. Coral Reefs 36, 1071–1082. doi: 10.1007/s00338-017-1598-1
Ben-Zvi O., Wangpraseurt D., Bronstein O., Eyal G., Loya Y. (2021). Photosynthesis and Bio-Optical Properties of Fluorescent Mesophotic Corals. Front. Mar. Sci. 8, 389. doi: 10.3389/fmars.2021.651601
Berg J. T., David C. M., Gabriel M. M., Bentlage B. (2020). Fluorescence Signatures of Persistent Photosystem Damage in the Staghorn Coral Acropora Cf. Pulchra (Anthozoa: Scleractinia) During Bleaching and Recovery. Mar. Biol. Res. 16, 643–655. doi: 10.1080/17451000.2021.1875245
Berkelmans R. (2002). Time-Integrated Thermal Bleaching Thresholds of Reefs and Their Variation on the Great Barrier Reef. Mar. Ecol. Progr. Ser. 229, 73–82. doi: 10.3354/meps229073
Blanckaert A. C., de Barros Marangoni L. F., Rottier C., Grover R., Ferrier-Pagès C. (2021). Low Levels of Ultra-Violet Radiation Mitigate the Deleterious Effects of Nitrate and Thermal Stress on Coral Photosynthesis. Mar. Poll. Bull. 167, 112257. doi: 10.1016/j.marpolbul.2021.112257
Brakel W. H. (1979). Small-Scale Spatial Variation in Light Available to Coral Reef Benthos: Quantum Irradiance Measurements From a Jamaican Reef. Bull. Mar. Sc. 29, 406–413.
Brown B. (1997). Coral Bleaching: Causes and Consequences. Coral reefs 16, S129–S138. doi: 10.1007/s003380050249
Brown B., Dunne R., Warner M., Ambarsari I., Fitt W., Gibb S., et al. (2000). Damage and Recovery of Photosystem II During a Manipulative Field Experiment on Solar Bleaching in the Coral Goniastrea Aspera. Mar. Ecol. Progr. Ser. 195, 117–124. doi: 10.3354/meps195117
Cacciapaglia C., van Woesik R. (2016). Climate-Change Refugia: Shading Reef Corals by Turbidity. Global Change Biol. 22, 1145–1154. doi: 10.1111/gcb.13166
Camp E. F., Schoepf V., Mumby P. J., Hardtke L. A., Rodolfo-Metalpa R., Smith D. J., et al. (2018). The Future of Coral Reefs Subject to Rapid Climate Change: Lessons From Natural Extreme Environments. Front. Mar. Sci. 5, 4. doi: 10.3389/fmars.2018.00004
Coelho V., Fenner D., Caruso C., Bayles B., Huang Y., Birkeland C. (2017). Shading as a Mitigation Tool for Coral Bleaching in Three Common Indo-Pacific Species. J. Exp. Mar. Biol. Ecol. 497, 152–163. doi: 10.1016/j.jembe.2017.09.016
Coles S. L., Bahr K. D., Ku’ulei S. R., May S. L., McGowan A. E., Tsang A., et al. (2018). Evidence of Acclimatization or Adaptation in Hawaiian Corals to Higher Ocean Temperatures. PeerJ 6, e5347. doi: 10.7717/peerj.5347
Coles S., Jokiel P. L. (1978). Synergistic Effects of Temperature, Salinity and Light on the Hermatypic Coral Montipora Verrucosa. Mar. Biol. 49, 187–195. doi: 10.1007/BF00391130
Comeau S., Carpenter R. C., Edmunds P. J. (2014). Effects of Irradiance on the Response of the Coral Acropora Pulchra and the Calcifying Alga Hydrolithon Reinboldii to Temperature Elevation and Ocean Acidification. J. Exp.Mar. Biol. Ecol. 453, 28–35. doi: 10.1016/j.jembe.2013.12.013
Condie S. A., Anthony K. R., Babcock R. C., Baird M. E., Beeden R., Fletcher C. S., et al. (2021). Large-Scale Interventions may Delay Decline of the Great Barrier Reef. R. Soc Open Sc. 8, 201296. doi: 10.1098/rsos.201296
Courtial L., Roberty S., Shick J. M., Houlbrèque F., Ferrier-Pagès C. (2017). Interactive Effects of Ultraviolet Radiation and Thermal Stress on Two Reef-Building Corals. Limnology Oceanography 62, 1000–1013. doi: 10.1002/lno.10481
Dajka J. C., Beasley V., Gendron G., Barlow J., Graham N. A. (2021). Weakening Macroalgal Feedbacks Through Shading on Degraded Coral Reefs. Aq. Conserv. Mar. Fresh. Ecosyst. 31, 1660–1669. doi: 10.1002/aqc.3546
Davis K. L., Colefax A. P., Tucker J. P., Kelaher B. P., Santos I. R. (2021). Global Coral Reef Ecosystems Exhibit Declining Calcification and Increasing Primary Productivity. Commun. Earth Environm. 2, 1–10. doi: 10.1038/s43247-021-00168-w
De’ath G., Fabricius K. (2010). Water Quality as a Regional Driver of Coral Biodiversity and Macroalgae on the Great Barrier Reef. Ecol. Appl. 20 (3), 840–850. doi: 10.1890/08-2023.1
Dinesen Z. D. (1983). Shade-Dwelling Corals of the Great Barrier Reef. Mar. Ecol. Prog. Ser. Oldendorf 10, 173–185. doi: 10.3354/meps010173
Dobson K. L., Ferrier-Pagès C., Saup C. M., Grottoli A. G. (2021). The Effects of Temperature, Light, and Feeding on the Physiology of Pocillopora Damicornis, Stylophora Pistillata, and Turbinaria Reniformis Corals. Water 13, 2048. doi: 10.3390/w13152048
Drollet J. H., Faucon M., Martin P. M. (1996). Elevated Sea-Water Temperature and Solar Uv-B Flux Associated With Two Successive Coral Mass Bleaching Events in Tahiti. Oceanogr. Lit. Rev. 9, 923. doi: 10.1071/MF9951153
Fabricius K. E. (2005). Effects of Terrestrial Runoff on the Ecology of Corals and Coral Reefs: Review and Synthesis. Mar. Poll. Bull. 50, 125–146. doi: 10.1016/j.marpolbul.2004.11.028
Fabricius K., Logan M., Weeks S., Brodie J. (2014). The Effects of River Run-Off on Water Clarity Across the Central Great Barrier Reef. Mar. Poll. Bull. 84, 191–200. doi: 10.1016/j.marpolbul.2014.05.012
Fabricius K., Mieog J., Colin P., Idip D., van Oppen M. (2004). Identity and Diversity of Coral Endosymbionts (Zooxanthellae) From Three Palauan Reefs With Contrasting Bleaching, Temperature and Shading Histories. Mol. Ecol. 13, 2445–2458. doi: 10.1111/j.1365-294X.2004.02230.x
Falkowski P. G., Dubinsky Z. (1981). Light-Shade Adaptation of Stylophora Pistillata, a Hermatypic Coral From the Gulf of Eilat. Nature 289, 172–174. doi: 10.1038/289172a0
Fine M., Banin E., Israely T., Rosenberg E., Loya Y. (2002). Ultraviolet Radiation Prevents Bleaching in the Mediterranean Coral Oculina Patagonica. Mar. Ecol. Prog. Ser. 226, 249–254. doi: 10.3354/meps226249
Gleason D. F., Wellington G. M. (1993). Ultraviolet Radiation and Coral Bleaching. Nature 365, 836–838. doi: 10.1038/365836a0
Gleason D., Wellington G. (1995). Variation in UVB Sensitivity of Planula Larvae of the Coral Agaricia Agaricites Along a Depth Gradient. Mar. Biol. 123, 693–703. doi: 10.1007/BF00349112
González-Espinosa P. C., Donner S. D. (2020). Predicting Cold-Water Bleaching in Corals: Role of Temperature, and Potential Integration of Light Exposure. Mar. Ecol. Prog. Ser. 642, 133–146. doi: 10.3354/meps13336
Gonzalez-Espinosa P. C., Donner S. D. (2021). Cloudiness Reduces the Bleaching Response of Coral Reefs Exposed to Heat Stress. Glob. Change Biol. 27, 3474–3486. doi: 10.1111/gcb.15676
Grottoli A. G., Rodrigues L. J. (2011). Bleached Porites Compressa and Montipora Capitata Corals Catabolize δ13c-Enriched Lipids. Coral Reefs 30, 687. doi: 10.1007/s00338-011-0756-0
Grottoli A. G., Rodrigues L. J., Palardy J. E. (2006). Heterotrophic Plasticity and Resilience in Bleached Corals. Nature 440, 1186–1189. doi: 10.1038/nature04565
Grottoli A. G., Warner M. E., Levas S. J., Aschaffenburg M. D., Schoepf V., McGinley M., et al. (2014). The Cumulative Impact of Annual Coral Bleaching can Turn Some Coral Species Winners Into Losers. Glob. Change Biol. 20, 3823–3833. doi: 10.1111/gcb.12658
Harrison D. P., Baird M., Harrison L., Utembe S., Schofield R., Escobar Correa R., et al. (2019). “Reef Restoration and Adaptation Program: Environmental Modelling of Large Scale Solar Radiation Management,” A Report Provided to the Australian Government by the Reef Restoration and Adaptation Program (Townsville: Reef Restoration and Adaptation Program).
Hoegh-Guldberg O. (1999). Climate Change, Coral Bleaching and the Future of the World’s Coral Reefs. Mar. Fresh. Res. 50, 839–866. doi: 10.1071/MF99078
Hoegh-Guldberg O., Fine M. (2004). Low Temperatures Cause Coral Bleaching. Coral Reefs 23, 444–444. doi: 10.1007/s00338-004-0401-2
Hoogenboom M. O., Frank G. E., Chase T. J., Jurriaans S., Álvarez-Noriega M., Peterson K., et al. (2017). Environmental Drivers of Variation in Bleaching Severity of Acropora Species During an Extreme Thermal Anomaly. Front. Mar. Sci. 4, 376. doi: 10.3389/fmars.2017.00376
Hughes T. P., Anderson K. D., Connolly S. R., Heron S. F., Kerry J. T., Lough J. M., et al. (2018). Spatial and Temporal Patterns of Mass Bleaching of Corals in the Anthropocene. Science 359, 80–83. doi: 10.1126/science.aan8048
Hughes T. P., Graham N. A. J., Jackson J. B. C., Mumby P. J., Steneck R. S. (2010). Rising to the Challenge of Sustaining Coral Reef Resilience. Trends Ecol. Evol. 25, 633–642. doi: 10.1038/nature21707
Hughes T. P., Barnes M. L., Bellwood D. R., Cinner J. E., Cumming G. S., Jackson J. B. C., et al. (2017). Coral reefs in the Anthropocene. Nature 546, 82–90. doi: 10.1038/nature22901
Hughes T. P., Kerry J. T., Connolly S. R., Baird A. H., Eakin C. M., Heron S. F., et al. (2019). Ecological Memory Modifies the Cumulative Impact of Recurrent Climate Extremes. Nat. Clim. Change 9, 40–43. doi: 10.1038/s41558-018-0351-2
IPCC, 2021: Climate Change2021: The Physical Science Basis. Contribution of Working Group I to the Sixth Assessment Report of the Intergovernmental Panel on Climate Change [Masson-Delmotte, V., P. Zhai, A. Pirani, S.L. Connors, C. Péan, S. Berger, N. Caud, Y. Chen, L. Goldfarb, M.I. Gomis, M. Huang, K. Leitzell, E. Lonnoy, J.B.R. Matthews, T.K. Maycock, T. Waterfield, O. Yelekçi, R. Yu, and B. Zhou (eds.)]. Cambridge University Press, Cambridge, United Kingdom and New York, NY, USA, In press, doi: 10.1017/9781009157896
Jeans J., Campbell D. A., Hoogenboom M. O. (2013). Increased Reliance Upon Photosystem II Repair Following Acclimation to High-Light by Coral-Dinoflagellate Symbioses. Photosynth. Res. 118, 219–229. doi: 10.1007/s11120-013-9918-y
Jokiel P. L. (1980). Solar Ultraviolet Radiation and Coral Reef Epifauna. Science 207, 1069–1071. doi: 10.1126/science.207.4435.1069
Jokiel P. L., Lesser M. P., Ondrusek M. E. (1997). UV-Absorbing Compounds in the Coral Pocillopora Damicornis: Interactive Effects of UV Radiation, Photosynthetically Active Radiation, and Water Flow. Limnology oceanography 42, 1468–1473. doi: 10.4319/lo.1997.42.6.1468
Jokiel P. L., York Jr. R. H. (1982). Solar Ultraviolet Photobiology of the Reef Coral Pocillopora Damicornis and Symbiotic Zooxanthellae. Bull. Mar. Sc. 32, 301–315.
Jones R., Giofre N., Luter H. M., Neoh T. L., Fisher R., Duckworth A. (2020). Responses of Corals to Chronic Turbidity. Sci. Rep. 10, 1–13. doi: 10.1038/s41598-020-61712-w
Juhi Z. S., Mubin N. A. A. A., Jonik M. G. G., Salleh S., Mohammad M. (2021). Impact of Short-Term Light Variability on the Photobiology of Turbid Water Corals. J. Sea Res. 175, 102088. doi: 10.1016/j.seares.2021.102088
Kavousi J., Keppel G. (2018). Clarifying the Concept of Climate Change Refugia for Coral Reefs. ICES J. Mar. Sci. 75, 43–49. doi: 10.1093/icesjms/fsx124
Kawaguti S. (1937). On the Physiology of Reef Corals II. The Effects of Light on Color and Form of Reef Corals. Contrib. Palao Trop. Biol. Stat. South Sea Islands 11, 199–208.
Kellogg C. A., Moyer R. P., Jacobsen M., Yates K. (2020). Identifying Mangrove-Coral Habitats in the Florida Keys. PeerJ 8, e9776. doi: 10.7717/peerj.9776
Kramer J., Ripkey N., Segard L., Troisi M., Kramer P. R., Coraspe P., et al. (2017). Turn Down the Heat–An Innovative Citizen Science Pilot Project to Reduce Impacts of Coral Bleaching. Proc. Gulf Caribb. Fish. Inst. 69, 231–242.
Kuanui P., Chavanich S., Viyakarn V., Omori M., Fujita T., Lin C. (2020). Effect of Light Intensity on Survival and Photosynthetic Efficiency of Cultured Corals of Different Ages. Est. Coast. Shelf Sc. 235, 106515. doi: 10.1016/j.ecss.2019.106515
Lesser M. P. (1996). Elevated Temperatures and Ultraviolet Radiation Cause Oxidative Stress and Inhibit Photosynthesis in Ymbiotic Dinoflagellates. Limn. Oceanogr. 41, 271–283. doi: 10.4319/lo.1996.41.2.0271
Lesser M. P. (2000). Depth-Dependent Photoacclimatization to Solar Ultraviolet Radiation in the Caribbean Coral Montastraea Faveolata. Mar. Ecol. Prog. Ser. 192, 137–151. doi: 10.3354/meps192137
Lesser M. P., Slattery M., Mobley C. D. (2021). Incident Light and Morphology Determine Coral Productivity Along a Shallow to Mesophotic Depth Gradient. Ecol. Evol. 11, 12445–13454. doi: 10.1002/ece3.8066
Lesser M. P., Slattery M., Stat M., Ojimi M., Gates R. D., Grottoli A. (2010). Photoacclimatization by the Coral Montastraea Cavernosa in the Mesophotic Zone: Light, Food, and Genetics. Ecology 91 (4), 990–1003. doi: 10.1890/09-0313.1
López-Londoño T., Galindo-Martínez C. T., Gómez-Campo K., González-Guerrero L. A., Roitman S., Pollock F. J., et al. (2021). Physiological and Ecological Consequences of the Water Optical Properties Degradation on Reef Corals. Coral Reefs, 40, 1–14. doi: 10.1007/s00338-021-02133-7
Loya Y. (1976). Recolonization of Red Sea Corals Affected by Natural Catastrophes and Man-Made Perturbations. Ecology 57, 278–289. doi: 10.2307/1934816
Macdonald R. K., Ridd P. V., Whinney J. C., Larcombe P., Neil D. T. (2013). Towards Environmental Management of Water Turbidity Within Open Coastal Waters of the Great Barrier Reef. Mar. Poll. Bull. 74, 82–94. doi: 10.1016/j.marpolbul.2013.07.026
McLachlan R. H., Price J. T., Solomon S. L., Grottoli A. G. (2020). Thirty Years of Coral Heat-Stress Experiments: A Review of Methods. Coral Reefs 39, 885–902. doi: 10.1007/s00338-020-01931-9
Mies M., Francini-Filho R. B., Zilberberg C., Garrido A. G., Longo G. O., Laurentino E., et al. (2020). South Atlantic Coral Reefs are Major Global Warming Refugia and Less Susceptible to Bleaching. Front. Mar. Sci. 7, 514. doi: 10.3389/fmars.2020.00514
Mizerek T. L., Baird A. H., Madin J. S. (2018). Species Traits as Indicators of Coral Bleaching. Coral Reefs 37, 791–800. doi: 10.1007/s00338-018-1702-1
Morgan K. M., Perry C. T., Johnson J. A., Smithers S. G. (2017). Nearshore Turbid-Zone Corals Exhibit High Bleaching Tolerance on the Great Barrier Reef Following the 2016 Ocean Warming Event. Front. Mar. Sci. 4, 224. doi: 10.3389/fmars.2017.00224
Morse D. E., Hooker N., Morse A. N., Jensen R. A. (1988). Control of Larval Metamorphosis and Recruitment in Sympatric Agariciid Corals. J. Exp. Mar. Biol. Ecol. 116, 193–217. doi: 10.1016/0022-0981(88)90027-5
Muir P. R., Marshall P. A., Abdulla A., Aguirre J. D. (2017). Species Identity and Depth Predict Bleaching Severity in Reef-Building Corals: Shall the Deep Inherit the Reef? Proc. R. Soc. B: Biol. Sci. 284, 20171551. doi: 10.1098/rspb.2017.1551
Muller E., Van Woesik R. (2009). Shading Reduces Coral-Disease Progression. Coral reefs 28, 757–760. doi: 10.1007/s00338-009-0504-x
Muller E., Van Woesik R. (2011). Black-Band Disease Dynamics: Prevalence, Incidence, and Acclimatization to Light. J. Exp. Mar. Biol. Ecol. 397, 52–57. doi: 10.1016/j.jembe.2010.11.002
Mumby P. J., Chisholm J. R., Edwards A. J., Andrefouet S., Jaubert J. (2001). Cloudy Weather may Have Saved Society Island Reef Corals During the 1998 ENSO Event. Mar. Ecol. Prog. Ser. 222, 209–216. doi: 10.3354/meps222209
Muñiz-Castillo A. I., Rivera-Sosa A., Chollett I., Eakin C. M., Andrade-Gómez L., McField M., et al. (2019). Three Decades of Heat Stress Exposure in Caribbean Coral Reefs: A New Regional Delineation to Enhance Conservation. Sci. Rep. 9, 1–14. doi: 10.1038/s41598-019-47307-0
Odum H. T., Odum E. P. (1955). Trophic Structure and Productivity of a Windward Coral Reef Community on Eniwetok Atoll. Ecol. Monogr. 25, 291–320. doi: 10.2307/1943285
O’Leary M., Morgan K., Browne N. K. (2021). Turbid Coral Reefs: Past, Present and Future—A Review. Diversity 13, 251. doi: 10.3390/d13060251
Oliver T. A., Palumbi S. R. (2011). Do Fluctuating Temperature Environments Elevate Coral Thermal Tolerance? Coral Reefs 30, 429–440. doi: 10.1007/s00338-011-0721-y
Piggot A. M., Fouke B. W., Sivaguru M., Sanford R. A., Gaskins H. R. (2009). Change in Zooxanthellae and Mucocyte Tissue Density as an Adaptive Response to Environmental Stress by the Coral, Montastraea Annularis. Mar. Biol. 156, 2379–2389. doi: 10.1007/s00227-009-1267-1
Rau G. H., McLeod E. L., Hoegh-Guldberg O. (2012). The Need for New Ocean Conservation Strategies in a High-Carbon Dioxide World. Nat. Clim. Change 2, 720–724. doi: 10.1038/nclimate1555
Raymundo L., Burdick D., Hoot W., Miller R., Brown V., Reynolds T., et al. (2019). Successive Bleaching Events Cause Mass Coral Mortality in Guam, Micronesia. Coral Reefs 38, 677–700. doi: 10.1007/s00338-019-01836-2
Rinkevich B., Loya Y. (1984). Does Light Enhance Calcification in Hermatypic Corals? Mar.Biol. 80, 1–6. doi: 10.1007/BF00393120
Rocha R., Calado R., Cartaxana P., Furtado J., Serôdio J. (2013a). Photobiology and Growth of Leather Coral Sarcophyton Cf. Glaucum Fragments Stocked Under Low Light in a Recirculated System. Aquaculture 414–415, 235–242. doi: 10.1016/j.aquaculture.2013.08.018
Rocha R., Pimentel T., Seroôdio J., Rosa R., Calado R. (2013b). Comparative Performance of Light Emitting Plasma (LEP) and Light Emitting Diode (LED) in Ex Situ Aquaculture of Scleractinian Corals. Aquaculture 402–403, 38–45. doi: 10.1016/j.aquaculture.2013.03.028
Rodrigues L. J., Grottoli A. G. (2007). Energy Reserves and Metabolism as Indicators of Coral Recovery From Bleaching. Limn. Oceanogr. 52, 1874–1882. doi: 10.4319/lo.2007.52.5.1874
Rodrigues L. J., Grottoli A. G., Pease T. K. (2008). Lipid Class Composition of Bleached and Recovering Porites Compressa Dana 1846 and Montipora Capitata Dana 1846 Corals From Hawaii. J. Exp. Mar. Biol. Ecol. 358, 136–143. doi: 10.1016/j.jembe.2008.02.004
Rodríguez-Román A., Hernández-Pech X., E. Thome P., Enríquez S., Iglesias-Prieto R. (2006). Photosynthesis and Light Utilization in the Caribbean Coral Montastraea Faveolata Recovering From a Bleaching Event. Limn. Oceanogr. 51, 2702–2710. doi: 10.4319/lo.2006.51.6.2702
Rogers C. S. (1979). The Effect of Shading on Coral Reef Structure and Function. J. Exp. Mar. Biol. Ecol. 41, 269–288. doi: 10.1016/0022-0981(79)90136-9
Rosic N., Rémond C., Mello-Athayde M. A. (2020). Differential impact of heat stress on reef-building corals under different light conditions. Mar. Environ. Res. 158, 104947. doi: 10.1016/j.marenvres.2020.104947
Roth A. A., Clausen C. D., Yahiku P. Y., Clausen V. E., Cox W. W. (1982). Some Effects of Light on Coral Growth. Pac. Sci. 36, 68–81.
Shick J. M., Lesser M. P., Jokiel P. L. (1996). Effects of Ultraviolet Radiation on Corals and Other Coral Reef Organisms. Global Change Biol. 2, 527–545. doi: 10.1111/j.1365-2486.1996.tb00065.x
Simpson M. C., Gössling S., Scott D., Hall C. M., Gladin E. (2008). “Climate Change Adaptation and Mitigation in the Tourism Sector: Frameworks, Tools and Practices,” in Climate Change Adaptation and Mitigation in the Tourism Sector: Frameworks, Tools and Practices ( CABI). Switzerland: Chatelaine. United Nations Environment Programme (UNEP).
Sipos L., Boros I. F., Csambalik L., Székely G., Jung A., Balázs L. (2020). Horticultural Lighting System Optimalization: A Review. Sci. Hortic. 273, 109631. doi: 10.1016/j.scienta.2020.109631
Skirving W., Enríquez S., Hedley J., Dove S., Eakin C., Mason R., et al. (2017). Remote Sensing of Coral Bleaching Using Temperature and Light: Progress Towards an Operational Algorithm. Rem. Sens 10, 1–19. doi: 10.3390/rs10010018
Stewart H. A., Kline D. I., Chapman L. J., Altieri A. H. (2021). Caribbean Mangrove Forests Act as Coral Refugia by Reducing Light Stress and Increasing Coral Richness. Ecosphere 12, e03413. doi: 10.1002/ecs2.3413
Suggett D., Dong L., Lawson T., Lawrenz E., Torres L., Smith D. (2013). Light Availability Determines Susceptibility of Reef Building Corals to Ocean Acidification. Coral reefs 32, 327–337. doi: 10.1007/s00338-012-0996-7
Tagliafico A., Rudd D., Rangel M. S., Kelaher B. P., Christidis L., Cowden K., et al. (2017). Lipid Enriched Diets Reduce the Impacts of Thermal Stress in Corals. Mar. Ecol. Prog. Ser. 573, 129–141. doi: 10.3354/meps12177
Tamir R., Eyal G., Kramer N., Laverick J. H., Loya Y. (2019). Light Environment Drives the Shallow-to-Mesophotic Coral Community Transition. Ecosphere 10 (9), e02839. doi: 10.1002/ecs2.2839
Teixeira C. D., Leitao R. L., Ribeiro F. V., Moraes F. C., Neves L. M., Bastos A. C., et al. (2019). Sustained Mass Coral Bleaching, (2016–2017) in Brazilian Turbid-Zone Reefs: Taxonomic, Cross-Shelf and Habitat-Related Trends. Coral Reefs 38, 801–813. doi: 10.1007/s00338-019-01789-6
Thimijan R. W., Heins R. D. (1983). Photometric, Radiometric, and Quantum Light Units of Measure: A Review of Procedures for Interconversion. Hortic. Sci. 18, 818–822.
Thinesh T., Meenatchi R., Pasiyappazham R., Jose P. A., Selvan M., Kiran G. S., et al. (2017). Short-Term in Situ Shading Effectively Mitigates Linear Progression of Coral-Killing Sponge Terpios Hoshinota. PloS One 12, e0182365 doi: 10.1371/journal.pone.0187004.
Tilstra A., Wijgerde T., Dini-Andreote F., Eriksson B. K., Salles J. F., Pen I., et al. (2017). Light Induced Intraspecific Variability in Response to Thermal Stress in the Hard Coral Stylophora Pistillata. PeerJ 5, e3802. doi: 10.7717/peerj.3802
Titlyanov E. A., Titlyanova T. V., Yamazato K., van Woesik R. (2001). Photo-acclimation dynamics of the coral Stylophora pistillata to low and extremely low light, J. Exp. Mar. Biol. Ecol. 263 (2), 211–225, doi: 10.1016/S0022-0981(01)00309-4.
Tollefson J. (2021). Can Artificially Altered Clouds Save the Great Barrier Reef? Nature 596, 476–478. doi: 10.1038/d41586-021-02290-3
Tolleter D., Seneca F. O., DeNofrio J. C., Krediet C. J., Palumbi S. R., Pringle J. R., et al. (2013). Coral Bleaching Independent of Photosynthetic Activity. Curr. Biol. 23, 1782–1786. doi: 10.1016/j.cub.2013.07.041
Toller W. W., Rowan R., Knowlton N. (2001). Zooxanthellae of the Montastraea annularis species complex: patterns of distribution of four taxa of Symbiodinium on different reefs and across depths. Biol. Bull. 201(3), 348–359. doi: 10.2307/1543614
Udelhofen P. M., Gies P., Roy C., Randel W. J. (1999). Surface UV Radiation Over Australia 1979-1992: Effects of Ozone and Cloud Cover Changes on Variations of UV Radiation. J. Geoph. Res. Atm. 104, 19135–19159. doi: 10.1029/1999jd900306
van Woesik R., McCaffrey K. R. (2017). Repeated Thermal Stress, Shading, and Directional Selection in the Florida Reef Tract. Front. Mar. Sci. 4, 182. doi: 10.3389/fmars.2017.00182
Veal C. J., Michael K. J., Nunez M. (2009). Partitioning of Underwater Direct and Diffuse Ultraviolet Irradiance in a Shallow Water Coral Reef. Mar. Fresh. Res. 60, 1244–1253. doi: 10.1071/MF08318
Wallace C. (1985). Seasonal Peaks and Annual Fluctuations in Recruitment of Juvenile Scleractinian Corals. Mar. Ecol. Prog. Ser., 21, 289–298. doi: 10.3354/meps021289
Wangpraseurt D., Jacques S., Lyndby N., Holm J. B., Pages C. F., Kühl M. (2019). Microscale Light Management and Inherent Optical Properties of Intact Corals Studied With Optical Coherence Tomography. J. R. Soc. Interface 16, 20180567. doi: 10.1098/rsif.2018.0567
West J. M., Salm R. V. (2003). Resistance and Resilience to Coral Bleaching: Implications for Coral Reef Conservation and Management. Conserv. Biol. 17 (4), 956–967. doi: 10.1046/j.1523-1739.2003.02055.x
Wethey D. S., Porter J. W. (1976). Sun and Shade Differences in Productivity of Reef Corals. Nature 262, 281–282. doi: 10.1038/262281a0
Yates K. K., Rogers C. S., Herlan J. J., Brooks G. R., Smiley N. A., Larson R. A. (2014). Diverse Coral Communities in Mangrove Habitats Suggest a Novel Refuge From Climate Change. Biogeosciences 11, 4321–4337. doi: 10.5194/bg-11-4321-2014
Yonge C. M., Nicholls A. G. (1930). “Studies on the Physiology of Corals V. The Effect of Starvation in Light and in Darkness on the Relationship Between Corals and Zooxanthellae,” in British Museum (Natural History). Great Barrier Reef Expedition 1928–1929, vol. 1., 177–211. London: British Museum (Natural History).
Keywords: irradiance, ultraviolet radiation, visible light, shading, fogging, cloud brightening, reef restoration, climate change
Citation: Tagliafico A, Baker P, Kelaher B, Ellis S and Harrison D (2022) The Effects of Shade and Light on Corals in the Context of Coral Bleaching and Shading Technologies. Front. Mar. Sci. 9:919382. doi: 10.3389/fmars.2022.919382
Received: 13 April 2022; Accepted: 06 June 2022;
Published: 12 July 2022.
Edited by:
Aldo Cróquer, The Nature Conservancy, Dominican RepublicReviewed by:
Lei Jiang, Chinese Academy of Sciences, ChinaCory J Krediet, Eckerd College, United States
Copyright © 2022 Tagliafico, Baker, Kelaher, Ellis and Harrison. This is an open-access article distributed under the terms of the Creative Commons Attribution License (CC BY). The use, distribution or reproduction in other forums is permitted, provided the original author(s) and the copyright owner(s) are credited and that the original publication in this journal is cited, in accordance with accepted academic practice. No use, distribution or reproduction is permitted which does not comply with these terms.
*Correspondence: Alejandro Tagliafico, YWxlamFuZHJvLnRhZ2xpYWZpY29Ac2N1LmVkdS5hdQ==