- State Key Laboratory of Marine Environmental Science, Fujian Key Laboratory of Marine Carbon Sequestration, College of Ocean and Earth Science, Xiamen University, Xiamen, China
Owing to serious environmental and climatic impacts of increasing carbon dioxide (CO2) concentrations, there is an urgent need for the development of efficient CO2 capture methods. Carbonic anhydrases (CAs) can mediate CO2 capture via a rapid reaction between CO2 and bicarbonate ions. However, because of their stability, most of the CAs are not suitable for use in hostile environments (high temperature, high alkalinity, high pressure, and solvent). Therefore, this review explores thermophilic microorganisms in submarine hydrothermal environments as a valuable source of thermostable tolerant CAs, and highlights the questions and future directions that must be addressed for the application of CAs in CO2 capture.
Introduction
Continuous increases in carbon dioxide (CO2) concentrations have significantly affected the environment and climate, with the current global atmospheric CO2 levels reaching nearly 420 ppm (Min et al., 2016; Schweitzer et al., 2021). The estimated value for global CO2 emissions is about 48 gigatons per year, and the CO2 emissions must be reduced to < 5 gigatons per year by 2050 to achieve the goal of limiting global temperature rise to 2°C by 2100 (Valluri et al., 2022). However, reduction of CO2 concentrations via conventional methods, including energy conversion, afforestation, clean energy, or carbon-free energy is very low (Nlü et al., 2021). By contrast, carbon capture, utilization, and sequestration (CCUS) is an essential technical solution with high CO2 emission reduction potential (Hasan et al., 2015). Carbon capture is a key process in CCUS, and the cost of CO2 reduction could increase by about 140% if carbon capture and storage technologies are not considered (Osman et al., 2020). The CO2 capture technologies include chemical absorption (e.g., using aqueous amine solutions) (Yu et al., 2012), physical absorption (e.g., using activated carbon and natural ores or solid wastes (steel slag)) (Olivares-Marin and Maroto-Valer, 2012; Chen et al., 2014), and biological methods (e.g., plant photosynthesis) (Klinthong et al., 2015). However, these methods present challenges related to solvent degradation, volatility, and corrosion, requirement of large amount of adsorbent, and long uptake period of plant culture, which must be addressed (Nguyen et al., 2010; Yu et al., 2012). Meanwhile, new techniques for CO2 capture should also be explored, and methods for highly specific and rapid enzymatic conversion of CO2 to other stable compounds using carbonic anhydrases (CAs) show promising potential (Ren et al., 2020).
CAs promote the precipitation of ore ions as carbonates for the purpose of capturing CO2. They can effectively catalyze the reversible hydration of CO2 to achieve rapid reaction between CO2 and bicarbonate ions (HCO3−) (Bhattacharya et al., 2003), which subsequently targets appropriate metal ions to precipitate environmental-friendly solid carbonates. These metal ions are supplied by natural minerals rich in magnesium and calcium (wollastonite, forsterite, serpentine, steel slag, etc., with known reserves of 300, 800, 500, and 240 million tons, respectively) (Liu et al., 2021). The large quantities and wide distribution of these natural minerals allow CAs to capture CO2 as carbonates in large-scale practical applications (Figure 1). The utilization of CAs for CO2 capture in CCUS allows faster CO2 absorption, permits the use of smaller process equipment, and reduces more energy losses during the capture process (Alvizo et al., 2014). However, large-scale carbon capture processes with CAs are limited by enzymatic stability at higher operating temperature and alkaline pH. CAs derived from microorganisms living in extreme environments have the characteristic of resistance to severe conditions, and are gradually emerging as a promising candidate for practical application of CO2 capture.
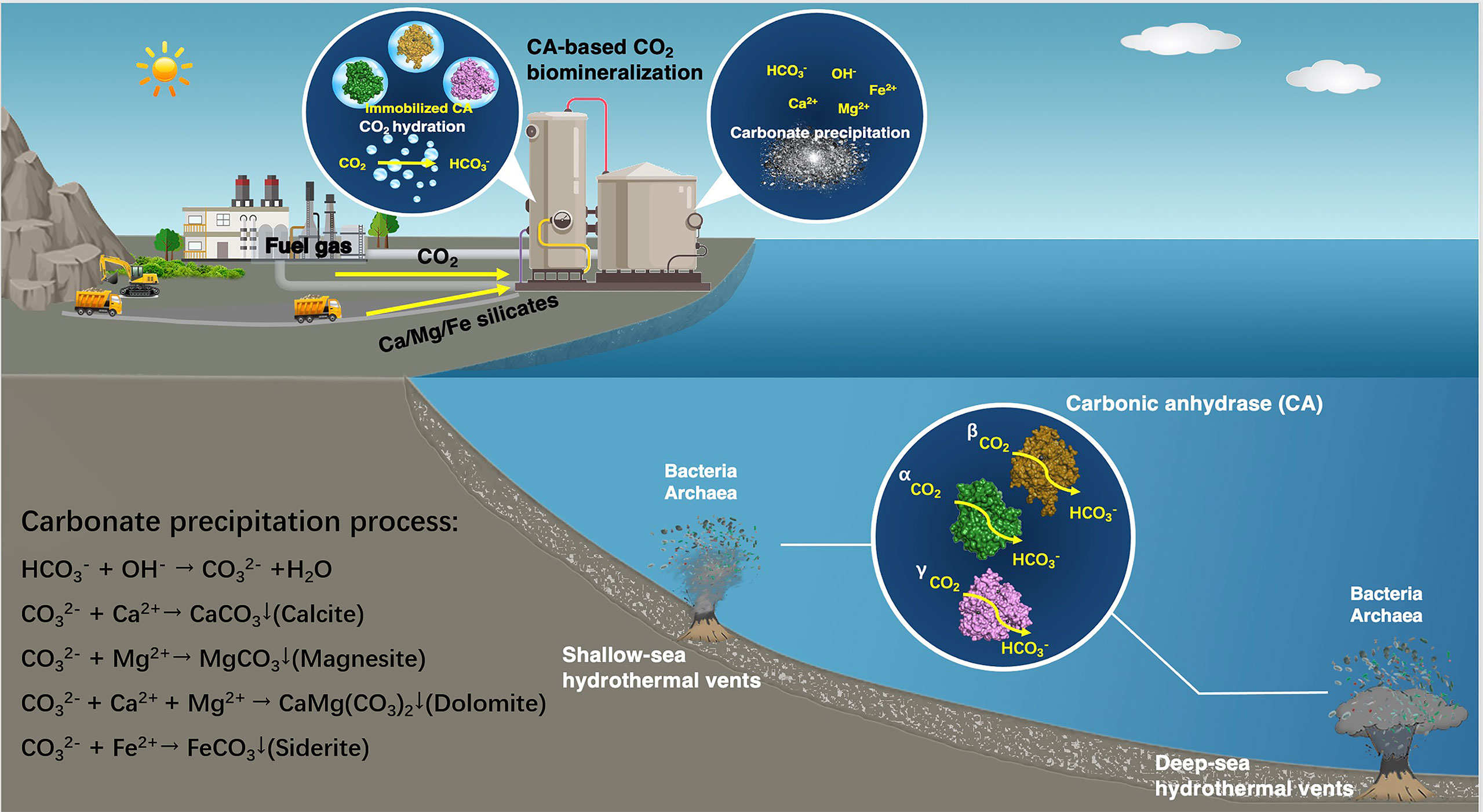
Figure 1 Schematic of the application of CAs from microorganisms thriving in submarine hydrothermal environment to CO2 sequestration.
Extremophiles as Source of CAs for CO2 Capture in CCUS
CAs are widely distributed in eukaryotes and prokaryotes, such as mammalian tissues, plants (Floryszak-Wieczorek and Arasimowicz-Jelonek, 2017), algae (Hewett-Emmett and Tashian, 1996), bacteria (Claudiu and Clemente, 2017), and archaea (Smith and Ferry, 2000), and have produced satisfactory outcomes in biomedical applications (Kaar et al., 2007; Kumar et al., 2021), such as biosensors (Thompson et al., 2000), physiological diagnoses (Supuran, 2011, Jonsson and Liljas, 2020), etc. In recent years, more attention has been paid to develop strategies to mediate carbon sequestration via microbial CAs. On the one hand, CAs can specifically recognize its substrate, CO2, from complex gases and react with it very quickly. On the other hand, the rapid microbial growth can help in the industrial production of CAs. To date, nearly 60 different CAs from bacterial and archaeal domains have been characterized (Bhagat et al., 2018), and most remain active only in a temperature range of < 65°C (Joel et al., 2015; Bhagat et al., 2018). However, the temperature of CO2-containing gas stream after combustion can reach > 100°C during the CO2 capture process (Himadri and Tulasi, 2017), and exposure of CAs to high-temperature or alkaline conditions during CO2 capture can result in severe performance reduction and even denaturation of CAs. Therefore, CAs resistant to high temperature and high alkalinity are needed for CO2 capture.
The temperature and pressure of submarine hydrothermal environments can reach about 350–407°C and about 298 bar, respectively (Koschinsky et al., 2008), and the abundant microbial populations in these ecosystems exhibit high temperature and pressure tolerance (Dick, 2019). When compared with some of the CAs from non-thermophilic bacteria (shorter half-lives and lower enzymatic activity at temperature ≥ 60°C) (Bhagat et al., 2018), thermophilic bacteria (optimum temperature ≥ 75°C) and hyperthermophilic bacteria (optimum temperature ≥ 80°C) that thrive in hydrothermal environments can be a source of heat-resistant CAs (Zeldes et al., 2015). For example, the most representative thermostable α-CA (around 100°C) isolated from the thermophilic bacterium Sulfurihydrogenibium azorense Az-Fu1 has been reported to present the highest CO2 hydratase activity (kcat = 4.40 × 106 s−1) among the known CAs (Luca et al., 2013), indicating that excellent CAs can be obtained from microorganisms living in hydrothermal environments.
A number of thermally stable CAs have been used to investigate the capture of CO2 from flue gas. For instance, CA from Desulfovibrio vulgaris has been used in the adsorption process of amines to accelerate the removal of CO2 from flue gas, and a 25-fold increase in the CO2 absorption rate has been achieved after CA addition (Alvizo et al., 2014). Furthermore, CA from Sulfurihydrogenibium yellowstonense YO3AOP1 has been utilized to capture CO2 from flue gas (Rossi, 2013), and has been subsequently immobilized in polyurethane foam for CO2 biomimetic absorption tests by three-phase trickle-bed reactor (Migliardini et al., 2013). These studies show that CAs from thermophilic microorganisms can be practically applied to the CO2 capture process.
Diversity of Microbial CAs in Submarine Hydrothermal Environments
The known hydrothermal environments include 61 shallow-sea hydrothermal vents (water depth < 200 m) and 201 deep-sea hydrothermal vents (water depth ≥ 200 m), which are widely distributed all over the world (Figure 2A). Analysis and genomic investigations of different CAs-producing microbial strains in submarine hydrothermal environments have shown that CAs-producing strains are commonly found in the classes Epsilonproteobacteria, Gammaproteobacteria, Methanococci, Aquificae, and Thermodesulfobacteria, with majority of the strains belonging to the class Epsilonproteobacteria. These microbial strains possess genes encoding α-, β-, and γ-CAs, with genes encoding β- and γ-CAs being predominant. In addition, many of these microbial strains can even possess genes encoding two or three types of CAs simultaneously (Figure 2B). At present, α-CAs-producing microorganisms successfully isolated from submarine hydrothermal environment mainly include S. yellowstonense YO3AOP1 (Capasso et al., 2012), S. azorense Az-Fu1 (Luca et al., 2013), Thermovibrio ammonificans HB-1 (James et al., 2014), Persephonella marina EX-H1 (Kanth et al., 2014), Hydrogenovibrio crunogena XCL-2 (Chingkuang et al., 2015). β-CAs-producing microorganisms include Methanobacterium thermoautotrophicum ΔH (Smith and Ferry, 1999), and γ-CAs-producing microorganisms include Methanosarcina thermophila TM-1 (Alber and Ferry, 1994; Capasso et al., 2012) and Aeribacillus pallidus TSHB1 (Bose and Satyanarayana, 2016) (Figure 2C). The current research on CAs is mainly focused on enzyme adaptation to alkaline pH and high temperature. The CA isolated from A. pallidus TSHB1 has been known to survive in the most alkaline environment with a pH of 11, while CAs isolated from S. yellowstonense YO3AOP1, S. azorense Az-Fu1, P. marina EX-H1, and H. crunogena XCL-2 have been recognized to be the most resistant to high temperatures, with the ability to maintain certain activity under 100°C. Overall, although the number of gene sequences encoding α-CAs is not dominant, α-CAs are the major CAs isolated and characterized with higher temperature tolerance than β- and γ-CAs, and the majority of α-CAs are known to remain catalytically active at around 100°C. It has been reported that the presence of an N-terminal signal peptide is a common feature of all known bacterial α-CAs, suggesting that α-CAs are located in the periplasmic space and are responsible for converting diffused CO2 into HCO3−, which is essential for bacterial metabolism. By contrast, β- and γ-CAs are located in the cytoplasmic space and are responsible for providing CO2 for carboxylase enzymes, pH homeostasis, and other intracellular functions (Capasso and Supuran, 2015; Supuran and Capasso, 2016). As α-CAs are more readily released from the periplasmic space of bacteria, with most of these enzymes having higher catalytic constants (kcat≈ 105–106 s−1) than γ- and β-CAs (kcat ≈ 104 s−1), α-CAs are more exploitable, and further research on engineering or immobilization of microbial CAs could lead to the development of more effective CAs for CO2 capture applications.
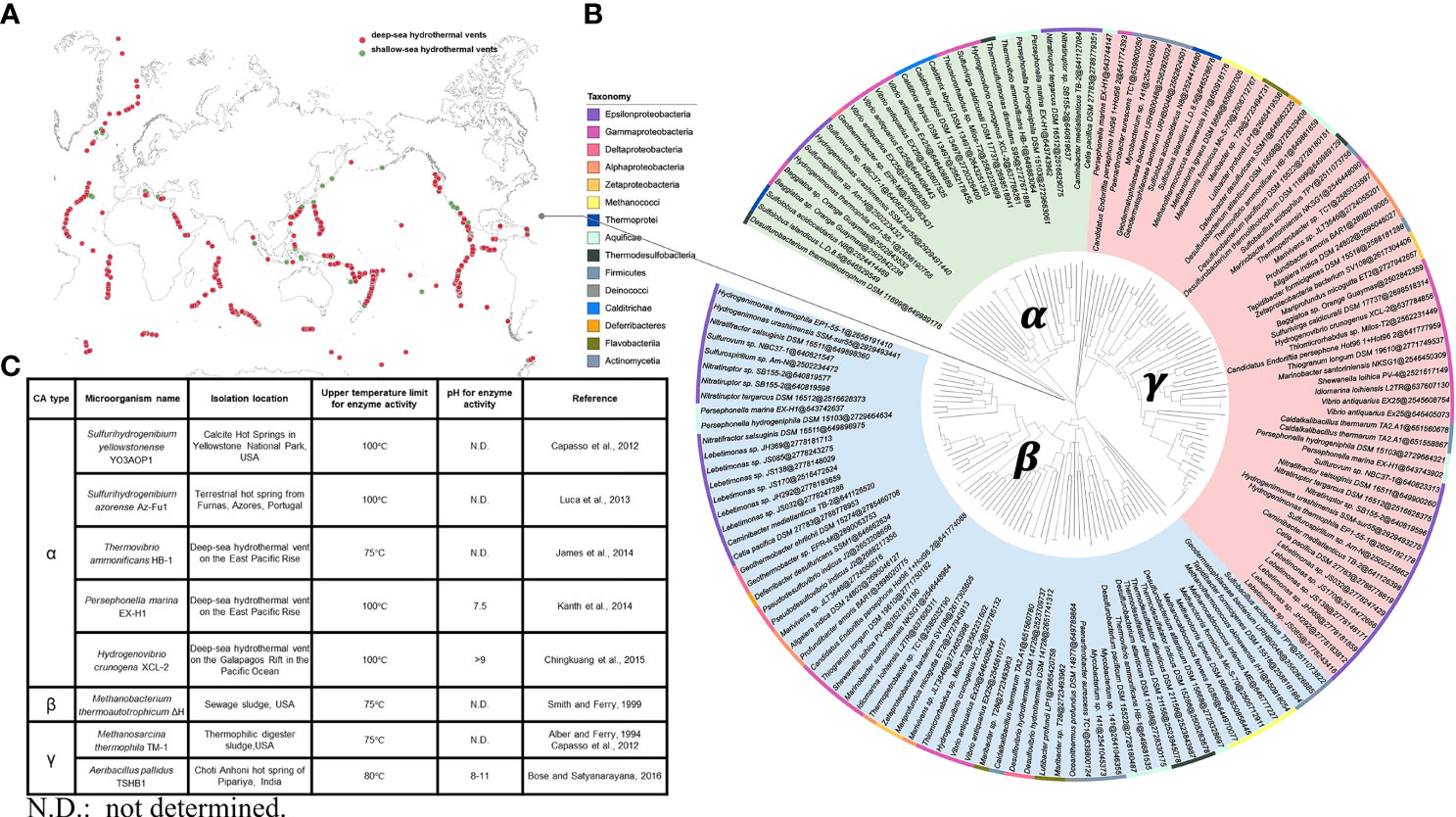
Figure 2 (A) Known hydrothermal environments, including shallow-sea hydrothermal vents and deep-sea hydrothermal vents. (B) A phylogenetic tree of different types of CAs-producing microbial strains from submarine hydrothermal systems. The three CAs classes, namely, α-, β-, and γ-CAs and the sequence accession number (https://img.jgi.doe.gov) after @ are shown. The rectangular bars representing the phyla of known strains are labeled with different background colors. (C) Classification, sources, and features of CAs from microorganisms in submarine hydrothermal environment.
Discussion
When compared with the cost of CO2 capture by aqueous mineral carbonation using wollastonite as feedstock ($ 122/ton CO2), application of CA to capture CO2 has been estimated to save about 50% of the cost (Supplementary Table S1). Here, we consider that the amount of CO2 that needs to be captured is 480 kt/year (8000 h), and that the immobilized CAs can increase the CO2 capture rate by about 25 times. The use of CAs to capture CO2 has the potential to overcome barriers in terms of plant quantity requirements and carbon conversion efficiency, save the investment of fixed assets (new plant space, equipment, and operating costs), and generate valuable chemicals. With the deepening of research on submarine hydrothermal microorganisms, CO2 capture using CAs is expected to reduce the cost of investment to cope with global environment and climate change.
Although the number of CAs-producing microorganisms that could be purely cultured has considerably increased over the past 80 years, only a small fraction of CAs-producing microorganisms isolated from submarine hydrothermal environments have been described in detail. Most of the microorganisms in submarine hydrothermal environments have been identified using only 16S rRNA sequencing, and are still difficult to be cultured and physiologically and metabolically characterized. For the application of CAs on an industrial scale, first, it is important to obtain more CAs from microorganisms in submarine hydrothermal environments. Many culturable microorganisms isolated from marine hydrothermal environments can fix carbon; for instance, chemoautotrophic microorganisms are very active in submarine hydrothermal environments and their CAs can help them in carbon fixation (Nakagawa and Takai, 2008). Therefore, these microorganisms can be cultured to obtain thermostable and alkali-tolerant CAs. To investigate the unculturable microorganisms in marine hydrothermal environments, metagenomics can be employed (Roumpeka et al., 2017), and techniques such as gene assembly, gene clustering, and metagenomic binning of species genomes have significantly enhanced our understanding of the taxonomic composition of microbial communities in submarine hydrothermal environments (Frioux et al., 2020). The study of high-quality metagenome-assembled genomes of bacteria and archaea can facilitate robust comparative genomic analyses of bacterial and archaeal diversity, allowing splicing of small fragmented genes into longer and complete CAs gene sequences as well as facilitating de novo assembly of CAs-producing microorganisms that cannot be found in databases.
Second, it has become an indispensable new trend to obtain thermostable and alkali-tolerant CAs based on engineering of microbial CAs, including genetic engineering, protein engineering (e.g., directed evolution, site-directed mutagenesis, etc.), and enzyme immobilization. By using techniques such as gene cloning and expression, recombinant CAs of several thermophilic bacteria have been expressed in Escherichia coli, which can maintain thermo-alkali stability and accelerate carbonate formation after expression (e.g., E. coli expressing Neisseria gonorrhoeae CA) (Jo et al., 2013). Directed evolution allows enzyme variants to acquire catalytic capacity by changing the catalytic site of CAs, and its combination with statistical analysis strategies for protein sequence activity relationships can generate new CAs (e.g., directed evolution can significantly enhance the properties of highly stable β-CAs derived from D. vulgaris, which can maintain activity at 107°C) (Alvizo et al., 2014). The nature of CAs and their catalytic properties can be modified via site-directed mutagenesis to make them more compatible with industrial requirements. The stability of recombinant CAs can be enhanced by using various immobilization techniques, and immobilization of CAs on specific carriers allows recycling of the enzyme. Various CAs immobilization methods, including immobilization onto polyurethane foam (Migliardini et al., 2013), magnetic particles (Ren et al., 2020), iron magnetic nanoparticles (Faridi et al., 2017), chitosan beads (Wanjari et al., 2011), silica beads (Raju et al., 2012), and other carriers (adsorption, cross-linked, covalent bonding, encapsulation or entrapment) have been investigated (Molina-Fernández and Luis, 2021), and some of these techniques have been shown to improve CAs activity and stability (e.g., CA from S. Yellowstonense YO3AOP1 immobilized on polyurethane foam maintains good stability) (Migliardini et al., 2013). Nevertheless, an unresolved problem that still exists is how to efficiently and reliably overexpress thermophilic enzyme genes in heterologous hosts. It is possible that the expressed proteins may not be able to withstand harsh environments (temperature, pressure, and pH) or may simply be prone to become insoluble, limiting the application of CAs. Besides, the recovery and reuse of free enzymes is expensive, and it has been predicted that immobilized CAs will be employed for industrial applications in the future. Therefore, there is a need to develop customized carrier materials that can withstand high temperatures and are rigid, as well as balance the degree of enzyme–carrier interaction so that CAs can be fully utilized for CO2 capture. The use of site-directed mutagenesis can specifically improve the complementarity of CAs and carrier surface, promote site-directed immobilization and rigidization of industrial CAs, and produce CAs that can meet specific needs. Moreover, immobilization of enzymes on nanoparticles and graphene materials, which possess unique size and physical properties, is a worthy future research direction. These nanomaterials can not only withstand high-temperature conditions, but can also maintain the folded structure of protein as much as possible and improve storage stability and performance.
Finally, application of thermostable and alkali-tolerant CAs to hydrothermal alteration rocks for CO2 sequestration is a more interesting research direction. Cations are essential to sequester CO2 as a solid carbonate, and magnesia olivine, wollastonite, serpentine, and basalt can provide cations (calcium, magnesium, iron, etc.) that can readily react with CO2 to produce solid inorganic carbonate minerals. Magnesia olivine and basalt can be altered and exhibit a fast dissolution rate relative to other silicates (Wang et al., 2019), and addition of carbon sources (e.g., CO2, HCO3−) has been reported to significantly enhance the alteration of magnesia olivine (Gadikota et al., 2014). Therefore, we conjecture that CAs from microorganisms thriving in hydrothermal environments can be introduced into the alteration process of ores. On the one hand, CAs can rapidly convert CO2 to HCO3− and enhance olivine alteration, so that ferrous ion could be released from olivine to participate in H2 production. On the other hand, the unconverted CO2 can lead to the formation of carbonates with Fe-bearing magnesite, which can be used in construction materials, chemical materials, and refining of magnesium metal and magnesium compounds.
Author Contributions
KT conceived the main frame of the manuscript and designed the figures. XM and LL wrote the manuscript. All authors contributed to the article and approved the submitted version.
Funding
This work was supported by the National Key Research and Development Program of China (2020YFA0608300), the Basic Science Center Program of Natural Science Foundation of China on Marine Carbon Sequestration and Biogeochemistry (42188102), and the National Natural Science Foundation of China (42141003).
Conflict of Interest
The authors declare that the research was conducted in the absence of any commercial or financial relationships that could be construed as a potential conflict of interest.
Publisher’s Note
All claims expressed in this article are solely those of the authors and do not necessarily represent those of their affiliated organizations, or those of the publisher, the editors and the reviewers. Any product that may be evaluated in this article, or claim that may be made by its manufacturer, is not guaranteed or endorsed by the publisher.
Supplementary Material
The Supplementary Material for this article can be found online at: https://www.frontiersin.org/articles/10.3389/fmars.2022.908818/full#supplementary-material
References
Alber B. E., Ferry J. G. (1994). A Carbonic Anhydrase From the Archaeon Methanosarcina Thermophila. Proc. Natl. Acad. Sci. U.S.A. 91, 6909–6913. doi: 10.1073/pnas.91.15.6909
Alvizo O., Nguyen L. J., Savile C. K., Bresson J. A., Lakhapatri S. L., Solis E., et al. (2014). Directed Evolution of an Ultrastable Carbonic Anhydrase for Highly Efficient Carbon Capture From Flue Gas. Proc. Natl. Acad. Sci. U.S.A. 111, 16436–16441. doi: 10.1073/pnas.1411461111
Bhagat C., Dudhagara P., Tank S. (2018). Trends, Application and Future Prospectives of Microbial Carbonic Anhydrase Mediated Carbonation Process for CCUS. J. Appl. Microbiol. 124, 316–335. doi: 10.1111/jam.13589
Bhattacharya S., Schiavone M., Chakrabarti S., Bhattacharya S. K. (2003). CO2 Hydration by Immobilized Carbonic Anhydrase. Biotechnol. Appl. Biochem. 38, 111–117. doi: 10.1042/BA20030060
Bose H., Satyanarayana T. (2016). Suitability of the Alkalistable Carbonic Anhydrase From a Polyextremophilic Bacterium Aeribacillus Pallidus TSHB1 in Biomimetic Carbon Sequestration. Bioprocess Biosyst. Eng. 39, 1–11. doi: 10.1007/s00449-016-1627-4
Capasso C., De Luca V., Carginalea V., Caramuscioc P., Cavalheiroc C. F., Canniod R. (2012). Characterization and Properties of a New Thermoactive and Thermostable Carbonic Anhydrase. Chem. Eng. 27, 271–276. doi: 10.3303/CET1227046
Capasso C., Supuran C. T. (2015). An Overview of the Alpha-, Beta- and Gamma-Carbonic Anhydrases From Bacteria: Can Bacterial Carbonic Anhydrases Shed New Light on Evolution of Bacteria? J. Enzyme Inhib. Med. Chem. 30, 325–332. doi: 10.3390/metabo7040056
Chen L. C., Peng P. Y., Lin L. F., Yang T., Huang C. M. (2014). Facile Preparation of Nitrogen-Doped Activated Carbon for Carbon Dioxide Adsorption. Aerosol. Air. Qual. Res. 14, 916–927. doi: 10.4209/aaqr.2013.03.0089
Chingkuang S., David N., Melissa A., Scott K. M. (2015). Activity and Anion Inhibition Studies of the Alpha-Carbonic Anhydrase From Thiomicrospira Crunogena XCL-2 Gammaproteobacterium. Bioorg. Med. Chem. Lett. 25, 4937–4940. doi: 10.1016/j.bmcl.2015.05.001
Claudiu S., Clemente C. (2017). An Overview of the Bacterial Carbonic Anhydrases. Metabolites 7, 56.
Dick G. J. (2019). The Microbiomes of Deep-Sea Hydrothermal Vents: Distributed Globally, Shaped Locally. Nat. Rev. Microbiol. 17, 271–283. doi: 10.1038/s41579-019-0160-2
Faridi S., Bose H., Satyanarayana T. (2017). Utility of Immobilized Recombinant Carbonic Anhydrase of Bacillus Halodurans TSLV1 on the Surface of Modified Iron Magnetic Nanoparticles in Carbon Sequestration. Energy Fuels. 31, 3002–3009. doi: 10.1021/acs.energyfuels.6b02777
Floryszak-Wieczorek J., Arasimowicz-Jelonek M. (2017). The Multifunctional Face of Plant Carbonic Anhydrase. Plant Physiol. Biochem. 112, 362. doi: 10.1016/j.plaphy.2017.01.007
Frioux C., Singh D., Korcsmaros T., Hildebrand F. (2020). From Bag-of-Genes to Bag-of-Genomes: Metabolic Modelling of Communities in the Era of Metagenome-Assembled Genomes. Comput. Struct. Biotechnol. J. 18, 1722–1734. doi: 10.1016/j.csbj.2020.06.028
Gadikota G., Matter J., Kelemen P., Park A. H. A. (2014). Chemical and Morphological Changes During Olivine Carbonation for CO2 Storage in thePresence of NaCl and Nahco3. Phys. Chem. Chem. Phys. Pccp. 16, 4679–4693. doi: 10.1039/c3cp54903h
Hasan M. M. F., First E. L., Boukouvala F., Floudas C. A. (2015). A Multi-Scale Framework for CO2 Capture, Utilization, and Sequestration: CCUS and CCU. Comput. Chem. Eng. 81, 2–21. doi: 10.1016/j.compchemeng.2015.04.034
Hewett-Emmett D., Tashian R. E. (1996). Functional Diversity, Conservation, and Convergence in the Evolution of the α-, β-, and γ-Carbonic Anhydrase Gene Families. Mol. Phylogenet. Evol. 5, 50–77. doi: 10.1006/mpev.1996.0006
Himadri B., Tulasi S. (2017). Microbial Carbonic Anhydrases in Biomimetic Carbon Sequestration for Mitigating Global Warming: Prospects and Perspectives. Front. Microbiol. 8, 1615. doi: 10.3389/fmicb.2017.01615
James P., Isupov M. N., Sayer C., Saneei V., Berg S., Lioliou M., et al. (2014). The Structure of a Tetrameric α-Carbonic Anhydrase From Thermovibrio Ammonificans Reveals a Core Formed Around Intermolecular Disulfides That Contribute to its Thermostability. Acta Crystallogr. D Biol. Crystallogr. 70, 2607–2618. doi: 10.1107/S1399004714016526
Joel K.J.Y., Stevens G. W., Caruso F., Kentish S. E. (2015). The Use of Carbonic Anhydrase to Accelerate Carbon Dioxide Capture Processes. J. Chem. Technol. Biotechnol. 90, 3–10. doi: 10.1002/jctb.4502
Jo B. H., Kim I. G., Seo J. H., Kang D. G., Cha H. J. (2013). Engineered Escherichia Coli With Periplasmic Carbonic Anhydrase as a Biocatalyst for CO2 Sequestration. Appl. Environ. Microbiol. 79, 6697–6705. doi: 10.1128/AEM.02400-13
Jonsson B.-H., Liljas A. (2020). Perspectives on the Classical Enzyme Carbonic Anhydrase and the Search for Inhibitors. Biophys. J. 119, 1275–1280. doi: 10.1016/j.bpj.2020.08.020
Kaar J. L., Oh H. I., Russell A. J., Federspiel W. J. (2007). Towards Improved Artificial Lungs Through Biocatalysis. Biomaterials 28, 3131–3139. doi: 10.1016/j.biomaterials.2007.03.021
Kanth B. K., Jun S.-Y., Kumari S., Pack S. P. (2014). Highly Thermostable Carbonic Anhydrase From Persephonella Marina EX-H1: Its Expression and Characterization for CO2-Sequestration Applications. Process Biochem. 49, 2114–2121. doi: 10.1016/j.procbio.2014.10.011
Klinthong W., Yang Y. H., Huang C. H., Tan C. S. (2015). A Review: Microalgae and Their Applications in CO2 Capture and Renewable Energy. Aerosol. Air. Qual. Res. 15, 712–742. doi: 10.4209/aaqr.2014.11.0299
Koschinsky A., Garbe-Sch D., Garbe-Schönberg D., Sander S., Schmidt K., Gennerich H. H., et al. (2008). Hydrothermal Venting at Pressure-Temperature Conditions Above the Critical Point of Seawater, 5°S on the Mid-Atlantic Ridge. Geology 36, 615–618. doi: 10.1130/G24726A.1
Kumar S., Rulhania S., Jaswal S., Monga V. (2021). Recent Advances in the Medicinal Chemistry of Carbonic Anhydrase Inhibitors. Eur. J. Med. Chem. 209, 112923. doi: 10.1016/j.ejmech.2020.112923
Liu W., Teng L., Rohani S., Qin Z., Liang B. (2021). CO2 Mineral Carbonation Using Industrial Solid Wastes: A Review of Recent Developments. Chem. Eng. J. 416, 129093. doi: 10.1016/j.cej.2021.129093
Luca V. D., Vullo D., Scozzafava A., Carginale V., Rossi M., Supuran C. T., et al. (2013). An α-Carbonic Anhydrase From the Thermophilic Bacterium Sulphurihydrogenibium Azorense is the Fastest Enzyme Known for the CO2 Hydration Reaction. Biorg. Med. Chem. 21, 1465–1469. doi: 10.1016/j.bmc.2012.09.047
Migliardini F., De Luca V., Carginale V., Rossi M., Corbo P., Supuran C. T., et al. (2013). Biomimetic CO2 Capture Using a Highly Thermostable Bacterial α-Carbonic Anhydrase Immobilized on a Polyurethane Foam. J. Enzyme Inhib. Med. Chem. 29, 146–150. doi: 10.3109/14756366.2012.761608
Min K. H., Son R. G., Ki M. R., Choi Y. S., Pack S. P. (2016). High Expression and Biosilica Encapsulation of Alkaline-Active Carbonic Anhydrase for CO2 Sequestration System Development. Chemosphere 143, 128–134. doi: 10.1016/j.chemosphere.2015.07.020
Molina-Fernández C., Luis P. (2021). Immobilization of Carbonic Anhydrase for CO2 Capture and its Industrial Implementation: A Review. J. CO2 Util. 47, 101475. doi: 10.1016/j.jcou.2021.101475
Nakagawa S., Takai K. (2008). Deep-Sea Vent Chemoautotrophs: Diversity, Biochemistry and Ecological Significance. FEMS Microbiol. Ecol. 65, 1–14. doi: 10.1111/j.1574-6941.2008.00502.x
Nguyen T., Hilliard M., Rochelle G. T. (2010). Amine Volatility in CO2 Capture. Int. J. Greenhouse Gas. Control. 4, 707–715. doi: 10.1016/j.ijggc.2010.06.003
Nlü A., Duman-Zdamar Z. E., Alolu B., Binay B. (2021). Enzymes for Efficient CO2 Conversion. Protein J. 40, 489–503. doi: 10.1007/s10930-021-10007-8
Olivares-Marin M., Maroto-Valer M. (2012). Development of Adsorbents for CO2 Capture From Waste Materials: A Review. Greenh. Gases 2, 20–35. doi: 10.1002/ghg.45
Osman A. I., Hefny M., Abdel Maksoud M. I. A., Elgarahy A. M., Rooney D. W. (2020). Recent Advances in Carbon Capture Storage and Utilisation Technologies: A Review. Environ. Chem. Lett. 19, 797–849. doi: 10.1007/s10311-020-01133-3
Raju R.Y., Mudliar S. N., Shekh A. Y. (2012). Immobilization of Carbonic Anhydrase in Alginate and its Influence on Transformation of CO2 to Calcite. Process Biochem. 47, 585–590. doi: 10.1016/j.procbio.2011.12.017
Ren S., Jiang S., Yan X., Chen R., Cui H. (2020). Challenges and Opportunities: Porous Supports in Carbonic Anhydrase Immobilization. J. CO2 Util. 42, 101305. doi: 10.1016/j.jcou.2020.101305
Rossi M. (2013). A New Heat-Stable Carbonic Anhydrase and Uses Thereof. Patent WO013064195A1, Switzerland: World Intellectual Property Organization, International Bureau.
Roumpeka D. D., Wallace R. J., Escalettes F., Fotheringham I., Watson M. (2017). A Review of Bioinformatics Tools for Bio-Prospecting From Metagenomic Sequence Data. Front. Genet. 8, 23. doi: 10.3389/fgene.2017.00023
Schweitzer H., Aalto N. J., Busch W., Chan D., Bernstein H. C. (2021). Innovating Carbon-Capture Biotechnologies Through Ecosystem-Inspired Solutions. One Earth 4, 49–59. doi: 10.1016/j.oneear.2020.12.006
Smith K. S., Ferry J. G. (1999). A Plant-Type (Beta-Class) Carbonic Anhydrase in the Thermophilic Methanoarchaeon Methanobacterium Thermoautotrophicum. J. Bacteriol. 181, 6247–6253. doi: 10.1128/JB.181.20.6247-6253.1999
Smith K. S., Ferry J. G. (2000). Prokaryotic Carbonic Anhydrases. FEMS Microbiol. Rev. 24, 335–366. doi: 10.1111/j.1574-6976.2000.tb00546.x
Supuran C. T. (2011). Carbonic Anhydrase Inhibitors and Activators for Novel Therapeutic Applications. Future Med. Chem. 3, 1165–1180. doi: 10.4155/fmc.11.69
Supuran C. T., Capasso C. (2016). New Light on Bacterial Carbonic Anhydrases Phylogeny Based on the Analysis of Signal Peptide Sequences. J. Enzyme Inhib. Med. Chem. 31, 1254–1260. doi: 10.1080/14756366.2016.1201479
Thompson R. B., Whetsell W. O. Jr., Maliwal B. P., Fierke C. A., Frederickson C. J. (2000). Fluorescence Microscopy of Stimulated Zn (II) Release From Organotypic Cultures of Mammalian Hippocampus Using a Carbonic Anhydrase-Based Biosensor System. J. Neurosci. Methods 96, 35–45. doi: 10.1016/S0165-0270(99)00183-1
Valluri S., Claremboux V., Kawatra S. (2022). Opportunities and Challenges in CO2 Utilization. J. Environ. Sci. 113, 322–344. doi: 10.1016/j.jes.2021.05.043
Wang J., Watanabe N., Okamoto A., Nakamura K., Komai T. (2019). Acceleration of Hydrogen Production During Water-Olivine-CO2 Reactions via High-Temperature-Facilitated Fe (II) Release. In. J. Hydrogen. Energ. 44, 11514–11524. doi: 10.1016/j.ijhydene.2019.03.119
Wanjari S., Prabhu C., Yadav R., Satyanarayana T., Labhsetwar N., Rayalu S. (2011). Immobilization of Carbonic Anhydrase on Chitosan Beads for Enhanced Carbonation Reaction. Process Biochem. 46, 1010–1018. doi: 10.1016/j.procbio.2011.01.023
Yu C. H., Huang C. H., Tan C. S. (2012). A Review of CO2 Capture by Absorption and Adsorption. Aerosol. Air. Qual. Res. 12, 745–769. doi: 10.4209/aaqr.2012.05.0132
Keywords: carbonic anhydrases, marine, hydrothermal system, carbon capture, carbon dioxide
Citation: Ma X, Liu L and Tang K (2022) Carbon Dioxide Sequestration by Microbial Carbonic Anhydrases From Submarine Hydrothermal Systems. Front. Mar. Sci. 9:908818. doi: 10.3389/fmars.2022.908818
Received: 31 March 2022; Accepted: 14 June 2022;
Published: 15 July 2022.
Edited by:
Lu Lin, Shandong University, ChinaReviewed by:
Abhay B. Fulke, National Institute of Oceanography (CSIR), IndiaYongyu Zhang, Qingdao Institute of Bioenergy and Bioprocess Technology (CAS), China
Copyright © 2022 Ma, Liu and Tang. This is an open-access article distributed under the terms of the Creative Commons Attribution License (CC BY). The use, distribution or reproduction in other forums is permitted, provided the original author(s) and the copyright owner(s) are credited and that the original publication in this journal is cited, in accordance with accepted academic practice. No use, distribution or reproduction is permitted which does not comply with these terms.
*Correspondence: Kai Tang, dGFuZ2thaUB4bXUuZWR1LmNu