- 1Southern Ocean Carbon-Climate Observatory (SOCCO), Smart Places, Council for Scientific and Industrial Research (CSIR), Cape Town, South Africa
- 2Department of Earth Sciences, Stellenbosch University, Stellenbosch, South Africa
Active chlorophyll-a fluorescence was measured during five summer research cruises (2008 – 2016), spanning the Atlantic sector of the Southern Ocean. This unique data set provides information for assessing zonal, inter-annual and intra-seasonal variability (early versus late summer) of photosynthetic efficiency (Fv/Fm). The zonal variability of Fv/Fm showed a typical latitudinal decline from a maximum in the Polar Frontal Zone (PFZ) (0.24±0.03) to a minimum in the Southern Antarctic Circumpolar Current Zone (SACCZ) (0.18±0.07). The inter-annual variability in Fv/Fm (between each cruise) was the highest in the SACCZ, while the Antarctic Zone (AZ) exhibited low inter-annual variability. Intra-seasonal variability between the zones was limited to a significantly higher mean Fv/Fm in the PFZ and AZ in early summer compared to late summer. Intra-seasonal variability between the cruises was, however, inconsistent as higher mean Fv/Fm in early summer were seen during some years as opposed to others. Ancillary physical and biogeochemical parameters were also assessed to investigate potential direct and indirect drivers of co-variability with Fv/Fm through a series of statistical t-tests, where significant differences in Fv/Fm were used as focus points to interrogate the plausibility of co-variance. Inter-zonal variability of surface seawater temperature (SST) and Silicate:Phosphate (Si:P) ratios were highlighted as co-varying with Fv/Fm in all zones, whilst community structure played an indirect role in some instances. Similarly, inter-annual variability in Fv/Fm co-varied with SST, Nitrate:Phosphate (N:P) and Si:P ratios in the PFZ, AZ and SACCZ, while community structure influenced inter-annual variability in the PFZ and SACCZ. Intra-seasonal variability in Fv/Fm was linked to all the ancillary parameters, except community structure in the AZ, whilst different ancillary parameters dominated differences during each of the cruises. These results were further scrutinized with a Principal Component Analysis for a subset of co-located data points, where N:P and Si:P ratios emerged as the principal indirect drivers of Fv/Fm variability. This study highlights the scope for using Fv/Fm to reflect the net response of phytoplankton photophysiology to environmental adjustments and accentuates the complex interplay of different physical and biogeochemical parameters that act simultaneously and oftentimes antagonistically, influencing inter-zonal, inter-annual and intra-seasonal variability of Fv/Fm.
1 Introduction
The Southern Ocean (SO) hosts a diverse, unique and complex ecosystem (Harris, 2006), which is vital to the global uptake, sequestration and export of carbon from the atmosphere to the ocean’s interior through the biological carbon pump (BCP) (Arrigo et al., 2008; Nunn et al., 2013; DeVries and Weber, 2017). The functioning of the SO BCP assists in the regulation of global atmospheric carbon dioxide (CO2) levels (Takahashi et al., 2002), and thus in the modulation of Earth’s climate (Hauck et al., 2015). Phytoplankton are essential to the SO BCP and, therefore, an understanding of the temporal and spatial distribution of photophysiology and the drivers of variability is important (Petrou et al., 2016; Deppeler and Davidson, 2017). Measurements of photosynthetic efficiency (Fv/Fm) provide information on the effects of physiological stress on the photosynthetic apparatus of phytoplankton cells and hence, are indicative of the potential for primary production. Factors that directly and indirectly impact phytoplankton photophysiology and primary productivity include physical conditions (e.g., seawater temperature and solar irradiation; Arrigo et al., 2010; Heiden et al., 2019), ambient nutrients (e.g., Boyer et al., 2013), and community structure (e.g., Suggett et al., 2009). These conditions vary regionally and under seasonal extremes in the SO, driving large fluctuations in phytoplankton physiology and primary production that impact the efficiency of the BCP (Moore et al., 2013; Deppeler and Davidson, 2017).
The frontal features of the Antarctic Circumpolar Current (ACC) define zones in the SO (Orsi et al., 1995; Pollard et al., 2002) that are distinguished by unique physical and chemical characteristics (Deppeler and Davidson, 2017), which in turn influence the spatial distribution of phytoplankton community structure, metabolic rates, Fv/Fm (Suggett et al., 2009) and primary production (Boyer et al., 2013). For example, warmer surface seawater temperature (SST) with decreasing latitude directly impact phytoplankton metabolic rates (Marañón et al., 2018), which may indirectly impact photosynthetic responses. Although Fv/Fm is not directly related to adjustments in SST, relationships may arise as a secondary response that reflects an imbalance in cellular metabolism. The very cold SSTs of the high latitude SO can also indirectly affect Fv/Fm by inducing changes in phytoplankton community structure (Feng et al., 2009; Finkel et al., 2010). Nutrient stress also directly impacts Fv/Fm (Spackeen et al., 2018) by curtailing photosynthesis when insufficient, resulting in lower Fv/Fm, and indirectly by impacting the temperature dependence of phytoplankton metabolic rates (Finkel et al., 2010; Marañón et al., 2018) and community structure. Concomitant with this is light availability, which also impacts nutrient demand (Sunda and Huntsman, 1995; Strzepek et al., 2012) further impacting Fv/Fm.
Since the seasonal cycle alters heat flux, water temperatures, stratification, mixed layer depth (MLD), nutrient supply and light availability, it is expected that there will be some seasonal influence on the variability of photophysiology, both directly and indirectly (Suggett et al., 2006). Under low light conditions (i.e., in winter or when MLD’s are deep in early spring), phytoplankton effectively maximise photosynthesis by increasing the size of their light-harvesting antenna or the number of photosynthetic units to increase light absorption (Sunda and Huntsman, 1997; Sunda and Huntsman, 2012; Strzepek et al., 2019). This photophysiological strategy to low light increases intracellular iron requirements, thereby decreasing Fv/Fm despite the availability of nutrients implied by deep MLD’s (Boyd, 2002). In late spring and early summer, high Fv/Fm values have been observed in the Sub-Antarctic Zone (SAZ) (Ryan-Keogh et al., 2018), supposedly driven by a shoaling of the MLD’s (or depths of active mixing), that provided the required light environment to support positive net community production and bloom formation (Mahadevan et al., 2012; Brody and Lozier, 2014), while nutrients were still plentiful from winter resupply (Tagliabue et al., 2014). On the contrary, in late summer and autumn, nutrient depletion from phytoplankton utilization has been proposed as the likely driver of low Fv/Fm (Ryan-Keogh et al., 2018). An important seasonal aspect that adds to the Fv/Fm variability is the occurrence of iron limitation, despite high macronutrient availability. High rates of biological activity in the SO are typically supported by high concentrations of the macronutrients nitrate and phosphate but are often seasonally constrained by low concentrations of the micronutrient iron (de Baar et al., 1990; Morel and Price, 2003), which likely causes a temporary lowering in Fv/Fm. Strong seasonal variability is also typical of the Marginal Ice Zone (MIZ), where higher summer SST melts the sea ice (increasing micronutrient supply) and contributes to the formation of a shallow stratified freshwater lens that improves the light environment, which together yields higher Fv/Fm and increased rates of phytoplankton production (Arrigo et al., 2008; Demidov et al., 2012).
As temperature, light, and nutrients also affect community structure the above temporal and regional changes are expected to be indirectly reflected in Fv/Fm variability through a change in community structure. For example, when neither light nor nutrients are limiting, such as conditions occurring south of the Polar Front (PF) in early summer, diatoms thrive (Boyd et al., 2010), which typically display high values of Fv/Fm (Ryan-Keogh et al., 2017). Low concentrations of silicic acid toward the end of the phytoplankton growing season, drive a shift in community structure towards flagellates and haptophytes as diatom production is constrained (Hutchins et al., 2001; Boyd et al., 2010). These smaller celled phytoplankton tend to thrive in low nutrient conditions due to their high surface area to volume ratio advantage, and typically have a lower Fv/Fm than diatoms (Suggett et al., 2009). A strong seasonal succession is also evident in the MIZ where P. antarctica tends to dominate the MIZ (Sow et al., 2020), along with large contributions of diatoms (Arrigo et al., 1999), which should result in higher Fv/Fm. However, in the MIZ, phytoplankton metabolism is primarily impacted by low SSTs that result in an upper limit to growth and productivity (Strzepek et al., 2019), which may indirectly drive lower Fv/Fm.
The complex spatial and temporal interplay of factors controlling phytoplankton photophysiology, production rates and the BCP's efficiency are expected to vary with climate change (Matebr and Hirst, 1999; Le Quéré et al., 2010). Warming and freshening of the SO due to global climate change (e.g., Durack and Wijffels, 2010) will enhance the degree of stratification (Hutchins and Boyd, 2016; Sallée et al., 2021), while an intensification of the westerly winds will deepen the MLD in summer (Sallée et al., 2021). These projected conditions are expected to lead to significant alterations in Fv/Fm through changes in light and nutrient availability. In addition, adjustments in phytoplankton community structure and function are expected whereby species that can physiologically adapt to outcompete under future physical and chemical conditions would be favored (Deppeler and Davidson, 2017; Strzepek et al., 2019). Such changes in the phytoplankton community are likely to impact Fv/Fm making it a powerful tool for assessing the individual and cumulative response of phytoplankton to the multitude of expected environmental adjustments associated with climate change.
Despite numerous incubation studies in the Atlantic SO that have probed phytoplankton’s photophysiological response to the manipulation of climatic stressors (e.g., Hutchins and Boyd, 2016; Ryan-Keogh et al., 2018; Viljoen et al., 2018), in situ investigations of the photophysiological response of phytoplankton to environmental changes over time and across the polar ocean are sparse. This data gap highlights the need for multi-year in situ monitoring programs with broad spatial coverage that can bridge numerous space-time knowledge gaps associated with a changing climate. Active chlorophyll-a (Chl-a) fluorescence can serve such ambitious monitoring programmes, as it is a powerful tool for deriving Fv/Fm as a proxy for the response of phytoplankton to physical, chemical or biological stressors (Kolber and Falkowski, 1992; Kolber et al., 1998). Fv/Fm can provide high-resolution observations of in situ phytoplankton photophysiology and subsequent primary production, thus enhancing our ability to understand the sensitivity of phytoplankton’s response to climate drivers. To our knowledge, this is the first multi-year austral summer study (5 cruises spanning 7 years) of in situ phytoplankton photophysiology that provides a unique opportunity for investigating the inter-zonal, inter-annual and intra-seasonal (early versus late summer) variability and distribution of Fv/Fm, alongside ancillary physical and biogeochemical parameters for the Atlantic SO.
2 Materials and methods
Photophysiological, environmental and biogeochemical data were collected from the ship’s non-toxic underway scientific seawater supply (~7 m water depth) during five South African National Antarctic Expedition (SANAE) cruises on the RV SA Agulhas and the RV SA Agulhas II to the Atlantic SO. The cruises were: SANAE 48 (December 2008 – February 2009), SANAE 49 (December 2009 – February 2010), SANAE 53 (November 2013 – February 2014), SANAE 54 (December 2014 – February 2015) and SANAE 55 (December 2015 – February 2016); hereafter referred to as S48, S49, S53, S54 and S55, respectively. An ongoing focus of the SANAE voyages is the repeat occupation of the hydrographic GoodHope Line (GHL) between South Africa and Antarctica, which has traversed the extent of the ACC since 2004 (Swart et al., 2012). Only data collected between 10˚W and 10˚E on the GHL were retained for this analysis. All active Chl-a fluorescence measurements which passed the quality control (QC) procedures below are displayed in Figure 1, with the individual frontal positions for each cruise indicated. The southbound transect typically takes place in December while the northbound return transect typically occurs in February. The cruise start and end dates appear in Supplementary Table S1, along with the frontal positions of the south- and northbound transects per cruise.
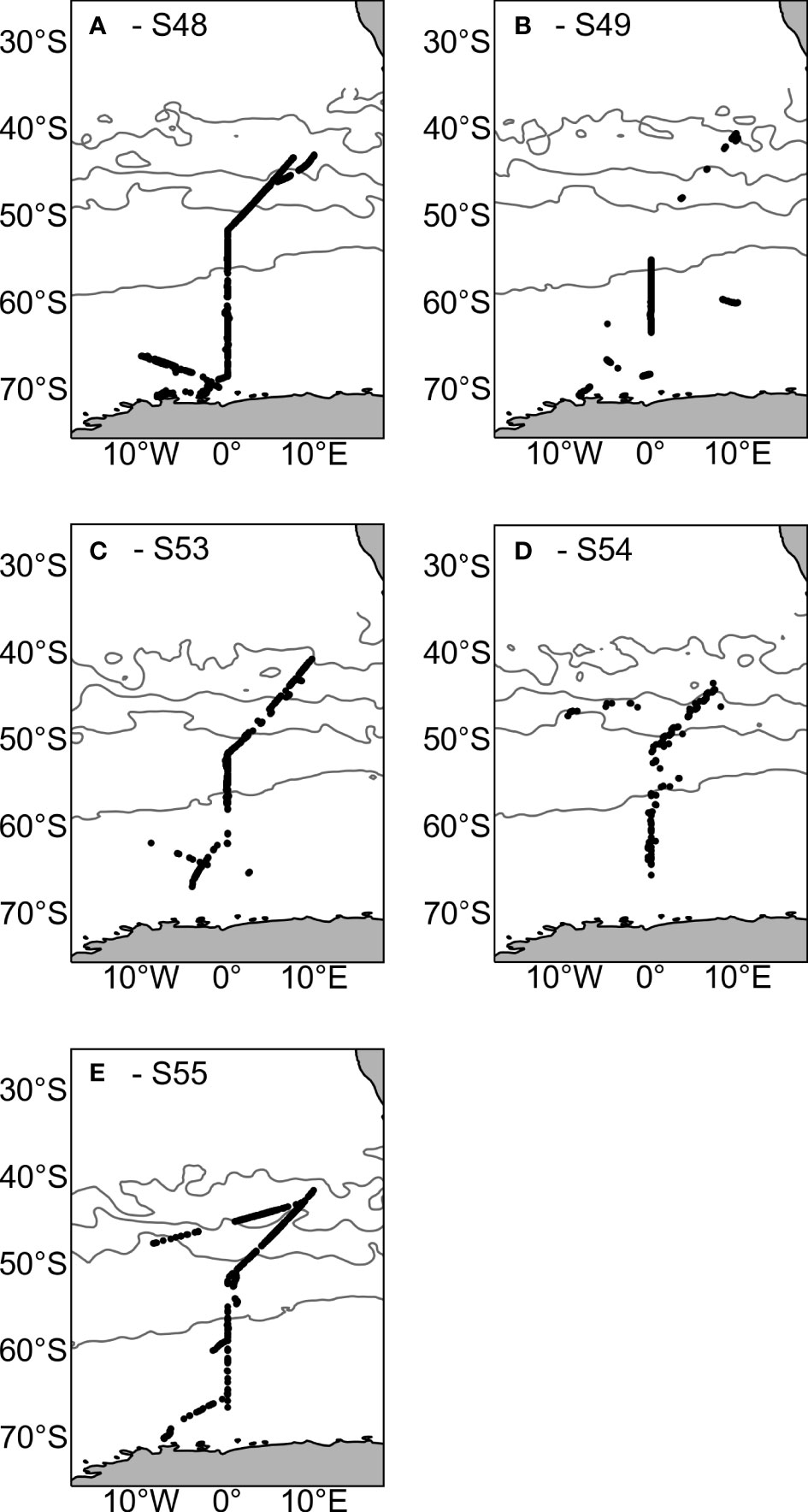
Figure 1 Individual plots of the night-time active chlorophyll-a fluorescence measurement points which passed the quality control (QC) procedure for each cruise independently (A) SANAE 48 (S48), (B) SANAE 49 (S49), (C) SANAE 53 (S53), (D) SANAE 54 (S54) and (E) SANAE 55 (S55), along with the respective frontal positions indicated for each cruise from north to south - Sub-Tropical Front (STF), Sub-Antarctic Front (SAF), Antarctic Polar Front (PF) and Southern Boundary (SBdy), delineating the corresponding zones between the fronts (Sub-Antarctic Zone (SAZ), Polar Frontal Zone (PFZ), Antarctic Zone (AZ) and Southern Antarctic Circumpolar Current Zone (SACCZ)).
Measurements of phytoplankton photophysiology from active Chl-a fluorescence were made continuously (every 30 - 90 seconds) and coupled with 4-hourly measurements of a suite of underway physical and biogeochemical parameters. The geographical frontal positions for each month of every cruise were obtained from maps of absolute dynamic topography from the CLS/AVISO product (Rio et al., 2011) using the boundary definitions from Swart et al. (2012). The positions of the Sub-Tropical Front (STF), Sub-Antarctic Front (SAF), Antarctic Polar Front (PF) and Southern Boundary (SBdy) for each individual cruise can be found in Supplementary Table S1. These fronts form zones, as defined in Pollard et al. (2002), as the SAZ, the Polar Frontal Zone (PFZ), the Antarctic Zone (AZ), and the Southern Antarctic Circumpolar Current Zone (SACCZ), respectively. Cruise-specific frontal positions were used to separate the zones for the statistical analyses in this paper, i.e., to compare zonal differences.
2.1 Active Chl-a fluorescence
Active Chl-a fluorescence data (Fv = Fm – Fo, where Fm is the maximum fluorescence and Fo the initial fluorescence) were collected with a benchtop Fast Induction and Relaxation (FIRe) system (Formerly Satlantic, now Seabird, S/N: 030) in underway flow-through mode (Gorbunov and Falkowski, 2004). The FIRe protocol consisted of 80 – 100 × 1 μs saturating flashlets with a 1 μs interval. Samples were continually replaced within a measurement interval of 30 - 90 seconds and 10 transients per measurement. To improve the signal-to-noise ratio, a pre-optimization transient averaging a 5-minute time window was applied across all cruises (Supplementary Table S2). Measurements were blank corrected on a daily basis using seawater filtered through a 0.2 µm filter (Cullen and Davis, 2003). Raw data were processed using the custom code Phytoplankton Photophysiology Utilities in Python 3.7 (Ryan-Keogh and Robinson, 2021) fitting the biophysical model of Kolber et al. (1998). The model fits the saturation phase of the data where the connectivity coefficient, ρ, was held at a constant value of 0.3 (Suggett et al., 2001) and the first flashlet was skipped (Ryan-Keogh and Robinson, 2021) to derive Fo, Fm and Fv/Fm.
2.1.1 Quality control
A series of quality control (QC) tests were implemented on the active Chl-a fluorescence data (Supplementary Table S2). (1) Measured values greater or less than the mean ± the standard deviation (SD), for each 5-minute averaged measurement, were excluded as outliers. (2) Data that fell outside the following photophysiological ranges were rejected: 200 < absorption cross-section (σPSII, Å2)< 2000 (as outlined in Ryan-Keogh and Robinson, 2021) and 0 < Fv/Fm < 0.65. The latter cut-off was applied as the maximum observed Fv/Fm values for phytoplankton under optimum growth conditions were found in the literature to be ca. 0.65 and 0.70, dependent on the taxonomy (Juneau and Harrison, 2005; Suggett et al., 2009). (3) Furthermore, data were excluded if the Root Mean Square Error (RMSE) value was greater than the mean RMSE for each cruise.
2.1.2 Diurnal variability
To test whether Non-Photochemical Quenching (NPQ; Owens et al., 1980; Falkowski, 1997) significantly impacted daytime Fv/Fm, day (local sunrise to local sunset) and night (local sunset to local sunrise) Fv/Fm values were compared to each other for all cruises (S48, S49, S53, S54 and S55) combined, across each geographic zone (SAZ, PFZ, AZ and SACCZ) (Supplementary Table S3). Please refer to section 2.3.1. for how the statistical t-tests were performed.
2.1.3 Chl-a concentrations
Seawater samples (250-500 mL) were collected from the ship's continuous underway supply for Chl-a and filtered through a Whatman Glass Fiber Filter (GF/F) filter (nominal pore size 0.7 µm). The filter was extracted in 90% acetone for 12–24 hours in the dark at –20˚C and measured on a Turner Designs 10AUTM fluorometer (during the S48 cruise) and Turner Trilogy (all other cruises), calibrated with a Chl-a standard (Sigma Aldrich) following the non-acidification method (Welschmeyer, 1994).
2.2 Ancillary data
2.2.1 Surface seawater temperature (SST)
SST was continuously measured every minute during each cruise by a Seabird thermo-salinograph installed at the intake of the ship's underway scientific water supply.
2.2.2 Macronutrients
Samples for macronutrients nitrate (), silicate (Si(OH)4) and phosphate () on S48 were manually analyzed on-board, following the methods described by Grasshoff et al. (1983) and Parsons et al. (1984). S49 macronutrient samples were analyzed similarly as above, with the exception of silicate, which was stored frozen at -20˚C and later analyzed on a Lachat QuikChem 8500 series 2 Flow Injection Autoanalyzer (FIA) (Wolters, 2002; Egan, 2008). On S53, S54 and S55, nitrate and silicate were run on the Lachat QuikChem 8500 FIA while phosphate was run manually following the methods described by Grasshoff et al. (1983) and Parsons et al. (1984). The nitrate:phosphate and silicate:phosphate molar ratios, hereafter referred to as N:P and Si:P, were computed for each station per cruise with phosphate as the common denominator due to its long ocean residence time compared to other biologically limiting macronutrients and it being a traditional tracer (Bigg and Killworth, 1988).
2.2.3 Community structure
Samples were collected for High-Performance Liquid Chromatography (HPLC) to determine the photosynthetic pigment composition by filtering between 1000 mL and 2000 mL of seawater onto 25 mm diameter Whatman GF/F filters that were stored in liquid nitrogen (S48 and S49) or in a -80˚C freezer (all other cruises) until further analysis on land. For S48 and S49, pigments were extracted in 90% acetone, aided by ultra-sonification for a few seconds, the extract was centrifuged and analyzed by HPLC as described by Barlow et al. (1997). Samples from S48 were analyzed at the Department of Environmental Affairs (DEA), South Africa, while samples from S49 were analyzed at the National Oceanography Centre, Southampton (NOCS), UK. For S53, S54 and S55, pigments were extracted in 100% methanol, aided by the use of ultra-sonification for a few seconds. The extract was filtered and analysed at Laboratoire d’Océanographie de Villefranche-sur-Mer, France, according to Ras et al. (2008). Root square transformations were applied to the pigment composition data for standardization prior to cluster analysis utilizing multi-dimensional scaling to group samples as outlined by Gibberd et al. (2013). The community structure of phytoplankton was then determined from the pigment ratios using Gibberd et al. (2013), and the CHEMTAX software (Mackey et al., 1996; Wright et al., 2010).
2.2.4 Diagnostic pigment ratios and the effective cell diameter (Deff) index
The diagnostic pigment ratios described by Vidussi et al. (2001) were used to determine micro-, nano- and picophytoplankton contributions at each station. A representative particle size-structure index for each sample was then calculated by assuming a representative size for microphytoplankton (20 μm), nanophytoplankton (10 μm) and picophytoplankton (1 μm) (Thomalla et al., 2017). The contribution of each size class was multiplied by the representative cell diameter at each station and summed to calculate an effective cell diameter (Deff) per station (Thomalla et al., 2017).
2.3 Statistical tests
2.3.1 t-tests
Data were grouped into daytime versus night-time (defined by local sunrise and sunset), season (early summer (December) and late summer (February)) and per zone (SAZ, PFZ, AZ, SACCZ) for each cruise (S48, S49, S53, S54 and S55) to determine: (a) day and night means of Fv/Fm for assessing diurnal variability, and (b) to assess the statistical significance between zones, years and seasons. The statistical significance was evaluated by making use of a Levene test to check for equal variances, where a standard student’s t-test was used for data of equal variance or a Welch's t-test for when data was of unequal variances. The t-tests were performed to compare the mean Fv/Fm values as follows: (1) inter-zonal variability (differences between zones) (2) inter-annual variability (differences between years) and (3) intra-seasonal variability as a comparison of early (December) versus late (February) summer. Results of the t-tests are reported as statistically significant when p<0.05, p<0.01 or p<0.001.
2.3.2 Principal Component Analysis
The Fv/Fm data were co-located with the 4-hourly ancillary data for each cruise, where a 15-minute mean interval was taken for Fv/Fm before and after each matching station in date, time, latitude and longitude to create a combined dataset of Fv/Fm and the ancillary data for all cruises. Worth noting is that since the Fv/Fm data were collected in 5-min averages and the ancillary data collected every 4 hours this co-located data set represents only a small subset of the total Fv/Fm data set (n = 68). After removing all missing values, a Principal Component Analysis (PCA) was performed using the Python package MLxtend (Raschka, 2018) for each co-located Fv/Fm and ancillary data parameter, i.e., SST, N:P, Si:P, the chosen dominant community structure (diatom:haptophyte ratio) and Deff, as well as the bulk concentrations of nitrate, phosphate and silicate.
3 Results
3.1 Diurnal variability
In vivo fluorescence can vary over a diel cycle with suppressed Chl-a fluorescence values expected during the day compared to night. This is due to NPQ being a photoprotective process during daytime, whereby excess energy is dissipated as heat at the cost of fluorescence to avoid photo-oxidative damage under high irradiance (Owens et al., 1980; Falkowski, 1997). Fv/Fm measurements during the night reflect dark-adapted states and can be used to establish the effect of non-light-induced stress on the photosynthetic apparatus. Differences between day and night data were found to be significant (p<0.05) for all zones (Figure 2), and as such, only the night data were used for further analysis for all zones.
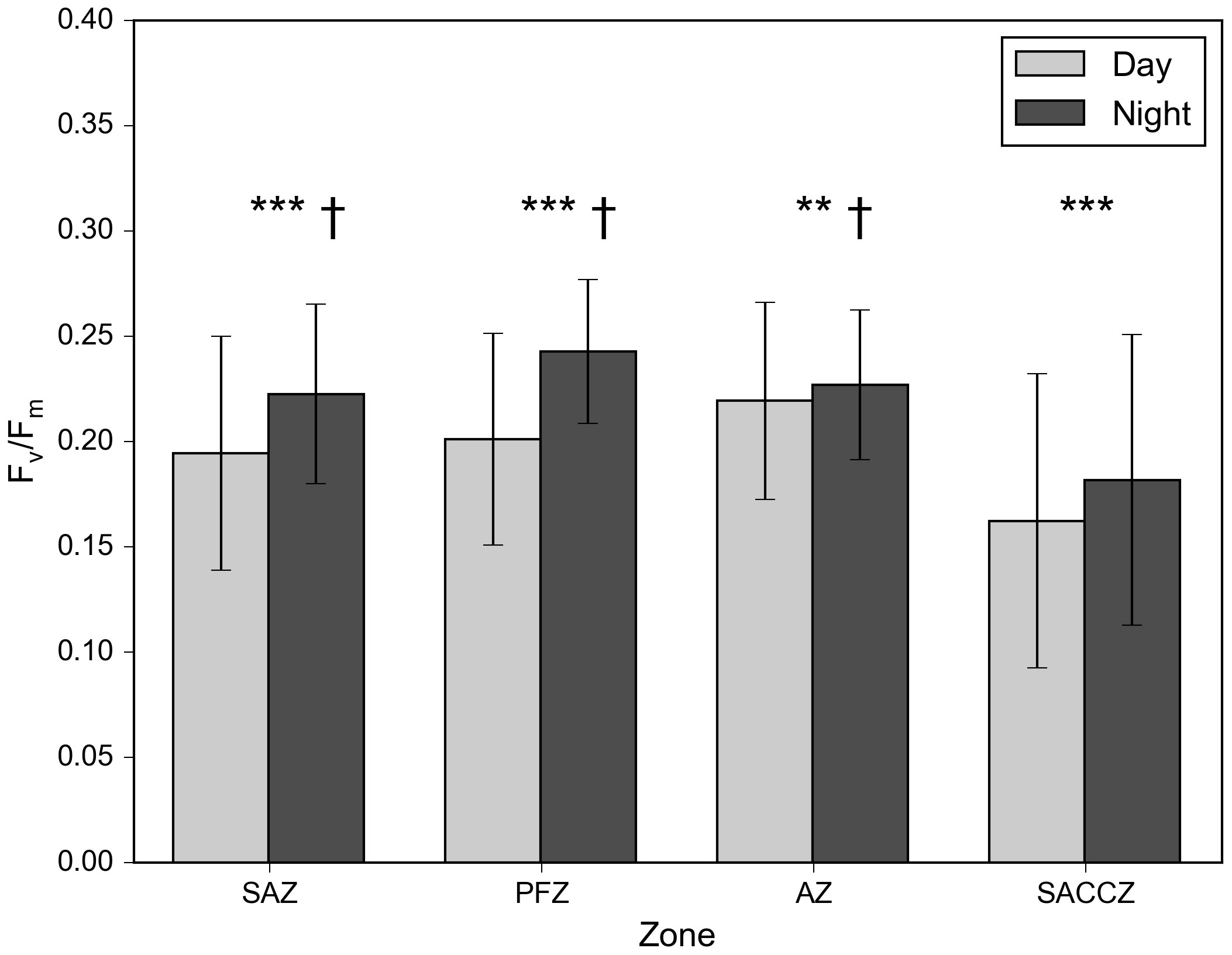
Figure 2 Diurnal variability of daytime and night-time means of Fv/Fm for all cruises grouped by zone. Error bars represent standard deviations. Significant differences are represented as *p<0.05, **p<0.01 and ***p<0.001, while † represents unequal variance where a Welch’s t-test was used.
3.2 Inter-zonal distribution and inter-annual variability
In this paper “inter-annual” refers to the comparison between the summer cruises. Overall, mean Fv/Fm values comprising all cruises were highest for the PFZ (0.24±0.03; p<0.001), followed by the AZ (0.23±0.04), SAZ (0.22±0.04) and the lowest for the SACCZ (0.18±0.07; p<0.001) (Figure 3; Table 1 and Supplementary Table S4). An investigation of inter-annual variability in Fv/Fm between each of the cruises for each zone showed that both the PFZ and SACCZ had particularly high inter-annual variability with most years being significantly different from each other (Tables 2B, D). The SACCZ was also characterized by higher SD’s (±0.04 - ±0.10) (Supplementary Table S5) compared to other zones (±0.003 - ±0.05), indicative of a higher range of inter-annual variability. In the AZ, inter-annual variability was particularly low with the majority of the cruises being similar, except S53 (Table 2C), which showed a higher mean Fv/Fm (0.25±0.04, p<0.001) compared to all other cruises (0.21 - 0.22) (Supplementary Table S5). The SAZ was also characterized by relatively high inter-annual variability in Fv/Fm, most notably during S49 which was typically higher than all other years, except S54 (Table 2A).
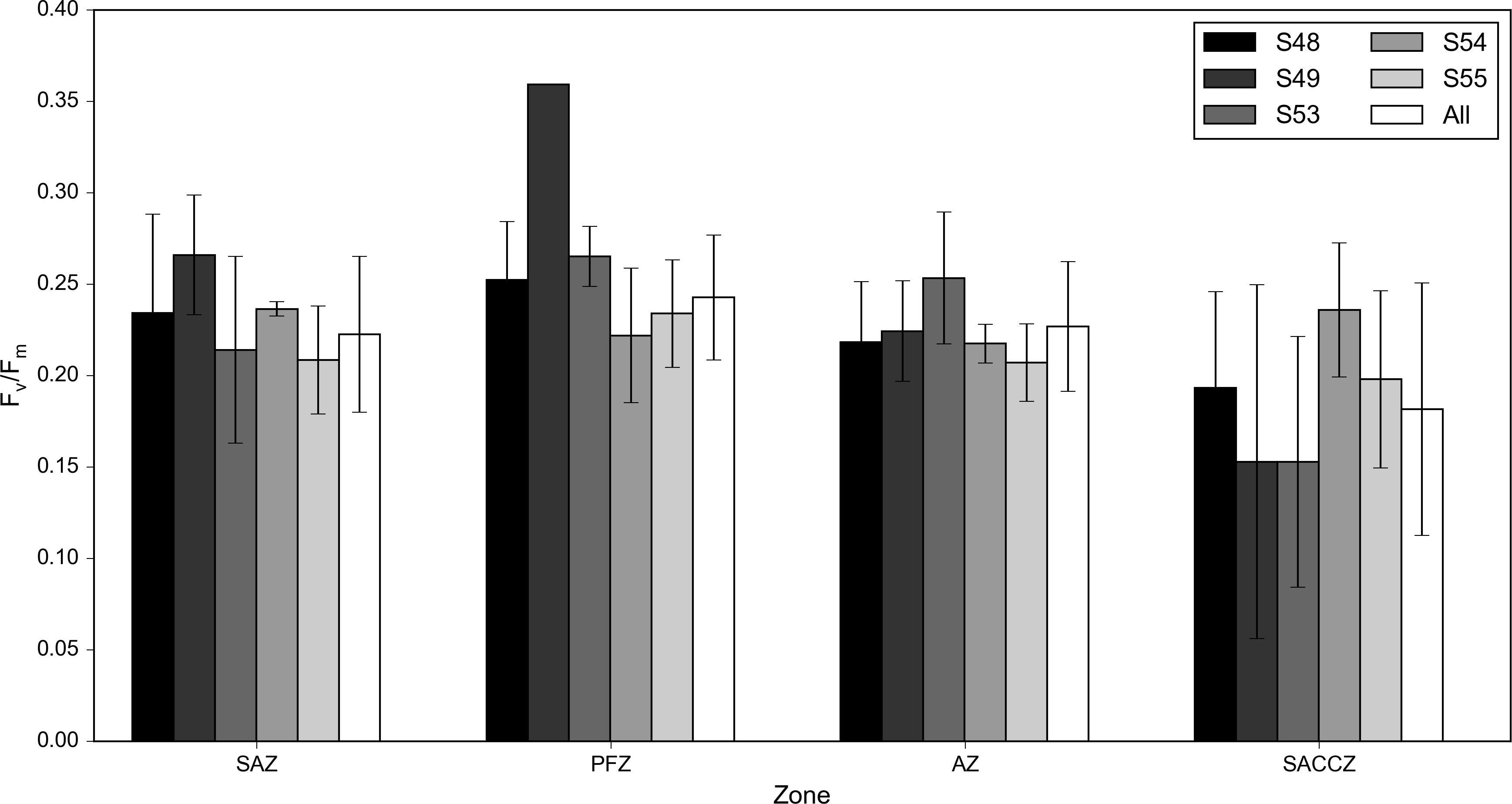
Figure 3 Inter-zonal distribution and inter-annual variability of the mean Fv/Fm. Error bars represent standard deviations. Note that S49 in the PFZ had too few data points to create a standard deviation (n=1).
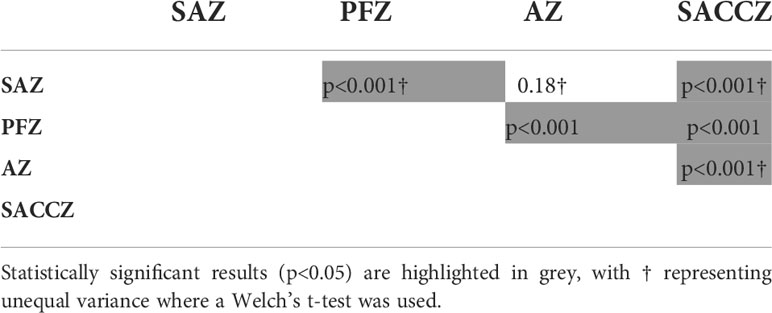
Table 1 Inter-zonal variability: p-values from the t-tests conducted on the Fv/Fm for all cruises combined and compared per zone.
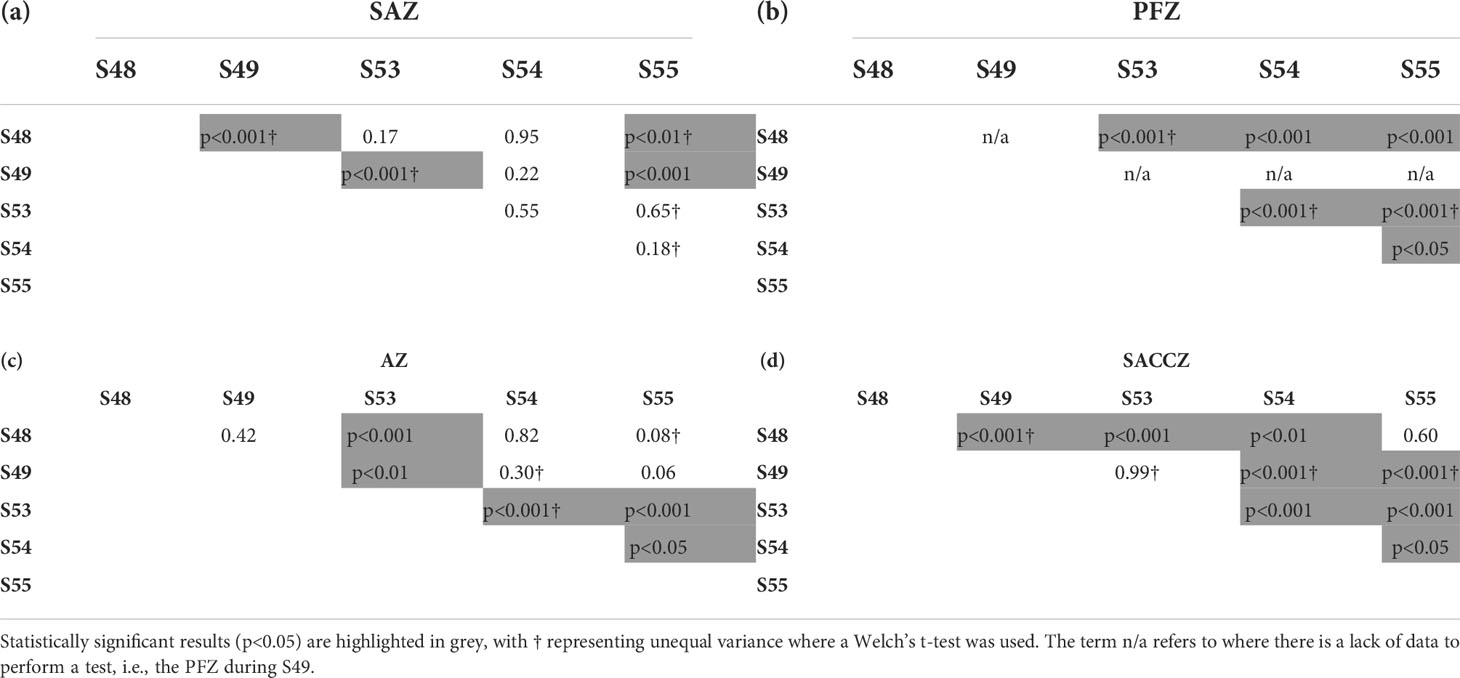
Table 2 Inter-annual variability per zone: p-values from the t-tests conducted on the Fv/Fm per cruise in each zone (Figure 3).
3.3 Intra-seasonal variability
As the cruises extended over the entire summer season, intra-seasonal differences were investigated between early (December) and late (February) summer (i.e., excluding all data from January). Averaged across all zones and all cruises (“All” in Figure 4A), the seasonal comparison of Fv/Fm was higher (p<0.05) during early summer (December, 0.22±0.04) than in late summer (February, 0.20±0.07) (Supplementary Table S6). However, this was not reflected consistently in all the individual zones. Although the same tendency was observed in the SACCZ (i.e., higher Fv/Fm in December 0.18±0.07), the opposite was observed in the PFZ and AZ, where higher Fv/Fm values were observed in February (p<0.001, Supplementary Table S7). The SAZ, in contrast, showed no significant difference between December and February (Supplementary Table S7). Similarly, when scrutinized on a cruise-by-cruise basis (Figure 4B), intra-seasonal differences in Fv/Fm were inconsistent, with higher values (p<0.05) observed in December during S49 and S53, whereas higher values were observed in February during S48 (p<0.001) and S54, whilst no intra-seasonal changes were seen for S55 (Supplementary Table S6).
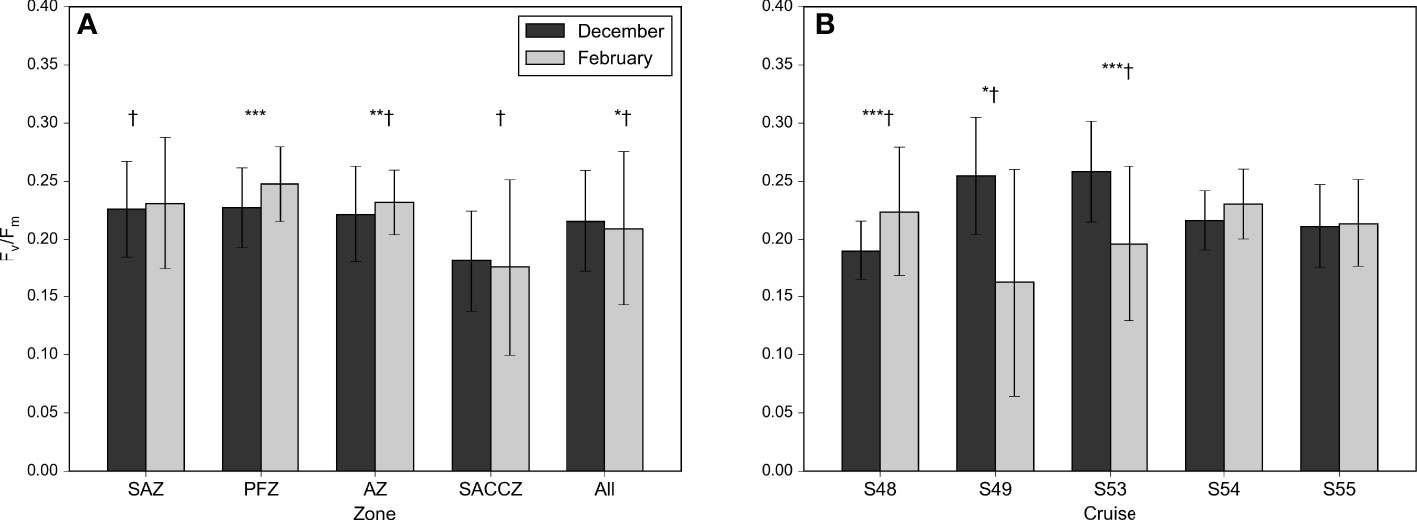
Figure 4 Intra-seasonal (early vs late summer) variability of Fv/Fm across (A) each zone and (B) per cruise in December and February. Error bars represent standard deviations. The overall mean Fv/Fm across all zones per season is shown as “All” in (A). Significant differences are represented as *p<0.05, **p<0.01 and ***p<0.001. Significant differences are represented as *p<0.05, **p<0.01 and ***p<0.001, while † represents unequal variance where a Welch’s t-test was used.
3.4 Investigating zonal, inter-annual and intra-seasonal co-variability between Fv/Fm and ancillary data
The ancillary data investigated here include SST, ambient macronutrient concentrations and ratios (N:P and Si:P) as well as indices of phytoplankton community structure (pigment ratios and effective cell diameter (Deff)). A summary of the mean values of these potential drivers per zone is available in Supplementary Table S4. For each significant difference observed in Fv/Fm between zones (inter-zonal, Table 1), cruises (inter-annual, Table 2) and December-February (intra-seasonal, Supplementary Tables S6, S7), the corresponding significant differences in ancillary data were similarly established. This means that ancillary data were only tested for significant differences if the Fv/Fm showed a significant difference. If a significant difference in Fv/Fm was also observed in an ancillary parameter, it could be considered as co-varying. As such, a co-varying parameter could be considered as a possible direct or indirect driver of the observed variability in Fv/Fm. Likewise, if no difference was observed in the ancillary data, it was excluded as a potential driver of the observed variability in Fv/Fm.
3.4.1 Surface seawater temperature (SST)
The mean SST averaged over all cruises decreased from maximum values of ~12°C in the SAZ southward towards minimum values of ~1°C in the SACCZ (Figure 5A and Supplementary Table S4). SSTs were different (p<0.001) between all the zones (Supplementary Table S8) and between some cruises within each zone (Supplementary Table S9). For example, inter-annual variability in SST was observed for S53 in the PFZ with lower (p<0.001) SST (~6°C) compared to S54 and S55 (7.2°C - 7.4°C), and in the AZ with lower (p<0.001) SST (~2°C) than S49, S54 and S55 (3.3°C - 3.8°C). SST was higher (p<0.001) in the SACCZ during S54 (~2°C) compared to all the other cruises (-0.1°C - 1.2°C) and during S55 (~1°C) compared to S49, S53 and S54 (-0.1°C - 2.1°C) (Supplementary Tables S5, S9). Intra-seasonal differences in SST were inconsistent (i.e. late summer was not consistently warmer) considering individual zones and individual cruises. For example, lower (p<0.001, Supplementary Table S7) SSTs were observed in early summer in the PFZ and AZ and during S49 (p<0.05), while lower SSTs were seen during S48 in late summer (p<0.05, Supplementary Table S6).
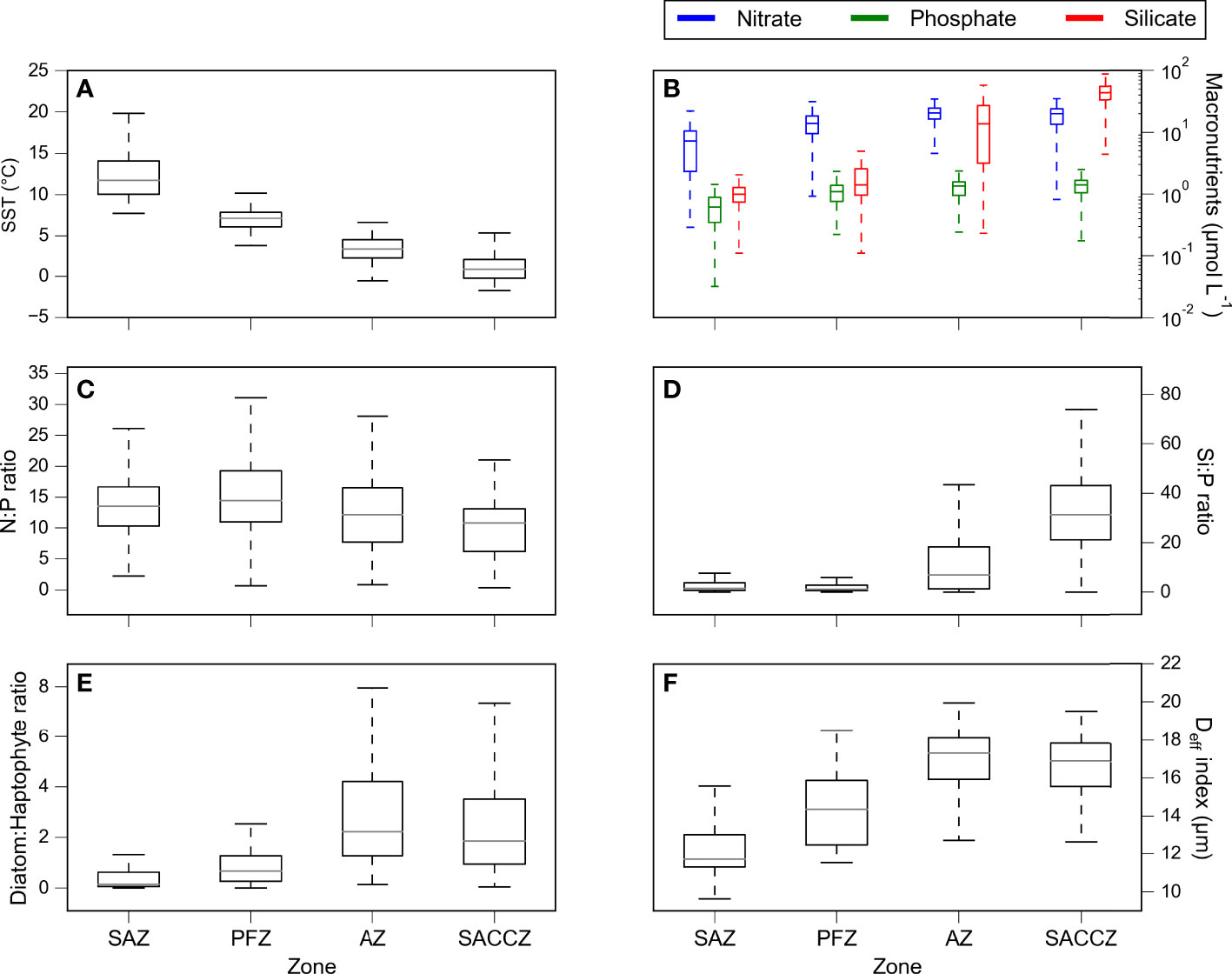
Figure 5 Ancillary parameters grouped per zone across all cruises for (A) Surface seawater temperature (SST) (°C), (B) Macronutrient concentrations (µmol L-1) plotted on a logarithmic scale, (C) Nitrate:Phosphate (N:P) (mol:mol) and (D) Silicate:Phosphate (Si:P) (mol:mol), (E) Diatom:Haptophyte ratio (% contribution to Chl-a) and (F) the phytoplankton effective diameter (Deff) index (µm). Boxplots represented as 25th and 75th percentile with median. Error bars represent standard deviations.
3.4.2 Macronutrient concentrations and macronutrient ratios
The macronutrient concentrations varied from the SAZ to the SACCZ (Figure 5B and Supplementary Table S4), where the nitrate concentrations increased from 7.1±5.2 μmol L-1 in the SAZ to ~20 μmol L-1 in the PFZ and SACCZ. The phosphate concentrations were the lowest in the SAZ (0.7±0.6 μmol L-1) and increased to ~1.4 μmol L-1 for the remaining three zones, substantiating phosphate as a good reference point to study the changes in nitrate and silicate as nutrient ratios, i.e. N:P and Si:P ratios. The silicate concentrations, however, steadily increased from 1.2±0.8 μmol L-1 in the SAZ to 45.7±18.5 μmol L-1 in the SACCZ.
The mean molar N:P ratios (mol:mol) for all cruises combined across all zones ranged from 10.7±6.7 in the SAZ to 13.6±6.8 in the SACCZ (Figure 5C and Supplementary Table S4). Significant differences were observed for the N:P ratios between all zones (p<0.05), except between the PFZ and SACCZ (Supplementary Table S10). Inter-annual differences in N:P ratios were observed between the cruises, notably in the PFZ, AZ and SACCZ for S53 (Supplementary Table S11). S53 stood out with mean N:P ratios that were significantly lower in the PFZ (7.5±3.4, p<0.001), compared to S48, S54 and S55 (13.2 - 15.4), and significantly lower in the AZ compared to all other cruises (6.4±6.7, p<0.001). In the SACCZ, S53 was lower (9.5±9.3, p<0.001) than S48, S54 and S55 (13.6 - 16.2) and higher during S55 (16.2±6.5, p<0.01) compared to S49, S53 and S54 (9.5 - 14.1). Intra-seasonal differences of higher N:P ratios during early summer (December) were only observed in the AZ (p<0.01) (Supplementary Table S7) and during S48 (p<0.001), while a lower N:P ratio was seen in December during S49 (p<0.01) (Supplementary Table S6).
The mean molar Si:P ratios (mol:mol) for all cruises (Figure 5D) showed substantial latitudinal variability with low ratios in the SAZ (4.1±7.4) and PFZ (3.8±10.7), increasing to 11.6±13.3 in the AZ and as high as 33.9±24.6 in the SACCZ (Supplementary Table S4). Inter-zonal differences were significantly higher for Si:P ratios between the SACCZ and all other zones (p<0.001) and between the PFZ and the AZ (p<0.001) (Supplementary Table S12). Inter-annual differences were again observed during S53 (p<0.05, Supplementary Tables S5, S13), with lower ratios in the PFZ (0.6±0.3, p<0.05) compared to cruises S48, S54 and S55 (1.5 - 7.0) and in the AZ (7.5±12.1, p<0.001) compared to S48, S54 and S55 (11.7 - 14.7). Lower Si:P ratios were seen in the SACCZ for S53 (26.4±23.3, p<0.001) compared to S54 and S55 (38.1 - 51.7), and for S55 (51.7±33.4, p<0.01) compared to S49, S53 and S54 (18.7 - 38.1). In contrast, Si:P ratios for S54 were higher (38.1±17.7, p<0.01) than for all the other cruises (18.7 - 26.4), but lower (p<0.001) than during S55 (51.7). Intra-seasonal differences with lower Si:P ratios in December compared to February were only observed in the AZ (p<0.01) and on S49 (p<0.001) (Supplementary Tables S7 and S6).
3.4.3 Community structure: pigment ratios
The two dominant phytoplankton groups across all cruises and all zones measured by their contribution to total Chl-a (in %), were diatoms (3% - 67%) and haptophytes (22% - 46%) (Supplementary Figure S1A). All other groups typically contributed less than 10%, e.g., dinoflagellates (4% - 7%), Prasinophytes (1% - 9%) and Cryptophytes (<5%), or showed higher contributions only in one particular zone, e.g. Chlorophytes (27%) and Synechococcus (12%) in the SAZ. As such, a focus was placed on the diatom to haptophyte ratio, which provides a single value index of change in community structure. The diatom:haptophyte ratio increased southwards from 0.4±0.6 in the SAZ to 1.0±1.1 in the PFZ, 5.0±12.0 in the AZ and then decreased to 2.7±2.6 in the SACCZ (Figure 5E and Supplementary Table S4). Inter-zonal differences in diatom:haptophyte ratios were significantly lower (p<0.05) in the SAZ compared to both the PFZ and SACCZ, and lower (p<0.001) in the PFZ compared to the SACCZ (Supplementary Table S14). In addition, inter-annual differences in the diatom:haptophyte ratio were evident in the PFZ and SACCZ, where for example, in the PFZ S54 (3.3±0.9) was higher (p<0.01) than S48, S53 and S55 (0.5 - 0.9) (Supplementary Tables S5 and S15). In the SACCZ, the diatom:haptophyte ratio was higher (p<0.001) during S53 (2.0±1.6) compared to S55 (3.3) (Supplementary Tables S5 and S15). Both the SAZ and AZ showed no significant inter-annual differences between cruises. Intra-seasonal comparisons of the diatom:haptophyte ratio in each zone showed no significant differences (Supplementary Table S7), whilst intra-seasonal variability in an inter-annual context indicated significantly higher ratios (p<0.01) during December on S53 compared to February (Supplementary Table S6).
3.4.4 Effective cell diameter (Deff) index
Phytoplankton were grouped into the following size fractions: microphytoplankton (fmicro > 20 µm), nanophytoplankton (2 µm > fnano< 20 µm) and picophytoplankton (fpico< 2 µm) (Supplementary Figure S1B). The fpico (0.3% – 6%) and fnano (29% – 67%) fractions decreased southwards, dominating in the SAZ and PFZ, whereas fmicro (26% – 70%) increased southward to dominate in the AZ and SACCZ. In order to attain a single value index to represent community size structure, an effective cell diameter (Deff) was calculated for each station (see methods section 2.2.4). The Deff index increased southwards from 12.1±2.3 µm in the SAZ to 14.3±1.9 µm in the PFZ and then up to 17.0±1.6 µm in the AZ, remaining similar in the SACCZ (16.6±1.7 µm) (Figure 5F and Supplementary Table S4). Deff was significantly lower (p<0.001) in the SAZ compared to both the PFZ and SACCZ and was lower in the PFZ compared to both the AZ and SACCZ (p<0.001) (Supplementary Table S16). In the PFZ, Deff values on S48 were lower (13.0±1.2; p<0.05) than on S53 (14.7±1.9), while in the SACCZ, the S49 Deff values were lower (15.2±1.5; p<0.05) than during S48, S54 and S55 (16.3 - 17.1) (Supplementary Tables S5 and S17). No inter-annual differences were evident in Deff in the SAZ or AZ. Intra-seasonal differences were evident with higher Deff in December in the PFZ and AZ (Supplementary Table S7), and with higher Deff in December on S53 (Supplementary Table S6).
3.5 Co-variability between the ancillary parameters and Fv/Fm
3.5.1 Inter-zonal co-variability
SST significantly differed between all zones and co-varied inter-zonally, similar to Fv/Fm (Supplementary Table S4). Zonal differences in N:P and Si:P ratios also co-varied with changes in Fv/Fm for the majority of zones, except for similarities in the PFZ and SACCZ for N:P ratios and in both the PFZ and the SAZ for Si:P ratios (Supplementary Tables S10 and S12). Changes in community structure, i.e., both the diatom:haptophyte ratio and Deff, co-varied with changes in Fv/Fm between zones, except between the AZ and SACCZ for which they were similar (Supplementary Tables S14 and S16).
3.5.2 Inter-annual co-variability
Ancillary parameters that matched the inter-annual significant differences in Fv/Fm are SST and both macronutrient ratios, mainly in the PFZ, AZ and SACCZ (Table 2 and Supplementary Tables S5, S11, S13). The diatom:haptophyte ratios and Deff, on the other hand, matched the inter-annual significant differences in Fv/Fm in the PFZ and SACCZ (Table 2 and Supplementary Tables S5, S15, S17). In the PFZ, significant differences in SST, as well as macronutrient ratios co-varied with a significantly higher mean Fv/Fm, most notably for S53, which had the lowest SST and nutrient ratios when compared to most of the other cruises. In the AZ, however, the lowest SST and N:P ratios for S53 coincided with the highest mean Fv/Fm. In the SACCZ, the highest mean SST and a high mean Si:P ratio co-varied with the high mean Fv/Fm for S54 compared to all other cruises, and the lowest mean N:P ratio for S53 compared to cruises S48, S54 and S55. The diatom:haptophyte ratio showed only a few significant inter-annual differences (p<0.05), such as in the PFZ and in the SACCZ (Supplementary Table S15).
3.5.3 Intra-seasonal co-variability
Differences assessed between all early and all late summer data, i.e., comparing December to February for all cruises across all zones combined, showed that both the N:P and Si:P ratios did not vary (Supplementary Table S6). Ancillary parameters which matched intra-seasonal differences observed in Fv/Fm included SST and Deff index in the PFZ and AZ, as well as the N:P and Si:P ratio only in the AZ (Supplementary Table S7). Ancillary parameters, which matched intra-seasonal differences observed in Fv/Fm, among the years were SST and N:P ratios during S48 and S49; Si:P ratios only during S49; as well as the diatom:haptophyte ratio and Deff index on S53 (Supplementary Table S6).
3.6 Principal Component Analysis between ancillary data and Fv/Fm
Despite the limited number of Fv/Fm data points that were co-located with ancillary data, when combined across all zones and seasons there was a sufficient sample size (n = 68) to perform a PCA analysis to identify the ancillary data which showed the strongest co-variance (Supplementary Figure S2). The sum of the first two principal components in the PCA explained 68% of the total variance in Fv/Fm. The grouping of the variables within each quadrant shows Fv/Fm to be in the opposite quadrant to the silicate concentration and the N:P and Si:P ratios, whilst SST was in the neighboring quadrant opposite to the nitrate and phosphate concentrations and the community structure indices.
4 Discussion
Active Chl-a fluorescence provides instantaneous, high-resolution spatial and temporal information on the response of phytoplankton to physiological stress caused by the local environment (Hughes et al., 2018). Here, we focus on Fv/Fm as an indicator of phytoplankton photosynthetic efficiency and examine the inter-zonal, inter-annual and intra-seasonal variability in Fv/Fm distribution across the Atlantic SO in summer. In addition, we investigate zonal, inter-annual and intra-seasonal co-variability in ancillary parameters to elucidate the possible direct and indirect drivers of variability in Fv/Fm. Finally, we use a PCA on a subset of co-located Fv/Fm and ancillary data to further assess the dominant drivers of overall Fv/Fm variability (i.e., across all years, zones and for all three months of summer).
4.1 Zonal, inter-annual and intra-seasonal variability in Fv/Fm
Fv/Fm exhibited large-scale spatial variability between the zones and was the highest in the PFZ (0.24±0.03). These values are similar to those previously reported for other open ocean regions such as those near the Kerguelen Plateau (0.2 - 0.4; Timmermans et al., 2008), but much lower than those observed downstream of sub-Antarctic islands (0.5 - 0.6; Moore et al., 2007; Timmermans et al., 2008). High Fv/Fm in the PFZ implies efficient photosynthesis that was the least constrained by environmental conditions. Optimal environmental summer conditions such as these are likely to result in higher rates of production for the PFZ and hence, possibly higher carbon drawdown in the region (Uitz et al., 2006). On the other hand, Fv/Fm in the SACCZ was the lowest (0.18±0.07), which potentially implies inefficient photosynthesis that was most likely limited by a combination of light and/or nutrients. In instances such as this, primary production is typically curtailed, leading to a potentially constrained biological carbon pump (Hardman-Mountford et al., 2013). However, Chl-a concentrations for this zone were the highest (Supplementary Figure S3), which implies that despite sub-optimal efficiency in photosynthesis (from possible light limitation or a combination of other factors), rates of production and biomass accumulation were substantial and unlikely to curtail export.
When averaged across all zones and cruises, Fv/Fm was typically higher in early summer when compared to late summer (Figure 5, Supplementary Table S3). This is in line with our understanding of seasonal nutrient limitation (in particular with regards to iron) following biological utilization and is in accordance with the literature (Deppeler and Davidson, 2017; Ryan-Keogh et al., 2018; Mtshali et al., 2019). However, when zones (and cruises) were interrogated on an individual basis this tendency was not consistently reflected. For example, no intra-seasonal difference was evident in the SAZ and SACCZ when all cruises were combined (Supplementary Table S4) and a higher Fv/Fm was instead observed in late summer in the PFZ and AZ. Similarly, when all zones were combined, although higher Fv/Fm was observed in early summer on S49 and S53, the opposite was true for S54 with no discernible difference on S54. This inconsistency of an intra-seasonal decline in Fv/Fm in late summer highlights the likelihood of a dynamic interplay of sub-seasonal variability in nutrient and light variability in addition to the phytoplankton community response that together determines the characteristics in Fv/Fm.
Different zones displayed different degrees of inter-annual variability in Fv/Fm. Using inter-annual variability as an approach for zonal characterization provides a dynamic understanding of phytoplankton regionalization that relies on underlying physical drivers in addition to climatological means (Thomalla et al., 2011). The SACCZ (and to a lesser extent the PFZ) had particularly high inter-annual variability in Fv/Fm, with most cruise occupations being significantly different from each other, suggesting that changes in the local environment between annual occupations were strongly impacting Fv/Fm. In addition, the highest range of SD in the SACCZ is indicative of the highest degree of inter-annual variability in Fv/Fm. Regions that are characterized by a high degree of inter-annual variability (i.e., the SACCZ and PFZ) are more likely to be influenced by sub-seasonal forcing of the nutrient and light supply e.g., through storm interactions that drive event scale variability in the MLD (Thomalla et al., 2011). Fv/Fm in the AZ on the other hand showed much lower inter-annual variability with mean values that were surprisingly similar for four of the five cruise occupations. Zones with particularly low inter-annual variability in Fv/Fm such as the AZ are more likely to be phase locked with the seasonal forcing of light and nutrients from a seasonal shoaling of the MLD (Thomalla et al., 2011). This does not mean that nutrients or light are not impacting Fv/Fm but merely that it is unlikely to vary sufficiently on intra-seasonal timescales to strongly influence inter-annual variability in Fv/Fm.
4.2 Investigating potential drivers of spatial and temporal variability in phytoplankton photophysiology
Iron plays a fundamental role in the photosynthetic process (Raven et al., 1999) and typically has a direct effect on Fv/Fm, which is why it is often referred to as the master variable. Fv/Fm variability in regions such as the SO that typically experience iron limitation, with low dissolved iron (DFe) concentrations drive low Fv/Fm. Hence, some of the low Fv/Fm results could be linked to iron stress, for e.g., in the SAZ, where Fv/Fm was lower in late summer as would be expected from biological utilization following the spring bloom (Deppeler and Davidson, 2017; Ryan-Keogh et al., 2018; Ryan-Keogh et al., ; Mtshali et al., 2019). However, without local DFe data, we are unable to adequately investigate its influence on Fv/Fm variability. A zonal comparison of Fv/Fm with DFe concentrations from the GEOTRACES data set (GEOTRACES Intermediate Data Product Group, 2021) did, however, show the SACCZ to be characterized by the highest concentrations of DFe (Supplementary Figure S4) but the lowest Fv/Fm (Figure 3). This implies that photosynthesis in this zone was unlikely to be iron-limited. but that Fv/Fm was more likely to be constrained by a combination of alternative drivers. Iron measurements were conducted as part of incubation studies on S54 and S55 with reported results suggesting that primary production was affected by iron-limitation during summer (Ryan-Keogh et al., 2017; Ryan-Keogh et al., 2018; Viljoen et al., 2018), with some potential iron-light co-limitation in the PFZ (Viljoen et al., 2018).
In the following section, the characteristics of zonal, inter-annual and intra-seasonal variability in Fv/Fm are investigated in the context of co-variability of the available ancillary data (summarized in Figure 6) in order to determine whether or not the ancillary parameter could be considered as a possible direct or indirect driver of Fv/Fm.
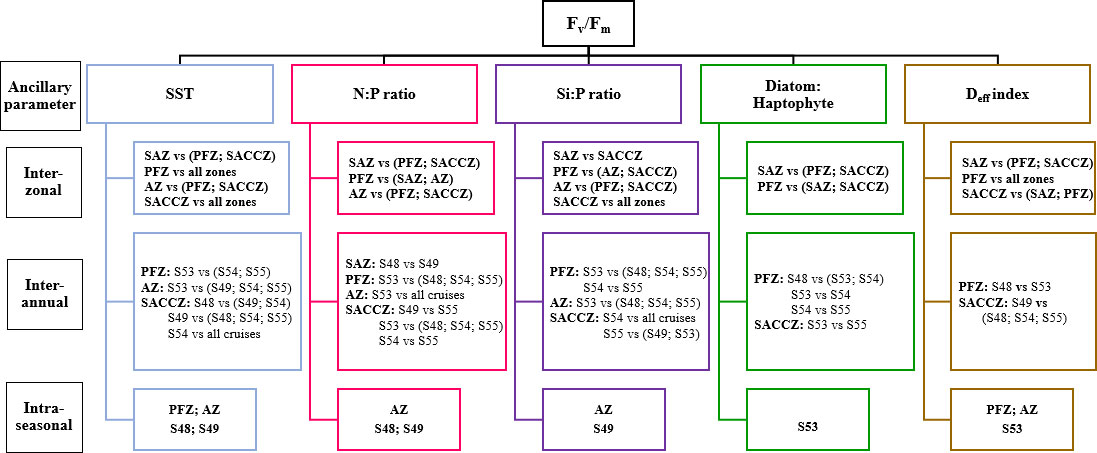
Figure 6 Schematic summary of the potential co-varying parameters (Surface seawater temperature, SST; Nitrate:Phosphate, N:P; Silicate:Phosphate, Si:P; Diatom:Haptophyte ratio and phytoplankton effective diameter (Deff) index) of Fv/Fm (where Fv/Fm was different at p<0.05), showing inter-zonal, inter-annual and intra-seasonal changes that matched changes in Fv/Fm. The zones/cruises before “vs” were different to each of the zones/cruises after “vs”. Note all significant differences are shown where p<0.05.
4.2.1 The dynamics between SST and Fv/Fm variability
At a global level, SST does not directly drive Fv/Fm, since both high and low Fv/Fm can be found in both tropical and polar waters. At a local level, however, positive correlations have been observed between SST and Fv/Fm, e.g., for dinoflagellates in the East China Sea (Shen et al., 2016). In our transects across the SO, a southward decrease in SST coincided with a zonal decrease in Fv/Fm (Supplementary Table S4), supporting the understanding that SST impacts the metabolic balance of phytoplankton (Maxwell and Johnson, 2000; Finkel et al., 2010; Marañón et al., 2018), which can indirectly impact the Fv/Fm signature. However, despite the general latitudinally co-varying trends, warmer SO waters did not always host higher Fv/Fm and colder SO waters did not always host lower Fv/Fm. For example, significantly warmer SSTs were observed in the SAZ compared to the AZ, but the Fv/Fm was nonetheless similar. Also, colder SSTs on S53 in the PFZ and AZ did not correspond to lower Fv/Fm. The response in Fv/Fm following changes in SST between early and late summer also varied. For example, on S48 higher December than February SSTs was accompanied by lower Fv/Fm, while on S54 lower December than February SSTs was accompanied by higher Fv/Fm. Nonetheless, when considering all years combined in the PFZ and AZ, December SSTs was significantly lower than for February, which coincided with significantly lower Fv/Fm. In summary, although changes in SST and Fv/Fm often co-vary, the response with SST was not consistent at inter zonal, inter-annual or intra-seasonal scales. This highlights the important understanding that other drivers are at play and that any relationship with SST is not direct but rather a secondary reflection of an imbalance in cellular metabolism.
4.2.2 The impact of macronutrients on Fv/Fm variability
SO phytoplankton are known to respond to changes in their chemical environment through an altered photosynthetic response (i.e. Fv/Fm response to nutrient stress) and/or a community structure response that favors particular species under those conditions, which in turn drives an indirect secondary response in Fv/Fm (e.g. Suggett et al., 2009). Ambient nitrate or phosphate concentrations are generally not considered to be limiting in the SO (Sedwick et al., 2002), which is in agreement with our measured ambient nutrient data (Supplementary Table S4). As such, and due to phosphate remaining relatively constant, we instead emphasized the role of variability in ambient macronutrient ratios in order to reflect preferential or limited acquisition and drawdown, e.g., as a result of community adjustments or iron limitation in the case of N:P ratios (Schoffman et al., 2016). N:P ratios covaried with Fv/Fm for all zones except the PFZ and SACCZ, while on an inter-annual basis mainly in the PFZ, AZ and SACCZ during S53 and intra-seasonally in the AZ and during S48 and S49 (Figure 6). The PCA results similarly show that the N:P ratio was in the direct opposite quadrant to Fv/Fm, suggesting that the observed significant zonal, inter-annual and intra-seasonal co-variability in Fv/Fm and N:P ratios could be the result of driver response. However, based on the understanding that nitrate uptake is curtailed under iron limiting conditions, due to the high iron demand for nitrate reductase (Timmermans et al., 1994), it is possible that these relationships observed in co-variability and PCA regressions (between Fv/Fm and N:P ratios) are instead a secondary signature of iron limitation, i.e., high N:P ratios under iron limiting conditions being reflected in low Fv/Fm ratios.
Silicate, on the other hand, is considered to be limiting to diatoms, particularly towards the end of the growing season, most notably north of the PF (Hutchins et al., 2001; Boyd et al., 2010). Our results supported this understanding with a zonal distribution in both the ambient silicate and the Si:P ratios, which increased significantly south in the AZ and SACCZ (Figures 5B, D; Supplementary Table 4). A response in Fv/Fm to low Si:P ratios could reflect an indirect community response to low silicate conditions limiting the production of diatoms who typically express higher Fv/Fm than flagellates (Suggett et al., 2009). However, were this the case, a characteristically lower Fv/Fm would be anticipated in low Si:P diatom-dominated waters north of the PF, which was not the case. This lack of consistency in any directional change in Fv/Fm being associated with macronutrient ratios was similarly observed inter-annually as well as intra-seasonally. This implies a more dominant role of other factors (e.g cold temperatures / low light) in driving low Fv/Fm south of the PF.
4.2.3 The impact of community structure and cell size on Fv/Fm variability
As discussed previously, changes in phytoplankton community structure impact the baseline Fv/Fm (Suggett et al., 2009), with diatoms having a higher Fv/Fm than other phytoplankton groups. The increase in the diatom:haptophyte ratio and Deff between the SAZ and PFZ indeed corresponded to such a significant increase in Fv/Fm, which is in line with expected changes that reflect the characteristic of higher Fv/Fm associated with larger diatom cells when conditions are favorable (i.e. not light or nutrient-limited) (Boyd et al., 2010; Koch et al., 2019). However, the diatom:haptophyte ratio and Deff continued to increase between the PFZ and SACCZ, but the Fv/Fm decreased, while similar community structure indices were observed between the AZ and SACCZ despite a significant decrease in Fv/Fm. These results imply that on the whole, zonal changes in Fv/Fm are not strongly influenced by community structure (as indexed by pigment ratios and Deff), or that neither the diatom:haptophyte ratio nor Deff is accurately reflecting the main shifts driving Fv/Fm. Sosik and Olson (2002), for example, found no significant differences in Fv/Fm between pico- and nanophytoplankton during late summer in the Pacific SO, despite an observed change in community composition toward nanophytoplankton cells south of the PF, thus favoring instead the role of other drivers.
When investigating the data on an inter-annual basis, a number of differences in the diatom:haptophyte ratio corresponded to expected differences in Fv/Fm. For example, lower Fv/Fm on S48 than S53 in the PFZ corresponded to lower diatom:haptophyte ratios. Similarly, in the SACCZ the highest Fv/Fm which was observed during S54, corresponded to a significantly higher diatom:haptophyte ratio. The same was, however, not true for Deff which was relatively consistent over the years, with almost no influence evident on inter-annual Fv/Fm variability. On the other hand, intra-seasonal patterns were observed in the community structure indices of both Deff and the diatom:haptophyte ratio which decreased (in all instances) from early to late summer, consistent with an expected phytoplankton community response to seasonal declines in nutrients (Hutchins et al., 2001). Changes in Fv/Fm, however, varied per zone and per year with only the SACCZ (all years) and S53 (all zones) showing a coincident seasonal decline in Fv/Fm. Similar to the role of macronutrients, it appears that although we are seeing sensible relationships between changes in Fv/Fm in response to changes in community structure, the lack of consistency suggests that additional factors are concurrently at play.
4.3 The particular case of S53 and S49
Examination of inter-annual variability within the zones showed S53 to be the only year displaying significant differences in Fv/Fm along with SST, N:P and Si:P ratios mainly for the PFZ and AZ compared to all the other cruises (Figure 6). As such, this year provides a unique test case for investigating the possible inter-annual co-variability in Fv/Fm. To begin with, S53 stood out as expressing the second-largest intra-seasonal variability in Fv/Fm (i.e., the second biggest decline in Fv/Fm between December and February), which coincided with a significant decline in both community structure indices (diatom:haptophyte ratio and Deff index). S53 was also notable in displaying the highest mean Fv/Fm in the AZ compared to all other cruises, but counterintuitively showed the lowest SST and nutrient ratios. Finally, in the SACCZ, S53 showed a low mean Fv/Fm coincident with the lowest SST and N:P ratio. In summary, S53 was certainly a unique year, and although it was not always possible to reconcile variability in Fv/Fm with an anticipated driver response, it nonetheless highlighted the important role of community structure, SST and N:P influencing inter-annual variability in Fv/Fm.
S49 also stood out as a year with particularly high Fv/Fm in the SAZ and particularly low Fv/Fm in the SACCZ, as well as the largest intra-seasonal variability (i.e., the biggest decline in Fv/Fm between December and February). As such, we similarly focus on this cruise to see if it can shed light from a co-varying response perspective (Figure 6). In the SAZ, the highest Fv/Fm during S49 coincided with high SST, the lowest N:P ratio, the highest diatom:haptophyte ratio and the largest phytoplankton cell sizes (Deff), implying that despite the low Si:P ratios large diatoms were primarily responsible for high Fv/Fm observed in the SAZ during S49. In the SACCZ, S49 exhibited low mean Fv/Fm coincident with the lowest diatom:haptophyte ratio and Deff, indicative of a possible role for small cells driving low Fv/Fm.
Climate variability associated with an increase in the positive phase of the Southern Annular Mode (Henson et al., 2010; Swart et al., 2014; Marshall et al., 2018) is considered the clearest and most persistent change in Southern Hemisphere climate in the last half century (Polvani et al., 2011) eliciting a poleward shift and increase in the intensity of the westerly winds. Interestingly, the Southern Annular Mode index prior to S49 and S53 was lower compared to all other years (Supplementary Figure S5) (Climate Data Sets, 2022). This could have impacted environmental conditions and subsequently accounted for inter-annual variability in Fv/Fm during those particular years, highlighting the important role that the intensity of the westerly winds likely plays in influencing inter-annual variability of environmental drivers.
4.4 Complex interactions rather than single factors affect photophysiology
In many ways it is unsurprising that the ancillary parameter analyses did not typically reveal a dominant candidate for influencing zonal, inter-annual or intra-seasonal variability in Fv/Fm. This is because there are a multitude of physio-chemical and biological drivers simultaneously impacting phytoplankton photosynthetic efficiency. As such, it is possible that multiple drivers can have an antagonistic impact on Fv/Fm such that the net effect may be zero. One example is the decrease in Fv/Fm in the AZ compared to the PFZ, despite higher Si:P and diatom:haptophyte ratios and larger cell sizes (Deff), demonstrating a possible indirect influence of cold SSTs that suppress any positive response in Fv/Fm to favorable nutrients and community structure adjustments. This complexity is further substantiated with similar Fv/Fm in the SAZ and AZ despite significant differences in all drivers, while no single driver (from those studied here) could account for the significantly higher Fv/Fm observed in the PFZ compared to all other zones. These examples (and the case of S53 in the AZ) in which ancillary parameters cannot always justify the changes seen in Fv/Fm highlights the complex interplay between direct and indirect drivers of phytoplankton physiology (including ones not specifically addressed in this study e.g., iron) acting simultaneously to impact (or not) Fv/Fm variability.
5 Conclusion
Relatively little is known about the temporal and spatial variability of Fv/Fm and its biogeochemical controls in the higher latitudes (Deppeler and Davidson, 2017). This in situ study spans the full latitudinal extent of the Atlantic SO during five summer voyages (2008 – 2016) to assess zonal, inter-annual and intra-seasonal variability in phytoplankton photosynthetic efficiency, Fv/Fm. Zonal variability showed that the PFZ had the highest Fv/Fm, whilst the SACCZ had the lowest. This implies efficient photosynthesis in the PFZ that was the least constrained by environmental conditions and the most likely to generate higher rates of production positively impacting the BCP. Strong inter-annual variability in Fv/Fm was observed in the SACCZ and PFZ, with most years being significantly different, while the AZ exhibited very low inter-annual variability in Fv/Fm with only one year being different from the remaining four. This zonal investigation of inter-annual variability provides a dynamic understanding of phytoplankton regionalization in response to the characteristics of the underlying drivers, which are either strongly seasonal (expressed as low inter-annual variability) or highly variable i.e., more likely influenced by sub-seasonal forcing of the nutrient and light supply (expressed as high inter-annual variability). Intra-seasonal differences in Fv/Fm between early and late summer reflected seasonal nutrient limitation following biological utilization when averaged across all zones and cruises. However, this response was not consistent when considered per zone or per cruise highlighting the likelihood of a dynamic interplay of sub-seasonal variability of environmental drivers. The characteristics of variability were investigated in the context of five biogeochemical ancillary data sets. Although variability appeared to be linked to SST, nutrient ratios and community structure depending on whether the data were grouped per zone or per cruise, no consistent patterns were evident in any single driver-response relationship. These results highlight the complex interplay between eco-physiological drivers and their photophysiological responses, which act simultaneously and oftentimes antagonistically making it difficult to detect a single driver influence from the net effect. This study alludes to how climate change may alter the impact of various environmental factors on phytoplankton photophysiology and, by extent, primary production and the BCP. More in situ studies on the drivers of photophysiology, including further potential key drivers such as iron, will provide a broader understanding of the factors limiting phytoplankton primary production in the SO, as well as assist in predicting how phytoplankton will respond to future conditions that are associated with climate change. Such information is vital for accurately predicting the future role of the SO in the global uptake and regulation of atmospheric CO2.
Data availability statement
The datasets presented in this study can be found in online repositories. The names of the repository/repositories and accession number(s) can be found below: https://zenodo.org/record/6989253; Zenodo, 10.5281/zenodo.6989253.
Author contributions
ST and TR-K conceptualized the study. AS and TR-K performed the data analysis and produced the figures. AS wrote the initial manuscript. ST, SF, AS, and TR-K contributed to the study design, interpretation of the results and writing of the manuscript. All authors contributed to the article and approved the submitted version.
Funding
AS, ST and TR-K were supported through the CSIR’s Southern Ocean Carbon-Climate Observatory (SOCCO) Programme (http://socco.org.za/) funded by the Department of Science and Innovation (DST/CON 0182/2017) and the CSIR’s Parliamentary Grant. We would like to acknowledge funding received from a number of grants from the National Research Foundation, South Africa, grant numbers 110731 (SF), 91313 (SF), 118751 (ST) and 110729 (ST).
Acknowledgments
The authors would like to acknowledge the assistance of the Captain and crew on-board the SA Agulhas I and SA Agulhas II during the SANAE cruises: 48, 49, 53, 54 and 55. The authors would also like to thank the two reviewers for their constructive comments that contributed to refining this manuscript.
Conflict of interest
The authors declare that the research was conducted in the absence of any commercial or financial relationships that could be construed as a potential conflict of interest.
Publisher’s note
All claims expressed in this article are solely those of the authors and do not necessarily represent those of their affiliated organizations, or those of the publisher, the editors and the reviewers. Any product that may be evaluated in this article, or claim that may be made by its manufacturer, is not guaranteed or endorsed by the publisher.
Supplementary material
The Supplementary Material for this article can be found online at: https://www.frontiersin.org/articles/10.3389/fmars.2022.912856/full#supplementary-material
References
Arrigo K. R., Mills M. M., Kropuenske L. R., van Dijken G. L., Alderkamp A. C., Robinson D. H. (2010). Photophysiology in two major southern ocean phytoplankton taxa: photosynthesis and growth of phaeocystis antarctica and fragilariopsis cylindrus under different irradiance levels. Integr. Comp. Biol. 50, 950–966. doi: 10.1093/icb/icq021
Arrigo K. R., Robinson D. H., Worthen D. L., Dunbar R. B., DiTullio G. R., VanWoert M., et al. (1999). Phytoplankton community structure and the drawdown of nutrients and CO2 in the southern ocean. Science 283, 365–367. doi: 10.1126/science.283.5400.365
Arrigo K. R., van Dijken G. L., Bushinsky S. (2008). Primary production in the Southern Ocean 1997-2006. J. Geophys. Res. Ocean 113 (C8). doi: 10.1029/2007JC004551
Barlow R. G., Cummings D. G., Gibb S. W. (1997). Improved resolution of mono-and divinyl chlorophylls a and b and zeaxanthin and lutein in phytoplankton extracts using reverse phase c-8 HPLC. Mar. Ecol. Prog. Ser. 161, 303–307. doi: 10.3354/meps161303
Bigg G. R., Killworth P. D. (1988). Conservative tracers and the ocean circulation. Philos. Trans. R. Soc London. Ser. A Math. Phys. Sci. 325, 177–187. doi: 10.1098/rsta.1988.0050
Boyd P. W. (2002). Environmental factors controlling phytoplankton processes in the southern ocean. J. Phycol. 38, 844–861. doi: 10.1046/j.1529-8817.2002.t01-1-01203.x
Boyd P. W., Strzepek R., Fu F., Hutchins D. A. (2010). Environmental control of open ocean phytoplankton groups: Now and in the future. Limnol. Oceanogr. 55, 1353–1376. doi: 10.4319/lo.2010.55.3.1353
Boyer T. P., Antonov J. I., Baranova O. K., Coleman C., Garcia H. E., Grodsky A., et al. (2013). World ocean database 2013. Silver Spring, MD: National Oceanographic Data Center, 208pp. (NOAA Atlas NESDIS, 72). doi: 10.25607/OBP-1454
Brody S. R., Lozier M. S. (2014). Changes in dominant mixing length scales as a driver of subpolar phytoplankton bloom initiation in the north Atlantic. Geophys. Res. Lett. 41, 3197–3203. doi: 10.1002/2014GL059707
Climatedataguide.ucar.edu (2022) Climate data sets | NCAR - climate data guide. Available at: https://climatedataguide.ucar.edu/climate-data.
Cullen J. J., Davis R. F. (2003). The blank can make a big difference in oceanographic measurements. Limnol. Oceanogr. Bull. 12, 29–35. doi: 10.1002/lob.200413229
de Baar H. J., Buma A. G., Nolting R., Cadee G., Jacques G., Treguer P. (1990). On iron limitation of the southern ocean: experimental observations in the weddell and Scotia seas. Mar. Ecol. Prog. Ser. 65, 105–122. doi: 10.3354/meps065105
Demidov A. B., Mosharov S. A., Gagarin V. I. (2012). Meridional asymmetric distribution of the primary production in the Atlantic sector of the southern ocean in the austral spring and summer. Oceanology 52, 623–634. doi: 10.1134/S0001437012050050
Deppeler S. L., Davidson A. T. (2017). Southern ocean phytoplankton in a changing climate. Front. Mar. Sci. 4. doi: 10.3389/fmars.2017.00040
DeVries T., Weber T. (2017). The export and fate of organic matter in the ocean: New constraints from combining satellite and oceanographic tracer observations. Global Biogeochem. Cycles 31, 535–555. doi: 10.1002/2016GB005551
Durack P. J., Wijffels S. E. (2010). Fifty-year trends in global ocean salinities and their relationship to broad-scale warming. J. Clim. 23, 4342–4362. doi: 10.1175/2010JCLI3377.1
Egan L. (2008). QuickChem method 31-107-04-1-C – nitrate and/or nitrite in brackish or seawater (Colorado, USA: Lachat Instruments).
Falkowski P. G. (1997). Evolution of the nitrogen cycle and its influence on the biological sequestration of CO2 in the ocean. Nature 387, 272–275. doi: 10.1038/387272a0
Feng Y., Hare C. E., Leblanc K., Rose J. M., Zhang Y., DiTullio G. R., et al. (2009). Effects of increased pCO2 and temperature on the north Atlantic spring bloom. i. the phytoplankton community and biogeochemical response. Mar. Ecol. Prog. Ser. 388, 13–25. doi: 10.3354/meps08133
Finkel Z. V., Beardall J., Flynn K. J., Quigg A., Rees T. A. V., Raven J. A. (2010). Phytoplankton in a changing world: cell size and elemental stoichiometry. J. Plankton Res. 32, 119–137. doi: 10.1093/plankt/fbp098
GEOTRACES Intermediate Data Product Group (2021). The GEOTRACES intermediate data product 2021 (IDP2021). (United Kingdom: NERC EDS British Oceanographic Data Centre NOC). doi: 10.5285/cf2d9ba9-d51d-3b7c-e053-8486abc0f5fd
Gibberd M. J., Kean E., Barlow R., Thomalla S., Lucas M. (2013). Phytoplankton chemotaxonomy in the Atlantic sector of the southern ocean during late summer 2009. Deep. Res. Part I Oceanogr. Res. Pap. 78, 70–78. doi: 10.1016/j.dsr.2013.04.007
Gorbunov M., Falkowski P. (2004). “Fluorescence induction and relaxation (FIRe) technique and instrumentation for monitoring photosynthetic processes and primary production in aquatic ecosystems,” in 13th international congress of photosynthesis (Montreal, QC: Allen Press), 1029–1031.
Grasshoff K., Ehrhardt M., Kremling K. (1983). Methods of seawater analysis. 2nd ed (Verlag Chemie, Weinhein, New York) 14 (1), 419.
Hardman-Mountford N. J., Polimene L., Hirata T., Brewin R. J., Aiken J. (2013). Impacts of light shading and nutrient enrichment geo-engineering approaches on the productivity of a stratified, oligotrophic ocean ecosystem. J. R Soc. Interface 10 (89), 20130701. doi: 10.1098/rsif.2013.0701
Harris J. (2006). Antarctic marine protist” 2005th ed in Arctic, Antarctic, And alpine research. Eds. Scott FJ, Marchant HJ. Canberra: Australian biological resources Study/Australian Antarctic division. 563 pp. doi: 10.1657/1523-0430(2006)38[301b:br]2.0.co;2
Hauck J., Völker C., Wolf-Gladrow D. A., Laufkötter C., Vogt M., Aumont O., et al. (2015). On the southern ocean CO2 uptake and the role of the biological carbon pump in the 21st century. Global Biogeochem. Cycles 29, 1451–1470. doi: 10.1002/2015GB005140
Heiden J. P., Völkner C., Jones E. M., van de Poll W. H., Buma A. G., Meredith M. P., et al. (2019). Impact of ocean acidification and high solar radiation on productivity and species composition of a late summer phytoplankton community of the coastal Western Antarctic peninsula. Limnol. Oceanogr. 64, 1716–1736. doi: 10.1002/lno.11147
Henson S. A., Sarmiento J. L., Dunne J. P., Bopp L., Lima I., Doney S. C., et al. (2010). Detection of anthropogenic climate change in satellite records of ocean chlorophyll and productivity. Biogeosciences 7, 621–640. doi: 10.5194/bg-7-621-2010
Hughes D. J., Douglas A., Campbell M. A., Doblin J. C., Kromkamp E., Lawrenz C., et al. (2018). Roadmaps and detours: Active chlorophyll- a assessments of primary productivity across marine and freshwater systems. Environ. Sci. Technol. 52, 12039–12054. doi: 10.1021/acs.est.8b03488
Hutchins D. A., Boyd P. W. (2016). Marine phytoplankton and the changing ocean iron cycle. Nat. Clim. Change 6, 1072–1079. doi: 10.1038/nclimate3147
Hutchins D. A., Sedwick P. N., DiTullio G. R., Boyd P. W., Queguiner B., Griffiths F. B., et al. (2001). Control of phytoplankton growth by iron and silicic acid availability in the subantarctic southern ocean: Experimental results from the SAZ project. J. Geophys. Res. Ocean. 106, 31559–31572. doi: 10.1029/2000JC000333
Juneau P., Harrison P. J. (2005). Comparison by PAM fluorometry of photosynthetic activity of nine marine phytoplankton grown under identical conditions. Photochem. Photobiol. 81, 649–653. doi: 10.1562/2005-01-13-RA-414.1
Koch F., Beszteri S., Harms L., Trimborn S. (2019). The impacts of iron limitation and ocean acidification on the cellular stoichiometry, photophysiology, and transcriptome of phaeocystis antarctica. Limnol. Oceanogr. 64, 357–375. doi: 10.1002/lno.11045
Kolber Z. S., Falkowski P. G. (1992). “Fast repetition rate (FRR) fluorometer for making In situ measurements of primary productivity,” in OCEANS 92 Proceedings in Mastering the Oceans Through Technology. (Brookhaven National Lab., Upton, NY (United States: IEEE). 637–641. doi: 10.1109/OCEANS.1992.607657
Kolber Z. S., Prášil O., Falkowski P. G. (1998). Measurements of variable chlorophyll fluorescence using fast repetition rate techniques: defining methodology and experimental protocols. Biochim. Biophys. Acta - Bioenerg. 1367, 88–106. doi: 10.1016/S0005-2728(98)00135-2
Le Quéré C., Takahashi T., Buitenhuis E. T., Rödenbeck C., Sutherland S. C. (2010). Impact of climate change and variability on the global oceanic sink of CO2. Global Biogeochemical Cycles 24 (4), GB4007. doi: 10.1029/2009GB003599
Mackey M. D., Mackey D. J., Higgins H. W., Wright S. W. (1996). CHEMTAX - a program for estimating class abundances from chemical markers: Application to HPLC measurements of phytoplankton. Mar. Ecol. Prog. Ser. 144, 265–283. doi: 10.3354/meps144265
Mahadevan A., D’asaro E., Lee C., Perry M. J. (2012). Eddy-driven stratification initiates north Atlantic spring phytoplankton blooms. Science 337, 54–58. doi: 10.1126/science.1218740
Marañón E., Lorenzo M. P., Cermeño P., Mouriño-Carballido B. (2018). Nutrient limitation suppresses the temperature dependence of phytoplankton metabolic rates. ISME J. 12, 1836–1845. doi: 10.1038/s41396-018-0105-1
Marshall A. G., Hemer M. A., Hendon H. H., McInnes K. L. (2018). Southern annular mode impacts on global ocean surface waves. Ocean Model. 129, 58–74. doi: 10.1016/j.ocemod.2018.07.007
Matebr R. J., Hirst A. C. (1999). Climate change feedback on the future oceanic CO2 uptake. Tellus B 51, 722–733. doi: 10.1034/j.1600-0889.1999.t01-1-00012.x
Maxwell K., Johnson G. N. (2000). Chlorophyll fluorescence - a practical guide. J. Exp. Bot. 51, 659–668. doi: 10.1093/jxb/51.345.659
Moore C. M., Mills M. M., Arrigo K. R., Berman-Frank I., Bopp L., Boyd P. W., et al. (2013). Processes and patterns of oceanic nutrient limitation. Nat. Geosci. 6, 701–710. doi: 10.1038/ngeo1765
Moore C. M., Seeyave S., Hickman A. E., Allen J. T., Lucas M. I., Planquette H., et al. (2007). Iron-light interactions during the CROZet natural iron bloom and EXport experiment (CROZEX) I: Phytoplankton growth and photophysiology. Deep. Res. Part II Top. Stud. Oceanogr. 54, 2045–2065. doi: 10.1016/j.dsr2.2007.06.011
Morel F. M. M., Price N. M. (2003). The biogeochemical cycles of trace metals in the oceans. Science 300, 944–947. doi: 10.1126/science.1083545
Mtshali T. N., van Horsten N. R., Thomalla S. J., Ryan-Keogh T. J., Nicholson S. A., Roychoudhury, et al. (2019). Seasonal depletion of the dissolved iron reservoirs in the Sub-Antarctic zone of the southern Atlantic ocean. Geophys. Res. Lett. 46, 4386–4395. doi: 10.1029/2018GL081355
Nunn B. L., Faux J. F., Hippmann A. A., Maldonado M. T., Harvey H. R., Goodlett D. R., et al. (2013). Diatom proteomics reveals unique acclimation strategies to mitigate fe limitation. PloS One 8 (10), e75653. doi: 10.1371/journal.pone.0075653
Orsi A. H., Whitworth T. III, Nowlin W. D. Jr. (1995). On the meridional extent and fronts of the Antarctic circumpolar current. Deep Sea Res. Part I Oceanogr. Res. Pap. 42, 641–673. doi: 10.1016/0967-0637(95)00021-W
Owens T. G., Falkowski P. G., Whitledge T. E. (1980). Diel periodicity in cellular chlorophyll content in marine diatoms. Mar. Biol. 59, 71–77. doi: 10.1007/BF00405456
Parsons T., Maita Y., Lalli C. (1984). A manual of chemical and biological methods for seawater analysis (Oxford: Pergamon).
Petrou K., Kranz S. A., Trimborn S., Hassler C. S., Ameijeiras S. B., Sackett O., et al. (2016). Southern ocean phytoplankton physiology in a changing climate. J. Plant Physiol. 203, 135–150. doi: 10.1016/j.jplph.2016.05.004
Pollard R. T., Lucas M. I., Read J. F. (2002). Physical controls on biogeochemical zonation in the southern ocean. Deep Sea Res. Part II Top. Stud. Oceanogr. 49, 3289–3305. doi: 10.1016/S0967-0645(02)00084-X
Polvani L. M., Waugh D. W., Correa G. J., Son S. W. (2011). Stratospheric ozone depletion: The main driver of twentieth-century atmospheric circulation changes in the southern hemisphere. J. Clim. 24, 795–812. doi: 10.1175/2010JCLI3772.1
Raschka S. (2018). MLxtend: Providing machine learning and data science utilities and extensions to python’s scientific computing stack. J. Open Source Softw. 3 (24), 638. doi: 10.21105/joss.00638
Ras J., Claustre H., Uitz J. (2008). Spatial variability of phytoplankton pigment distributions in the subtropical south pacific ocean: comparison between in situ and predicted data. Biogeosciences 5, 353–369. doi: 10.5194/bgd-4-3409-2007
Raven J. A., Evans M. C. W., Korb R. E. (1999). The role of trace metals in photosynthetic electron transport in O-2-evolving organisms. Photosynth. Res. 60, 111–149. doi: 10.1023/a:1006282714942
Rio M. H., Guinehut S., Larnicol G. (2011). New CNES-CLS09 global mean dynamic topography computed from the combination of GRACE data, altimetry, and in situ measurements. J. Geophys. Res. Ocean. 116 (C7). doi: 10.1029/2010JC006505
Ryan-Keogh T., Robinson C. (2021). Phytoplankton photophysiology utilities: A Python toolbox for the standardization of processing active chlorophyll-a fluorescence data. Front. Mar. Sci. Aquat. Physiol. 8. doi: 10.3389/fmars.2021.525414
Ryan-Keogh T. J., Thomalla S. J., Mtshali T. N., Little H. (2017). Modelled estimates of spatial variability of iron stress in the Atlantic sector of the southern ocean. Biogeosciences 14, 3883–3897. doi: 10.5194/bg-14-3883-2017
Ryan-Keogh T. J., Thomalla S. J., Mtshali T. N., Van Horsten N. R., Little H. J. (2018). Seasonal development of iron limitation in the sub-Antarctic zone. Biogeosciences 15, 4647–4660. doi: 10.5194/bg-15-4647-2018
Sallée J. B., Pellichero V., Akhoudas C., Pauthenet E., Vignes L., Schmidtko S., et al. (2021). Summertime increases in upper-ocean stratification and mixed-layer depth. Nature 591, 592–598. doi: 10.1038/s41586-021-03303-x
Schoffman H., Lis H., Shaked Y., Keren N. (2016). Iron–Nutrient Interactions within Phytoplankton. Front. Plant Sci. 7, 1223. doi: 10.3389/fpls.2016.01223
Sedwick P., Blain S., Quéguiner B., Griffiths F., Fiala M., Bucciarelli E., et al. (2002). Resource limitation of phytoplankton growth in the crozet basin, subantarctic southern ocean. Deep Sea Res. Part II: Topical Stud. Oceanogr. 49 (16), 3327–3349. doi: 10.1016/S0967-0645(02)00086-3
Shen A., Ma Z., Jiang K., Li D. (2016). Effects of temperature on growth, photophysiology, rubisco gene expression in prorocentrum donghaiense and karenia mikimotoi. Ocean Sci. J. 51, 581–589. doi: 10.1007/s12601-016-0056-2
Sosik H. M., Olson R. J. (2002). Phytoplankton and iron limitation of photosynthetic efficiency in the southern ocean during late summer. Deep Sea Res. Part I Oceanogr. Res. Pap. 49, 1195–1216. doi: 10.1016/S0967-0637(02)00015-8
Sow S., Trull T., Bodrossy L. (2020). Oceanographic fronts shape phaeocystis assemblages: A high-resolution 18S rRNA gene survey from the ice-edge to the equator of the south pacific. Front. Microbiol. 11, 1847. doi: 10.3389/fmicb.2020.01847
Spackeen J. L., Bronk D. A., Sipler R. E., Bertrand E. M., Hutchins D. A., Allen A. E. (2018). Stoichiometric N:P ratios, temperature, and iron impact carbon and nitrogen uptake by Ross Sea microbial communities. J. Geophys. Res. Biogeosci. 123, 2955–2975. doi: 10.1029/2017JG004316
Strzepek R. F., Boyd P. W., Sunda W. G. (2019). Photosynthetic adaptation to low iron, light, and temperature in southern ocean phytoplankton. Proc. Natl. Acad. Sci. 116, 4388–4393. doi: 10.1073/pnas.1810886116
Strzepek R. F., Hunter K. A., Frew R. D., Harrison P. J., Boyd P. W. (2012). Iron-light interactions differ in southern ocean phytoplankton. Limnol. Oceanogr. 57, 1182–1200. doi: 10.4319/lo.2012.57.4.1182
Suggett D., Kraay G., Holligan P., Davey M., Aiken J., Geider R. (2001). Assessment of photosynthesis in a spring cyanobacterial bloom by use of a fast repetition rate fluorometer. Limnol. Oceanogr. 46, 802–810. doi: 10.4319/lo.2001.46.4.0802
Suggett D. J., Moore C. M., Hickman A. E., Geider R. J. (2009). Interpretation of fast repetition rate (FRR) fluorescence: Signatures of phytoplankton community structure versus physiological state. Mar. Ecol. Prog. Ser. 376, 1–19. doi: 10.3354/meps07830
Suggett D. J., Moore C. M., Marañón E., Omachi C., Varela R. A., Aiken J., et al. (2006). Photosynthetic electron turnover in the tropical and subtropical Atlantic ocean. Deep. Res. Part II Top. Stud. Oceanogr. 53, 1573–1592. doi: 10.1016/j.dsr2.2006.05.014
Sunda W. G., Huntsman S. A. (1995). Iron uptake and growth limitation in oceanic and coastal phytoplankton. Mar. Chem. 50, 189–206. doi: 10.1016/0304-4203(95)00035-P
Sunda W. G., Huntsman S. A. (1997). Interrelated influence of iron, light and cell size on marine phytoplankton growth. Nature 390, 389–392. doi: 10.1038/37093
Swart N. C., Fyfe J. C., Saenko O. A., Eby M. (2014). Wind-driven changes in the ocean carbon sink. Biogeosciences 11, 6107–6117. doi: 10.5194/bg-11-6107-2014
Swart S., Thomalla S., Monteiro P., Ansorge I. (2012). Mesoscale features and phytoplankton biomass at the GoodHope line in the southern ocean during austral summer. Afr. J. Mar. Sci. 34, 511–524. doi: 10.2989/1814232X.2012.749811
Tagliabue A., Sallée J. B., Bowie A. R., Lévy M., Swart S., Boyd P. W. (2014). Surface-water iron supplies in the southern ocean sustained by deep winter mixing. Nat. Geosci. 7, 314–320. doi: 10.1038/ngeo2101
Takahashi T., Sutherland S. C., Sweeney C., Poisson A., Metzl N., Tilbrook B., et al. (2002). Global sea–air CO2 flux based on climatological surface ocean pCO2, and seasonal biological and temperature effects. Deep Sea Res. Part II Top. Stud. Oceanogr. 49, 1601–1622. doi: 10.1016/S0967-0645(02)00003-6
Thomalla S. J., Fauchereau N., Swart S., Monteiro P. M. (2011). Regional scale characteristics of the seasonal cycle of chlorophyll in the southern ocean. Biogeosciences 8, 2849–2866. doi: 10.5194/bg-8-2849-2011
Thomalla S., Kean E., Lucas M., Gibberd M. J., Barlow R. (2017). Photosynthesis versus irradiance relationships in the Atlantic sector of the southern ocean. Afr. J. Mar. Sci. 39, 43–57. doi: 10.2989/1814232X.2017.1303399
Timmermans K., Stolte W., de Baar H. (1994). Iron-mediated effects on nitrate reductase in marine phytoplankton. Mar. Biol. 121 (2), 389–396. doi: 10.1007/BF00346749
Timmermans K. R., van der Woerd H. J., Wernand M. R., Sligting M., Uitz J., de Baar H. J. (2008). In situ and remote-sensed chlorophyll fluorescence as indicator of the physiological state of phytoplankton near the isles kerguelen (Southern ocean). Polar Biol. 31 (5), 617–628. doi: 10.1007/s00300-007-0398-4
Uitz J., Claustre H., Morel A., Hooker S. B. (2006). Vertical distribution of phytoplankton communities in open ocean: An assessment based on surface chlorophyll. J. Geophys. Res. Ocean. 111 (C8). doi: 10.1029/2005JC003207
Vidussi F., Claustre H., Manca B. B., Luchetta A., Jean-Claude M. (2001). Phytoplankton pigment distribution in relation to upper Francesca claustre for the whole tchl a concentration mg estimated production value being mg m and the highest picophytoplankton contribution of tchl a gyres by low tchl a concentrations. J. Geophys. Res. 106, 939–956. doi: 10.1038/nm0497-414
Viljoen J. J., Philibert R., Van Horsten N., Mtshali T., Roychoudhury A. N., Thomalla S., et al. (2018). Phytoplankton response in growth, photophysiology and community structure to iron and light in the polar frontal zone and Antarctic waters. Deep Sea Res. Part I Oceanogr. Res. Pap. 141, 118–129. doi: 10.2495/EEIA100071
Welschmeyer N. A. (1994). Fluorometric analysis of chlorophyll a in the presence of chlorophyll b and pheopigments. Limnol. Oceanogr. 39, 1985–1992. doi: 10.4319/lo.1994.39.8.1985
Wolters M. (2002). Quickchem method 31-114-27-1-D – silicate in brackish or seawater (Colorado, USA: Lachat Instruments).
Wright S. W., van den Enden R. L., Pearce I., Davidson A. T., Scott F. J., Westwood K. J. (2010). Phytoplankton community structure and stocks in the southern ocean (30–80 e) determined by CHEMTAX analysis of HPLC pigment signatures. Deep Sea Res. Part II Top. Stud. Oceanogr. 57, 758–778. doi: 10.1016/j.dsr2.2009.06.015
Keywords: Fast Induction and Relaxation (FIRe) fluorometry, fluorescence, physical and biogeochemical parameters, phytoplankton photophysiology, photosynthetic efficiency, zonal variability, inter-annual variability, intra-seasonal variability
Citation: Singh A, Thomalla SJ, Fietz S and Ryan-Keogh TJ (2022) Spatial and temporal variability of phytoplankton photophysiology in the Atlantic Southern Ocean. Front. Mar. Sci. 9:912856. doi: 10.3389/fmars.2022.912856
Received: 04 April 2022; Accepted: 16 September 2022;
Published: 12 October 2022.
Edited by:
Alex J. Poulton, Heriot-Watt University, United KingdomReviewed by:
Mitsuhide Sato, Nagasaki University, JapanSven Alexander Kranz, Florida State University, United States
Copyright © 2022 Singh, Thomalla, Fietz and Ryan-Keogh. This is an open-access article distributed under the terms of the Creative Commons Attribution License (CC BY). The use, distribution or reproduction in other forums is permitted, provided the original author(s) and the copyright owner(s) are credited and that the original publication in this journal is cited, in accordance with accepted academic practice. No use, distribution or reproduction is permitted which does not comply with these terms.
*Correspondence: Thomas J. Ryan-Keogh, dHJ5YW5rZW9naEBjc2lyLmNvLnph