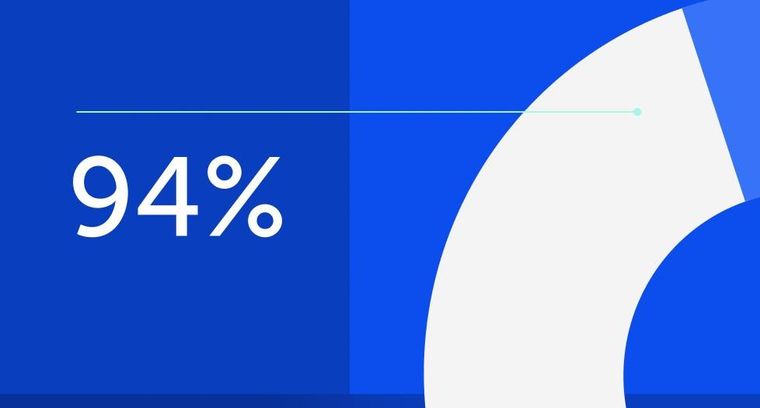
94% of researchers rate our articles as excellent or good
Learn more about the work of our research integrity team to safeguard the quality of each article we publish.
Find out more
ORIGINAL RESEARCH article
Front. Mar. Sci., 06 September 2022
Sec. Marine Ecosystem Ecology
Volume 9 - 2022 | https://doi.org/10.3389/fmars.2022.917985
This article is part of the Research TopicZooplankton and Nekton: Gatekeepers of the Biological Pump - Volume IIView all 5 articles
Across temperate and equatorial oceans, a diverse community of fish and zooplankton occupies the mesopelagic zone, where they are detectable as sound-scattering layers. At high latitudes, extreme day-night light cycles may limit the range of some species, while at lower latitudes communities are structured by dynamic ocean processes, such as temperature. Using acoustic and oceanographic measurements, we demonstrate that latitudinal changes in mesopelagic communities align with polar boundaries defined by deep ocean temperature gradients. At the transition to cold polar water masses we observe abrupt weakening and vertical dispersion of acoustic backscatter of mesopelagic organisms, thereby altering the structure of the mesopelagic zone. In the Canadian Arctic, we used biological sampling to show that this boundary is associated with a significant change in the pelagic fish community structure. Rapid ocean warming projected at mesopelagic depths could shift these boundaries with far-reaching effects on ecosystem function and biogeochemical cycles.
At mid-latitudes, the mesopelagic zone (i.e. depths ranging from 200 - 1000 m) houses communities of macrozooplankton and micronekton (Irigoien et al., 2014; Sutton et al., 2017) which occur in localized biomass peaks below the sunlit waters (Bianchi et al., 2013). As mid-trophic level organisms, many species in these communities link primary and microbial production with higher trophic levels, including megafauna (Lehodey et al., 2010; Choy et al., 2016; Bode et al., 2021; Schaber et al., 2022). Due to their nocturnal feeding migrations to surface waters, mesopelagic organisms also play an active role in the biological carbon pump (Davison et al., 2013; Irigoien et al., 2014). Global biomass estimates put mesopelagic fish resources between 2-20 gigatonnes, or roughly 100 times more than the annual catch of all other fisheries combined (Hidalgo and Browman, 2019). For a large portion of the global oceans, this mesopelagic biomass, measured as deep sound-scattering layers (DSLs), is dominated by lanternfishes and other low light adapted species (Catul et al., 2011). However, at high latitudes extreme seasonal variation and productivity sustains mostly polar-adapted organisms with unique life-history strategies and migration behaviors (Knutsen et al., 2017; Geoffroy et al., 2019; Vedenin et al., 2020).
Toward the poles, marine ecosystems are heavily influenced by seasonality, with extreme variation of light and sea surface temperature over the year. While temperature has direct effects on physiological rates (Gillooly et al., 2001), light influences the ecology of vertically migrating plankton and fish, with critical impacts on their predation and survival (Kaartvedt, 2008; Ljungström et al., 2021). According to prevailing ecological theory, diel variation in light explains the horizontal distribution range of vertically migrating mesopelagic fish, such as lanternfish (family Myctophidae) (Langbehn et al., 2021). Under this theory, the extreme light regime presented at high latitudes, with periods of constant illumination and constant darkness, hinders the establishment of viable populations of mesopelagic fish toward the poles (Langbehn et al., 2021). Polar and equatorward mesopelagic communities should thus vary considerably in their structure and functioning along the gradient of seasonal light availability. Yet, observational challenges to studying mesopelagic systems at high latitudes leave it unclear if transitions in mesopelagic structure occur gradually across photoperiod gradients or are constrained by other ocean processes, such as temperature.
Temperature is a dynamic ocean variable that influences marine community structure on global scales (Hoegh-Guldberg and Bruno, 2010). In ectotherms, metabolism is universally temperature-dependent (Gillooly et al., 2001; Bruno et al., 2015), and because metabolism fuels all organism processes and activities, temperature constraints scale from individual processes, to species interactions (Rall et al., 2010) and ecosystems (López-Urrutia et al., 2006). Temperature thus shapes ecological communities and influences properties of the environment, including, for example, carbon remineralization depths (Boscolo-Galazzo et al., 2018; Boscolo-Galazzo et al., 2021). Temperature may also modulate both food demand and availability, altering the biological profile of the pelagic realm. As we currently lack comprehensive knowledge of the life-history of mesopelagic fishes (and their thermal tolerances), predictions of community-wide response to environmental change remains limited, particularly in poleward systems.
The relative importance of environmental gradients, such as photoperiod (Kaartvedt, 2008) and temperature (Proud et al., 2017) or mesoscale features (Godø et al., 2012) to changes in mesopelagic community structure are uncertain. Acoustic measurements of backscatter enable mapping of the ‘biological profile’, revealing the distribution of pelagic macrozooplankton and micronekton throughout the water column (Benoit-Bird and Lawson, 2016). In this study, we use hull-mounted echosounders and ADCPs lowered in the water column along transects crossing polar fronts in both the Eastern Canadian Arctic and Southern Ocean to assess the impact of temperature boundaries on the distribution of mesopelagic organisms. We demonstrate that poleward dispersal of the globally coherent DSL is aligned with basin-scale mesopelagic temperature boundaries.
Shipboard acoustics were collected by two vessels during different sampling campaigns (Supplementary Figure 1). In the Canadian Arctic, data were collected aboard the research icebreaker CCGS Amundsen during the Integrated Studies and Ecosystem Characterization of the Labrador Sea Deep Ocean (ISECOLD) cruise in July and August 2018. In the Southern Ocean, data were collected in February 2008 along the 30°E section aboard the Norwegian research vessel G.O. Sars. Shipboard measurements from both cruises were made using an EK60 38 kHz scientific echosounder calibrated using the standard-sphere method (Demer et al., 2015).
The lowered acoustic Doppler profilers (LADCP) were mounted on CTD-rosettes and lowered to within 5 m of the bottom. The 300 kHz LADCP data in the Canadian Arctic were collected aboard the CCGS Amundsen during the ISECOLD (2018 and 2019 cruise), the Department of Fisheries and Oceans Canada Atlantic Zone Offshore Monitoring Program (AZOMP) in 2019, and the Takuvik Green Edge expedition to Baffin Bay in 2016. Data for these expeditions were available from the Polar Data Catalogue hosted by the Canadian Cryospheric Information Network (CCIN) (Forest et al., 2020). LADCP data for the Southern Ocean were downloaded from the National Centers of Environmental Information (NCEI) database (Firing et al., 2019) and were available from sections in 2005, 2007, 2008, and 2014.
Temperature and salinity profiles for the Canadian Arctic were collected from the CCGS Amundsen during the 2016, 2018 and 2019 campaigns using a Seabird © SBE 911+ deployed concurrently with the LADCP. Additional profiles from 2014 were downloaded from the Clivar & Carbon Hydrographic Data Office (CCHDO) database (Lee and Gobat, 2020). Temperature and salinity for the Southern Ocean from 2008 were also collected using a Seabird © SBE 911+ with concurrent LADCP measurements and were downloaded from the CCHDO database. (Speer, 2020)
Backscatter from shipboard echosounders (Sv, dB re 1 m-1) was calculated according to MacLennan et al. (2002). Sound-speed and absorption were calculated from CTD data (Mackenzie, 1981) and applied to backscatter values. For display purposes, -60 to -87 dB range was applied to the data to highlight the dominant mesopelagic signal.
Backscatter from LADCP units was calculated according to Mullison (2017) (eq. 1) with factory supplied calibration constants per beam. Vertical profiles of sound-speed and absorption were calculated from the concurrent CTD profiles and were used when converting the LADCP counts to (relative) backscattering values.
where C is an instrument specific constant, T is the temperature at the transducer (in °K), R is the along-beam range to the measurement, LDBM is 10log10 transmit pulse length in meters, PDBW is 10log10 transmit power in watts, α is the acoustic absorption (in dB m-1), E is the echo strength (in counts), Er is received noise (in counts), and kc is the Returned Signal Strength Indicator (RSSI) slope, a beam specific constant relating amplitude counts to the decibel scale. For Canadian Arctic data, we used factory supplied calibration constants and for the Southern Ocean data we used a generic instrument value of 0.45 dB count-1The high levels of sound-absorption at 300 kHz means that a hull-mounted transducer will have a very limited observational range before the signal from organisms drop below the noise-level. As backscatter is largely dependent on organism size; organisms much smaller than the wavelength (4.5 mm at 300 kHz and 1500 m s-1 sound speed) will give very little echo. Thus, the deployment of high-frequency acoustic equipment on a lowered platform, such as the CTD, enables observation of relatively small, weakly scattering animals at depths that surpass the range and vertical coverage of high frequency hull-mounted sounders.
The Gini index is a metric used to quantify resource inequalities (Gini Index, 2008), ranging from 0 for a perfectly evenly distributed resource to a value of 1 for maximum inequality. We here use the Gini index to quantify the level of uniformity of backscatter within the vertical domain i.e., a high index will reflect that much of the backscatter strength originates from a discrete scattering layer. To minimize influence of changes stemming from diel vertical migration, nighttime data were omitted whereby only casts when the sun was >6 degrees above the horizon were used. Gini indices were calculated for each vertical profile using the R package ‘ineq’ (Zeileis, 2014).
Mesopelagic fish were captured at 26 stations aboard the CCGS Amundsen using an Isaac-Kidd midwater trawl (IKMT) in 2020-2021 (Supplementary Figure 2). The net had a 13.5 m2 (4.5 m x 3 m) aperture and was equipped with a 3/4 inch stretched forward mesh and a ½ inch stretched codend mesh. Tows were performed obliquely at a speed of 2-3 m/s to depths ranging between 100 and 800 m to match the depth of the acoustic scattering layers. Catch was sorted to species, where possible. No net data were available across the polar front in the Southern Ocean and hence, community analysis was restricted to the Canadian Arctic in this study.
To compare the assemblage structures of adult fishes captured at mesopelagic depths on either side of the polar front, multivariate analysis was performed using the ‘vegan’ R package (Okansen et al., 2020). To reduce weighting of the dominant family Myctophidae, data was standardized using a Wisconsin double standardization and square root transformed. Similarity matrices of the abundance of mesopelagic fish across sites were then constructed using a Bray-Curtis Index (Bray and Curtis, 1957). In our final analysis, we used a family level taxonomy and omitted records containing singular occurrences of species, which were associated with coastal assemblages. An analysis of similarity (ANOSIM; Clarke, 1993) was performed on the resulting dissimilarity matrix to statistically evaluate community differences on either side of the polar boundary. Non-metric multidimensional scaling (nMDS) was used to provide a two-dimensional visual representation of fish assemblage structure.
In both the northern and southern hemispheres, backscatter decreased by up to 97% when crossing the boundaries of distinct polar and subpolar water masses in the upper mesopelagic zone (Figure 1). This pattern emerged from acoustic data, continuously recorded on transects crossing these boundaries, at 38 kHz, a frequency efficient at detecting swim-bladdered fish. In the Canadian Arctic, the disruption of the deep-scattering layer occurred in the Davis Strait (~ 66˚N) at the interface between Arctic intermediate water and the West Greenland Irminger Current (Curry et al., 2014), known as the West Greenland Polar Front. Mesopelagic (200-1000m) temperatures in the Labrador Sea ranged from 4-5˚C and decrease to 1-2˚C in Baffin Bay In the southern hemisphere, the disruption of the deep-scattering layer occurred along the Antarctic Polar front, where Antarctic intermediate water and upper circumpolar deep water converge (Orsi et al., 1995). Here, mesopelagic temperatures decreased more gradually with latitude and ranged from 2.5-3.5˚C north of the polar front, and dropped to approximately 2˚C south of the front.
Figure 1 38 kHz acoustic backscatter across polar transition zones. Backscatter volume strength (Sv, db re 1 m-1) collected during a latitudinal transect crossing the Arctic Circle in the Davis Strait (A) and the Antarctic sub-polar current (B). Echograms display acoustic backscatter calculated for 1 km long x 2.5 m deep intervals. Upper and lower thresholds of -87 to -60 dB were applied to facilitate visualization of the deep-scattering layers. Solid grey and red dashed lines represent Sv of upper water column (0-750 m) and a two-sided 25 km moving average, respectively. Temperature panels show mean +/- standard deviation of temperature in the mesopelagic zone (200-1000 m). Dotted blue line in left map panel indicates the position of the Arctic circle and solid blue line in right map panel indicates the Antarctic polar front.
A total of 1620 mesopelagic fish were captured in the Canadian Arctic in 2020-2021. The family-level community analysis showed that sites across the West Greenland polar front, distinguished by the deep water masses of the Labrador Sea and Baffin Bay, had significantly different fish communities (ANOSIM, R=0.67, p=0.001). P-value is based on 999 permutations. The resulting nMDS plot (Figure 2) revealed all but one sites grouped according to their positions relative to the polar front. South of the front, the fish community in the Atlantic waters of the Labrador Sea was characterized by a combination of four major fish families: Myctophidae (lanternfishes), Stomiidae (dragonfishes), Gonostomatidae (bristlemouths), and Bathylagidae (pencil-smelts). North of the front, the fish community in the Arctic waters of Baffin Bay was characterized by the families Gadidae (cods) and Liparidae (snailfishes). While there was a significant difference in overall community structure, a small number (n=40) of Benthosema glaciale were captured in the cold waters of Baffin Bay.
Figure 2 Family-level fish community response across the West Greenland Polar Front in the Canadian Arctic. Ordination plot using non-metric multidimensional scaling (nMDS, stress=0.057) of IKMT adult fish captured in the Canadian Arctic. Each sample (yellow) represent an individual site. Colored ellipses are computed to enclose all points along the boundary of groups representing fish families found in the Labrador Sea (red) and Baffin Bay (blue). Families (and representative images) are plotted as centroids and are computed as the weighted average scores in the ordination space.
High frequency LADCP profiles, at frequencies suitable to detect mesozooplankton down to ~0.5-1 cm in size, revealed a similar poleward reduction in backscatter in the mesopelagic zone (Figures 3A, B). These profiles further highlighted a concurrent shift in the dispersion of backscatter below the photic zone, through the meso- and bathy- pelagic water column (Figures 3C, D). Using the Gini inequality index (Gini Index, 2008), which is independent of the coincident reduction in relative backscatter levels (Sv [rel, dB re 1m-1]), we found biological backscatter in subpolar waters to be concentrated within a deep-scattering layer (high inequality). In contrast, in polar waters we observed a consistently uniform distribution of organisms in the water column. In both hemispheres, our observations were strongly tied to water masses on either side of the polar front (Figures 3E, F).
Figure 3 LADCP backscatter and Temperature-Salinity plots profiles across polar transition zones. 300 kHz LADCP profiles from the deep basins of the Labrador Sea in 2018 and 2019 and Baffin Bay in 2016 (A) and 150 kHz LADCP profiles along the southern transect (30°E line in 2008) across the Polar Frontal Zone (PFZ) (B). Vertical LADCP profiles in the Southern Ocean are presented as average backscatter per decimal degree. Vertical LADCP profiles in the Labrador Sea and Baffin Bay are presented as averages (solid lines) and 95% confidence intervals (dashed lines and grey shading). The corresponding Gini Index between 200 m depth and the seafloor for all stations deeper than 1500m along the northern transects (C) and corresponding Gini Index between 200-3000m for all stations in the Southern Ocean (D). Temperature-salinity diagrams from concurrent measurements in the Canadian Arctic (Baffin Bay/Labrador Sea) (E) and the Southern Ocean (F).
The poleward dissolution of the deep-scattering layer (DSL) and subsequent dispersion over the water column clearly aligns with deep temperature boundaries (i.e., polar fronts) between basin-scale oceanographic regimes. In both hemispheres, the high-latitude change in mesopelagic structure coincides with boundaries between previously defined biogeographical provinces (Longhurst, 2007; Sutton et al., 2017). In the northern hemisphere, changes in backscatter occurred near the Arctic circle (66° N) where photoperiod should begin to limit the success of low-light adapted lanternfishes (Kaartvedt, 2008; Ljungström et al., 2021), yet the boundary also occureddirectly at the transition between warm and cold basin waters (Figure 1). In the southern hemisphere, similar changes occurred along the Antarctic polar front, roughly 1700 km north of the Antarctic circle where photoperiod is presumed to constrain certain species. Together, these observations lend support to the idea that temperature plays a key role in structuring mesopelagic communities. We show that at high latitudes both the absolute levels of backscatter in DSLs, as well as the relative proportion of total mesopelagic backscatter found inside DSLs, are reduced. At lower latitudes, studies (Irigoien et al., 2014; Peña et al., 2014; Davison et al., 2015) implicitly suggest that a high proportion of pelagic biomass of micronekton and zooplankton are found within scattering layers, whereas our observations suggest that at high latitudes, the biomass is more evenly distributed over the water column (Figure 3). We call this effect the vertical dispersion of biomass, and note that it is likely to result in very different vertical patterns of intra- and interspecific interactions, i.e. vertical patterns of ecology. It remains unclear if the observed correlation between temperature and vertical dispersion is due to an altered prey field or physiological limitations of certain species; however, the outcome likely yields a major shift in resource partitioning, active carbon export, and overall ecosystem function.
Among DSLs in mid to high-latitude oceanic systems, a large proportion of 38 kHz acoustic backscatter is attributed to swim-bladdered fish (Irigoien et al., 2014), but due to a potential shift in swim-bladder morphology, interpretations of weakening of mesopelagic backscatter as indicative of biomass decreases across such boundaries can be misleading (Dornan et al., 2019). However, the same pattern observed here was also evident in higher frequency backscatter (300 and 150 kHz). Higher acoustic frequencies detect smaller components without air-inclusions, such as euphausiids and larger crustaceans, and are less prone to be affected by resonance effects that can bias density estimates (Stanton et al., 2010). The strong agreement between low and high-frequency acoustics suggests that the abrupt transition in total backscatter at the West Greenland Polar Front reflects a change in communities and/or a reduction in abundance of larger metazoans. This conclusion is supported by our catch data, which revealed that in the Canadian Arctic, the dispersion of the DSL is mirrored by a transition in the mesopelagic fish community. In the warmer waters of the Labrador Sea, the mesopelagic fish assemblage contains a variety of families, each with different adaptation to deep oceanic waters. Unlike single species dominated systems, the diversity of fish families present in the mesopelagic zone is likely to enhance community tolerance to environmental change (Lindegren et al., 2016).
The glacier lanternfish Benthosema glaciale (Family Myctophidae) was the most abundant fish species in the Labrador Sea. Their populations are predicted to decrease steadily along a latitudinal photoperiod gradient (Langbehn et al., 2021). Since this species is a significant contributor to acoustic sound scattering throughout the northern hemisphere (Pepin, 2013; Klevjer et al., 2020b), we expected to see a similar gradual dissipation of the DSL with latitude. This prediction is somewhat in line with previous findings which document Benthosemas’ occurrence above the Arctic circle, albeit in low numbers (Jensen, 1948; Jørgensen et al., 2005). However, our study rather demonstrates a drastic reduction in Myctophidae abundance north of the polar front, at least in the Canadian Arctic, which implicates other environmental drivers such as temperature. Moreover, the absence of larval specimens in previous surveys suggests that a component of its life-history may be inhibited by polar conditions, at least in the eastern Canadian Arctic (Sameoto, 1989). Nonetheless, there is evidence that pelagic physical boundaries (i.e., fronts) are frequently permeable to individual species. Due to their size and limited swimming capabilities, many lanternfishes are considered passive drifters, subject to advective dispersal in ocean currents (Kaartvedt et al., 2009). Therefore, their presence in small numbers in the Canadian Arctic is most likely due to the minor inflow of Atlantic-origin water entering Baffin Bay along Greenland’s coast. In the European Arctic, where the volume of Atlantic water inflow is much higher, transient Atlantic origin DSLs have been observed in the Arctic Ocean (Geoffroy et al., 2019; Priou et al., 2021; Snoeijs-Leijonmalm et al., 2022). Despite growing documentation of these DSLs permeating the Artic water, there is little to suggest that boreal fish populations forming these scattering layers can sustain themselves long enough to considerably alter the mesopelagic ecosystem.
While many of the species of mesopelagic fish in the southern hemisphere differ from those in the northern hemisphere, a similar family-level assemblage forms the subpolar DSL (Escobar-Flores et al., 2020). Therefore, we suggest that the overall fish community function and its resulting sensitivity to temperature is similar to that seen in the north. A study highlighting latitudinal effects on body size of mesopelagic fish (following Bergmann’s rule) found that the thermal range limit of small lanternfishes with a maximum size of ~50 mm is 2.5°C (Saunders and Tarling, 2018). Perhaps without coincidence, our observed backscatter boundary occurs at this same temperature in both hemispheres (Figure 1). As body size is critical in both metabolic constraint and feeding behavior, certain large bodied lanternfish have seemingly overcome this temperature constraint by feeding on larger, energy rich prey such as krill (Saunders et al., 2019) whilst simultaneously relying on mass immigration from warmer waters to sustain their populations (Saunders et al., 2017). While the dissolution of the DSL in poleward waters has been previously reported in the Southern Ocean (e.g. Escobar-Flores et al., 2020; Dornan et al., 2022); the relationship of this phenomenon to environmental variables and feeding guilds has remained uninvestigated. Furthermore, the similar shift in vertical structure of larger metazoans in both hemispheres lends further support to a significant shift in the ‘biological profile’ of the water column.
Our estimates of the vertical distribution of zooplankton and fish using an LADCP offer clues to the overall functioning of the pelagic ecosystem (Ressler et al., 1997; Burd and Thomson, 2012). By using high frequency lowered acoustics, we captured a snapshot of the daytime vertical distribution of larger metazoans (down to ~0.5-1 cm in size). Differences in the biological profile of across water mass boundaries, such as reduced backscatter (lower Sv (rel, dB)) and increased dispersal (low Gini index) suggest important changes in organic flux pathways. For instance, high biomass within the mesopelagic zone can lead to increased recycling of particulate (Henson et al., 2012), and a greater depth and magnitude of carbon re-mineralization (Marsay et al., 2015). In addition to a shift in biomass distribution, different mesopelagic communities across the fronts can also modify the carbon export rate and transfer efficiency due to variation in size, ontogeny, and behavior (Vedenin et al., 2020; Saba et al., 2021). This shift in mesopelagic functioning is further supported by studies showing distinct biogeochemical signatures with different carbon export regimes across polar boundaries (Vichi et al., 2011; Fan et al., 2020).
These temperature boundaries where the backscatter and dispersion of mesopelagic organisms changed were located at different latitudes (and light regimes) in each hemisphere and the overall stability of these boundaries remains unclear. Our high frequency LADCP measurements suggest that the pattern of vertical distribution across the polar front was consistent over several years in the Southern Ocean (Supplementary Figure S3). Using 38 kHz measurements from 2010, Escobar-Flores et al. (2020) report a similar boundary seen at the Antarctic Polar front (APF) (~ 63˚S) in the New Zealand sector of the Southern Ocean. Together, these findings suggest that temperature control in the mesopelagic zone may be a circumpolar phenomenon in the southern hemisphere. In the Indian Ocean sector, poleward displacement of the APF is linked to inter-annual changes in large-scale ocean circulation patterns (Kim and Orsi, 2014), such as the Southern Annular Mode (Gille, 2014). While the Antarctic circumpolar front is largely regulated by seafloor topography (Freeman et al., 2016), new evidence of past frontal movement may shift the existing paradigm of front stability (Civel-Mazens et al., 2021).
There have been considerably fewer investigations into variations in the West Greenland polar front; however, its stability will likely depend on the rate and volume of Atlantic water entering the Arctic through various gateways. In the European Arctic gateway, observations of northward shifts in boreal fish assemblage suggest that the movement of polar temperature boundaries are already underway (Fossheim et al., 2015) and a similar process is therefore possible in the northwest Atlantic. Overall, rapidly changing deep ocean temperature regimes (Brito-Morales et al., 2020) are likely to disrupt these boundaries, with far-reaching implications for ecosystem function and biogeochemical cycling.
The study of deep-ocean pelagic communities at high latitudes using net sampling and acoustic measurements remains a major logistical challenge. In the absence of systematic assessments at high-latitude systems, we compiled opportunistic measurements across multiple years, survey platforms, and instruments. Based on previously published work, we are confident that distinct water masses exist across these boundaries and that their biological communities broadly show predictable changes in structure and functioning in the transition to polar waters. Since our measurements are constrained to months that are ice-free and have warmer sea surface temperatures, we cannot ignore the possibilities that these boundaries may shift geographically or may be permeable under varying seasonal conditions. At lower-latitudes, mid-trophic mesopelagic biomass tends to respond to climate-atmospheric events (i.e. El Nino-Southern Oscillation) in 6 month lag intervals (Lehodey et al., 2010). Overall, observations of temporal response in poleward mesopelagic systems remain rare and our study provides a basis for further investigation.
Characterizing fish and zooplankton biomass and abundance remains a considerable challenge; net selectivity and avoidance behavior can play a large role in biasing catch composition (Kaartvedt et al., 2012). The unknown contribution of certain taxonomic groups to overall backscatter further inhibits our ability to make any conclusions regarding absolute abundance or biomass across these boundaries. For instance, smaller members of the family Gonostomatidae (e.g. Cyclothone sp.) may be excluded by larger mesh sizes, but bear swim-bladders that may resonate at lower frequencies, making significant contributions to backscatter (Peña et al., 2014). Unfortunately, we do not have biological data to match our acoustic measurements in the Southern Ocean and we therefore rely on the support of previous studies to assess how the micronekton communities may differ across these frontal boundaries.
Together, our acoustic and biological measurements offer a picture of the mesopelagic community transition in polar regions, which is abrupt and clearly aligns with a steep change in temperature between water masses. The change in mesopelagic communities across the West Greenland polar front suggests that community structures (and subsequent function) are altered by deep (>200 m) temperature gradients. The detailed mechanisms of how temperature drives highly-complex mesopelagic assemblages remains outside of the scope of this paper. However, our study provides a basis for future investigations into the functional biogeography of mesopelagic communities and their response to environmental forcing.
The high degree of similarity in both the northern and southern vertical profiles shows that the relationship between community structure and temperature is independent of the species present and suggests a major shift in the functioning of the upper ocean. While the change in vertical distribution of both micronekton and plankton is clearly associated with water mass transition, we lack a mechanistic understanding of the causes of the observed differences. Previous studies have suggested that mesopelagic fish biomass in high latitude systems are restricted by latitudinal light gradients (Kaartvedt, 2008; Ljungström et al., 2021), but empirical studies have found that while backscatter typically drops off across these gradients (Norheim et al., 2016, Escobar-Flores et al., 2020), biomass of mesopelagic fish does not follow the same patterns (Escobar-Flores et al., 2020; Klevjer et al., 2020a; Dornan et al., 2022). The front between Austral and Antarctic mesopelagic structure, however, starts much closer to the equator than the front in the northern hemisphere, suggesting that photoperiod is unlikely to be the only limitation to DSL forming fish communities in polar waters. The tight coupling with temperature documented in our data suggests that global warming will affect vertical structure and functioning of high-latitude marine ecosystems, including at mesopelagic depths.
EK60 Acoustic Data from both 2018 CCGS Amundsen and 2008 G.O. Sars campaigns are available from the author’s private repository: https://memorialu-my.sharepoint.com/:f:/g/personal/julek_chawarski_mi_mun_ca/Eqcw8Kt9ztNMi2tcAWdO6hYBUZCng75vXabh2miN97XRlg?e=VnhkdW For future reference, EK60 Acoustic data from the CCGS Amundsen are available by contacting Amundsen Science at info@as.ulaval.ca and metadata are available on the Polar Data Catalogue (www.polardata.ca; entry CCIN #13165). Net sampling data from the Canadian Arctic in 2020-2021, collected aboard the CCGS Amundsen, are available by contacting Amundsen Science at info@as.ulaval.ca Data for the temperature section crossing the Davis Strait are available at https://www.rvdata.us/search/cruise/KN213-02 (Cruise DOI: 10.7284/900550) LADCP and CTD data from the CCGS Amundsen used to produce Figure 2 (A, C, E) are available on the Polar Data Catalogue (www.polardata.ca; entries CCIN#12713 and CCIN#12714) Main text (Figures 1B and 2, D, F): Dataset: I06S_2008, 33RR20080204 https://www.ncei.noaa.gov/access/ocean-carbon-data-system/oceans/RepeatSections/clivar_i06.html Supplementary LADCP (Figure S2–A): Dataset P16S_2005, 33RR20050109 https://www.ncei.noaa.gov/access/ocean-carbon-data-system/oceans/RepeatSections/clivar_p16s.html Supplementary LADCP (Figure S2–B): Dataset I08S_2007, 33RR20070204 https://www.ncei.noaa.gov/access/ocean-carbon-data-system/oceans/RepeatSections/clivar_i08s.html Supplementary LADCP (Figure S2–C): Dataset P18_2007, 33RO20071215 https://www.ncei.noaa.gov/access/ocean-carbon-data-system/oceans/RepeatSections/clivar_p18.html Supplementary LADCP (Figure S2–D): Dataset GO-SHIP P16S_2014, EXPOCODE 320620140320 https://www.ncei.noaa.gov/access/ocean-carbon-data-system/oceans/RepeatSections/clivar_p16s.html.
The animal study was reviewed and approved by Memorial University of Newfoundland Animal Care Committee.
JC and TK conceived of this topic, collected, and analyzed data, and co-wrote the manuscript. DC obtained funding and led the ISECOLD project in the Labrador Sea. DC and MG contributed to the writing of the paper as well as discussion of results and comments on the manuscript.
This work is financially supported by the Ocean Frontier Institute through the Canada First Research Excellence fund, the Natural Sciences and Engineering Research Council through the Discovery Grant program, and ArcticNet a Network of Centres of Excellence Canada. Ship time was provided by the Department of Fisheries and Oceans Canada (DFO) and Amundsen Science. The R/V G.O Sars data were collected during the AKES expedition, funded by The Royal Norwegian Ministry of Fisheries and Coastal Affairs, Institute of Marine Research, The University of Bergen, Norwegian Antarctic Research Expeditions (NARE), The Research Council of Norway, and was sponsored by Norsk Hydro (StatoilHydro), the Norwegian Petroleum Directorate and ABB Norway. TAK was supported by the Ocean Frontier Institute (OFI) Visiting Fellows Program, and The Research Council of Norway is thanked for the financial support for the project «From swarming behaviour to trophic interactions: forecasting dynamics of Antarctic krill in ecosystem hotspots using behaviour-based models» (SWARM, RCN 267416). Some of the data presented herein were collected by the Canadian research icebreaker CCGS Amundsen and made available by the Amundsen Science program, which was supported by the Canada Foundation for Innovation and Natural Sciences and Engineering Research Council of Canada. The views expressed in this publication do not necessarily represent the views of Amundsen Science or that of its partners.
We acknowledge the support of the scientific staff and crew of the research icebreaker CCGS Amundsen and R/V G.O Sars. Special thanks to Marcel Babin, Marc Ringuette, Pascal Guillot, Cyril Aubry, Thibaud Dezutter, Jordan Sutton, Jennifer Herbig, Eugenie Jacobsen, Jonathan Fisher, and Clark Richards.
The authors declare that the research was conducted in the absence of any commercial or financial relationships that could be construed as a potential conflict of interest.
All claims expressed in this article are solely those of the authors and do not necessarily represent those of their affiliated organizations, or those of the publisher, the editors and the reviewers. Any product that may be evaluated in this article, or claim that may be made by its manufacturer, is not guaranteed or endorsed by the publisher.
The Supplementary Material for this article can be found online at: https://www.frontiersin.org/articles/10.3389/fmars.2022.917985/full#supplementary-material
Benoit-Bird K. J., Lawson G. L. (2016). Ecological Insights from Pelagic Habitats Acquired Using Active Acoustic Techniques. Ann. Rev. Mar. Sci. 8, 463–490. doi: 10.1146/annurev-marine-122414-034001
Bianchi D., Stock C., Galbraith E. D., Sarmiento J. L. (2013). Diel vertical migration: Ecological controls and impacts on the biological pump in a one-dimensional ocean model. Global Biogeochem. Cycles 27, 478–491. doi: 10.1002/gbc.20031
Bode A., Olivar M. P., Hernández-León S. (2021). Trophic indices for micronektonic fishes reveal their dependence on the microbial system in the north Atlantic. Sci. Rep. 11, 1–10. doi: 10.1038/s41598-021-87767-x
Boscolo-Galazzo F., Crichton K. A., Barker S., Pearson P. N. (2018). Temperature dependency of metabolic rates in the upper ocean: A positive feedback to global climate change? Glob. Planet. Change 170, 201–212. doi: 10.1016/j.gloplacha.2018.08.017
Boscolo-Galazzo F., Crichton K. A., Ridgwell A., Mawbey E. M., Wade B. S., Pearson P. N. (2021). Temperature controls carbon cycling and biological evolution in the ocean twilight zone. Science 371, 1148–1152. doi: 10.1126/science.abb6643
Bray J. R., Curtis J. T. (1957). An Ordination of the Upland Forest Communities of Southern Wisconsin. Ecol. Monogr. 27, 325–349. doi: 10.2307/1942268
Brito-Morales I., Schoeman D. S., Molinos J. G., Burrows M. T., Klein C. J., Arafeh-Dalmau N., et al. (2020). Climate velocity reveals increasing exposure of deep-ocean biodiversity to future warming. Nat. Clim. Chang. 10, 576–581. doi: 10.1038/s41558-020-0773-5
Bruno J. F., Carr L. A., O’Connor M. I. (2015). Exploring the role of temperature in the ocean through metabolic scaling. Ecology 96, 3126–3140. doi: 10.1890/14-1954.1
Burd B. J., Thomson R. E. (2012). Estimating zooplankton biomass distribution in the water column near the Endeavour Segment of Juan de Fuca Ridge using acoustic backscatter and concurrently towed net. Oceanography 25, 269–276. doi: 10.5670/oceanog.2012.25
Catul V., Gauns M., Karuppasamy P. K. (2011). A review on mesopelagic fishes belonging to family Myctophidae. Rev. Fish Biol. Fish 21, 339–354. doi: 10.1007/s11160-010-9176-4
Choy C. A., Wabnitz C. C. C., Weijerman M., Woodworth-jefcoats P. A., Polovina J. J. (2016). Finding the way to the top : how the composition of oceanic mid-trophic micronekton groups determines apex predator biomass in the central North Pacific. Mar. Ecol. Prog. Ser. 549, 9–25. doi: 10.3354/meps11680
Civel-Mazens M., Crosta X., Cortese G., Michel E., Mazaud A., Ther O., et al. (2021). Antarctic Polar Front migrations in the Kerguelen Plateau region, Southern Ocean, over the past 360 kyrs. Glob. Planet. Change 202, 103526. doi: 10.1016/j.gloplacha.2021.103526
Clarke K. R. (1993). Non-parametric multivariate analyses of changes in community structure. Aust. J. Ecol. 18, 117–143. doi: 10.1111/j.1442-9993.1993.tb00438.x
Curry B., Lee C. M., Petrie B., Moritz R. E., Kwok R. (2014). Multiyear volume, liquid freshwater, and sea ice transports through Davis strait 2004-10. J. Phys. Oceanogr 44, 1244–1266. doi: 10.1175/JPO-D-13-0177.1
Davison P. C., Checkley D. M., Koslow J. A., Barlow J. (2013). Carbon export mediated by mesopelagic fishes in the northeast Pacific Ocean. Prog. Oceanogr 116, 14–30. doi: 10.1016/j.pocean.2013.05.013
Davison P. C., Koslow J. A., Kloser R. J. (2015). Acoustic biomass estimation of mesopelagic fish: backscattering from individuals, populations, and communities. ICES J. Mar. Sci. 72, 1413–1424. doi: 10.1093/icesjms/fsv023
Demer D. A., Berger L., Bernasconi M., Bethke E., Boswell K. M., Chu D., et al. (2015). Calibration of acoustic instruments. ICES J. Mar. Sci. 326, 1–133 doi: 10.25607/OBP-185
Dornan T., Fielding S., Saunders R. A., Genner M. J. (2019). Swimbladder morphology masks Southern Ocean mesopelagic fish biomass. Proc. R. Soc B Biol. Sci. 286, 1–8. doi: 10.1098/rspb.2019.0353
Dornan T., Fielding S., Saunders R. A., Genner M. J. (2022). Large mesopelagic fish biomass in the Southern Ocean resolved by acoustic properties. Proc. R. Soc B Biol. Sci. 289, 1–10. doi: 10.1098/rspb.2021.1781
Escobar-Flores P. C., O’Driscoll R. L., Montgomery J. C., Ladroit Y., Jendersie S. (2020). Estimates of density of mesopelagic fish in the Southern Ocean derived from bulk acoustic data collected by ships of opportunity. Polar Biol. 43, 43–61. doi: 10.1007/s00300-019-02611-3
Fan G., Han Z., Ma W., Chen S., Chai F., Mazloff M. R., et al. (2020). Southern Ocean carbon export efficiency in relation to temperature and primary productivity. Sci. Rep. 10, 1–11. doi: 10.1038/s41598-020-70417-z
Firing E., Hummons J. M., Thurnerr A. M., Visbeck M. (2019). Ocean currents from lowered acoustic Doppler profilers (LADCP) from the Climate Variability (CLIVAR) project from 2003 to 2014 assembled at the University of Hawaii and Lamont-Doherty Earth Observatory, Columbia University (R/V Roger Revelle cruise KNOX14). Available at: https://www.ncei.noaa.gov/archive/accession/CLIVAR-LADCP.
Forest A., Guillot P., Linkowski T., Morriset S LADCP data collected by the CCGS Amundsen in the Canadian Arctic. LADCP data Collect. by CCGS Amundsen Can. Arct. (2020). doi: 10.5884/12714
Fossheim M., Primicerio R., Johannesen E., Ingvaldsen R. B., Aschan M. M., Dolgov A. V. (2015). Recent warming leads to a rapid borealization of fish communities in the Arctic. Nat. Clim. Chang. 5, 673–677. doi: 10.1038/nclimate2647
Freeman N. M., Lovenduski N. S., Gent P. R. (2016). Temporal variability in the Antarctic Polar Front (2002-2014). J. Geophys. Res. Ocean 121, 7263–7276. doi: 10.1002/2016JC012145
Geoffroy M., Daase M., Cusa M., Darnis G., Graeve M., Hernández N. S., et al. (2019). Mesopelagic sound scattering layers of the high Arctic: Seasonal variations in biomass, species assemblage, and trophic relationships. Front. Mar. Sci. 6. doi: 10.3389/fmars.2019.00364
Gille S. T. (2014). Meridional displacement of the Antarctic Circumpolar Current. Philos. Trans. R. Soc A Math. Phys. Eng. Sci. 372, 1–13. doi: 10.1098/rsta.2013.0273
Gillooly J. F., Brown J. H., West G. B., Savage V. M., Charnov E. L. (2001). Effects of Size and Temperature on Metabolic Rate. Science 293, 2248–2251. doi: 10.1126/science.1061967
Gini Index (2008). The Concise Encyclopedia of Statistics (New York, NY: Springer New York), 231–233. doi: 10.1007/978-0-387-32833-1_169
Godø O. R., Samuelsen A., Macaulay G. J., Patel R., Hjøllo S. S., Horne J., et al. (2012). Mesoscale eddies are oases for higher trophic marine life. PloS One 7, 1–9. doi: 10.1371/journal.pone.0030161
Henson S. A., Sanders R., Madsen E. (2012). Global patterns in efficiency of particulate organic carbon export and transfer to the deep ocean Global Biogeochem. Cycles. 26, 1–14. doi: 10.1029/2011GB004099
Hidalgo M., Browman H. I. (2019). Developing the knowledge base needed to sustainably manage mesopelagic resources. ICES J. Mar. Sci. 76, 609–615. doi: 10.1093/icesjms/fsz067
Hoegh-Guldberg O., Bruno J. F. (2010). The impact of climate change on the world’s marine ecosystems. Science 328, 1523–1528. doi: 10.1016/s0262-4079(10)61509-6
Irigoien X., Klevjer T. A., Røstad A., Martinez U., Boyra G., Acuña J. L., et al. (2014). Large mesopelagic fishes biomass and trophic efficiency in the open ocean. Nat. Commun. 5, 1–10. doi: 10.1038/ncomms4271
Jensen A. S. (1948). Contributions to the ichthyofauna of Greenland. Skr. Udbiget Univ. Zool. Mus. Kobenhavn 9, 1–182.
Jørgensen O. A., Hvingel C., Møller P. R., Treble M. A. (2005). Identification and mapping of bottom fish assemblages in Davis Strait and southern Baffin Bay. Can. J. Fish. Aquat. Sci. 62, 1833–1852. doi: 10.1139/f05-101
Jensen A. S. (1948). Contributions to the ichthyofauna of Greenland, 8-24. Skr. Udbiget Univ. Zool. Mus. Kobenhavn 9, 1–182.
Kaartvedt S. (2008). Photoperiod may constrain the effect of global warming in arctic marine systems. J. Plankton Res. 30, 1203–1206. doi: 10.1093/plankt/fbn075
Kaartvedt S., Røstad A., Klevjer T. A., Staby A. (2009). Use of bottom-mounted echo sounders in exploring behavior of mesopelagic fishes. Mar. Ecol. Prog. Ser. 395, 109–118. doi: 10.3354/meps08174
Kaartvedt S., Staby A., Aksnes D. L. (2012). Efficient trawl avoidance by mesopelagic fishes causes large underestimation of their biomass. Mar. Ecol. Prog. Ser. 456, 1–6. doi: 10.3354/meps09785
Kim Y. S., Orsi A. H. (2014). On the variability of antarctic circumpolar current fronts inferred from 1992-2011 altimetry. J. Phys. Oceanogr 44, 3054–3071. doi: 10.1175/JPO-D-13-0217.1
Klevjer T. A., Melle W., Knutsen T., Aksnes D. L. (2020a). Vertical distribution and migration of mesopelagic scatterers in four north Atlantic basins. Deep Sea Res. Part II Top. Stud. Oceanogr. 180, 1–11. doi: 10.1016/j.dsr2.2020.104811
Klevjer T., Melle W., Knutsen T., Strand E., Korneliussen R., Dupont N., et al. (2020b). Micronekton biomass distribution, improved estimates across four north Atlantic basins. Deep. Res. Part II Top. Stud. Oceanogr, 1801–11. doi: 10.1016/j.dsr2.2019.104691
Knutsen T., Wiebe P. H., Gjøsæter H., Ingvaldsen R. B., Lien G. (2017). High Latitude Epipelagic and Mesopelagic Scattering Layers—A Reference for Future Arctic Ecosystem Change. Front. Mar. Sci. 4, 1–21. doi: 10.3389/fmars.2017.00334
Langbehn T. J., Aksnes D. L., Kaartvedt S., Fiksen Ø., Ljungström G., Jørgensen C. (2022). Poleward distribution of mesopelagic fishes is constrained by seasonality in light. Glob. Ecol. Biogeogr. 31, 546–561. doi: 10.1111/geb.13446
Lee C., Gobat J. (2020). CTD data from Cruise 316N20130914, exchange version. Accessed from CCCHDO. doi: 10.7284/900550
Lehodey P., Murtugudde R., Senina I. (2010). Bridging the gap from ocean models to population dynamics of large marine predators: A model of mid-trophic functional groups. Prog. Oceanogr 84, 69–84. doi: 10.1016/j.pocean.2009.09.008
Lindegren M., Checkley D. M., Ohman M. D., Koslow J. A., Goericke R. (2016). Resilience and stability of a pelagic marine ecosystem. Proc. R. Soc B Biol. Sci. 283, 1–9. doi: 10.1098/rspb.2015.1931
Ljungström G., Langbehn T. J., Jørgensen C. (2021). Light and energetics at seasonal extremes limit poleward range shifts. Nat. Clim. Chang. 11, 530–536. doi: 10.1038/s41558-021-01045-2
López-Urrutia Á., Martin E. S., Harris R. P., Irigoien X. (2006). Scaling the metabolic balance of the oceans. Proc. Natl. Acad. Sci. 103, 8739–8744. doi: 10.1073/pnas.0601137103
Mackenzie K. V. (1981). Nine-term equation for sound speed in the oceans. J. Acoust. Soc Am. 70, 807–812. doi: 10.1121/1.386920
MacLennan D. N., Fernandes P. G., Dalen J. (2002). A consistent approach to definitions and symbols in fisheries acoustics. ICES J. Mar. Sci. 59, 365–369. doi: 10.1006/jmsc.2001.1158
Marsay C. M., Sanders R. J., Henson S. A., Pabortsava K., Achterberg E. P., Lampitt R. S. (2015). Attenuation of sinking particulate organic carbon flux through the mesopelagic ocean. Proc. Natl. Acad. Sci. U. S. A 112, 1089–1094. doi: 10.1073/pnas.1415311112
Mullison J. (2017). Backscatter Estimation Using Broadband Acoustic Doppler Current Profilers - Updated. ASCE Hydraul. Meas. Exp. Methods Conf. 031, 1–6.
Norheim E., Klevjer T. A., Aksnes D. L. (2016). Evidence for light-controlled migration amplitude of a sound scattering layer in the Norwegian Sea. Mar. Ecol. Prog. Ser. 551, 45–52 doi: 10.3354/meps11731.
Okansen J., Blanchet G., Friendly M., Kindt R., Legendre P., McGlinn D., et al. (2020). Vegan: Community ecology package.
Orsi A. H., Whitworth T., Nowlin W. D. (1995). On the meridional extent and fronts of the Antarctic Circumpolar Current. Deep. Res. Part I 42, 641–673. doi: 10.1016/0967-0637(95)00021-W
Peña M., Olivar M. P., Balbín R., López-Jurado J. L., Iglesias M., Miquel J., et al. (2014). Acoustic detection of mesopelagic fishes in scattering layers of the Balearic Sea (western Mediterranean). Can. J. Fish. Aquat. Sci. 71, 1186–1197. doi: 10.1139/cjfas-2013-0331
Pepin P. (2013). Distribution and feeding of Benthosema glaciale in the western Labrador Sea: Fish-zooplankton interaction and the consequence to calanoid copepod populations. Deep. Res. Part I Oceanogr. Res. Pap. 75, 119–134. doi: 10.1016/j.dsr.2013.01.012
Priou P., Nikolopoulos A., Flores H., Gradinger R., Kunisch E., Katlein C., et al. (2021). Dense mesopelagic sound scattering layer and vertical segregation of pelagic organisms at the Arctic-Atlantic gateway during the midnight sun. Prog. Oceanogr 196, 102611. doi: 10.1016/j.pocean.2021.102611
Proud R., Cox M. J., Brierley A. S. (2017). Biogeography of the Global Ocean’s Mesopelagic Zone. Curr. Biol. 27, 113–119. doi: 10.1016/j.cub.2016.11.003
Rall B. C., Vucic-Pestic O., Ehnes R. B., Emmerson M., Brose U. (2010). Temperature, predator–prey interaction strength and population stability. Glob. Change Biol. 16, 2145–2157. doi: 10.1111/j.1365-2486.2009.02124.x
Ressler P. H., Biggs D. C., Wormuth J. H. (1997). Acoustic estimates of zooplankton and micronekton biomass using an ADCP. Journal of the Acoustical Society of America. 2167–2168. doi: 10.1121/1.421730
Saba G. K., Burd A. B., Dunne J. P., Hernández-León S., Martin A. H., Rose K. A., et al. (2021). Toward a better understanding of fish-based contribution to ocean carbon flux. Limnol. Oceanogr 66, 1639–1664. doi: 10.1002/lno.11709
Sameoto D. (1989). Feeding Ecology of the Lantern Fish Benthosema Glaciale in a Subarctic Region. Polar Biol. 9, 167–178. doi: 10.1007/BF00297172
Saunders R. A., Collins M. A., Stowasser G., Tarling G. A. (2017). Southern Ocean mesopelagic fish communities in the Scotia Sea are sustained by mass immigration. Mar. Ecol. Prog. Ser. 569, 173–185. doi: 10.3354/meps12093
Saunders R. A., Hill S. L., Tarling G. A., Murphy E. J. (2019). Myctophid Fish (Family Myctophidae) Are Central Consumers in the Food Web of the Scotia Sea (Southern Ocean). Front. Mar. Sci. 6, 1–22. doi: 10.3389/fmars.2019.00530
Saunders R. A., Tarling G. A. (2018). Southern ocean mesopelagic fish comply with Bergmann’s rule. Am. Nat. 191, 343–351. doi: 10.1086/695767
Schaber M., Gastauer S., Cisewski B., Hielscher N., Janke M., Peña M., et al. (2022). Extensive oceanic mesopelagic habitat use of a migratory continental shark species. Sci. Rep. 12, 1–14. doi: 10.1038/s41598-022-05989-z
Snoeijs-Leijonmalm P., Flores H., Sakinan S., Hildebrandt N., Svenson A., Castellani G., et al. (2022). Unexpected fish and squid in the central Arctic deep scattering layer. Sci. Adv. 8, 1–17. doi: 10.1126/sciadv.abj7536
Stanton T. K., Chu D., Jech J. M., Irish J. D. (2010). New broadband methods for resonance classification and high-resolution imagery of fish with swimbladders using a modified commercial broadband echosounder. ICES J. Mar. Sci. 67, 365–378. doi: 10.1093/icesjms/fsp262
Sutton T. T., Clark M. R., Dunn D. C., Halpin P. N., Rogers A. D., Guinotte J., et al. (2017). A global biogeographic classification of the mesopelagic zone. Deep. Res. Part I Oceanogr. Res. Pap. 126, 85–102. doi: 10.1016/j.dsr.2017.05.006
Vedenin A., Kulagin D., Musaeva E., Vereshchaka A. (2020). The southern Polar Front as a key to mesoplankton migratory behavior. Sci. Rep. 10, 1–14. doi: 10.1038/s41598-020-70720-9
Vichi M., Allen J. I., Masina S., Hardman-Mountford N. J. (2011). The emergence of ocean biogeochemical provinces: A quantitative assessment and a diagnostic for model evaluation. Global Biogeochem. Cycles 25, 1–17. doi: 10.1029/2010GB003867
Zeileis A. (2014) ineq: Measuring inequality, concentration, and poverty. Available at: https://cran.r-project.org/package=ineq.
Keywords: mesopelagic zone, deep scattering layer (DSLs), hydroacoustics, marine ecology and ecosystem, polar, biogeography
Citation: Chawarski J, Klevjer TA, Coté D and Geoffroy M (2022) Evidence of temperature control on mesopelagic fish and zooplankton communities at high latitudes. Front. Mar. Sci. 9:917985. doi: 10.3389/fmars.2022.917985
Received: 11 April 2022; Accepted: 17 August 2022;
Published: 06 September 2022.
Edited by:
Alberto Basset, University of Salento, ItalyReviewed by:
Kerrie M. Swadling, University of Tasmania, AustraliaCopyright © 2022 Chawarski, Klevjer, Coté and Geoffroy. This is an open-access article distributed under the terms of the Creative Commons Attribution License (CC BY). The use, distribution or reproduction in other forums is permitted, provided the original author(s) and the copyright owner(s) are credited and that the original publication in this journal is cited, in accordance with accepted academic practice. No use, distribution or reproduction is permitted which does not comply with these terms.
*Correspondence: Julek Chawarski, anVsaWFuLmNoYXdhcnNraUBnbWFpbC5jb20=
Disclaimer: All claims expressed in this article are solely those of the authors and do not necessarily represent those of their affiliated organizations, or those of the publisher, the editors and the reviewers. Any product that may be evaluated in this article or claim that may be made by its manufacturer is not guaranteed or endorsed by the publisher.
Research integrity at Frontiers
Learn more about the work of our research integrity team to safeguard the quality of each article we publish.