- 1National Oceanography Centre, Southampton, United Kingdom
- 2James Hutton Institute, Aberdeen, United Kingdom
- 3British Antarctic Survey, Cambridge, United Kingdom
Lipid-storing copepods are fundamental to the functioning of marine ecosystems, transferring energy from primary producers to higher trophic levels and sequestering atmospheric carbon (C) in the deep ocean. Quantifying trophic transfer and biogeochemical cycling by copepods requires improved understanding of copepod metabolic rates in both surface waters and during lipid-fueled metabolism over winter. Here we present new biomass turnover rates of C and nitrogen (N) in Calanoides acutus, Calanoides natalis, Calanus glacialis and Calanus hyperboreus alongside published data for Calanus finmarchicus and Calanus pacificus. Turnover rates in metabolically active animals, normalised to 10°C, ranged between 0.007 – 0.105 d-1 and 0.004 – 0.065 d-1 for C and N, respectively. Turnover rates of C were typically faster than those for N, supporting the understanding that non-protein C, e.g. lipid, is catabolised faster than protein. Re-analysis of published data indicates that inactive, overwintering C. finmarchicus turn over wax ester lipids at a rate of 0.0016 d-1. These and other basal rate data will facilitate the mechanistic representation of copepod physiology in global biogeochemical models, thereby reducing uncertainties in our predictions of future ocean ecosystem functioning and C sequestration.
Introduction
Marine copepods dominate the zooplankton communities of pelagic ecosystems and play fundamental ecological and biogeochemical roles therein. They convert protistan microplankton into energy-dense and nutritious biomass that is subsequently preyed upon by countless species of higher predators including fish, birds and mammals. Their metabolic- and grazing activities result in the remineralization of nutrients (e.g. nitrogen, N) in the surface ocean and the export of organic carbon (C) to depth via the production of large, dense, fast-sinking faecal pellets. The latter are thought to dominate the gravitational flux of C in the ocean’s ‘biological carbon pump’ (Nowicki et al., 2022), which helps regulate the concentration of atmospheric CO2 by sequestering C in the deep ocean. Copepods also actively transport C to deeper waters via diel- and ontogenetic migrations (Steinberg and Landry, 2017). The latter occurs as lipid-rich developmental stages descend into the deep ocean towards the end of the growth season to overwinter through a process now referred to as the ‘seasonal lipid pump’, and may sequester a similar amount of carbon as the sinking flux of detritus in the North Atlantic and Arctic Oceans (Jónasdóttir et al., 2015; Visser et al., 2017; Jónasdóttir et al., 2019).
The biogeochemical significance of copepods in marine ecosystems is often quantified using numerical models. The underlying assumptions and parameterisations that are used to represent copepod physiology in these models have profound consequences for our understanding of oceanic nutrient recycling and C sequestration (Anderson et al., 2013; Maps et al., 2014; Jónasdóttir et al., 2015). New models that explicitly represent copepod physiology, e.g. biomass turnover and the metabolic costs associated with acquiring resources and generating energy, have helped develop our understanding of how the quantity and quality of food influence copepod growth and the fate of C and N in ecosystems (e.g. Anderson et al., 2017; Anderson et al., 2020). The empirical data required to parameterise such models are, however, often lacking. Here we present new data on the rates at which food-deprived copepods catabolise C and N, alongside other literature-extracted data, to quantify the typical range of basal metabolic rates of these important marine animals.
Methods
Biomass turnover rates in active (non-diapausing) copepods were estimated by incubating groups of animals without food and determining the decline in biomass C and N concentrations through time by analysing sub-samples collected across the experimental durations (Table 1). Our experimental design is conceptually analogous to previous work (Mayor et al., 2011), differing only in the species/stages of copepod examined and the length of the incubations. Stage V and female stage VI copepodites (CV and CVI, respectively) collected from surface waters (200 – 0 m) across a range of contrasting locations (Table 1) were carefully identified and staged under a dissection microscope (Wild M5) with cool illumination. Experimental animals were picked into 0.2 µm filtered seawater (FSW) using swan-necked forceps and acclimated overnight to experimental conditions at the in-situ temperature (Table 1). Replicate groups of animals, except in the case for Calanus hyperboreus which were maintained as individuals owing to their large size, were then transferred into 500 mL Nalgene wide mouth bottles (n = 18) containing FSW and incubated at the required temperature. Copepods from triplicate experimental bottles were carefully removed at one of the six sampling points over the 10-35 day incubations. Sampled animals were transferred into tin cups and stored frozen (-80°C) for subsequent elemental analysis. Duplicate samples of ~500 eggs produced by Calanus glacialis after 3 days of incubation were carefully counted onto pre-combusted (450°C, overnight) 25 mm GFF filters and stored frozen (-80°C). All experimental work was conducted in temperature-controlled laboratories. Elemental analysis of freeze-dried samples was conducted using a Flash EA 1112 Series Elemental Analyser (Thermo Fisher Scientific, Germany).
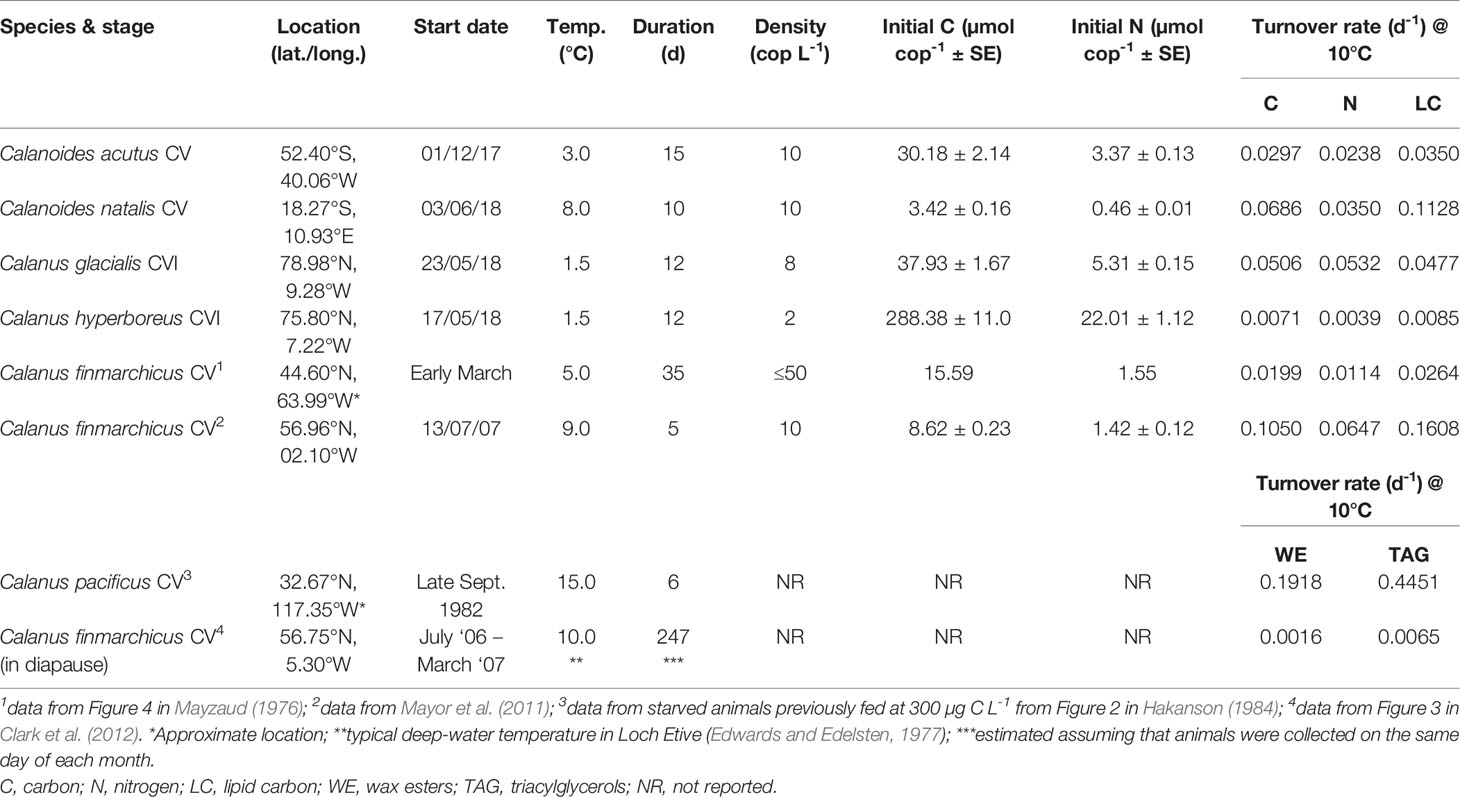
Table 1 Experimental details and biomass turnover rates in food-deprived marine calanoid copepods, normalised to 10°C using Q10 = 2.
Additional data from studies of similar experimental design, i.e. where the decline in copepod biomass over time was measured in food-deprived or non-feeding animals, were extracted from published works (Mayzaud, 1976; Hakanson, 1984; Clark et al., 2012) by measuring the distance between the x-axis and each datum using the Vertical Dimension tool in CorelDRAW X7 and scaling this to the vertical distance of a known amount on the y-axis. The data from Clark et al. (2012) relate to inactive Calanus finmarchicus sampled from a population in diapause, assumed to occur between July and the following March. We also present previously published data for active C. finmarchicus (Mayor et al., 2011) for comparison.
The non-protein-associated C, or total lipid carbon (LC), content at each experimental time point was estimated from biomass C and N concentrations by assuming protein has a molar C:N of 3.7 (Vollenweider, 1985): LC = C – [N × 3.7]. Instantaneous turnover rates were derived via linear regression analysis of log-transformed biomass data against time, i.e. assuming an exponential rate of change (Figure 1). Turnover rates of the LC pool, which provide an estimate of the rate at which the whole lipid pool turns over, are also presented because this is considered to represent ‘other basal metabolism’ (e.g. Anderson et al., 2021 and references therein). The turnover rates of wax esters (WE) and triacylglycerols (TAG), calculated from data extracted from published studies (Hakanson, 1984; Clark et al., 2012), refer to these individual lipid classes only, and are not synonymous with LC rates. Data analysis was conducted in the R programming language (version 3.6.1; R Core Team, 2019).
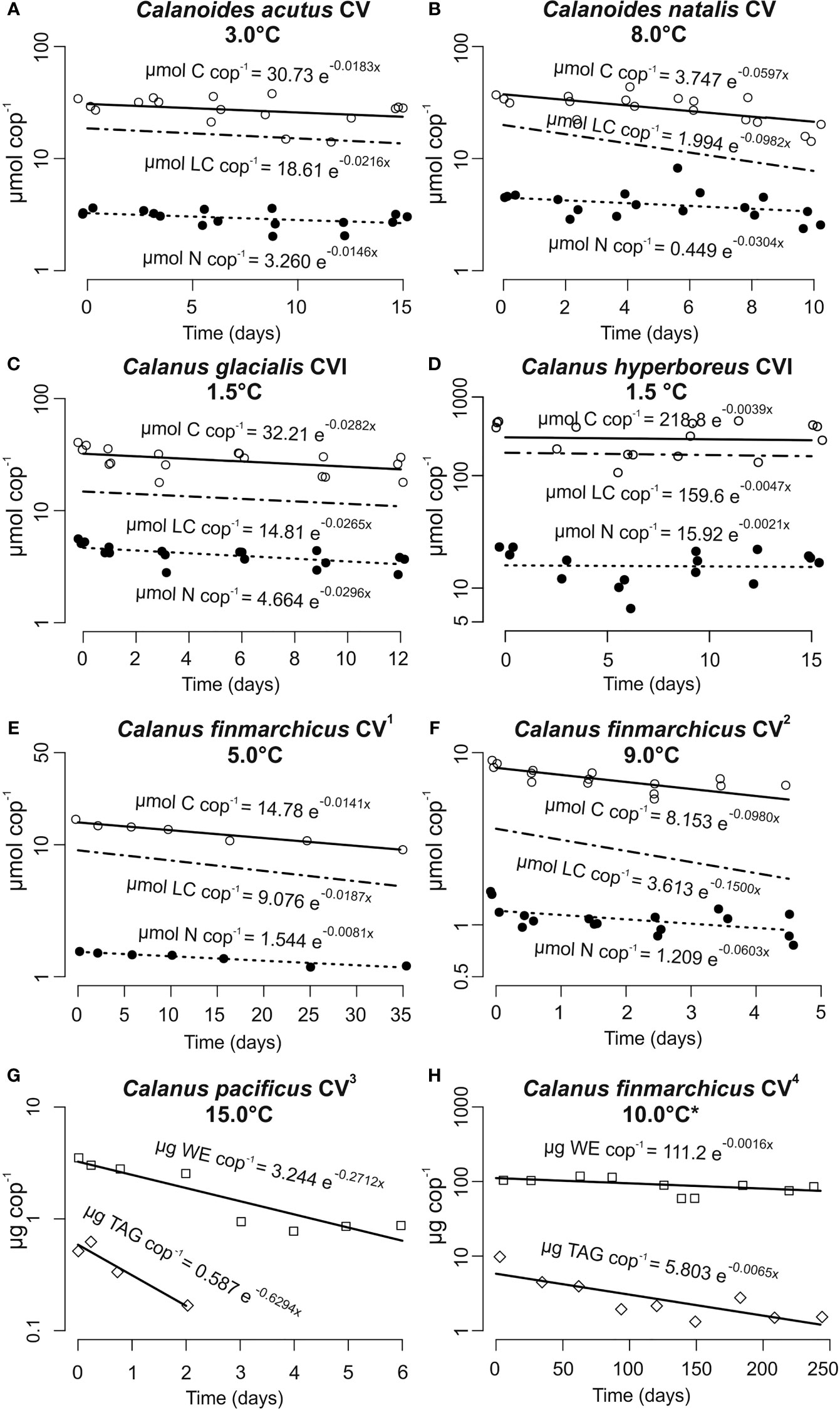
Figure 1 Biomass turnover rates of lipid-storing marine calanoid copepods during food deprivation. Note different scales on the x- and y-axes. Equation exponents provide the instantaneous turnover rate (d-1) for carbon (C) (open circles); nitrogen (N) (filled circles); lipid carbon (LC, no symbols as data were derived from C and N); wax esters (WE, open squares) and triacylglycerols (TAG, open diamonds). Data extracted from 1Mayzaud (1976); 2Mayor et al. (2011); 3Hakanson (1984); 4Clark et al. (2012). The total lipid pool in Clark et al. (2012) turned over at -0.0021 d-1 (data not shown); *typical deep-water temperature in Loch Etive (Edwards and Edelsten, 1977).
Results
Biomass turnover rates in Calanoides acutus CV, Calanoides natalis CV, Calanus glacialis CVI, Calanus hyperboreus CVI and Calanus finmarchicus CV at the experimental temperatures are presented in Figure 1. After adjusting rates to 10°C using a Q10 = 2, turnover rates for total C ranged between 0.007 - 0.105 d-1 (mean ± SD = 0.047 ± 0.016) and between 0.004 - 0.065 d-1 for total N (mean ± SD = 0.032 ± 0.011; Table 1). Estimated specific lipid carbon (LC) turnover rates normalised to 10°C ranged between 0.009 - 0.161 d-1 (mean ± SD = 0.065 ± 0.026; Table 1). Literature-extracted data on the turnover rates of WE and TAG in active Calanus pacificus CVs from off La Jolla, San Diego (0.1918 d-1 and 0.4451 d-1, respectively) were two orders of magnitude higher than the respective rates observed in diapausing (= inactive) C. finmarchicus CVs from Loch Etive, Scotland (0.0016 d-1 and 0.0065 d-1, respectively).
Discussion
The specific C and N turnover rates reported here are in good agreement with previously observed tissue turnover and excretion rates in calanoid copepods (Butler et al., 1969; Butler et al., 1970; Helland et al., 2003; Graeve et al., 2005). The switch between fed and starved metabolism in zooplankton takes place over several days (Mayzaud, 1976) and thus the proportional contribution of these two metabolic modes to the observed rates varied between experiments with different incubation periods. This variation potentially explains why the highest turnover rates were observed for Calanus finmarchicus CV over a 5-day incubation (Table 1). Nevertheless, the observed rates of biomass turnover were comparable across the different species, particularly when the large geographic distances between the study sites and differences in the developmental stages are taken into consideration.
Estimated specific rates of lipid carbon turnover (LC = ‘other basal metabolism’; Anderson et al., 2017) were typically greater than the observed rates of total C and N (Table 1). These observations support the view that lipids are preferentially used to meet metabolic demands (Mayzaud, 1976; Helland et al., 2003), sparing proteins for growth (Li et al., 2012). This trend was not observed in Calanus glacialis CVI, which produced eggs sporadically during the incubations (mean = ~19 eggs female-1 day-1); the elevated turnover rate of N relative to C in these females is consistent with the production of protein-rich eggs (26.7 nmol C egg-1, 5.6 nmol N egg-1, molar C:N = 4.7; n = 2). Exactly if/how Calanus spp. store protein for producing eggs when food is scarce or even absent remains unclear (Mayor et al., 2009) and reflects our limited understanding of protein physiology in copepods and how this varies throughout their ontogeny, particularly for egg production immediately after diapause when food concentrations are low. Understanding how copepods provision their eggs with protein will help us better assess how their populations will respond to future climate-driven changes in the elemental stoichiometry of marine primary producers, the C:N of which is expected to increase (e.g. Tanioka and Matsumoto, 2020; Velthuis et al., 2022).
The estimated specific turnover rates of LC in starved animals, with the exception of C. hyperboreus, were all at least one order of magnitude faster than the specific rate at which wax esters turned over in diapausing C. finmarchicus CV (Table 1). This is consistent with the understanding that animals in diapause are inactive and capable of down-regulating their metabolism to rates that are considerably lower than occur in active, non-diapausing animals (Ingvarsdóttir et al., 1999). This suggestion is further supported by comparing the turnover rates of WE and TAGs in diapausing C. finmarchicus (Clark et al., 2012) to those in active C. pacificus (Hakanson, 1984); the latter displayed rates that were two orders of magnitude higher (Table 1). Interestingly, TAGs in diapausing C. finmarchicus turned over approximately four times faster than wax esters (0.0065 d-1 and 0.0016 d-1, respectively; Figure 1H), suggesting that they may play an important, and previously overlooked, role in overwintering metabolism. One study (Yebra et al., 2006) suggests that protein turnover rates in diapausing C. finmarchicus CVs may be similar those of metabolically active animals (Table 1), implying that these animals release both dissolved C and N at overwintering depths, thereby weakening the extent to which the lipid pump decouples C and N (Jónasdóttir et al., 2015). We urgently need more information on protein metabolism during diapause such that we can understand how best to represent the lipid pump in biogeochemical models.
The presented data suggest that the physiologies of active, lipid-storing copepods are broadly similar across species, thereby simplifying the parameterisation of their metabolic rates in global biogeochemical models. If or how these rates vary relative to those in non-lipid storing animals remains unknown and further work on species from lower latitudes is required to resolve this important question. Including ontogenetic variation of basal rates in ecosystem models, e.g. during diapause and gonad maturation, will help improve their ability to resolve and quantify the biogeochemical implications of seasonal phenomena, e.g. the lipid pump. Improving knowledge of the sources and rates at which protein (N) turns over is essential for developing understanding of how marine copepods interact with the cycling of nutrients in the ocean. Mechanistically representing the effects of copepod physiology on ocean biogeochemistry in marine ecosystem models will improve our confidence in their ability to forecast how the direct and indirect effects of climate change will affect globally important processes, such as the ocean’s biological carbon pump.
Data Availability Statement
The raw data supporting the conclusions of this article will be made available by the authors, without undue reservation.
Ethics Statement
Ethical review and approval was not required for the animal study because this study presents data on invertebrates only.
Author Contributions
DM, KC, and TA conceived and designed the study. DM and KC conducted the experiments. BT conducted the elemental analyses. DM analysed the data and drafted the manuscript. All authors contributed to manuscript revision, read, and approved the submitted version.
Funding
This work was funded by the Natural Environment Research Council (UK) (COMICS: NE/M020835/1; DIAPOD: NE/P006353/1).
Conflict of Interest
The authors declare that the research was conducted in the absence of any commercial or financial relationships that could be construed as a potential conflict of interest.
Publisher’s Note
All claims expressed in this article are solely those of the authors and do not necessarily represent those of their affiliated organizations, or those of the publisher, the editors and the reviewers. Any product that may be evaluated in this article, or claim that may be made by its manufacturer, is not guaranteed or endorsed by the publisher.
Acknowledgments
We gratefully acknowledge assistance from the Masters and crews of the RRS Discovery (DY086 and DY090) and the RRS James Clark Ross (JR17005 and JR18007). This manuscript was improved following comments from two anonymous reviewers.
References
Anderson T. R., Hessen D. O., Boersma M., Urabe J., Mayor D. J. (2017). Will Invertebrates Require Increasingly Carbon-Rich Food in a Warming World? Am. Nat. 190, 725–742.doi: 10.1086/694122
Anderson T. R., Hessen D. O., Mayor D. J. (2021). Is the Growth of Marine Copepods Limited by Food Quantity or Quality? Limnol. Oceanogr. Lett. 6, 127–133. doi: 10.1002/lol2.10184
Anderson T. R., Hessen D. O., Mitra A., Mayor D. J., Yool A. (2013). Sensitivity of Secondary Production and Export Flux to Choice of Trophic Transfer Formulation in Marine Ecosystem Models. J. Mar. Sys. 125, 41–53. doi: 10.1016/j.jmarsys.2012.09.008
Anderson T. R., Raubenheimer D., Hessen D. O., Jensen K., Gentleman W. C., Mayor D. J. (2020). Geometric Stoichiometry: Unifying Concepts of Animal Nutrition to Understand How Protein-Rich Diets can be “Too Much of a Good Thing”. Front. Ecol. Evol. 8, 196. doi: 10.3389/fevo.2020.00196
Butler E. I., Corner E. D. S., Marshall S. M. (1969). On the Nutrition and Metabolism of Zooplankton VI. Feeding Efficiency of Calanus in Terms of Nitrogen and Phosphorous. J. Mar. Biol. Ass. U. K. 49, 977–1001. doi: 10.1017/S0025315400038054
Butler E. I., Corner E. D. S., Marshall S. M. (1970). On the Nutrition and Metabolism of Zooplankton VII. Seasonal Survey of Nitrogen and Phosphorous Excretion by Calanus in the Clyde Sea Area. J. Mar. Biol. Ass. U.K. 50, 525–560. doi: 10.1017/S0025315400004707
Clark K. A., Brierley A. S., Pond D. W. (2012). Composition of Wax Esters Linked to Diapause Behaviour of Calanus finmarchicus in a Sea Loch Environment. Limnol. Oceanogr. 57, 65–75. doi: 10.4319/lo.2012.57.1.0065
Edwards A., Edelsten D. J. (1977). Deep Water Renewal of Loch Etive: A Three Basin Scottish Fjord. Estuar. Coast. Mar. Sci. 5, 575–595. doi: 10.1016/0302-3524(77)90085-8
Graeve M., Albers C., Kattner G. (2005). Assimilation and Biosynthesis of Lipids in Arctic Calanus Species Based on Feeding Experiments With a 13C Labelled Diatom. J. Exp. Mar. Biol. Ecol. 317, 109–125. doi: 10.1016/j.jembe.2004.11.016
Hakanson J. L. (1984). The Long and Short Term Feeding Conditions in Field-Caught Calanus pacificus, as Determined From the Lipid Content. Limnol. Oceanogr. 29, 794–804. doi: 10.4319/lo.1984.29.4.0794
Helland S., Nejstgaard J. C., Fyhn H. J., Egge J. K., Båmstedt U. (2003). Effects of Starvation, Season, and Diet on the Free Amino Acid and Protein Content of Calanus finmarchicus Females. Mar. Biol. 143, 297–306. doi: 10.1007/s00227-003-1092-x
Ingvarsdóttir A., Houlihan D. F., Heath M. R., Hay S. J. (1999). Seasonal Changes in Respiration Rates of Copepodite Stage V Calanus Finmarchicus (Gunnerus). Fish. Oceanogr. 8 (Suppl. I), 73–83. doi: 10.1046/j.1365-2419.1999.00002.x
Jónasdóttir S. H., Visser A. W., Richardson K., Heath M. R. (2015). Seasonal Copepod Lipid Pump Promotes Carbon Sequestration in the Deep North Atlantic. Proc. Nat. Acad. Sci. 112, 12122–12126. doi: 10.1073/pnas.1512110112
Jónasdóttir S. H., Wilson R. J., Gislason A., Heath M. R. (2019). Lipid Content in Overwintering Calanus finmarchicus Across the Subpolar Eastern North Atlantic Ocean. Limnol. Oceanogr. 64, 2029–2043. doi: 10.1002/lno.11167
Li X., Jiang Y., Liu W., Ge X. (2012). Protein-Sparing Effect of Dietary Lipid in Practical Diets for Blunt Snout Bream (Megalobrama amblycephala) Fingerlings: Effects on Digestive and Metabolic Responses. Fish Physiol. Biochem. 38, 529–541. doi: 10.1007/s10695-011-9533-9
Maps F., Record N. R., Pershing A. J. (2014). A Metabolic Approach to Dormancy in Pelagic Copepods Helps Explaining Inter-and Intra-Specific Variability in Life-History Strategies. J. Plankt. Res. 36, 18–30. doi: 10.1093/plankt/fbt100
Mayor D. J., Anderson T. R., Pond D. W., Irigoien X. (2009). Limitation of Egg Production in Calanus finmarchicus in the Field: A Stoichiometric Analysis. J. Mar. Sys. 78, 511–517. doi: 10.1016/j.jmarsys.2008.12.020
Mayor D. J., Cook K., Thornton B., Walsham P., Witte U. F. M., Zuur A. F., et al. (2011). Absorption Efficiencies and Basal Turnover of C, N and Fatty Acids in a Marine Calanoid Copepod. Funct. Ecol. 25, 509–518. doi: 10.1111/j.1365-2435.2010.01791.x
Mayzaud P. (1976). Respiration and Nitrogen Excretion of Zooplankton. IV. The Influence of Starvation on the Metabolism and the Biochemical Composition of Some Species. Mar. Biol. 37, 47–58. doi: 10.1007/BF00386778
Nowicki M., DeVries T., Siegel D. A. (2022). Quantifying the Carbon Export and Sequestration Pathways of the Ocean’s Biological Carbon Pump. Global Biogeochem. Cy. 36, e2021GB007083. doi: 10.1029/2021GB007083
R Core Team (2019). R: A Language and Environment for Statistical Computing (Vienna, Austria: R Foundation for Statistical Computing). Available at: https://www.R-project.org/.
Steinberg D. K., Landry M. R. (2017). Zooplankton and the Ocean Carbon Cycle. Annu. Rev. Mar. Sci. 9, 413–444. doi: 10.1146/annurev-marine-010814-015924
Tanioka T., Matsumoto K. (2020). A Meta-Analysis on Environmental Drivers of Marine Phytoplankton C: N: P. Biogeoscience 17, 2939–2954. doi: 10.5194/bg-17-2939-2020
Velthuis M., Keuskamp J. A., Bakker E. S., Boersma M., Sommer U., van Donk E., et al. (2022). Differential Effects of Elevated Pco2 and Warming on Marine Phytoplankton Stoichiometry. Limnol. Oceanogr. 67, 598–607. doi: 10.1002/lno.12020
Visser A. W., Grønning J., Jónasdóttir S. H. (2017). Calanus hyperboreus and the Lipid Pump. Limnol. Oceanogr. 62, 1155–1165. doi: 10.1002/lno.10492
Vollenweider R. A. (1985). Elemental and Biochemical Composition of Plankton Biomass; Some Comments and Explorations. Arch. Hydrobiol. 105, 11–29.
Keywords: lipid turnover, protein turnover, basal metabolism, diapause, ecosystem model, physiology, stoichiometry
Citation: Mayor DJ, Cook KB, Thornton B, Atherden F, Tarling GA and Anderson TR (2022) Biomass Turnover Rates in Metabolically Active and Inactive Marine Calanoid Copepods. Front. Mar. Sci. 9:907290. doi: 10.3389/fmars.2022.907290
Received: 29 March 2022; Accepted: 12 May 2022;
Published: 16 June 2022.
Edited by:
Albert Calbet, Institute of Marine Sciences, Spanish National Research Council (CSIC), SpainReviewed by:
Lidia Yebra, Spanish Institute of Oceanography (IEO), SpainKristina Øie Kvile, Norwegian Institute for Water Research (NIVA), Norway
Copyright © 2022 Mayor, Cook, Thornton, Atherden, Tarling and Anderson. This is an open-access article distributed under the terms of the Creative Commons Attribution License (CC BY). The use, distribution or reproduction in other forums is permitted, provided the original author(s) and the copyright owner(s) are credited and that the original publication in this journal is cited, in accordance with accepted academic practice. No use, distribution or reproduction is permitted which does not comply with these terms.
*Correspondence: Daniel J. Mayor, ZGFuLm1heW9yQG5vYy5hYy51aw==