Corrigendum: Advection and composition of Dinophysis spp. populations along the European Atlantic shelf
- 1IFREMER, DYNECO, Pelagos Laboratory, Plouzané, France
- 2IFREMER, DYNECO, Phycotoxins Laboratory, Nantes, France
- 3AZTI, Marine Research Division, Basque Research and Technology Alliance (BRTA), Pasaia, Spain
The main objective was to study relationships between the regional biogeography of Dinophysis species and water masses circulation along the European Atlantic coast. Hydrodynamic connectivities were estimated with a Lagrangian approach. Available and validated physical hindcasts from regional hydrodynamical models, with different resolutions were used. The target area is the Bay of Biscay (NE Atlantic) and connectivity was evaluated between a set of spatially distributed stations and during temporally specified periods. Different indexes related to connectivity properties such as mean, median, most frequent transit times were calculated. To illustrate the dispersion pattern, a molecular approach was jointly set-up to describe the species composition of this genus. At the seasonal scale, a high connectivity within the Bay of Biscay was observed with a slight northward connectivity from Galicia coastal waters to the Shelf of the Bay of Biscay. By comparison to the connectivity between shelf waters of French Brittany and English Channel waters, a higher connectivity between shelf waters of French Brittany and the Celtic Sea shelf was observed. The species mixing in the Bay of Biscay from Galicia waters to the Celtic Sea was confirmed by the genetic analyses despite the absence of Dinophysis sacculus in natural samples. The molecular methodology developed for this work, permitting at least the description of the species composition, also highlights, at the European scale, an unexpected low genetic variability which echoes the complex taxonomic classification inside the genus and the difficulties encountered by national monitoring programs to reach a taxonomic resolution at species level. It is now necessary to start some monitoring at the species level before realizing mid- or long-term forecasts.
1 Introduction
Marine protists form a large part of microbial communities situated at the base of marine food webs but, within the several thousands known species, a small number produce biotoxins that can affect human health and/or induce severe economical losses. Dinophysis genus [including more than 120 phototrophic and heterotrophic species, Jensen and Daugbjerg (2009)] has several toxic species [≈ 11, Zingone and Larsen (2022)] and due to its high economical impact on the worldwide coastal fishing and aquaculture activities, understanding its biogeography and the connectivities between coastal areas appears of primary importance. This genus is widely distributed, and seems to exhibit highly variable preferendums among species, Dinophysis acuminata surviving across a wide temperature range (Kamiyama et al., 2010) while other species have restricted environmental tolerances, as for example D. norvegica limited to boreal regions or D. tripos typically found in tropical-temperate waters (Reguera et al., 2012). To our knowledge, despite these differences, it is also possible to underline similarities in their life cycles: they are planktonic without known cyst stage, mixotrophic and kleptoplastidic. Their toxicity is due to the production of several lipophilic toxins such as the okadaïc acid and dinophysistoxins that cause diarrheic syndromes in humans (DSP, Diarrheic Shellfish Poisoning). Dinophysis spp. usually do not bloom at very high densities (rarely over 100 000 cells/L), but even when they constitute a small proportion of the microplankton community, they can contaminate seafood. Another relevant point is the apparent difficulty of taxonomic identification using optical microscopy. At least, nine species were observed along the European Atlantic coast (Bresnan et al., 2021), but various reports and publications detailed morphological variation among species that can be greater than initially described, specifically for the Dinophysis acuminata complex (Lassus and Bardouil, 1991; Bravo et al., 1995; Raho et al., 2013; Wolny et al., 2020; Séchet et al., 2021). Monitoring programs thus provide some historical and well distributed dataset to identify areas with recurrent blooms of this genus but the interpretation of the time series remains difficult at the species level and probably introduced bias in our perception of their ecology. For example, Bresnan et al. (2021) consider members of the Dinophysis acuminata complex are coastal species but according to their long growing season (occurring from spring to early autumn) and life duration, a coastal limitation of these species seems unlikely.
Like other marine species, their biogeography remains primarily constrained by environmental selection and connectivity between each environment through the advection of water parcels. Two different hypotheses are put forward to assess population connectivity and community composition. One idea is that ‘everything is everywhere but the environment selects’ (Bass Becking, 1934; Fenchel and Finlay, 2004; de Wit and Bouvier, 2006), whereas the other concept assumes that ‘the regions of the oceans are not so well connected’ (Martiny, 2006; Casteleyn, 2010) leading to local populations with their own ecological niches and physiological capacities. However, due to the fluid properties in marine environment, each parcel of water can reach any other part of the ocean, it’s just a matter of time. There is thus no opposition between the two hypotheses, as suggested by Jonsson and Watson (2016). Neither concept is entirely accurate excluding the other, as species biogeography depends on the organisms observed, the efficiency of the selection process, the rate of the evolution processes as well as their transport velocities by ocean currents. As an example, it has been observed that some microbial communities have strong genetic differentiation at small spatial scales (Godhe et al., 2013). In the same way, investigating the physical transport pathways that can potentially facilitate the migration of harmful algal blooms (HABs) species between different coastal regions along the Atlantic coast, will enhance our ability to respond adequately to such events occurring in a changing marine environment, e.g., to get monitoring programs climate-ready in advance of major changes and eventually to highlight potential new hazards.
The main objective of this work was to investigate the composition and distribution of the Dinophysis community along the European Atlantic Area. A methodology based on two complementary approaches was used: a single nucleotide polymorphism (SNP) approach to attempt a description of the Dinophysis community at least at the species level, in synergy with a Lagrangian approach to investigate the seasonal and inter-annual variability of hydrodynamical connectivity patterns of shelf waters. Additionally, the variability of connectivity matrices obtained by different hydrodynamic models is examined.
2 Material and Methods
2.1. Selection of Target Areas and In-Situ Samples
To study seasonal connectivities between water masses with some potential presence and due to the large distribution of the species, 8 large targets (Figure 1) were well distributed along the north Atlantic margin. The target areas also correspond to water masses where this genus has been regularly observed (Delmas et al., 1992; Xie et al., 2007; Farrell et al., 2012; Batifoulier et al., 2013; Diaz et al., 2013). Reversely, to describe community composition, in-situ samples were not so well distributed. Due to the methodological development, an opportunistic in-situ sampling strategy based on scientific cruises or bloom events in waters close to Marine stations were used during the study. Some surface water (0-5m) was randomly sampled (Figure 1). When some Dinophysis cells were significantly observed in microplankton samples (> 500 cells/liters), a large volume of seawater (between 2 and 16 liters) was collected. The sea water was next gravity filtered through nylon filters between 20 and 200 µm and the intermediate fraction was conserved. Cells were next resuspended in seawater and the volume was set to 200 ml. The 200 ml sample was next divided in two parts. A 20 ml fraction was fixed with Lugol for microscopic observations and the 180 ml remaining was re-filtered on a polycarbonate filter (5, 10 or 20 µm mesh size). After filtration, filters were rolled in cryotubes with an addition of RNAlater, flash-frozen in liquid nitrogen and conserved in -80°C. At the end of the project, 23 samples were collected from Spain to North of the Bay of Biscay (Figure 1) but unfortunately no sample was obtained for the English channel, Celtic Sea and North Sea.
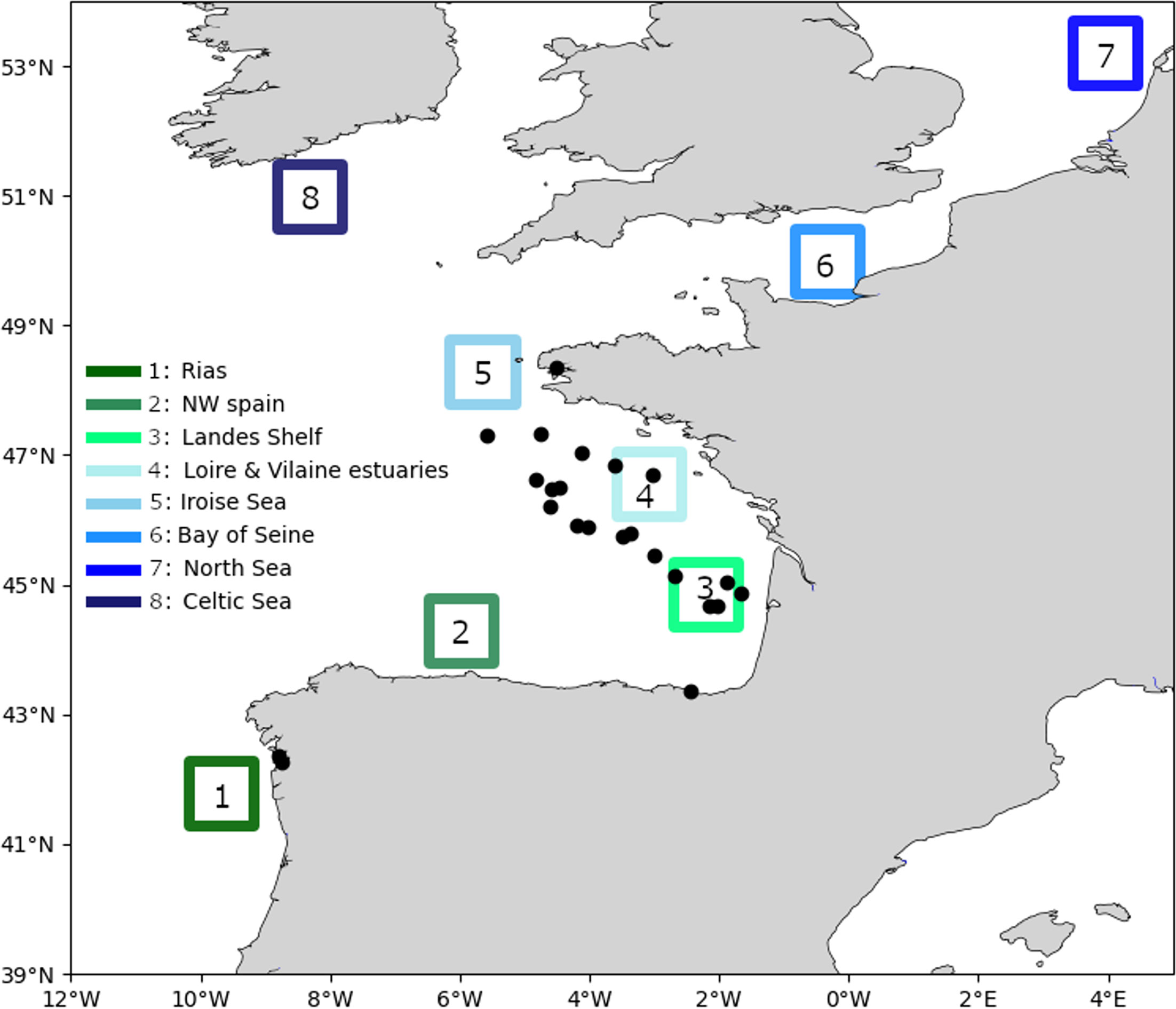
Figure 1 Positions of the target areas defined for the connectivities (boxes from 1 to 7) and in-situ samples. In-situ samples of the surface water (0-5m) were made in Ria de Vigo (July 2018), coastal Basque waters (2019 and 2020), Bay of Brest (May to July 2013, 2014 and 2015) and over the continental shelf (May 2018 and 2019).
2.2. Water Masses Connectivity
Lagrangian methods in ocean models are commonly used for connectivity purposes and this approach makes it possible to study, in time and space, all ocean connections [e.g., Blanke and Raynaud (1997); Alberto et al. (2011); Watson (2011); Mora (2012); Van Sebille (2018)]. It should be indicated that within this general framework, biological connectivity is usually described as the “realised” exchange of individuals (or genes) between marine populations (taking into account the initial populations size) whereas the hydrological connectivity describes the potential exchange of individuals between water masses (i.e. potential individual exchanges between populations without taking into account any of the selection processes occurring during advection neither any difference between populations size).
2.2.1. Particle Properties
Connectivities being highly sensitive to particle properties (life duration, vertical position, …) estimates from fish larvae provided by previous work in this area (Huret et al., 2010) can’t be used. Setting biological parameters characterizing the focused organisms, is a key step when studying the hydrological connectivity and this work constitutes a first attempt for Dinophysis populations. These species are slow-growing dinoflagellates (Stolte and Garcés, 2006; Velo-Suarez et al., 2010b), nutritionally versatile to inherit the ability to photosynthesis (such species are klepto-chloroplastida, i. e. they use the chloroplasts of their preys to perform photosynthesis), many are obligate mixotrophs, and some are solely heterotrophic in nature (Hansen, 1991; Jacobson and Andersen, 1994; Kim et al., 2008). Due to these traits, cells are able to survive several months and their densities remain low compared to other plankton species in their size fraction. Thus, the duration of the particle advection was fixed to 3 months, without any mortality even if the particles spend a long period in aphotic deep waters. The main part of living protists biomass being located in near surface waters, because autotrophic cells (including Dinophysis prey) need sufficient light to perform photosynthesis, releasing cells in the mixed surface water layer was considered as a pragmatic methodology. It was also considered, as a first approximation and even if Dinophysis cells have some motility capacities (vertical migrations, gyrotaxis, …), that they are transported within water masses without any specific buoyancy, i.e. the particles buoyancy was assigned to neutral. Finally, assuming that Dinophysis populations spend their whole life cycle in the pelagic environment without an overwintering strategy, the simulation duration spanned two seasons: spring-summer (from April to August) and autumn-winter (October to February) to study the potential transport during contrasted hydrodynamical conditions.
2.2.2. Lagrangian Model
The position of the numerical particles during each time step was calculated using the off-line tool Ichthyop (Lett et al. (2008), https://www.ichthyop.org/) which is regularly used to describe fish larvae dispersion. Velocity, temperature, and salinity fields were obtained from hydrodynamic models as input time series. The process of particle dispersion by turbulent phenomena is perfectly explained and predicted by the Lagrangian modelling approach introduced by Taylor (1921). This approach was widely used for turbulent flows (e.g., (Pope, 1985; Pope, 1994; Pope, 2000; Mitarai et al., 2003). A total of 100 000 virtual particles were deployed (1 100 particles per day at a random initial time of the day) in the surface layer of each square station. Overall in this study, more than 8.106particles were used. The position of each particle was obtained by interpolating U (velocity fields) between grid points and integrating the following equation:
Here, Un(τ, a) is the velocity of the nth Lagrangian particle and Xn(τ, a) is the position of nth fluid particle. The particle velocity is related to the Eulerian flow at the particle location:
u(x,t) is the Eulerian velocity at a given location x and time t respectively, and tn is the release time of the nth Lagrangian particle. Noting that the transport of particles is based on 3D simulations, it is important to keep in mind that the calculated velocity fields are both in the horizontal and vertical directions (some trajectories of numerical particles are presented in Figure S1).
2.2.3. Velocity Fields
Ocean circulation from the Iberian Peninsula to Celtic sea (Figure 2) was generated with the state-of-the-art ocean general circulation model MARS3D. The MARS3D model uses a free surface primitive equation designed to describe hydrodynamics from regional to local scales. The sigma coordinates are used on the vertical dimension to resolve simultaneously shallow and deep waters. In our study, two different configurations of the MARS3D model in the Bay of Biscay (M4000 and M1000 with different resolutions and external forcing) were used to cover several decades.
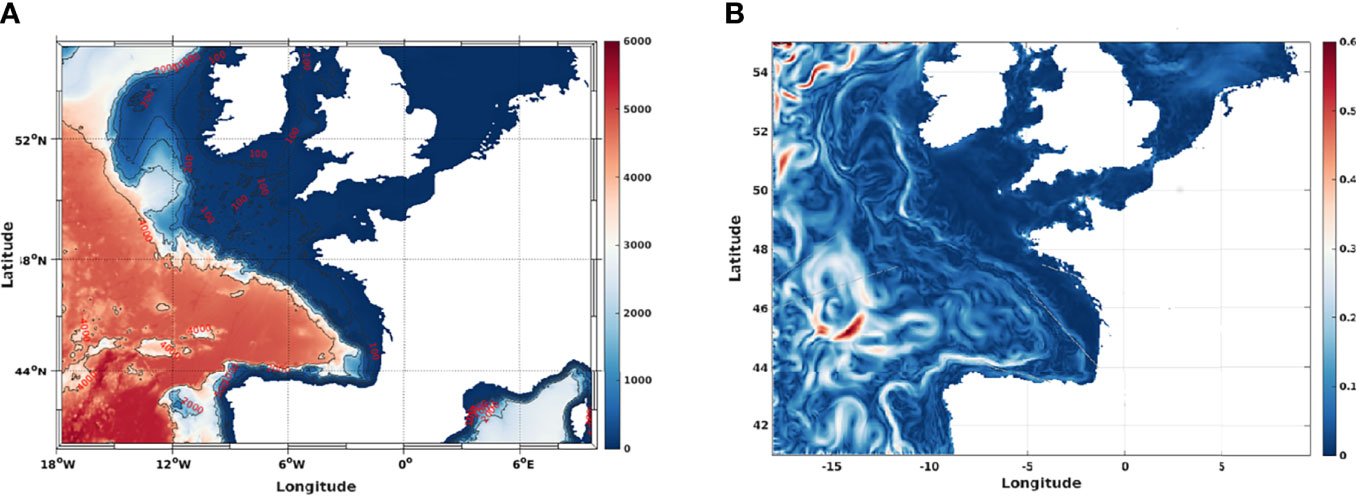
Figure 2 (A) Bathymetry of the modelled region, (B) Sample daily averaged surface current in the Bay of Biscay in the particle tracking modelling exercise.
The first configuration (M4000 i.e. MARS3D-MANGAE4000, 1958-2014) describes circulation in the Bay of Biscay and its extension to the western English Channel. It was already validated using hydrology analyses of the French continental shelf (Lazure et al., 2009). The horizontal resolution was of 4 km with 30 vertical levels in sigma coordinates and the domain covered from 4.68°E to 14.25°W, and 40.96°N to 52.48°N. The bathymetry was provided by the SHOM (Hydrological and Oceanographic Service of the French Navy). A resolution of 0.15m at the surface and 3.5 m in the middle of a 100m water column corresponded to the grid spacing in sigma coordinate. It should be noted that the period of simulation ranges from 1958 to 2014 and daily averaged outputs were used. The DFS4.3 [Drakkar Forcing Set; Brodeau et al. (2010)] model was used for atmospheric fields. This model was based on ERA40 ECMWF reanalysis (Uppala et al., 2005) from 1958 to 1989 and ERA-Interim ECMWF reanalysis (Dee et al., 2011) from 1989 to 2014. The turbulence closure scheme was based on the equation of the evolution of the turbulent kinetic energy (Gaspar et al., 1990). The global ORCA025 simulation, which was an application of the NEMO model (Madec et al., 1998), was used to provide data on temperature, salinity, SSH and currents at the open boundaries of the model as initial condition. Tides are important because they play a major role in the mixing of temperature and salinity at the shelf break, in the bottom friction over the shelf, and in the currents close to the coast. For our simulation, the FES2004 solution (Lyard et al., 2006) providing 14 tidal constituents (M2, S2, K2, N2, 2N2, O1, P1, K1,Q1, Mf, Mtm, Mm, Msqm and M4) were used. The second configuration (M1000 i.e. BACH1000_100lev Configuration, 2001-2010) also extended from the Bay of Biscay to the English Channel from 41.00 to 52.50°N, and from 14.30°W to 4.50°E but with a 1 km spatial horizontal resolution and a time step of 60 seconds (Theetten et al., 2017). This configuration had 1449 × 1282 grid points and used 100 vertical sigma levels. The outputs were also daily averaged. The bathymetry was a composite of several IFREMER digital terrain models (DTMs) and the interpolated topographies were smoothed using a local filter of below 0. which echoes the low value of 25 (Haidvogel and Beckmann, 1999). River runoffs were provided from 95 chronological records located on the Spanish, French, Dutch, British and Irish coasts. One of the major differences between the previous configuration was related to initial conditions for temperature, salinity, sea surface height and, baroclinic and barotropic velocities. In this configuration the initial conditions were derived from a DRAKKAR global configuration named ORCA12_L46-MJM88 (Molines et al., 2014). Another difference was the use of a sponge layer (Marchesiello et al., 2001) on the North, South and West boundaries. The same tide, with 14 components was imposed at the model boundaries, and the same atmospheric forcing as the M4000 were used. To estimate interannual variability of velocity fields, Lagrangian particle transport exercises were performed for 5 years (from 2010 to 2014).
2.2.4. Lagrangian Indices
Based on Lagrangian integration, different approaches have been used to quantitatively describe the connectivity between marine areas. These include Lagrangian probability density functions (PDFs) (Mitarai et al., 2009), graph theory (Rossi et al., 2014) and characteristic time scales associated with surface ocean connectivity (Jonsson and Watson, 2016). Most of these methods are based on the general definition of a “connectivity time,” which depends on oceanographic distances and is defined as the mean time required for particles to move from one location to another (Cowen et al., 2007; Mitarai et al., 2009). Jonsson and Watson (2016) proposed to use the “minimum connectivity time” (Tmin), defined as the fastest travel time from source to destination for numerical particles, inferred from a Dijkstra algorithm (Dijkstra, 1959). This minimum connection time also shows usually a better agreement and correspondence with genetic dispersal in marine connectivity Alberto et al. (2011). Following this idea, Costa et al. (2017) used graph theory and transfer probabilities to calculate “betweeness”, i.e., the shortest paths between different sites within variable ocean dynamics. The benefit of using the minimum connection time rather than the average transit time has been addressed in some empirical work (e.g., Doos (1995); Cowen et al. (2007); Mora (2012). Consequently, in our study, we chose to estimate the minimum, mean, median and most frequent value of the “minimum connectivity time” (, ,, and respectively) for all particles traveling from one station to another.
2.3. SNP Based Dinophysis Diversity
Due to the impossibility to consistently discriminate between several species by morphological criteria and usual molecular approach (Park et al., 2019; Séchet et al., 2021), a SNP approach was used due to its strong resolutive capacity down to the populations scale. Biological samples were sonicated on ice for 30 s in LBP buffer (Macherey-Nagel) and RNA extraction was performed using NucleoSpin ®RNA Plus kit (Macherey-Nagel) following the manufacturer’s protocol. Extracted RNA was quantified using a Biotek Epoch spectrophotometer and the quality estimated on RNA 6000 nanochips using a Bioanalyzer (Agilent). Library preparation was performed using the Illumina mRNA TruSeq stranded kit starting from 0.5 µg of total RNA. Library quality was assessed on a Bioanalyzer using high-sensitivity DNA analysis chips and quantified using Kappa Library Quantification Kit. Paired-end sequencing was performed using 2 x 150 bp cycles on Illumina Hiseq3000 at the GeT-PlaGe France Genomics sequencing platform (Toulouse, France). Read quality was assessed using FastQC (http://ww.w.bioinformatics.bbsrc.ac.uk/projects/fastqc/), and Trimmomatic Bolger et al. (2014); Metegnier et al. (2020) (V. 0.33) was used to trim ambiguous, low quality reads and sequencing adapters with parameters ILLUMINACLIP: Adapt.fasta: 2:30:10 LEADING: 3 TRAILING: 3 MAXINFO: 135: 0,8 MINLEN: 80. Two strategies were investigated to obtain Dinophysis reference transcriptomes from the 14 Dinophysis strains available in Table 1. (excluding the US strain). First one per species was obtained using Trinity Haas et al. (2013) by pooling the reads of the different strains belonging to the same species. Second, a single genus wide de novo assembly was obtained by pooling the reads from the 14 strains. Only transcripts longer than 200 bp were retained. When several isoforms were identified, only the longest one was retained. The reads from each strain were individually mapped to the seven reference transcriptomes using the BWA-MEM aligner Li (2013) and the percentage of remapping on each reference calculated. As the percentage of remapping on the genus wide reference was higher than 99% for all strains for a total size of 802 660 transcripts (to be compared to the sum of the six species specific reference transcriptomes: 1 921 084 transcripts), the genus wide reference transcriptome was selected for the analyses presented below. As described previously Metegnier et al. (2020), all the MMETSP reference transcriptomes Keeling et al. (2014) were downloaded from Cyverse (http://www.cyverse.org). All references corresponding to unknown species were discarded. The reference corresponding to Dinophysis acuminata reference was replaced by the one obtained from the present study. Within each transcriptome, for each transcript, only the longest isoform was considered. When several transcriptomes per species were available (several strains or culture conditions), they were merged into a single reference, and CD-HIT-EST Li and Godzik (2006) was used to remove homologous sequences within each reference. A total of 313 species specific reference transcriptomes, representing 213 unique genus, were considered and concatenated to build the meta-reference. Reads from the 14 Dinophysis strains described above as well as from another D. acuminata strain obtained from the MMETSP database were aligned to the genus wide Dinophysis reference transcriptome. Reads from the 120 meta-transcriptomic datasets were aligned to the meta-reference. Alignments were performed using the BWA-MEM aligner Li (2013). Samtools Li and Godzik (2006) was used to discard reads displaying low quality alignments (MapQ<10) as well as the ones mapping to several transcripts (FLAGS < 1,000). For each meta-transcriptomic dataset, the relative abundance of the transcripts for each gene in each sample was calculated Metegnier et al. (2020). SNPs from the strains were identified using Freebayes (Garrison and Marth, 2012) as described in Le Gac et al. (2016). VCFTOOLS (Danecek et al., 2011) was used to keep SNPs with two alleles, a quality criterion higher than 40, and covered more than 10 times in each strain. It corresponded to a total of 296,887 SNPs. Nucleotide divergence was calculated for each pair of strains as the number of variant sites divided by the total number of sites covered more than ten times in each strain. Strains were clustered based on nucleotide divergence using hclust in R. The allelic frequencies of the natural populations based on the meta-transcriptomic samples were obtained using FreeBayes (Garrison and Marth, 2012) enforcing diploidy, and extracting coverage for each of the two alleles at the various SNP positions identified in the strain datasets. PLINK (Purcell et al., 2007) was used to prune SNPs displaying strong linkage disequilibrium (10 kb windows, 10 bp window step size, 0.1 r² threshold), leaving 73,318 SNPs after pruning. These SNPs were used to perform a PCA analysis as a first exploratory investigation of the Dinophysis community composition in the meta-transcriptomic samples. Median proportion at diagnostic SNPs, i.e. SNPs displaying a given allele in all strains from a given species and the alternative allele in all the other strains, were used to estimate the relative proportion of the Dinophysis species in the in-situ samples.
3 Results
3.1. Hydrodynamic Connectivity
In order to avoid redundant information, some correlations between the Lagrangian indices ( , ,, and ) were performed. As expected, the indices were highly correlated and only the index showing the highest correlations was thus presented hereafter (, see Table 2). In the same way, in order to describe the maximum inter-annual variability of connectivity patterns, correlations for between years were performed and the years showing the most different patterns were retained (years 2011 and 2013, Table 3). This simple analysis also showed the high stability of the connectivity patterns in spring an autumn (Figure 3) but also that inter-annual variability appeared as global trend affecting all stations in a similar way (by increasing or reducing the connectivity times due to a more energetic circulation). For the sake of simplification, the global seasonal patterns are thus described hereafter based on the network based on for 2011.

Table 2 Correlation between the transit time indices from all years (the mean, median, most frequent value and minimum, of the “minimum connectivity time”: ,, and and respectively).
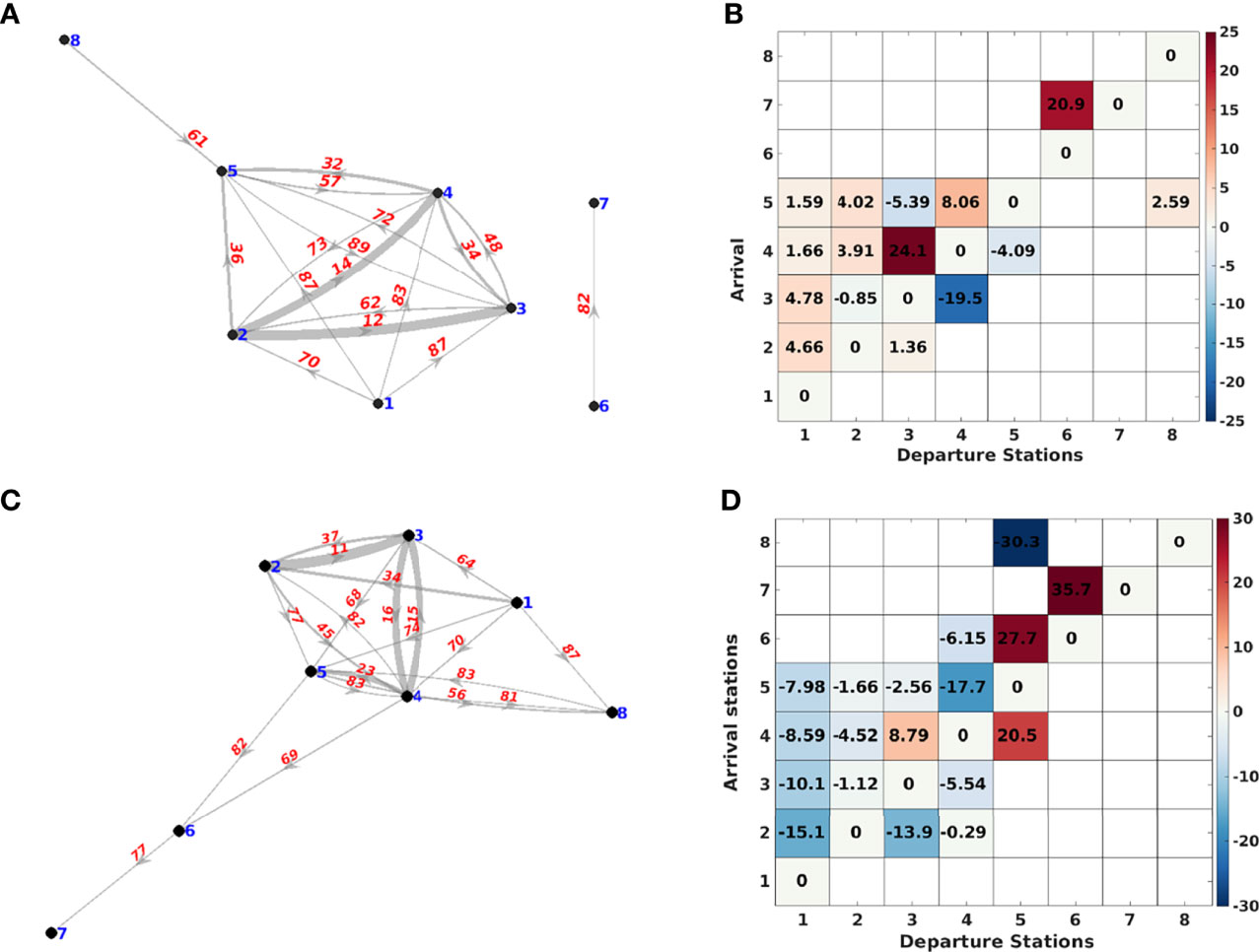
Figure 3 (A) Dinophysis connectivity network showing the mean “min transit time” (days) between the departure stations and the arrival stations in spring 2011, (B) Difference between the “mean transit time” values during spring 2011, 2013 (2011-2013), (C) Dinophysis connectivity network showing the “mean transit time” (days) between the departure stations and the arrival stations in winter 2011, (D) Difference between the “mean transit time” values during winter 2011, 2013 (2011-2013). A graphical representation of connectivity times keeping geographical distances between node is illustrated in Figure S4.
During the spring-summer (April to August) period, the water masses from English Channel and North Sea appeared not connected to other areas with our setting, and the Celtic Sea weakly connected to the Bay of Biscay and Iberian coast. A of approximately 88 days was calculated for particles traveling in a northward direction from Galicia (Station 1) to the English Channel entrance (Station 5). A duration longer than 3 months is thus required for cells to go further north and to reach Celtic Sea. A global northward advection along the coast was shown, with short calculated between coastal waters of the north Iberian shelf (station 2) and stations 3, 4 and 5. The fastest transit pathway for particles in spring-summer, was found between station 2 and 3 with estimated to be 12 and 11 days in 2011 and 2013 respectively (Figures 3A, B). It is even quicker during the winter period with between these two stations being 11 and 10 days in 2011 and 2013 respectively (Figures 3C, D). Several transit are also unidirectional. Particles released in Galician waters and North-West Spain (stations 1 and 2) travelled towards stations in the Bay of Biscay while a significant reverse connectivity flow was not observed (Figure 3). In the same idea, the from station 4 to station 5 is estimated to be around 32 days in summer 2011 (respectively 23 days in winter), while the inverse particle movement can take almost twice (respectively four times longer in winter). As a general pattern, simulations show the particles were transported on further distances during the autumn-winter period compared to spring-summer ((Figures 3A, C). It is illustrated by the increase of the number of edge per node in the winter. Stations 6 and 7 are also connected to the other stations. The fastest connectivity pathways calculated were however roughly the same than during the summer in the Bay of Biscay. All the described patterns shown a low inter-annual variability with some differences shorter than a week in many cases. The water masses from the Bay of Biscay appear thus strongly connected with a general circulation oriented slightly Northward.
To analyze the results consistency, a comparison was made between two models (Figure 4) with different model resolution and configuration. The 4 km resolution flow fields are more energetic than in the 1 km resolution model, whatever the considered year. Implicitly, some lower transit time were expected but this dynamic also leads to the generation of a large number of (sub) mesoscale eddies (cyclonic and anticyclonic) in the centre of Bay of Biscay (out of the shelf) which can greatly influence the particles retention. The presence of eddies, resulting from the continental margin current instabilities interacting with the bottom topography, is also more frequent with the 4 km resolution model. These dynamic conditions indeed reduce the calculated transit times for any Dinophysis cells present in these currents (Figure 5). These differences are however moderate because it affects mainly the movement of numerical particles in the central (oceanic) part of the Bay of Biscay. Over the shelf, the main ocean circulation drivers remains wind and density gradients caused by rivers plumes.
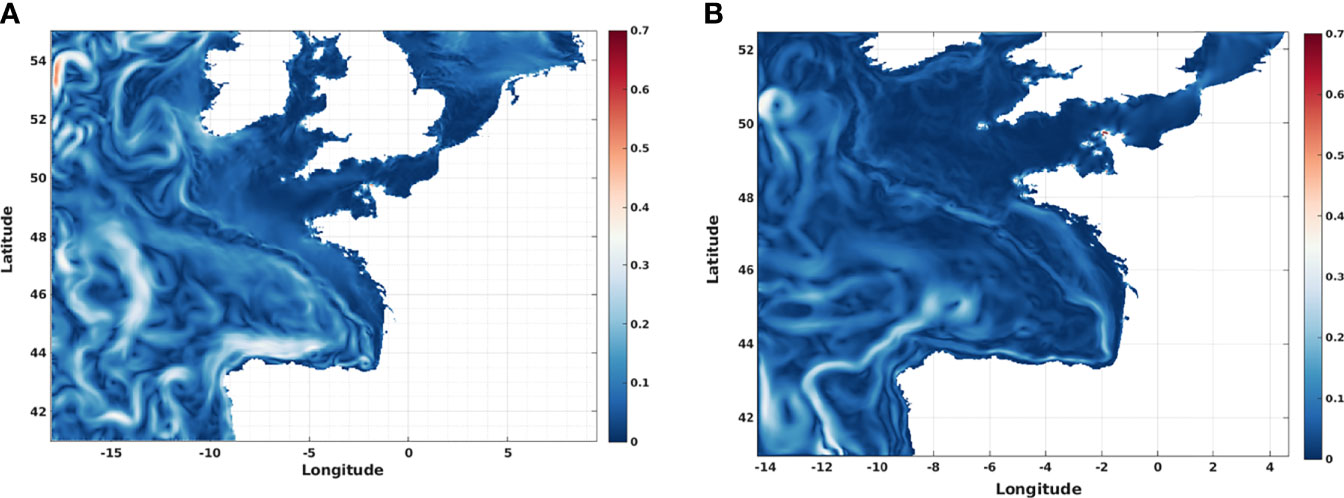
Figure 4 Sample surface flow fields of the hydrodynamical models in November 2010. (A) The 4 km horizontal resolution model and (B) The 1 km horizontal resolution model.
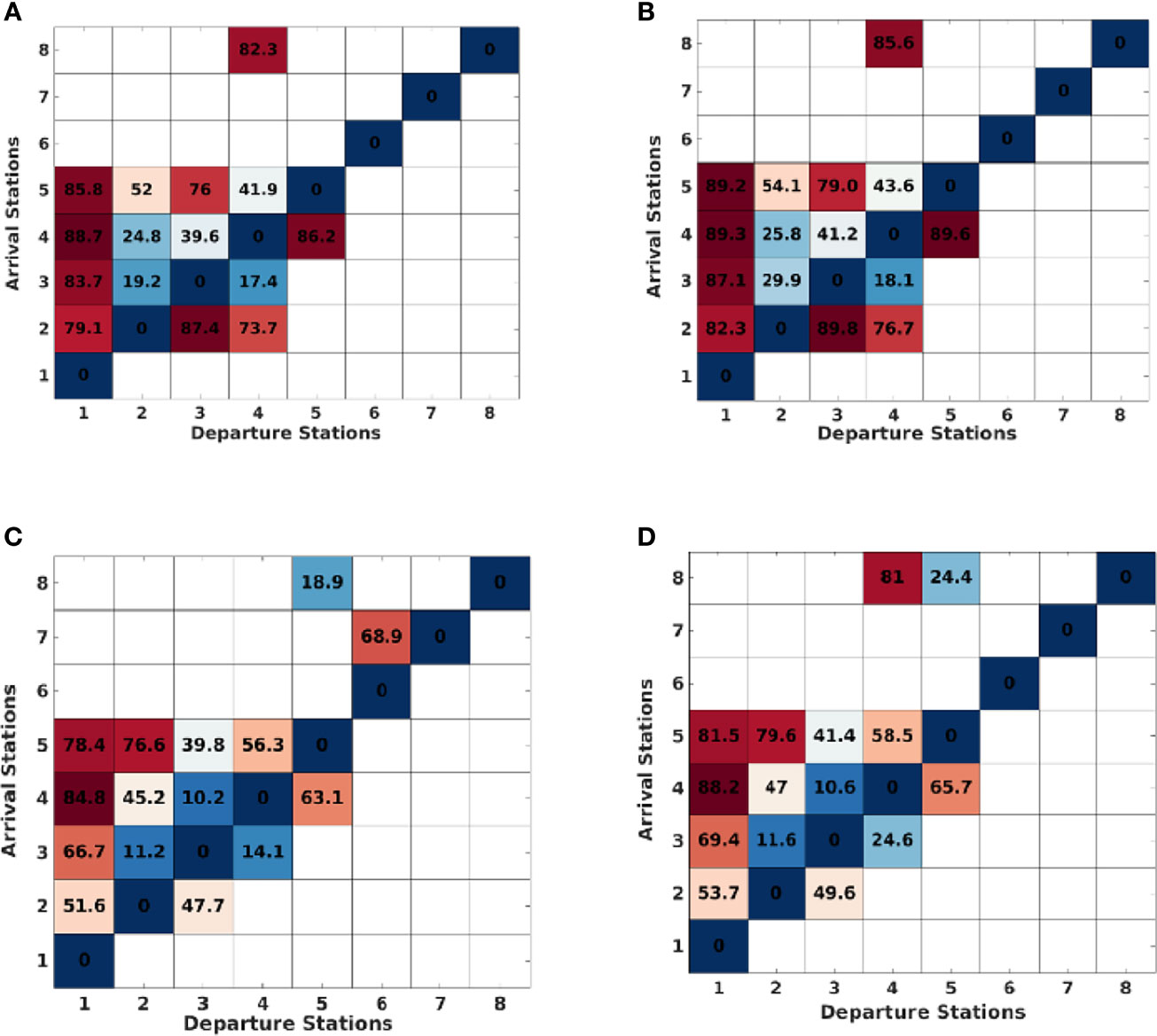
Figure 5 Dinophysis connectivity matrix showing the “mean transit time” (days) in spring 2010, (A) Model resolution = 4km, (B) Model resolution = 1km, and in winter 2010, (C) Model resolution = 4km, (D) Model resolution = 1km.
3.2. Dinophysis Community
SNP analyses of the fifteen Dinophysis strains belonging to six different species enabled a clear distinction among the six species (Figure 6). Three strongly divergent species pairs were identified. The two species D. acuminata and D. sacculus, that are difficult to distinguish morphologically or by using regular genetic markers Séchet et al. (2021), displayed low levels of nucleotide divergence (< 0.0025, corresponding to ≈ 20 000 SNPs displaying genetic differences among strains of the two species). Two other species, D. acuta and D. fortii were even less divergent (< 0.0012, ≈ 11 000 SNPs). The two other sister species D. caudata and D. tripos displayed a higher level of nucleotide divergence level around 0.007 (≈ 60 000 SNPs). Within each of the four species represented by at least two strains, the level of intraspecific variability was extremely low, with a nucleotide divergence of 2.6 10-6 (20 SNPs) and 2.96 10.-6 (30 SNPs) within D. acuta and D. caudata, respectively. Between the three european D. acuminata, nucleotide divergence was around 5 10-6 (< 100 SNPs), but raised to 1.4 10-3 (≈ 13 000 SNPs) between the European and American strains (on the same order scale than the observed inter-specific differences). Finally, we note a genetic divergence of 2 10-4 (≈ 1 500 SNPs) between the two D. sacculus strains from the Mediterranean sea (D15 and D17) and the two D. sacculus strains from the Atlantic ocean (D13 and D14), while nucleotide divergence was 10-4 and 2 10-7 among strains from the Mediterranean sea and the Atlantic ocean, respectively. This very low level of intraspecific variability precluded the analysis of genetic structure within each species but the identification of species specific SNPs enabled us to investigate the relative proportion of the various species in the in-situ samples from Galicia waters to the entrance of the Channel.
Following Metegnier et al. (2020), environmental reads obtained from 120 in-situ samples were aligned to a metareference database composed of 313 species specific reference transcriptomes (Figure S2). A large proportion of environmental reads did not align to the metareference (20 to 80%), probably because several species abundant in the natural samples were not represented in the metareference database. Dinophysis genus, which is assumed to be oceanic, only represented a very small proportion of environmental reads (< 5%) despite the differential filtration used and the samples pre-selection based on microscopic observations. This observation was in agreement with the microscopic observations of our samples. Despite the significant presence of Dinophysis cells, they were never the most abundant fraction of the samples. The analysis was thus restricted to 59 environmental samples with more than 20 000 reads aligning to the Dinophysis reference transcriptome. Samples corresponded to samples from the Bay of Brest (prefix label: RB), Bay of Vigo (prefix label: Remedios), Bay of Arcachon (prefix label: Arcachon), Basque waters (prefix label: Azti), Iroise Sea (prefix label: Mascoet) and waters over the continental shelf in the Bay of Biscay (prefix label: Pelgas). The relative abundance of the resolved Dinophysis species across natural populations can be compared with the strain genotypes inferred above (Figure 7). The Bay of Brest and Vigo samples are mainly composed of the D. acuminata/D. sacculus complex (not resolved using this graph). D. acuta, caudata and fortii were never the dominant among Dinophysis community. Few samples from the continental shelf and Basque waters had a significant fraction of D. tripos. To improve the taxonomic resolution of our approach, the analysis was restricted to the SNPs diagnostic of the various species, only considering the 15 in-situ samples with more than 5 diagnostic SNPs for each species each covered by more than 10 reads (Figure 8). It indicated clearly that the two Dinophysis species from our cultivated set often co-occured in the natural communities from Galicia to the Bay of Biscay. D. acuminata is often well represented, except in a few samples where D. tripos tends to dominate. Due to the low number of samples, the analysis of specific environmental factors was not realised. A significant fraction of one or several Dinophysis species, which were not included in our reference transcriptome, is also observed. The species composition tends to vary at the same locality, and there is no clear geographical pattern of co-occurence.
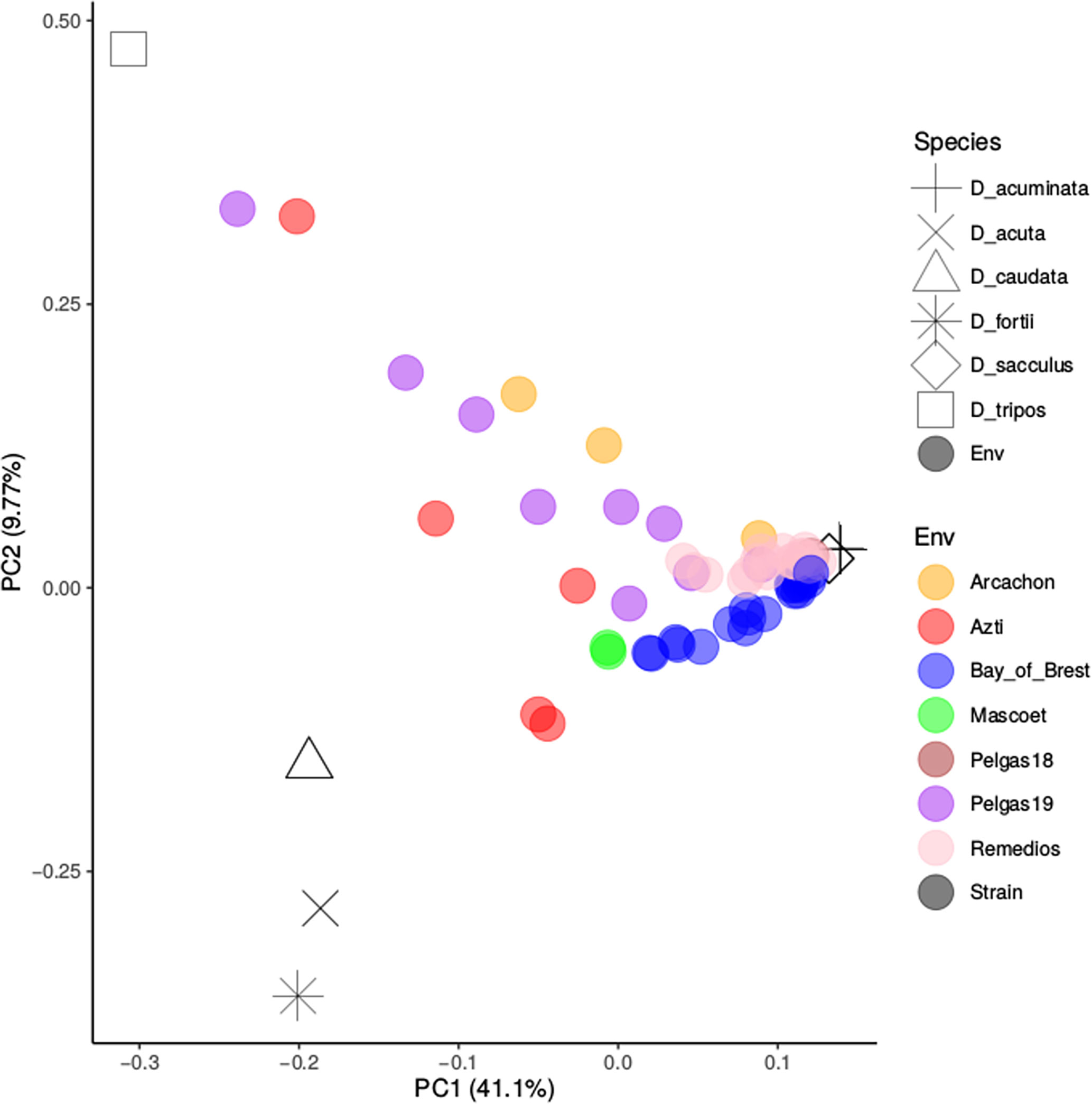
Figure 7 PCA analysis of species composition in Dinophysis spp. populations. The in-situ samples are represented by colored dots and the strains by black symbols.
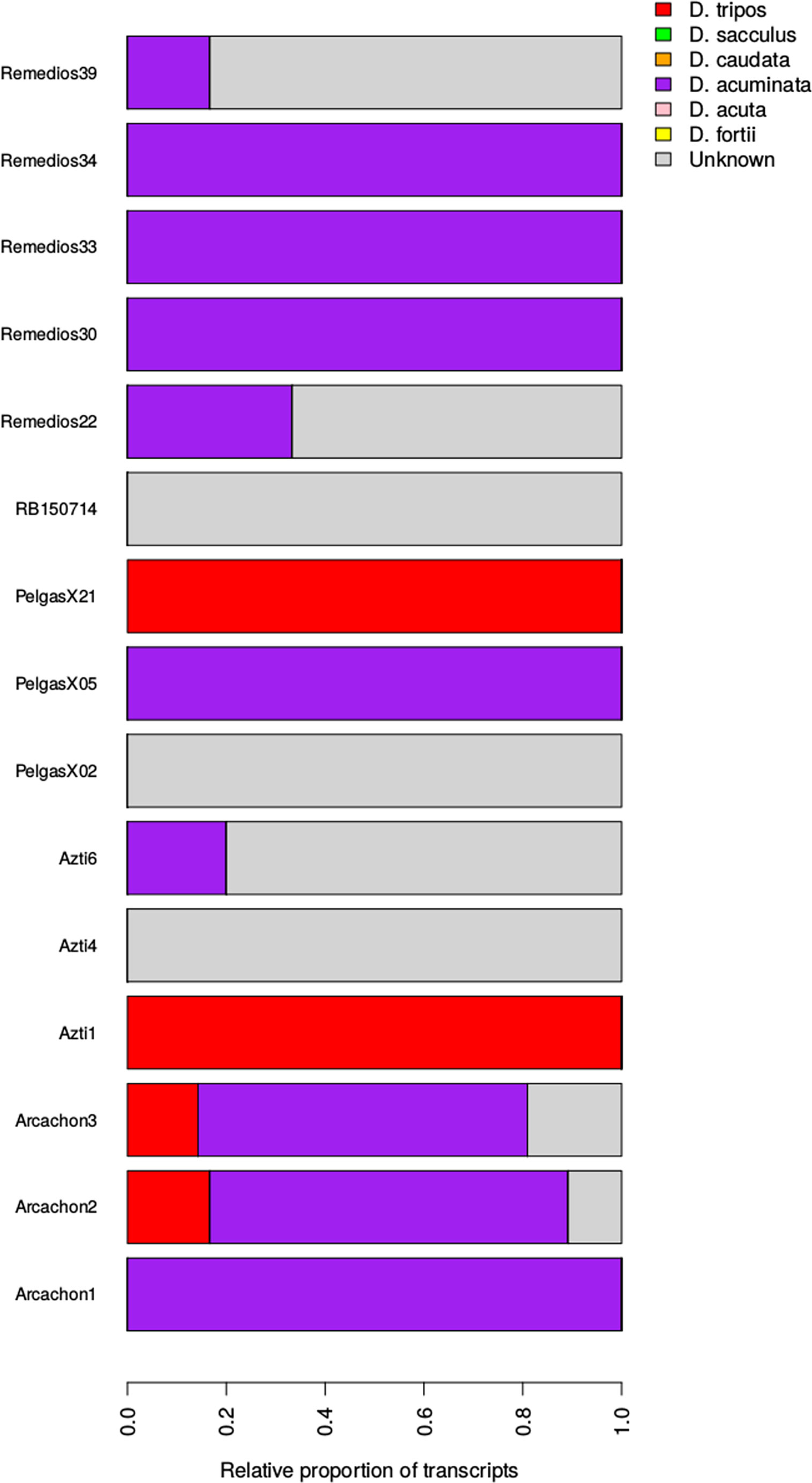
Figure 8 Relative proportion of Dinophysis species based on diagnostic SNPs at the species level (7 taxonomic groups).
4 Discussions
From all hydrodynamics configurations, the connectivity pattern described in autumn-winter is in agreement with the general cyclonic circulation previously reported for the region Charria et al. (2017). It is mostly influenced by the eastward Iberian Poleward Current in deep ocean Le Cann and Serpette (2009) and by the northwestward current over the continental shelf Lazure et al. (2008). At the annual scale, without any specific behaviour and selection, populations should be well mixed at the European scale involving potential migrations between all water masses over the shelf. Reversely during the summer, connectivities indicate that the English Channel and the North Sea seems seasonally isolated from the other areas. During the summer, the advection of some blooms occurring over the Atlantic Shelf to the English channel is thus unlikely. An environmental selection should lead to a species composition different from those observed in the Bay of Biscay. D. norvegica could illustrate such a process with some observations typically distributed in northerly waters of the Atlantic (Bresnan et al., 2021). Unfortunately, this assumption cannot be verified because we were not able to sampled this northern part of the coast and no reference transcriptome of this species was available. We must also indicate that the porosity of this barrier is probably underestimated by the Lagrangian methodology that assumed only three limited areas for particle departure in the Bay of Biscay. By increasing their sizes or slightly change their positions, a small connection could appear over the summer. This boundary is then only a seasonal semi-permeable barrier.
Reversely, all the water masses from the Bay of Biscay are well connected (high number of edges per nodes with some exchanges in both directions) and this result helps in the analysis of the observed species composition. Despite the limitation associated to the low number of reads from in-situ samples, probably due to the large part of the other plankton community also retained on the filters, only two identified species were detected in the Bay of Biscay without any spatial pattern. A dominance of D. acuminata is observed over the Bay of Biscay but D. tripos can also constitute a significant fraction of the community (over the French shelf and along the Basque country). The variability of the relative abundances thus appears more related to some local dynamics or to a patchy distribution of cells. The unknown fraction (Figures 8, S3) is due to one or several Dinophysis species without reference transcriptome. From microscopic observation, some round cells were observed in our samples. Due to the regular observations of Phalacroma rotundatum or D. ovum in the area (Reguera et al., 2014), they represent probably a large fraction of this unknown group. It should be noted that there was no specific strategy (period, geographical position, etc…) for conducting the in-situ sampling, which can be considered as random. The species absence could be bias by the relatively “small” number of retained samples (15) but it should also indicates the global trend. A second analyse was thus made to increase the number of retained samples (47) by using a lower taxonomic resolution (Figure S3). The same trend was highlighted with a dominance D. acuminata and only one detection of D. acuta/fortii in Basque waters. At the species level, the absence of detection for D. sacculus in all our samples is finally surprising because it was relatively easily isolated and cultivated from French coastal waters. This observation raises two questions. The first one is the porosity used for the filtration but, to be meaningful, almost all of the cells of this species must be lower than (20 µm). The second question is a coastal adaptation for this species, because with some high migration and invasion capacities along the coast, all the six species could grow in this area when favorable conditions would be encountered. These high potential migration rates also shown that to describe ecological niches at a species level (i.e. to understand species biogeography and to forecast some scenarii) implementing monitoring programs with an higher taxonomic resolution is highly required.
Connectivity between Galicia (Rias) and the South of the Bay of Biscay is mostly impacted by the general oceanic circulation and the continental slope current. Weak anticyclonic (clockwise) circulation can become cyclonic (anticlockwise) close to the continental margin but the populations flow, at the seasonal scale, appears unidirectional from Galacia waters to the Bay of Biscay. In the same way, we simulated rather short travel times from Asturias waters (station 2) towards the Arcachon area (station 3) than in the opposite direction. This pattern during the spring-summer is slightly different from the described general circulation. Summer circulation of the surface layer is considered as anticyclonic (southward over the abyssal plain and along the Basque coast, Valencia et al. (2004)) or weak and highly variable by being windy driven over the French shelf Charria et al. (2017). Here, particles tend to move northward even if inverse movement remains possible. This observations is likely related to the vertical mixing that brings some cells below surface layers where advection processes can be different. Coastal jets, which refer in the North hemisphere to alongshore flows caused by the horizontal density gradient made by tidal mixing, is a such process occurring at depth of the neighbouring pycnocline where highest cell densities are very often observed and with a northward direction. This process can occurred over the French shelf, as observed in Celtic Sea Raine (2014), and leads to a northward advection, regardless of the weather conditions. Some other and fast windy driven processes also occurs in this area such as western winds leading to a fast (up to 19 cm.s-1) northward advection circulation Batifoulier et al. (2013) but these processes are not resolved with our model configurations and the position of our releasing stations.
The relatively long connectivity time (2-3 months) from Galicia to the Bay of Biscay also indicates that the positive correlation of the inter-annual variability between cell abundances monitored in these two areas Diaz et al. (2013) is more related to favorable environmental conditions to Dinophysis spp. at large scale than to population advection. From Galicia (Remedios samples) to the entrance of the English Channel (Bay of Brest, RB), D. acuminata appears as dominant, followed by the unknown species group and several observation of D. tripos and D. acuta/fortii. There is, once again, no northward trend in the community composition from Galicia to the English Channel. Moreover, due to the low genetic variability observed at the European scale it was not possible to analyze the intra-specific variability. However, some significant differences between D. acuminata strains isolated over different years and from North America and Europe seems to indicate the existence of biogeographic patterns at the global scale. This very low genetic variability is thus significant and highlights some unexpected properties that echo the Lewontin’s paradox (Filatov, 2019).
As indicated previously for the summer barrier, a possible drawback of our methodology is its sensitivity to the setting of the particles parameters. For example, the highest cell concentrations of Dinophysis spp. are regularly observed at marked density gradients, related to a sudden vertical change in temperature and/or salinity in the water column Maestrini S. Y. (1998). Dinophysis blooms (when cell densities become significant in the whole sampled community) are thus frequently observed in species-specific horizontal thin layers related to physical shear and vertical swimming behavior (Moita et al., 2006; Velo-Suarez et al., 2010a). These distributions could have an impact on the populations advection over short time scales. Nonetheless, due to their presence at low density in the other layers and the transport duration longer (three month here) than the life duration of these thin layers, it is unlikely that some significant differences in the described seasonal patterns of connectivity time could be expected. The last uncertainty is related to the settings of the hydrodynamic configurations. Unresolved mixing processes can change significantly the simulated movement of particles (McWilliams, 2016; )?. By increasing the horizontal resolution, connectivity times should decrease and the average number of edge per node in the connectivity network increase. In our case, these effects are however strongly masked by the more energetic circulation simulated with the coarser resolution. As a reminder, apart from the horizontal resolution, boundaries conditions and vertical resolution were the main differences. Of these two differences, the lower vertical resolution of the 4km configuration appears as the main driver of the energy difference by creating an overestimation of the vertical mixing along strong bathymetry gradient (slopes), which is a regular problem for models using a sigma grid. Particles remaining in the surface water layer or in a subsurface layer move then more rapidly in the coarse model configuration due to (sub) mesoscale eddies generated by continental slope currents in the Bay of Biscay. Nevertheless, the same general patterns are conserved in both configurations with: a confirmed simulated northward connectivity from Galicia to the Bay of Biscay, a high connectivity within the Bay of Biscay and a higher connectivity between shelf waters of French Brittany and Celtic Sea shelf sea than from shelf waters of French Brittany and English Channel and North Sea. According to the network stability over a decade from different configurations, we tried to evaluate it over a longer period by reproducing this methodology with some velocity fields from other scenarios [from 1975 to 2100, POLCOMS-ERSEM on the Northeast Atlantic, Kay et al. (2018)]. This investigation was however dismissed due to the differences between the hydrodynamic solutions used in this work and those provided.
5 Conclusions
This study allowed us to characterise the main connectivity patterns along the European northeast Atlantic coast for water masses with some Dinophysis populations, and its stability during the last decade. At the populations scale, some mixing of Dinophysis populations from the Western Iberian shelf, the Bay of Biscay and the Celtic Sea are likely with a northward trend (especially in the Celtic Sea) throughout the year. A significant boundary, that could limit biological flows during the summer, occurs at the entrance of the English Channel. The study also points out the interest of following the specific dynamics because, if nowadays a strong dominance of Dinophysis acuminata is observed, all species can migrate and bloom all along the European coast. Despite the unsolved problem of low proportions for Dinophysis cells in the sample, this work provides a methodology in that way with some references sequences to clearly identify and describe the species dynamics.
Data Availability Statement
Sequencing reads were deposited to the European Nucleotide Archive and are accessible under the accessions PRJEB53578 (metaT reads) and PRJEB53438 (Dinophysis RNAseq reads).
Author Contributions
SH, MS, MP, and ML contributed to the initial idea, data analysis, and the manuscript writing. VS, and MR contributed to the cultures and water sampling. All authors contributed to the article and approved the submitted version.
Funding
This study was funded by PRIMROSE (EC Interreg Atlantic Area EAPA 182/2016). MR was funded by the Basque Government (ES), through the project “BIOTOX” from the European Maritime and Fisheries Fund (EMFF-00002-INA2021-33). SH was also funded by the project CoCliME which is part of ERA4CS, an ERA-NET initiated by JPI Climate, and funded by EPA (IE), ANR (FR), BMBF (DE), UEFISCDI (RO), RCN (NO), and FORMAS (SE), with co-funding by the European Union (Grant 690462).
Conflict of Interest
The authors declare that the research was conducted in the absence of any commercial or financial relationships that could be construed as a potential conflict of interest.
Publisher’s Note
All claims expressed in this article are solely those of the authors and do not necessarily represent those of their affiliated organizations, or those of the publisher, the editors and the reviewers. Any product that may be evaluated in this article, or claim that may be made by its manufacturer, is not guaranteed or endorsed by the publisher.
Acknowledgments
We thank Rodriguez Francisco, Pilar Rial (IEO, Vigo) and Liliane Carpentier (IFREMER, Nantes) for the isolation and source of biological material belonging to Dinophysis strains from NW Spain and France. We also thank Marivi Lucero (AZTI), Pascale Malestroit and Marie-Madeleine Danielou (IFREMER) for their contributions to the sampling and samples selection.
Supplementary Material
The Supplementary Material for this article can be found online at: https://www.frontiersin.org/articles/10.3389/fmars.2022.914909/full#supplementary-material
References
Alberto F., Raimondi P., Reed D., Coelho N., Leblois R., Whitmer A., et al. (2011). Isolation by Oceanographic Distance Explains Genetic Structure for Macrocystis Pyrifera in the Santa Barbara Channel. Mol. Ecol. 20, 2543–2554. doi: 10.1111/j.1365-294X.2011.05117.x
Bass Becking L. (1934). Geobiologie of Inleiding Tot De Milieukunde (The Hague, The Netherlands: W. P. Van Stockum & Zoon).
Batifoulier F., Lazure P., Velo-Suarez L., Maurer D., Bonneton P., Charria G., et al. (2013). Distribution of Dinophysis Species in the Bay of Biscay and Possible Transport Pathways to Arcachon Bay. J. Mar. Syst., 109–110. doi: 10.1016/j.jmarsys.2011.12.007
Blanke B., Raynaud S. (1997). Kinematics of the Pacific Equatorial Undercurrent: An Eulerian and Lagrangian Approach From GCM Results. J. Phys. Oceanog. 27, 1038–1053. doi: 10.1175/1520-0485
Bolger A. M., Lohse M., Usadel B. (2014). Trimmomatic: A Flexible Trimmer for Illumina Sequence Data. Bioinformatics 30, 2114–2120. doi: 10.1093/bioinformatics/btu170
Bravo I. I., Reguera B. B., Fraga S. S. (1995). Description of Different Morphotypes of D. Acuminata Complex in the Galician Rias Bajas in 1991 (Lavoisier Publishing Inc).
Bresnan E., Arévalo F., Belin C., Branco M. A. C., Cembella A. D., Clarke D., et al. (2021). Diversity and Regional Distribution of Harmful Algal Events Along the Atlantic Margin of Europe. Harm. Algae. 102, 101976. doi: 10.1016/j.hal.2021.101976
Brodeau L., Barnier B., Tréguier A.-M., Penduff T., Gulev S. K. (2010). An ERA40-Based Atmospheric Forcing for Global Ocean Circulation Models. Ocean. Model. 31, 88–104. doi: 10.1016/j.ocemod.2009.10.005
Casteleyn G. (2010). Itlimits to Gene Flow in a Cosmopolitan Marine Planktonic Diatom. Proc. Natl. Acad. Sci. U.S.A. 107, 12952–12957. doi: 10.1073/pnas.1001380107
Charria G., Theetten S., Vandermeirsch F., Yelekci O., Audiffren N. (2017). Interannual Evolution of (Sub)Mesoscale Dynamics in the Bay of Biscay. Ocean. Sci. 13, 777–797. doi: 10.5194/os-13-777-2017
Costa A., Petrenko A., Guizien K., Doglioli A. (2017). On the Calculation of Betweenness Centrality in Marine Connectivity Studies Using Transfer Probabilities. PLos One 12, 0189021. doi: 10.1371/journal.pone.0189021
Cowen R., Gawarkiewicz G., Pineda J., Thorrold S., Werner F. (2007). Enpopulation Connectivity in Marine Systems. Oceanography 20, 14–20. doi: 10.5670/oceanog.2007.26
Danecek P., Auton A., Abecasis G., Albers C. A., Banks E., DePristo M. A., et al. (2011). Engthe Variant Call Format and VCFtools. Bioinf. (Oxford. England). 27, 2156–2158. doi: 10.1093/bioinformatics/btr330
Dee D. P., Uppala S. M., Simmons A. J., Berrisford P., Poli P., Kobayashi S., et al. (2011). the ERA-Interim Reanalysis: Configuration and Performance of the Data Assimilation System. Q. J. R. Meteorol. Soc. 137, 553–597. doi: 10.1002/qj.828
Delmas D., Herbland A., Maestrini S. (1992). Environmental-Conditions Which Lead to Increase in Cell-Density of the Toxic Dinoflagellates Dinophysis Spp in Nutrient-Rich and Nutrient-Poor Waters of the French Atlantic Coast. Mar. Ecol. Prog. Ser. 89, 53–61. doi: 10.3354/meps089053
de Wit R., Bouvier T. (2006). ‘Everything Is Everywhere, But, the Environment Selects’; What Did Baas Becking and Beijerinck Really Say? Environ. Microbiol. 8, 755–758. doi: 10.1111/j.1462-2920.2006.01017.x
Diaz P. A., Reguera B., Ruiz-Villarreal M., Pazos Y., Velo-Suarez L., Berger H., et al. (2013). Climate Variability and Oceanographic Settings Associated With Interannual Variability in the Initiation of Dinophysis Acuminata Blooms. Mar. Drugs 11, 2964–2981. doi: 10.3390/md11082964
Dijkstra E. W. (1959). A Note on Two Problems in Connexion With Graphs. Numer. Math. 1, 269–271. doi: 10.1007/BF01386390
Doos K. (1995). Inter Ocean Exchange of Water Masses. J. Geophys. Res. Ocean. 100, 13499–13514. doi: 10.1029/95jc00337
Farrell H., Gentien P., Fernand L., Lunven M., Reguera B., González-Gil S., et al. (2012). Scales Characterising a High Density Thin Layer of Dinophysis Acuta Ehrenberg and Its Transport Within a Coastal Jet. Harm. Algae. 1536–46. doi: 10.1016/j.hal.2011.11.003
Fenchel T., Finlay B. (2004). The Ubiquity of Small Species: Patterns of Local and Global Diversity. Bioscience 54, 777–784. doi: 10.1641/0006-3568(2004)054[0777:TUOSSP]2.0.CO;2
Filatov D. A. (2019). Extreme Lewontin’s Paradox in Ubiquitous Marine Phytoplankton Species. Mol. Biol. Evol. 36, 4–14. doi: 10.1093/molbev/msy195
Garrison E., Marth G. (2012). Haplotype-Based Variant Detection From Short-Read Sequencing. arXiv:1207.3907, ArXiv: 1207.3907.
Gaspar P., Grégoris Y., Lefevre J.-M. (1990). A Simple Eddy Kinetic Energy Model for Simulations of the Oceanic Vertical Mixing: Tests at Station Papa and Long-Term Upper Ocean Study Site. J. Geophys. Res.: Ocean. 95, 16179–16193. doi: 10.1029/JC095iC09p16179
Godhe A., Egardt J., Kleinhans D., Sundqvist L., Hordoir R., Jonsson P. R. (2013). Seascape Analysis Reveals Regional Gene Flow Patterns Among Populations of a Marine Planktonic Diatom. Proc. R. Soc. B.: Biol. Sci. 280, 20131599. doi: 10.1098/rspb.2013.1599
Haas B. J., Papanicolaou A., Yassour M., Grabherr M., Blood P. D., Bowden J., et al. (2013). engDe Novo Transcript Sequence Reconstruction From RNA-Seq Using the Trinity Platform for Reference Generation and Analysis. Nat. Protoc. 8, 1494–1512. doi: 10.1038/nprot.2013.084
Hansen P. (1991). DXinophysis - a Planktonic Dinoflagellate Genus Which can Act Both as a Prey and a Predator of a Ciliate. Mar. Ecol. Prog. Ser. 69, 201–204. doi: 10.3354/meps069201
Huret M., Petitgas P., Woillez M. (2010). Dispersal Kernels and Their Drivers Captured With a Hydrodynamic Model and Spatial Indices: A Case Study on Anchovy (Engraulis Encrasicolus) Early Life Stages in the Bay of Biscay. Prog. Oceanog. 87, 6–17. doi: 10.1016/j.pocean.2010.09.023
Jacobson D., Andersen R. (1994). The Discovery of Mixotrophy in Photosynthetic Species of Dinophysis (Dinophyceae) - Light and Electron-Microscopic Observations of Food Vacuoles in Dinophysis-Acuminata, D-Norvegica and 2 Heterotrophic Dinophysoid Dinoflagellates. Phycologia 33, 97–110. doi: 10.2216/i0031-8884-33-2-97.1
Jensen M. H., Daugbjerg N. (2009). Molecular Phylogeny of Selected Species of the Order Dinophysiales (Dinophyceae)—Testing the Hypothesis of a Dinophysioid Radiation1. J. Phycol. 45, 1136–1152. doi: 10.1111/j.1529-8817.2009.00741.x
Jonsson B., Watson J. (2016). The Timescales of Global Surface- Ocean Connectivity. Nat. Commun. 7, 1-6. doi: 10.1038/ncomms11239
Kamiyama T., Nagai S., Suzuki T., Miyamura K. (2010). Effect of Temperature on Production of Okadaic Acid, Dinophysistoxin-1, and Pectenotoxin-2 by Dinophysis Acuminata in Culture Experiments. Aquat. Microb. Ecol. 60, 193–202. doi: 10.3354/ame01419
Kay S., Andersson H., Catalán I. A., Drinkwater K. F., Eilola K., Jordà G., et al. (2018). Projections of Physical and Biogeochemical Parameters and Habitat Indicators for European Seas, Including Synthesis of Sea Level Rise and Storminess. Public 1.3.
Keeling P. J., Burki F., Wilcox H. M., Allam B., Allen E. E., Amaral-Zettler L. A., et al. (2014). The Marine Microbial Eukaryote Transcriptome Sequencing Project (MMETSP): Illuminating the Functional Diversity of Eukaryotic Life in the Oceans Through Transcriptome Sequencing. PLos Biol. 12, e1001889. doi: 10.1371/journal.pbio.1001889
Kim S., Kang Y., Kim H., Yih W., Coats D., Park M. (2008). Growth and Grazing Responses of the Mixotrophic Dinoflagellate Dinophysis Acuminata as Functions of Light Intensity and Prey Concentration. Aquat. Microb. Ecol. 51, 301–310. doi: 10.3354/ame01203
Lassus P., Bardouil M. (1991). Le Complexe Dinophysis Acuminata: Identification Des Espèces Le Long Des Côtes Françaises. Cryptogam. Algol. 12, 1–9.
Lazure P., Dumas F., Vrignaud C. (2008). Circulation on the Armorican Shelf (Bay of Biscay) in Autumn. J. Mar. Syst. 72, 218–237. doi: 10.1016/j.jmarsys.2007.09.011
Lazure P., Garnier V., Dumas F., Herry C., Chifflet M. (2009). Development of a Hydrodynamic Model of the Bay of Biscay, Validation of Hydrology, Cont. Shelf. Res. 29, 985–997. doi: 10.1016/j.csr.2008.12.017
Le Cann B., Serpette A. (2009). Intense Warm and Saline Upper Ocean Inflow in the Southern Bay of Biscay in Autumn-Winter 2006-2007. Continent. Shelf. Res. 29, 1014–1025. doi: 10.1016/j.csr.2008.11.015
Le Gac M., Metegnier G., Chomérat N., Malestroit P., Quéré J., Bouchez O., et al. (2016). Evolutionary Processes and Cellular Functions Underlying Divergence in Alexandrium Minutum. Mol. Ecol. 25, 5129–5143. doi: 10.1111/mec.13815
Lett C., Verley P., Mullon C., Parada C., Brochier T., Penven P., et al. (2008). A Lagrangian Tool for Modelling Ichthyoplankton Dynamics. Environ. Model. Soft. 23, 1210–1214. doi: 10.1016/j.envsoft.2008.02.005
Li H. (2013). Aligning Sequence Reads, Clone Sequences and Assembly Contigs With BWA-MEM. arXiv:1303.3997, ArXiv: 1303.3997.
Li W., Godzik A. (2006). Cd-Hit: A Fast Program for Clustering and Comparing Large Sets of Protein or Nucleotide Sequences. Bioinformatics 22, 1658–1659. doi: 10.1093/bioinformatics/btl158
Lyard F., Lefevre F., Letellier T., Francis O. (2006). Modelling the Global Ocean Tides: Modern Insights From FES2004. Ocean. Dynam. 56, 394–415. doi: 10.1007/s10236-006-0086-x
Madec G., Delecluse P., Imbard M., Lévy C. (1998). OPA 8.1 Ocean General Circulation Model Reference Manual. 91.
Marchesiello P., McWilliams J., Shchepetkin A. (2001). Open Boundary Conditions for Long-Term Integration of Regional Oceanic Models. Ocean. Model. 3, 1–20. doi: 10.1016/S1463-5003(00)00013-5
Martiny J. (2006). Microbial Biogeography: Putting Microorganisms on the Map. Nat. Rev. Microbiol. 4, 102–112. doi: 10.1038/nrmicro1341
McWilliams J. (2016). Submesoscale Currents in the Ocean. Proc. R. Soc A. 472, 20160117. doi: 10.1098/rspa.2016.0117
Metegnier G., Paulino S., Ramond P., Siano R., Sourisseau M., Destombe C., et al. (2020). Species Specific Gene Expression Dynamics During Harmful Algal Blooms. Sci. Rep. 10, 6182, 1–14. doi: 10.1038/s41598-020-63326-8
Mitarai S., Riley J. J., Kosály G. (2003). A Lagrangian Study of Scalar Diffusion in Isotropic Turbulence With Chemical Reaction. Phys. Fluid. 15, 3856–3866. doi: 10.1063/1.1622950
Mitarai S., Siegel D., Watson J., Dong C., Mc Williams J. (2009). Quantifying Connectivity in the Coastal Ocean With Application to the Southern California Bight. J. Geophys. Res. 114, 10026. doi: 10.1029/2008JC005166
Moita M., Sobrinho-Gonçalves L., Oliveira P., Palma S., Falcão M. (2006). A Bloom of Dinophysis Acuta in a Thin Layer Off North-West Portugal. Afr. J. Mar. Sci. 28, 265–269. doi: 10.2989/18142320609504160
Molines J., Barnier B., Penduff T., Treguier A., Le Sommer J. (2014). “Orca12.L46 Climatological and Interannual Simulations Forced With DFS4.4: GJM02 and MJM88,” in Drakkar Group Experiment Report GDRI-DRAKKAR-2014-03-19.
Mora C. (2012). High Connectivity Among Habitats Precludes the Relationship Between Dispersal and Range Size in Tropical Reef Fishes. Ecography 35, 89–96. doi: 10.1111/j.1600-0587.2011.06874.x
Park J. H., Kim M., Jeong H. J., Park M. G. (2019). Revisiting the Taxonomy of the “Dinophysis Acuminata Complex’’ (Dinophyta)’. Harm. Algae. 88, 101657. doi: 10.1016/j.hal.2019.101657
Pope S. (1985). PDF Methods for Turbulent Reactive Flows. Prog. Energy Combust. Sci. 11, 119–192. doi: 10.1016/0360-1285(85)90002-4
Pope S. (1994). Lagrangian PDF Methods for Turbulent Flows. Annu. Rev. Fluid. Mech. 26, 23–63. doi: 10.1146/annurev.fl.26.010194.000323
Purcell S., Neale B., Todd-Brown K., Thomas L., Ferreira M. A. R., Bender D., et al. (2007). PLINK: A Tool Set for Whole-Genome Association and Population-Based Linkage Analyses. Am. J. Hum. Genet. 81, 559–575. doi: 10.1086/519795
Raho N., Rodríguez F., Reguera B., Marín I. (2013). Are the Mitochondrial Cox1 and Cob Genes Suitable Markers for Species of Dinophysis Ehrenberg? Harm. Algae. 28, 64–70. doi: 10.1016/j.hal.2013.05.012
Raine R. (2014). A Review of the Biophysical Interactions Relevant to the Promotion of HABs in Stratified Systems: The Case Study of Ireland. Deep. Sea. Res. Part II.: Top. Stud. Oceanog. 101, 21–31. doi: 10.1016/j.dsr2.2013.06.021
Reguera B., Riobó P., Rodríguez F., Díaz P. A., Pizarro G., Paz B., et al. (2014). Dinophysis Toxins: Causative Organisms, Distribution and Fate in Shellfish. Mar. Drugs 12, 394–461. doi: 10.3390/md12010394
Reguera B., Velo-Suarez L., Raine R., Park M. G. (2012). Harmful Dinophysis Species: A Review. Harm. Algae. 14, 87–106. doi: 10.1016/j.hal.2011.10.016
Rossi V. E., Ser-Giacomi C. L., Hernández-G arcía E. (2014). Hydrodynamic Provinces and Oceanic Connectivity From a Transport Network Help Designing Marine Reserves. Geophys. Res. Lett. 41, 2883–2891. doi: 10.1002/2014GL059540
Séchet V., Sibat M., Billien G., Carpentier L., Rovillon G.-A., Raimbault V., et al. (2021). Characterization of Toxin-Producing Strains of Dinophysis Spp. (Dinophyceae) Isolated From French Coastal Waters, With a Particular Focus on the D. Acuminata-Complex. Harm. Algae. 101974, 1–14. doi: 10.1016/j.hal.2021.101974
Stolte W., Garcés E. (2006). “Ecological Aspects of Harmful Algal In Situ Population Growth Rates,” in Ecology of Harmful Algae. Eds. Granéli E., Turner J. T. (Berlin, Heidelberg: Springer), 139–152. doi: 10.1007/978-3-540-32210-8_11
Theetten S., Vandermeirsch F., Charria G. (2017). BACH1000_100lev-51 : A MARS3D Model Configuration for the Bay of Biscay, SEANOE. doi: 10.17882/43017
Uppala S. M., KÅllberg P. W., Simmons A. J., Andrae U., Bechtold V. D. C., Fiorino M., et al. (2005). The ERA-40 Re-Analysis. Q. J. R. Meteorol. Soc. 131, 2961–3012. doi: 10.1256/qj.04.176
Valencia V., Franco J., Borja Á., Fontán A. (2004). “Chapter 7 - Hydrography of the Southeastern Bay of Biscay,” in Elsevier Oceanography Series, vol. 70 . Eds. Borja Á., Collins M. (Elsevier), 159–194. doi: 10.1016/S0422-9894(04)80045-X
Van Sebille E. (2018). Lagrangian Ocean Analysis: Fundamentals and Practices. Ocean. Model. 121, 49–75. doi: 10.1016/j.ocemod.2017.11.008
Velo-Suarez L., Fernand L., Gentien P., Reguera B. (2010a). Hydrodynamic Conditions Associated With the Formation, Maintenance and Dissipation of a Phytoplankton Thin Layer in a Coastal Upwelling System. Continent. Shelf. Res. 30, 193–202. doi: 10.1016/j.csr.2009.11.002
Velo-Suarez L., Reguera B., Gonzalez-Gil S., Lunven M., Lazure P., Nezan E., et al. (2010b). Application of a 3D Lagrangian Model to Explain the Decline of a Dinophysis Acuminata Bloom in the Bay of Biscay. J. Mar. Syst. 83, 242–252. doi: 10.1016/j.jmarsys.2010.05.011
Watson J. (2011). Currents Connecting Communities: Nearshore Community Similarity and Ocean Circulation. Ecology 92, 1193–1200. doi: 10.1890/10-1436.1
Wolny J. L., Egerton T. A., Handy S. M., Stutts W. L., Smith J. L., Whereat E. B., et al. (2020). Characterization of Dinophysis Spp. (Dinophyceae, Dinophysiales) From the Mid-Atlantic Region of the United States1. J. Phycol. 56, 404–424. doi: 10.1111/jpy.12966
Xie H., Lazure P., Gentien P. (2007). Small Scale Retentive Structures and Dinophysis. J. Mar. Syst. 64, 173–188. doi: 10.1016/j.jmarsys.2006.03.008
Zingone A., Larsen J. (2022). “Dinophysiales,” in IOC-UNESCO Taxonomic Reference List of Harmful Micro Algae (HABs). Available at: http://www.marinespecies.org/hab on 2022-05-01.
Keywords: community, diversity, transcriptome, connectivity, biogeography, Atlantic
Citation: Hariri S, Plus M, Le Gac M, Séchet V, Revilla M and Sourisseau M (2022) Advection and Composition of Dinophysis spp. Populations Along the European Atlantic Shelf. Front. Mar. Sci. 9:914909. doi: 10.3389/fmars.2022.914909
Received: 07 April 2022; Accepted: 24 May 2022;
Published: 14 July 2022.
Edited by:
Joe Silke, Marine Institute, IrelandReviewed by:
Wonho Yih, Kunsan National University, South KoreaCarmela Caroppo, National Research Council (CNR), Italy
Copyright © 2022 Hariri, Plus, Le Gac, Séchet, Revilla and Sourisseau. This is an open-access article distributed under the terms of the Creative Commons Attribution License (CC BY). The use, distribution or reproduction in other forums is permitted, provided the original author(s) and the copyright owner(s) are credited and that the original publication in this journal is cited, in accordance with accepted academic practice. No use, distribution or reproduction is permitted which does not comply with these terms.
*Correspondence: Marc Sourisseau, TWFyYy5Tb3VyaXNzZWF1bEBpZnJlbWVyLmZy