- 1Sorbonne Université, CNRS, Laboratoire d’Ecogéochimie des Environnements Benthiques, LECOB, Banyuls-sur-Mer, France
- 2Sorbonne Université, CNRS, Biologie Intégrative des Organismes Marins, BIOM, Banyuls-sur-Mer, France
Biomineralisation process which is the induction of the precipitation of a mineral by an organism, generates hard tissues such as bones, teeth, otoliths and shells. Biomineralisation rate is not constant over time. This is likely due to variations of environmental and/or physiological conditions, leading to the formation of growth increments or rings. For bivalves, increments are considered as the unit of time recorded in shells. Therefore, shells are used as biological archives of (paleo)environmental and (paleo)climatic conditions. However, the environmental drivers leading to the periodic formation of increments are still poorly understood. Tackling the question of the integration of the environment by the organism is challenging: is there a direct effect of the environmental variability on bivalve shell biomineralisation? Or is biomineralisation controlled by a biological clock? In this review, the different temporal units observed in bivalve shells and the possible regulatory processes are explored and some research trajectories are suggested.
Introduction
One of the main keys of the last 500 Myrs is the flourishment of hard structures produced by organisms. This process called biomineralisation offers large possibilities for evolution of organisms by its use in the support of the general shape of organisms together with an efficient protection of soft tissues (Marin et al., 2007; Murdock, 2020). The production of hard structures by organisms (shell, skeleton) allows better conditions for the preservation of traces of life on the earth, conducing to define this period as the “Phanerozoic times”. Biomineralised structures are now considered as fundamental constituents of the environment, found from the micrometric scale in the skeleton of phytoplankton (the major primary producers) to kilometric scales, visible from the space, such as the Great Barrier Reef formed by corals. The ecological and economic values associated with biomineralisation include the sink of carbon, the shellfish production, the supply of building materials, the formation of habitats (reefs and mounds) by engineer species used as refuges for biodiversity, and their use as archive of (paleo)environmental and (paleo)climatic conditions (Moberg and Folke, 1999; Coen et al., 2007).
Various strategies for mineralisation can be experienced by organisms, through extrinsic biologically-influenced mineralisation (also called organomineralisation) to intrinsic biologically-induced or controlled mineralisation (biomineralisation s.s.) (Weiner and Dove, 2003; Dupraz et al., 2009). In the animal kingdom, biologically-controlled mineralisation is the rule. This process infers enzymatic regulation and active pumping of elements from the external medium, leading to a strong connection between biomineralisation and the metabolism of the organism. Consequently, biomineralisation is not homogeneous nor continuous with time. The growth of shells and skeletons can slow down or even stop, likely related to the dynamics of environmental and physiological conditions. This is notably the case in many mid-latitude species during winter, when the temperature is cold and the food is sparse (Schöne, 2008). Therefore, various growth patterns can be observed depending on the experienced environmental conditions and serve as the basis of sclerochronological analysis.
Sclerochronology is the study of physical and chemical variation in the accretionary tissues of calcified biomaterials, where the increment is the unit of time (Schöne et al., 2003b; Schöne, 2008; Huyghe et al., 2019). By introducing a temporal basis in the study of hard tissues of organisms, this newly research field offers a tremendous potential in various disciplinaries such as physiology, ecology, paleoclimatology. The study of growth patterns has long been used as a timescale in calcified tissues (Réaumur, 1709; Pulteney, 1781; von Hessling, 1859). Since growth rate of organisms is largely controlled by environmental drivers, changes in skeleton biomineralisation provide a reliable archive of the environmental variations experienced by organisms (Kennish and Olsson, 1975). This concept is successfully applied for a variety of biomaterials, including shell and skeleton produced by invertebrates such as bivalves and corals, but also in vertebrates (i.e., otoliths of teleost fish and teeth of mammals) (Skinner and Jahren, 2003; Murdock, 2020). The introduction of geochemical approaches allows for a precise quantification of environmental data, as several parameters (temperature, salinity, food, etc) are archived in the form of stable isotopes or minor and trace elements (Epstein et al., 1953; Dodd, 1965). Nowadays, sclerochronology (implying increment counting) and sclerochemistry (when coupled with geochemical proxies), feed various environmental monitoring programs on climate change, pollution events or health status surveys of calcifying species (Schöne, 2013; Schöne and Krause, 2016; Steinhardt et al., 2016). Thanks to technology improvements, we are now able to work from long-term time series (i.e., decennial to centennial) to high-temporal resolution archives (i.e., daily to hourly) (Huyghe et al., 2019; de Winter et al., 2020; Poitevin et al., 2020; Yan et al., 2020). Paradoxically, the inducer of increments formation is still uncertain, and particularly the discrimination between the role of the environment and/or the one of physiological induced changes on growth patterns. Endogenous time-keeping mechanisms, also called biological clocks, have been proposed to regulate periods of growth (Richardson et al., 1979) but the precise role of the environment as a time giver for biomineralisation activity remains to be defined.
The lack of knowledge concerning biomineralisation processes has an impact on the study of the shell growth in a temporal framework and their use as (paleo)environmental archives, which is regularly raised by sclerochronologists (Schöne, 2008; Trofimova et al., 2020). In fact, a gap persists between shell growth increment analysis and the study of biomineralisation processes, as they do not refer to similar timescales. This has led to the separation of scientific communities interested in mineralised tissues on one hand with scientists mostly focused on mechanisms inducing nucleation from calcium carbonate to the formation of the neocrystal, and on the other hand with researchers developing approaches to determine the time frame in which the shell forms in order to use these calcified pieces as biological archives. As new advances in the characterisation of molecular biological clocks and their effect on the physiological activity of bivalves have been made recently, we think that the gap is about to be bridged. In this review, we first report the current knowledge about shell biomineralisation and the formation of bivalve shell increments. Then we explore possible regulatory mechanisms that could involve the environment and/or biological clocks, including temporal and spacial scales poorly investigated yet. Further research directions are finally provided. Many reviews in the field of sclerochronology have been done recently, covering particularly the use of bivalves shells as (paleo)environmental archives (Steinhardt et al., 2016; Butler and Schöne, 2017; Trofimova et al., 2020; Peharda et al., 2021). This review addresses an additional aspect of the research by linking sclerochronology to chronobiology and enriches the multidisciplinary dimension of sclerochronology.
Shells Formation
Hard tissues formed by many organisms can have various functions, such as protection for shells, structuration for bones or equilibrium for otoliths. The process by which hard tissues are formed is the biomineralisation, which refers to the active process of precipitation of a mineral by an organism. Organisms produce various minerals, including silica, bioapatite, iron oxides and hydroxides, but the main component produced is calcium carbonate through three polymorphs: calcite, aragonite and, less frequently, vaterite (Skinner and Jahren, 2003). The mineral units are self-assembled within the inter- and intracrystalline organic framework. In bivalve shells, the organic matrix represents on average 0.01 to 5% of the weight, and is composed of a set of proteins, peptides, free amino acids, lipids, polysaccharides and pigments (Marin et al., 2012). The organic fraction is found under an insoluble hydrophobic phase, which is the main component of the framework for crystal growth, and a soluble acidic phase rich in aspartic acid (Asp), that makes the organic compounds bind calcium ions (Marin et al., 2007). As such, organic compounds control shell mineralisation, together with the crystal shape and growth (Marin and Luquet, 2004).
The shell of bivalve is composed of superimposed layers (Figure 1). The external layer, called periostracum, is rich in organic compounds. Most bivalves have two subperiostracal layers that can be made of either calcite only, or aragonite only or both. The area of muscle insertion is composed of aragonite. Calcite and aragonite crystals can follow different growth axes, leading to the formation of diverse morphologies and arrangements, called microstructures (Checa, 2018). A wide variety of microstructures can be found in bivalve shells (Figure 2). For example, Mytilidae have fibrous prismatic calcite in the outer part of the ostracum and aragonite nacreous in the inner part.
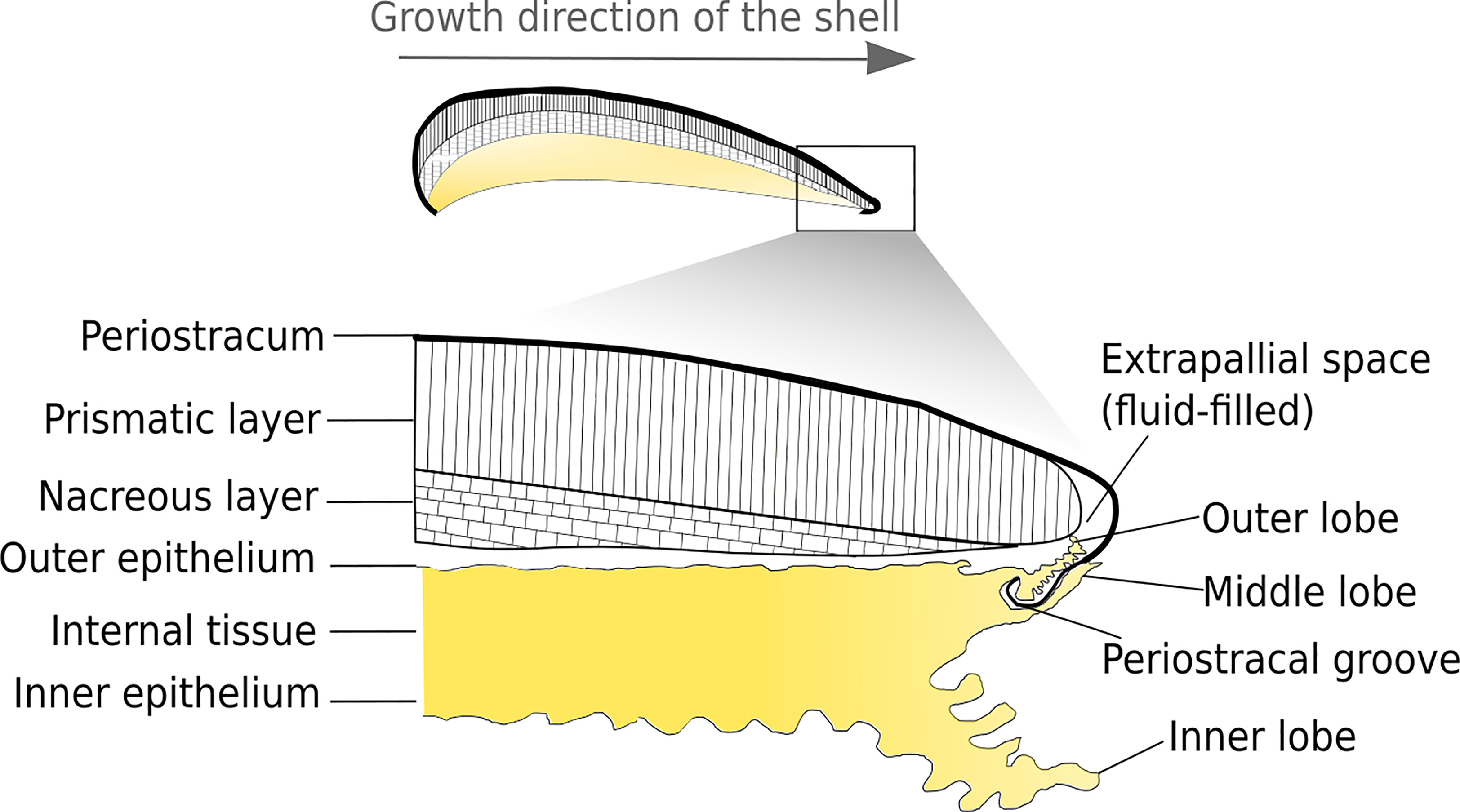
Figure 1 Longitudinal view of M. galloprovincialis showing the structural organisation between shell and the underlying mantle. The shell is composed of three layers: the nacreous layer (bottom), the prismatic layer (middle) and the periostracum (upper part). The periostracum seals with the mantle the extrapallial space where mineralisation occurs.
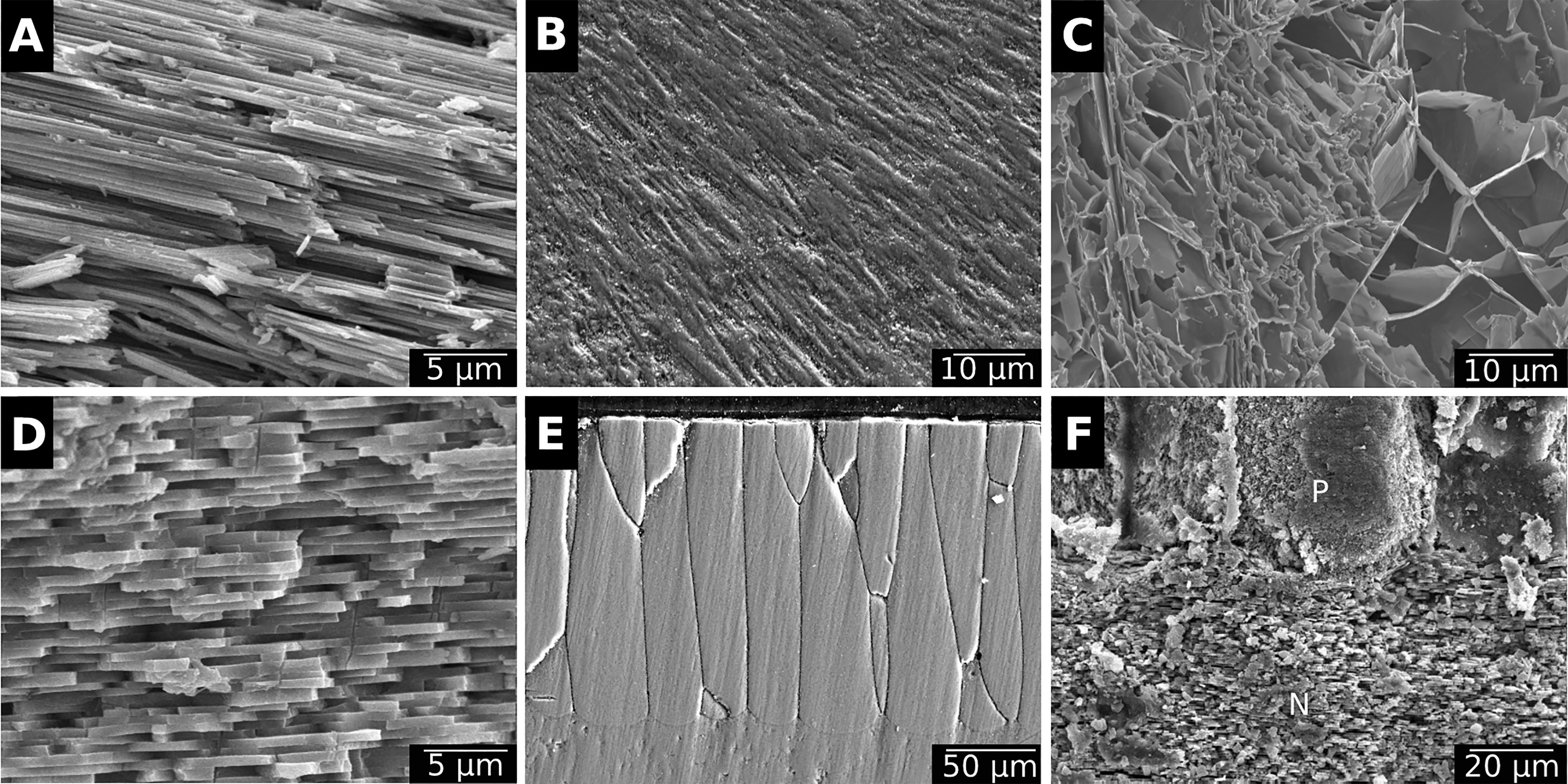
Figure 2 Examples of the various microstructures found in bivalve shells. (A) Crossed foliated calcite in the outer layer of the Antarctic scallop Adamussium colbecki. (B) Fibro-prismatic calcite from the outer layer of the deep-sea mussel Bathyomodiolus azoricus.(C) Chalky calcite in the shell of the Japanese oyster Magallana gigas. (D) Nacre in the inner layer of the deep-sea mussel B. azoricus.(E) Prismatic aragonite from the outer layer of the freshwater mussel Anodonta cygnea. (F) Contact between the prismatic (P) and the nacreous (N) layers in the shell of A.cygnea.
Bivalves shells are produced by the mantle, a polarised tissue composed of three layers: the outer epithelium, the internal tissue and the inner epithelium (Figures 3A, B). The outer epithelium is also called the calcifying epithelium. The edge of the mantle, where mineralisation occurs, is usually composed of three lobes: the outer, middle and inner lobes having different cell types and therefore different functions (Figures 2, 3A). The basal bulb, a group of cells located at the base of the outer and middle lobes (Figure 3B), produces the periostracum which covers the outer shell isolating from the external environment a space between the mantle and the shell, called extrapallial space (EPS). The EPS is filled with the extrapallial fluid (EPF), a supersaturated fluid retaining shell components in solution before their incorporation into the matrix (Richardson et al., 1981; Marin et al., 2012). The inorganic part of the EPF is constituted of precursor ions of calcium carbonate and also of others ions such Na+, K+, Mg2+, Cl- and . Bicarbonate and chloride ions concentrations are higher in the EPF than in sea water, the other ions being present in approximatively the same concentration in both fluid (Wilbur and Saleuddin, 1983). Calcium intake by bivalves occurs at different places. Calcium absorption from water takes place in gills and from food in the digestive system. The ions can reach the extrapallial space passively by diffusion through Ca2+ channels, actively by Ca2+-ATPase, but also by intercellular spaces (Figure 4) (Carré et al., 2006). Dissolved inorganic carbon (DIC) from seawater is a source of . Bicarbonate ions are transported to the EPS via active transport though exchangers and transporters (Coimbra et al., 1988; Marin et al., 2012; Alves and Oliveira, 2013). The metabolism also releases CO2, which can be transformed by the carbonic anhydrase (CA) into . Carbonic anhydrase also generates hydrogen ions, acidifying the EPS. Therefore, an active pumping regulates the pH of the EPF (Marin et al., 2012).
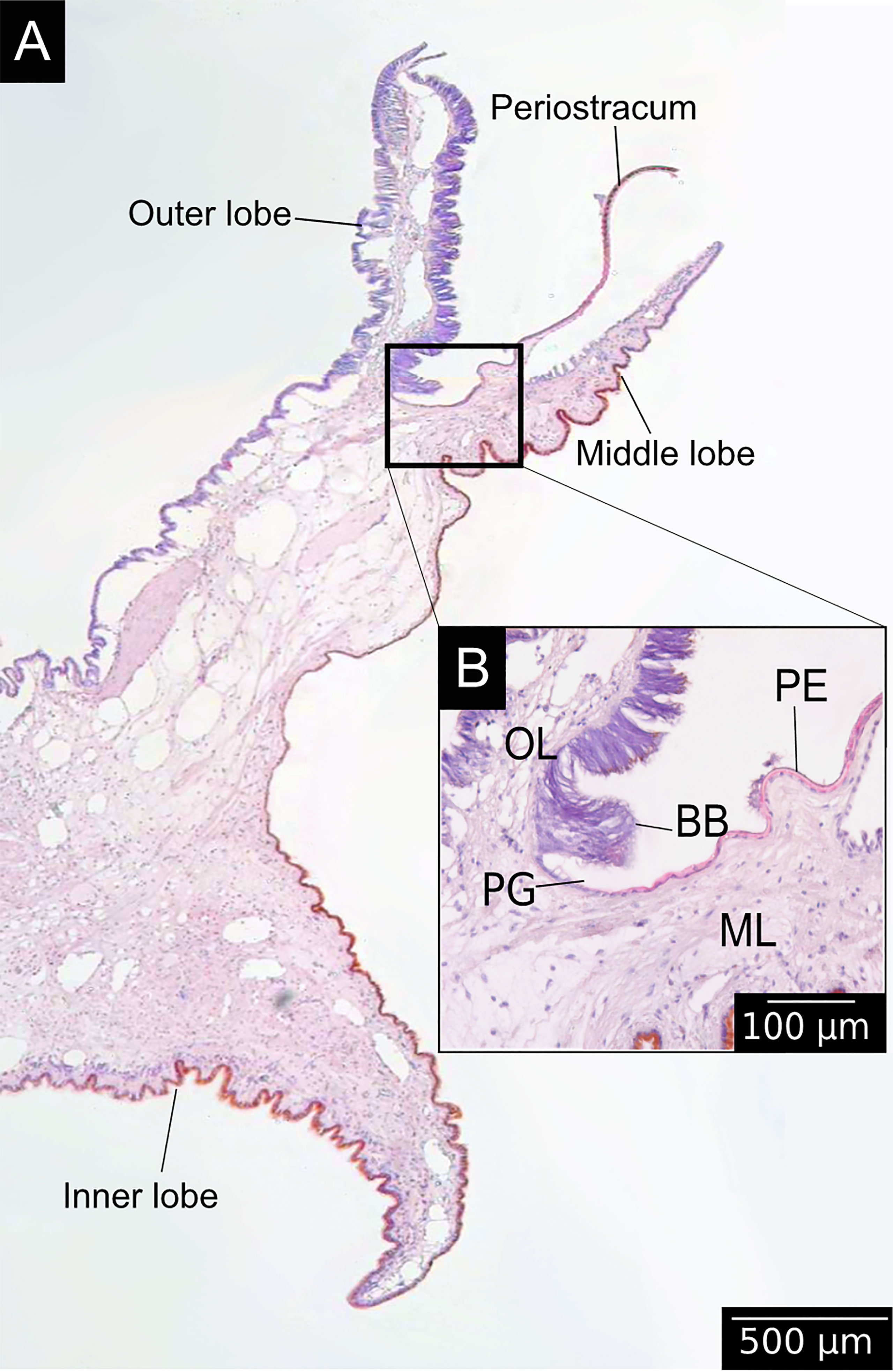
Figure 3 Longitudinal histological section of the mantle edge of M. galloprovincialis stained with hematoxylin and eosin. (A) View of a histological section of the periostracum bound to the mantle, in an area divided in three lobes: the outer lobe, the middle lobe and the inner lobe (see the position in the organism in Figure 1). (B) Details of the periostracal groove (PG) located between the outer (OL) and the middle (ML) lobes. The basal bulb (BB) is a group a of cells secreting the periostracum (PE).
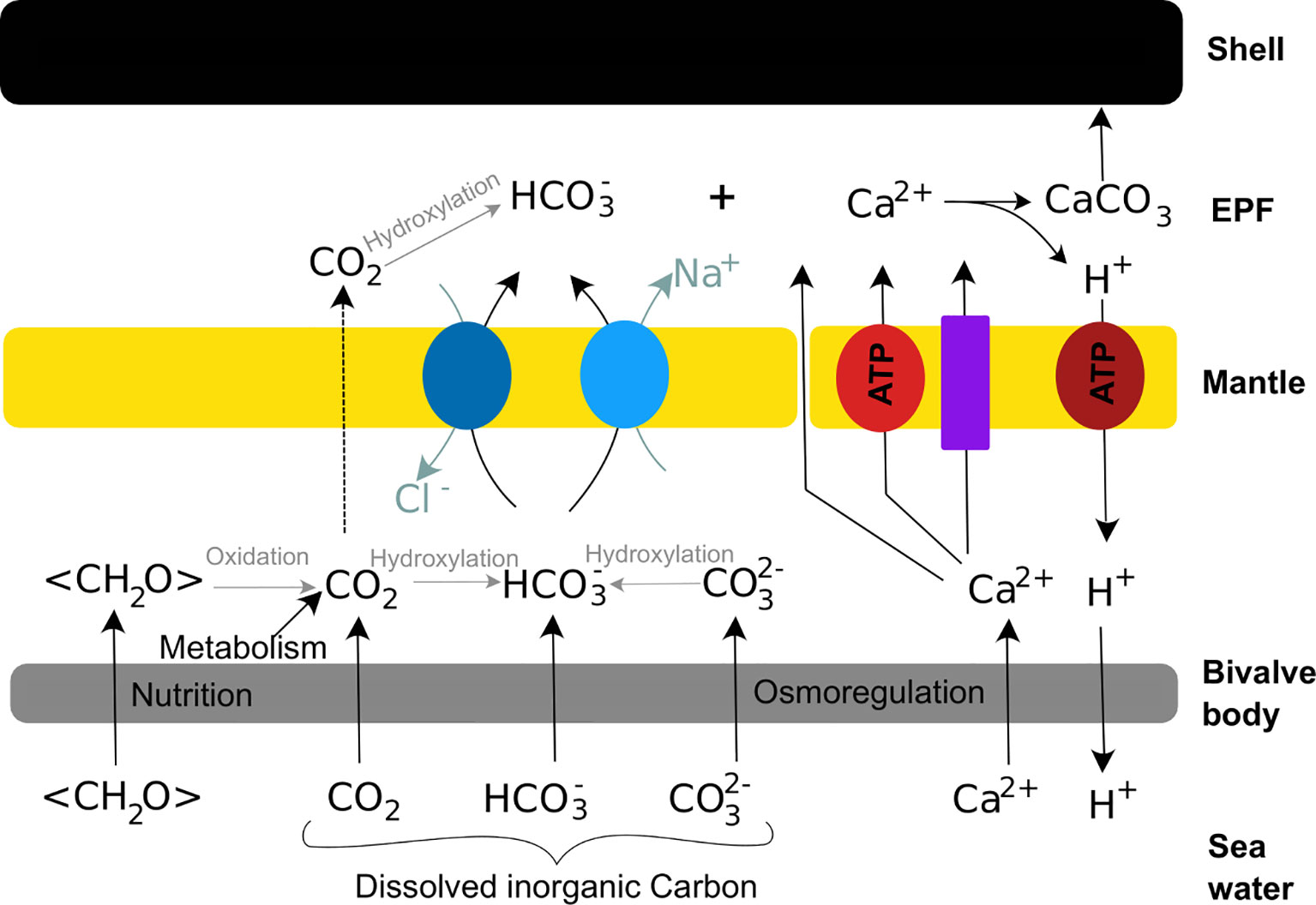
Figure 4 Schematic calcification model of bivalve shells. Calcium reaches the extrapallial fluid (EPF) passively through Ca2+ channels (purple rectangle) and cells junctions, and actively throughout Ca-ATPase (light red circle). Bicarbonate ions resulting from dissolved inorganic matter (DIC) and nutrition join the EPF through exchangers (dark blue circles) and transporter (light blue circles). Carbon dioxide diffuses trough cells to the EPF (dotted arrow). The excess of proton produced by the formation of calcium carbonate is actively pumped out the EPF (dark red circle).
The mineralisation of a calcium carbonate crystal begins with the nucleation, which consists of a highly thermodynamically instable nucleus of CaCO3 regulated by a local supersaturation of organic material. This step is especially favoured by differential expression of polysaccharides and proteins that have different charge density through time (Giuffre et al., 2013). Then, crystal growth and organisation are biologically and physically controlled (Checa, 2018). Two physical control mechanisms are described. The first one is the crystal competition, where the fastest growth axis is outcompeting the others, this type of physical process is observed in case of foliated calcite microstructure and nacre synthesis of bivalves (Checa et al., 2006; Checa et al., 2007). The other physical process is the self-organisation of the organic matrix, acting as a template for crystal growth (Checa, 2018). The precursor of the organic template is a fluid that is self-organised by surface tensions (Checa et al., 2005; Checa et al., 2016). This has been described in the freshwater mussel Cristaris plicate, where an organic honeycomb template determines crystal growth direction and size (Tong et al., 2002). The process of self-organisation is coupled with a biological control by the subcellular recognition of the extracellular matrix via its physicochemical properties (Checa et al., 2005; Checa, 2018). This relationship between mantle cells and the extracellular matrix leads to the secretion by the outer epithelium cells of either organic (fluid precursor) or mineral materials. The biological control by the outer epithelium of the mantle is assumed to involve molecular pathways. First studies have found four transcription factors namely Pf-MSX (Zhao et al., 2014), Pf-AP-1 (Zheng et al., 2015), Pf-Rel (Sun et al., 2015) and Pf-POU3F4 (Gao et al., 2016), and two receptors called PfBMPR1B and PfBAMBI (Li et al., 2017) involved in the regulation of the matrix proteins of the pearl oyster Pinctada fucata. More recently, bioinformatic tools have been used in order to predict molecular pathways involved in the biomineralisation process. The molecular response to shell damage of the Antarctic clam Laternula elliptica has been used to observe the restart of the biomineralisation (Sleight et al., 2020). RNA-seq data analysis underlines two hypothetical pathways: one linked to ions and proteins transport from the outer epithelium to the biomineralisation area, and the other linked to the secretion of organic matrix proteins within the nacreous layer of the shell. This work also points out new potential receptors for which further studies are now required to confirm their putative role in the biomineralisation process.
SHELLS AS BIOLOGICAL ARCHIVES
The molecular pathways involved in the biomineralisation process are constantly activated throughout bivalve’s live. This means that shell formation should be continuous. However, bivalve shell growth rate is not constant from larval to adult stages (Schöne, 2008). The growth can be described by a Von Bertalanffy growth model, where juvenile specimens have an exponential growth while the growth rate in older individuals slows down and stabilises. At a higher temporal resolution, growth slows down or stops on a regular basis (Lutz and Rhoads, 1977; Karney et al., 2012). This phenomenon is characterised by low carbonate deposition while the organic matrix is still produced, inducing a change in the ratio between mineral and organic material, and the formation of growth lines. Growth lines separate the shell formation into small units of time, so-called growth increments or rings (Figure 5). The growth increment have thus to be distinguished from the shell microstructure, as the later only refers to the crystal shape. Growth lines are discernible very early during the development of bivalves. The first accretion line is visible after 32 hours post fertilisation in M. galloprovincialis larvae (Miglioli et al., 2019). This corresponds to a major body organisation change during the planktonic phase, when the larva turns from a ciliated free-swimming planktonic larval form, called trochophore, into a D-veliger larva, meaning a free-living planktonic larva with a shell. Growth increments are then produced during the entire life of the organism.
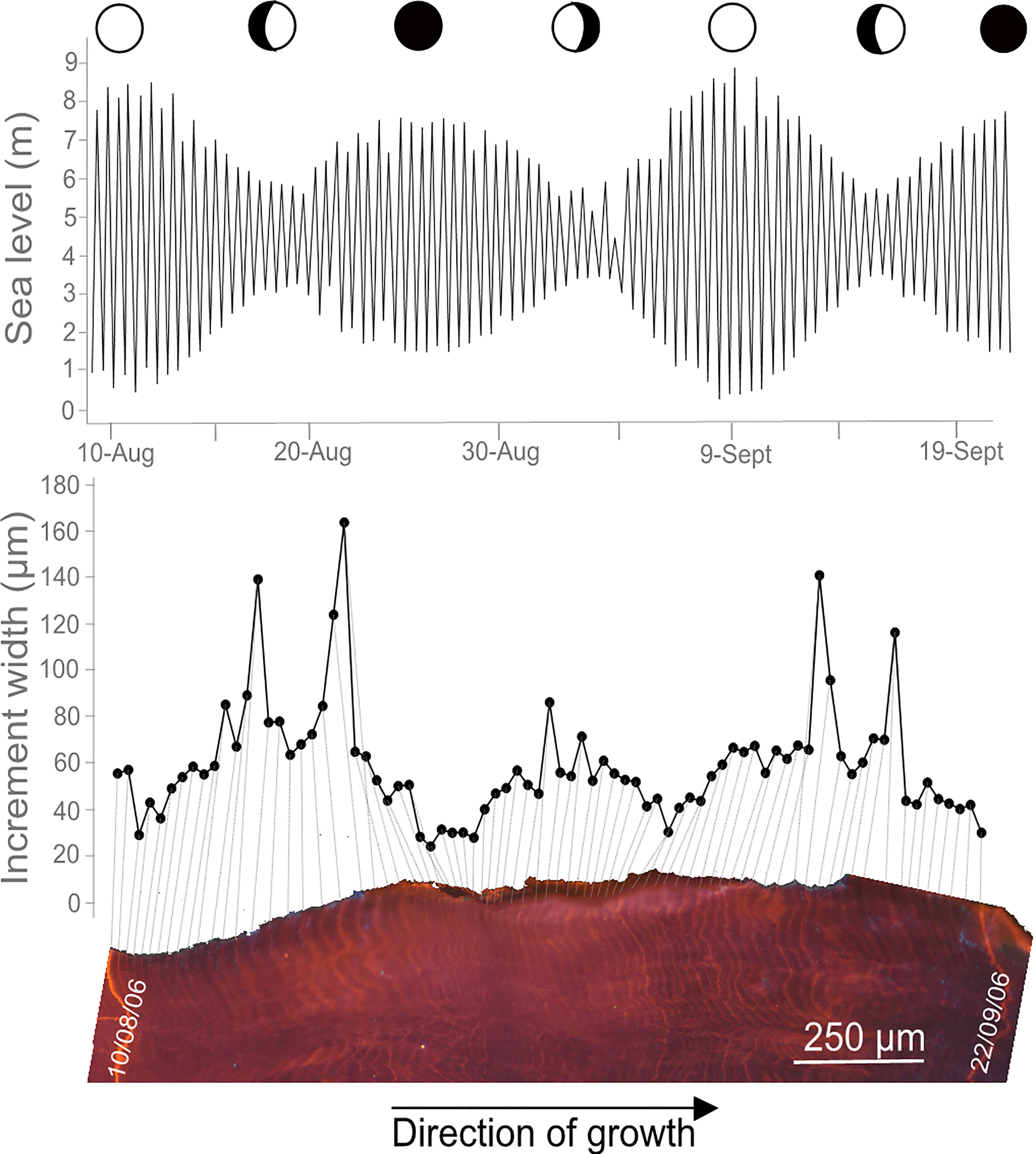
Figure 5 Growth increments in Magallana gigas in relation with its environment. Section of the hinge area of M. gigas shell showing growth increments and Mn-staining under cathodoluminescence microscopy. The increments are formed tidally and vary in width following the semi-lunar cycle, with higher growth rates during neap tides, likely related to temperature and phytoplankton changes in the surrounding environment (see Huyghe et al., 2019 for further details).
It is observed that the shell growth lines mainly result from periodic (e.g., seasonal, daily, tidal) processes, although random growth anomalies can appear (i.e., induced by stressful events such as predation, storm, pollution) (Schöne, 2008). Therefore, growth pattern observable on the shell of an individual primarily reflects the variability in its environment (Figure 5). Considering that shells record the environment surrounding organisms, they can be considered as biological archives.
A bibliographical survey based on the keywords “bivalve shell” and “sclerochronology” has gathered 55 bivalve species investigated for their shell growth patterns (Figure 6A). This survey includes species from various types of environments, from freshwater to the deep-sea and from polar to tropical waters. Annual growth lines are the most common observed pattern in the literature (>60% of bivalves), particularly in the shells from mid and high latitude species, and are mainly associated to winter low temperatures that slow down or even stop the growth (Schöne, 2013; Killam and Clapham, 2018) (Table 1). They may also be due to spawning events, assuming that this paramount physiological trait is very energy-consuming, limiting calcification (Jones, 1980). Counting annual growth lines in long-lived species can reveal growth rate changes at lower frequencies, such multi-annual environmental dynamics. The ocean quahog Arctica islandica and the pearl freshwater mussel Margaritifera falcata exhibit long-term growth periods related to the North Atlantic Oscillation (NAO), which is characterised by colder winters every 5 to 30 years (Schöne et al., 2003a; Schöne et al., 2007).At shorter time-scales, one and two increments per day are classically observed (Schöne et al., 2005). Interestingly, the bibliographic database reveals that in bivalve shells daily growth increments are more commonly observed than semi-daily ones (Figure 6A). While semi-daily increments are closely related to the tidal regime in areas exhibiting two tides per day, daily increments can both correspond to circadian (24h) or circalunidian (24.8h) growth rate changes (Hallmann et al., 2009; Schwartzmann et al., 2011).
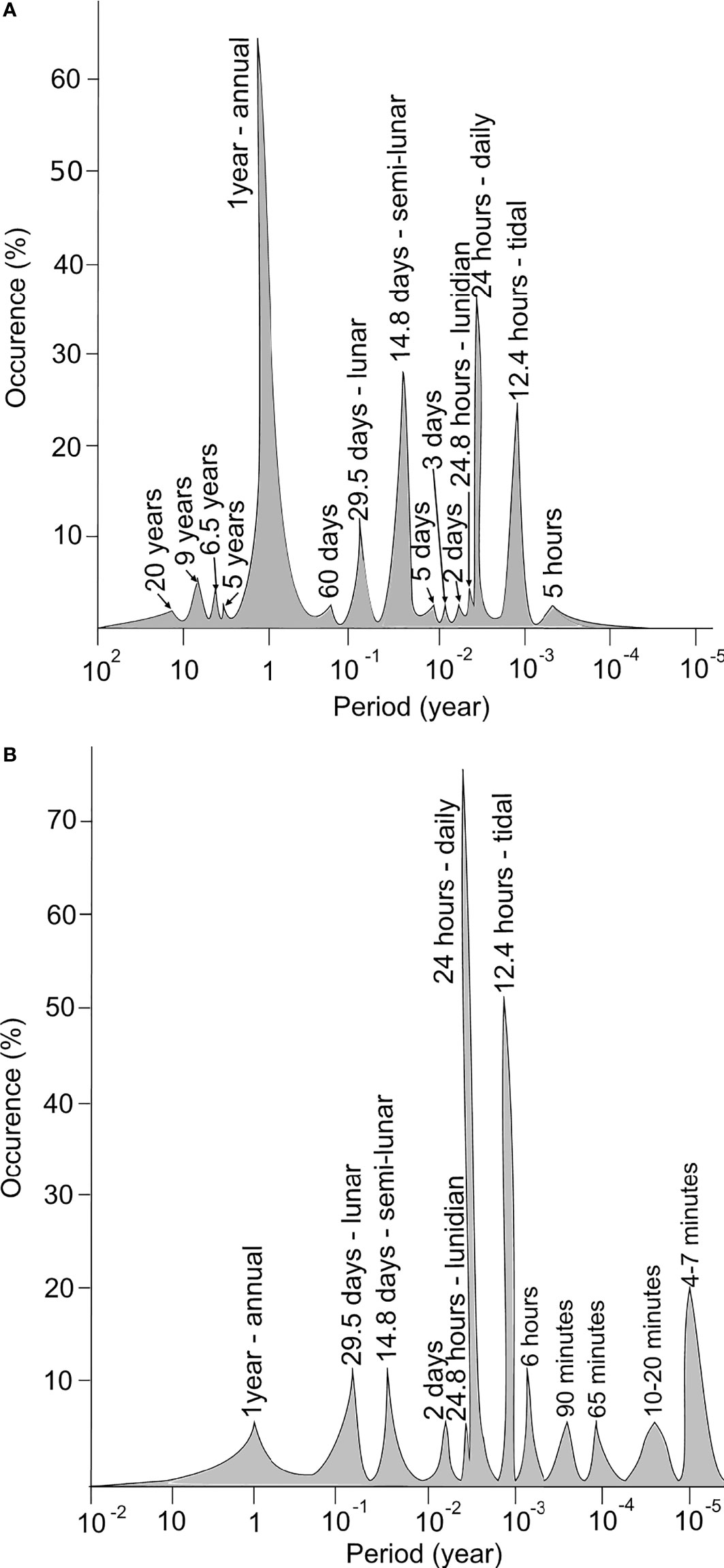
Figure 6 Bibliographic review of periodicities related to the shell growth patterns (A) and biological clocks in bivalves (B). The bibliographic survey for shell increments has been based on the keywords “bivalve shell” and “sclerochronology” and gathers 55 species. The bibliographic review for the biological clocks has been done with the keywords “biological clock bivalve”, “circadian clock” and “valvometry”, and gathers 17 species. Articles involving ecotoxicological studies are not taken into consideration.
The growth line count also highlights frequent growth rhythms at periods close to 14.8 and then 29.5 days, that are related to semi-circalunar and circalunar fluctuations (Figure 6A; Table 1). Less conventional growth cycles are observed (i.e., 60 days, five days,…) but their relationship with environmental cues are poorly described or correspond to local specific conditions. The 60 days pattern observed in the shell of the deep species Bathymodiolus thermophilus might reflect current velocity changes in the hydrothermal habitats induced by ridge-crest jets (Nedoncelle et al., 2013; Nedoncelle et al., 2015). The five and three days patterns of the mussel M. galloprovincialis observed in the Mediterranean lagoon of Salses-Leucate (France) could be due to regular wind events occurring in this area (Andrisoa et al., 2019).
The two-day periodicity recorded in the shell of the tropical Comptopallium radula could be synchronised with temperature or sea level pressure as both of these parameters display two-day cycles (Thébault et al., 2006).
Although a synchronism with environmental variables appears, the precise origin of growth increments is still discussed in literature. While the integration of environmental variables in shells is a fundamental question for the community of sclerochronologists (Trofimova et al., 2020), two hypotheses have been proposed to explain increment formation: it may be directly controlled by environmental parameters or mediated by biological clocks.
Environmental Drivers in Shell Formation
As illustrated above, tidal rhythms have been identified in the growth patterns of bivalve shells. Lutz and Rhoads (1977) linked this pattern to direct environmental constraints on the physiology of the organisms. At low tide during the emersion period, valves are closed; the metabolism becomes anaerobic, inducing a drop of pH and then an increased calcium ions concentration in the EPF. This has been interpreted as a phase of decalcification; calcium ions integrated into the shell are re-solubilised into the EPF. This hypothesis is sustained by analogous observations made in the EPF of multiple bivalve species such as Mercenaria mercenaria and Crassostrea rhizophorae (Crenshaw, 1972; Littlewood and Young, 1994). When the cockle M. mercenaria closes its valves, the pH decreases and the Ca2+ concentration increases within 15 minutes (Crenshaw, 1972). The same observation has been made in the case of the tropical oyster C. rhizophorae (Littlewood and Young, 1994). At high tide, valves open and metabolism becomes aerobic again. Subsequently, the biomineralisation activity starts again in an area already containing organic material but that has lost its CaCO3, leading to the formation of a growth line (Lutz and Rhoads, 1977). The increment is formed in a second time during the immersion. This has been observed in different bivalve species such as Saxidomus gigantea which is subjected to semi-diurnal or diurnal tides as a function of its location along the West coast of America (Hallmann et al., 2009). When bivalves are exposed to semi-diurnal tides, a faint additive line assumed as a growth line, appears inside of the increment. Therefore, bivalves exposed to semi-diurnal tidal regimes exhibit shells forming two increments per day, while those that experience daily tides produce one increment per lunar day. Also, the distinctness of the growth line is often linked with the duration of the emersion (Richardson, 1987; Schöne et al., 2003b; Hallmann et al., 2009).
Emersion/immersion cycles are not the only environmental variable reported to initiate increment formation as many bivalve shells from subtidal or freshwater ecosystems also exhibit such tidal growth rhythms (Verrecchia, 2005; Poulain et al., 2011). For example in the deep-sea, tidal increments are observed in the shell of the hydrothermal mussel B. thermophilus, living at 2,500 m depth, and interpreted as a result of tidal oscillations in the vent systems (Nedoncelle et al., 2013; Nedoncelle et al., 2015). The role of temperature, salinity, dissolved oxygen or nutrient availability is thus regularly mentioned as there is a general consensus in the literature that environmental fluctuations drive the increment width variation (Rodríguez-Tovar, 2014). Other subtidal bivalve species like Pectinids exhibit daily growth increments in their shell, likely related to the day/night light alternation. In an experiment conducted to understand the potential environmental drivers of such daily increments, Clark (2005) submitted Flabellipecten diegensis to shorter night and day cycles by having an alternation of eight hours of light and eight hours of darkness. After 22 days, specimens had experienced 33 light/dark cycles and had more or less 30 increments. Changing light exposure is thus suggested as a potential shell production driver.
Biological Clocks in Bivalves
Interestingly, bivalves from intertidal flats constantly immersed and under stable conditions of temperature, water flow, light and food supply in the laboratory, show semi-daily increment formation. This has been observed for the common cockle Cerastoderma edule (Richardson et al., 1980) and the Manilla clam Ruditapes philippinarum (Richardson, 1988) and interpreted as possibly resulting from endogenous rhythms in the process of biomineralisation controlled by biological clocks. In this design, the environment acts as a time giver for endogenous clocks. Since Richardson pioneer studies, biological clocks are now regularly mentioned as a timer for bivalve shell increment formation (Schöne, 2008; Poulain et al., 2011; Warter et al., 2018; Zhao et al., 2020).
Biological timing devices, named biological clocks, are known to orchestrate the biological activities of organisms with the environment, allowing the organisms to anticipate cyclic variations and to increase their fitness (Rosbash, 2009). The clock is made of three major components: i) a central molecular oscillator that keeps track of time, ii) environmental inputs that give the time (also named zeitgebers) and control the central oscillator to ensure the entertainment of the clock and iii) a series of rhythmic outputs corresponding to rhythms of biochemical, physiological or behavioural activities of the organism such as the daily migration throughout the water column by phytoplankton (Figueroa et al., 1998) or the tidal valve gape activity of bivalves (Comeau et al., 2018; Tran et al., 2020) (Figure 7). The clock is set and reset by environmental cues and can be auto-entertained, which means that the oscillations persist under constant conditions (Aschoff, 1981). The biological clock is a time-keeping system highly conserved among organisms and its constituents are described for many species, from cyanobacteria, fungi to mammals among others (Ditty et al., 2003; Tan et al., 2004; Partch et al., 2014). Although its structure can slightly differ, the core is based on one or coupled feedback loops composed of activating and repressing elements. The outputs of this conserved structure are circadian (Dunlap, 1999). Homologous clock genes (clock and bmal as activators and cryptochrome 1 and 2, period and timeless as repressors) have been described for bivalves, such as the oyster Magallana gigas (Perrigault and Tran, 2017), the mussels M. edulis (Chapman et al., 2020) and M. californianus (Connor and Gracey, 2011), the scallops Argopecten irradians (Pairett and Serb, 2013) and Chlamys islandica (Perrigault et al., 2020) and even in the deep-sea [i.e., Bathymodiolus azoricus (Mat et al., 2020)]. The main periods associated with biological clocks in bivalves are daily and tidal, but others with lower (several days to a year) or higher frequencies (hours to minutes) have also been reported (Figure 6B).
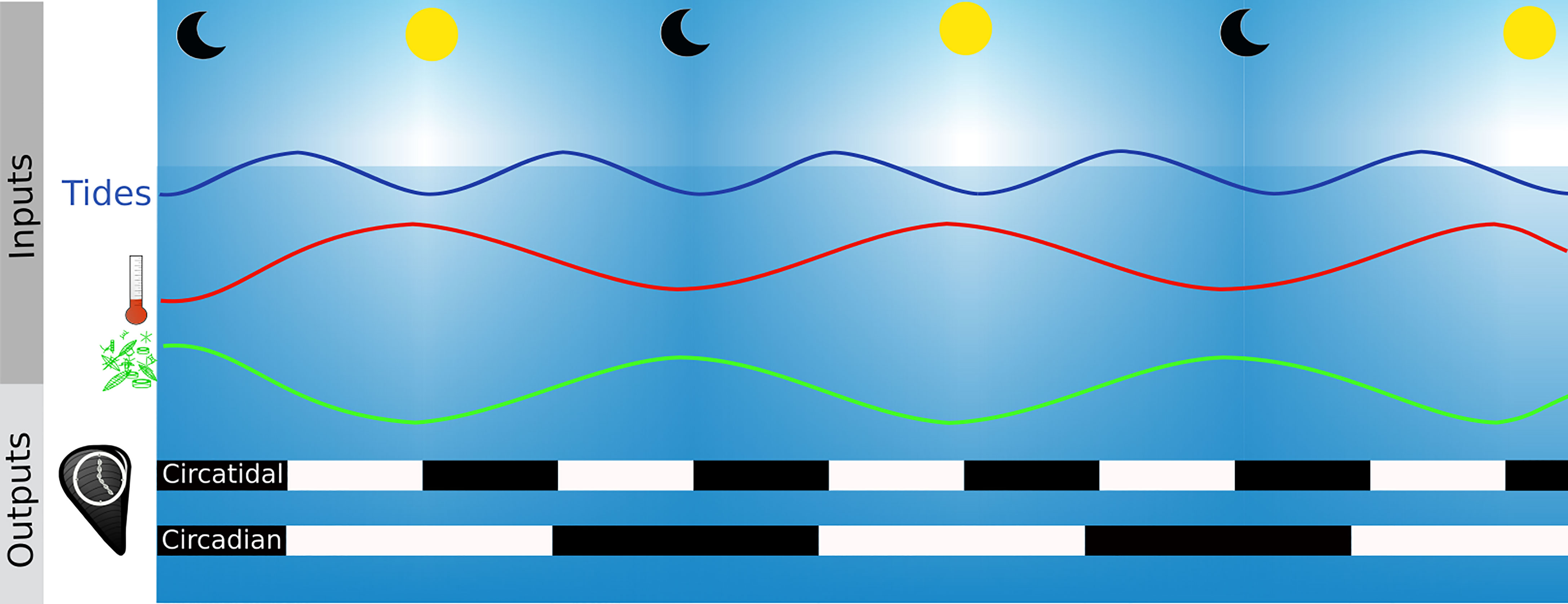
Figure 7 Functioning of biological clocks. Clock inputs are environmental and biotic variations called zeitgebers such as the photoperiod, the thermoperiod (red line), tides (blue line) or phytoplankton migration in the water columns (green lines). The zeitgeber is the time giver of the molecular clock of organisms and the outputs are rhythmic periodical processes such as mussel valves opening (black and white bars). Black bars are time when mussels are closed and white bars when they are open.
Photoperiod and temperature are the main zeitgebers of the circadian biological clock (Dunlap, 1999; Rensing and Ruoff, 2002). Experiments on M. edulis have shown that genes of the endogenous clock (i.e., Clk, Cry1, ROR/HR3, Per and Rev-erb/NR1D1) oscillate with a different amplitude when bivalves are placed in complete darkness (DD- Dark/Dark condition) with a 24 hours temperature cycle of 3.6 ± 0.2°C compared to those placed in DD and constant temperature (Chapman et al., 2020). It should be noted that the clock system responds to a periodical variation of the temperature and not to the temperature as a calorific energy. Part of the definition of a biological clock is that the system is temperature compensated. This means that the molecular reactions involved in the feedback loop are not slowed down in cooler temperatures or accelerated in warmer temperatures. Otherwise the benefit of having a biological clock is lost because the given time would be different in function of the temperature.
Various theories have been proposed to explain the circatidal rhythm observed in organisms living in littoral areas, based on either (i) one unimodal circatidal clock, strictly distinct from one unimodal circadian clock (Naylor, 1958), (ii) two unimodal circalunidian clocks coupled in antiphase (Palmer and Williams, 1986) or (iii) one single bimodal oscillator governing both circadian and circatidal patterns (Enright, 1976). Recent work of Tran et al. (2020) suggests that oysters are in line with the third hypothesis. Similar studies on other species should be performed to check if this hypothesis is valid for all bivalves.
In chronobiology, the behaviour of an organism is frequently used as an output of biological clocks (Bartness and Albers, 2000). As indicated before, in bivalves shell gaping is a useful tool to study rhythmic behaviour (Perrigault and Tran, 2017; Comeau et al., 2018). Valves gape activity can be measured by valvometry. This technique consists in a Hall sensor and a magnet glued on each valve in order to continuously record the voltage, which is proportionally disrupted by the valve aperture through the decrease of the magnetic field (i.e., magnetic flux density) (Nagai et al., 2006). Analyses conducted in this field revealed a predominantly circatidal valve activity for bivalve species from tidal environments (Tran et al., 2011), while species from subtidal and freshwater habitats exhibit circadian rhythms (García-March et al., 2008; Schwartzmann et al., 2011; Hartmann et al., 2016; Comeau et al., 2018). In aquaria, M. gigas submitted to a photoperiodic regime [i.e., 12 hours of light and 12 h in darkness (LD 12:12)], without tidal current, shows a circadian activity of valve aperture (Mat et al., 2012). Free-running conditions, which means the suppression of environmental inputs, is used to test for the endogenous character of the biological clock. Under constant light conditions (i.e., total darkness (DD) or continuous light (LL) over 24 hours), the circadian behaviour of M. gigas is kept although a gradual shift appears from a nocturnal to a diurnal activity after two months (Mat et al., 2012). The authors concluded that the circadian clock does exist but is weak in oysters.
A yearlong study on M. gigas in the Arcachon Bay, on the Atlantic shoreline in France, described an effect of the sun-earth-moon system on their behaviour (Tran et al., 2011). Constantly immersed, oysters have mainly a tidal shell gaping activity. This tidal activity changes around the synodic month with a longer closure time of the valves during neap tides and an increase with spring tides. Therefore oyster behaviour also follows circalunar oscillations and might be entertained by clocks at such frequencies.
Rhythms of Shell Biomineralisation: The Biological Clocks Hypothesis
As seen before, environmental rhythmic variations do not exclusively provide explanations regarding the number of increments formed per day, which raises the question of an auto-entertained rhythm of biomineralisation. Similar periodicities observed in shell growth patterns and clock genes expression of bivalves (Figures 6A, B) support the prevailing concept that biological clocks are likely to be involved in the control of rhythms of biomineralisation. The existence and nature of the interaction between the two remain to be determined. Although valvometry, a classical output of biological clocks in bivalves, can be used to measure the shell growth rate, direct relationships between valve activity and growth increment formation is still lacking (Schwartzmann et al., 2011; Andrade et al., 2016). The oyster M. gigas exhibits changes in the width of tidal increments according to the moon cycle, showing circalunar (i.e., 29.5 days) and semi-circalunar (i.e., 14.8 days) variations (Huyghe et al., 2019). The tidal activity in valve closure also describes a synodic frequency (Tran et al., 2011). Surprisingly, studies showed that shell growth increments (i.e., growth rate) are wider during neap tides, while valve opening increases at spring tides (Tran et al., 2011; Huyghe et al., 2019). Precautions must be taken when comparing these studies, because oysters have been reared in different habitats; they were continuously immersed in the experiment of Tran et al. (2011) while specimens of Huyghe et al. (2019) were studied in an intertidal habitat with periodic aerial exposures.
At the molecular level, a single bimodal circadian/circatidal clock can entrain valve aperture of oysters at daily or tidal frequencies, following the predominance of the environmental drivers (Mat et al., 2012; Tran et al., 2020). However, such plasticity has not been demonstrated yet nor correlated with daily or tidal incrementation in shells for a single population according to their environment. Oysters constantly bred immersed in the French Mediterranean Thau lagoon, characterised by a reduced tidal regime (<20 cm), exhibit daily growth increments rather than tidal ones as observed in oysters from coastal area of the English Channel (Langlet et al., 2006; Huyghe et al., 2019). Water temperature and phytoplankton vertical migrations, known to be associated with the photoperiod, have been proposed as potential drivers of the daily dynamics of shell growth (Huyghe et al., 2019). Similar observations have been made in mussels. They exhibit tidal growth increments in areas dominated by a strong tidal regime, such as along the coast of the United Kingdom and offshore in the North Sea, independently from their position in the water column (i.e. in intertidal and subtidal conditions) (Richardson, 1989; Richardson et al., 1990). Also, daily growth increments are formed in the French Mediterranean Salses-Leucate lagoon, where tides are strongly reduced (Andrisoa et al., 2019). Altogether, these data suggest that biomineralisation rhythm could vary within the same species and might be controlled by the strongest clock driver present in its environment. This could occur through the activation of genes involved in biomineralisation processes by the biological clocks. For some of genes (i.e., coding for chitinase, nacrein, N16 and MSI60), seasonal and circadian expression rhythms have been described in molluscs (Miyazaki et al., 2008; Banni et al., 2011). Moreover, the mantle transcriptomes of three Pectinids (i.e., Pecten yessoensis, Pecten magellanicus, Argopecten irradians) has allowed to identify the expression of genes (i.e., cry, calmodulin, opsin and rhodopsin among others) with light-mediated functions, tightly linked with biological clocks (Sun et al., 2016). The research area called “shellomics” (i.e. proteomics applied on shells), could help decipher the molecular toolbox for shell construction (Marin, 2020). Omics applied on a temporal basis would help to understand the possible link between biological clocks with the shell growth.The deep-sea habitat is a promising environment to test the hypothesis of the role of biological clocks on shell biomineralisation and growth rhythms as many environmental drivers are constant or exhibit slight oscillations (e.g., photoperiod, temperature). Mussels living at deep-sea hydrothermal vents produce lunidian and tidal growth increments, as observed for Bathymodiolus brevior from the South-West Pacific at 2000 m depth (Schöne and Giere, 2005) and B. thermophilus on the East Pacific Rise at 2500 m depth (Nedoncelle et al., 2013; Nedoncelle et al., 2015). Measurements of pressure, current velocity and orientation, temperature, pH and sulfide concentration in the deep-sea habitat revealed daily and tidal changes (Nedoncelle et al., 2015), which might result from tidal pumping of vent fluids and mixing with the deep and cold seawater (Crone and Wilcock, 2005; Scheirer et al., 2006). Deep-sea mussels harbour chemosymbionts in their gills that are able to use specific nutrients present in the hydrothermal flume such as sulfide or methane. As a result, the symbionts provide an important source of organic carbon and thus energy fuel for the mussel (Duperron, 2010). This energy supply is used for shell growth when, alternatively, mussels benefit from oxygen of seawater and their symbionts are exposed to electron donors (i.e., H2S). Growth rates are greater during periods of larger tidal ranges (Nedoncelle et al., 2015). Interestingly, in situ behavioural and transcriptomic analysis of biological clock genes of the deep-sea mussel B. azoricus from the Mid-Atlantic Ridge, showed that circatidal oscillations are dominant although a circadian response is observed in the laboratory, when mussels are submitted to daily stimuli (i.e., LD photoperiodic condition) (Mat et al., 2020). Based on evidences related to the fractionation of carbon isotopes (i.e., disequilibrium in the relative partitioning of heavier and lighter isotopes between seawater and shells) induced by chemosymbionts activity, the shell δ13C could be used to track changes in community composition over time, particularly the ratio between methanotrophic and sulfo-oxidising symbionts for bivalve species which host a dual symbiosis, as suggested for Bathymodiolus from various hydrothermal vent habitats (Nedoncelle et al., 2014).
The role of symbionts on the physiology of bivalve is an important component for species that host photosynthetic algae in their tissues as phototrophy is the main source of energy for them (Klumpp and Griffiths, 1994). Effects of symbionts on biomineralisation is likely explained by the hypothesis of the light-enhanced calcification (LEC), in which symbionts might increase the energy and the (in)organic components supply throughout photosynthesis (for review see Allemand et al., 2011). The LEC is the current explanation for the higher calcification rate of photosymbiotic species (few cm per year) compared to other non-symbiotic close-related species (Beckvar, 1981; Hawkins and Klumpp, 1995; Allemand et al., 2011). The photoperiod is likely implicated in the shell incrementation rhythm as all symbiotic bivalves show daily growth bands (Pannella and MacClintock, 1968; Schwartzmann et al., 2011; Gannon et al., 2017). The valve activity also exhibits daily patterns, from widely open during the day to provide light to symbionts, to partially or entirely closed at night, as illustrated for the giant clam Hippopus hippopus (Schwartzmann et al., 2011). At molecular scales, multiple studies have been done on Tridacna squamosa proteins linked to the biomineralisation process. Light-dependent expression and protein levels are reported in most of them (Ip et al., 2015; Boo et al., 2017; Hiong et al., 2017; Ip et al., 2017; Ip et al., 2018; Cao-Pham et al., 2019; Chew et al., 2019). Rhythmic production pattern under various photoperiodic conditions (e.g., LD, DD, LL) of those proteins has not been studied yet and could indicate if a biological clock is driving their synthesis.
The Smallest Time Units Recorded in Shells
Daily to tidal units are classically recognised as the basic timeframe of the growth by increment in bivalve shells. But as suggested above (Figure 6A), smaller time units have been described (Yamaguchi et al., 2006; Huyghe et al., 2019). They are reported as the consequence of several growth breaks during the formation of a growth increment, leading to the production of five to ten sub-increments, named microincrements, per day without recognisable factor controlling their periodicity. Actually, ultradian growth stops are not that infrequent in bivalve shells, as illustrated by Dauphin et al. (2003), with amounts of micro growth lines at micrometer scales in the shells of Pinctada margaritifera and Pinna nobilis. Schöne (2008) has also reported the presence of several ultradian growth microincrements within daily increments in the shell of Chione cortezi and Anodonta cygnea. Close similar growth microincrements were observed in the skeleton of the coral Pocillopora damicornis and in mouse teeth (Brahmi et al., 2012; Ono et al., 2019). The mechanisms involved to produce those growth microincrements remain however unknown.
In the case of bivalves, it is suggested that under stressful conditions (e.g., temperatures above 31°C for M. galloprovincialis and Merceneria merceneria), the impaired physiological activity may affect shell growth (Ansell, 1968; Anestis et al., 2007). But it is also assumed that ultradian metronomes must be essential to orchestrate cellular functioning, such as cell division, heart rate and chemical reactions (Lloyd and Murray, 2005). Based on video records, Rodland et al. (2006) measured the behavioural activity of three bivalve species (A. cygnea, A. islandica and M. edulis) and found rhythmic siphon activities on a period of 3 to 7 min and 10 to 20 min, with a duration of valve contraction over 60 to 90 min. These authors suggest that intrinsic mechanisms (i.e., guided by physiological and/or genetic factors), rather than the environment, control such periodicities. The direct link between intrinsic mechanisms and shell microincrements is not established but Rodland et al. (2006) hypothesised that hourly rhythms in valve opening could produce microincrements between 1 and 5 µm, similar to those observed by Schöne et al. (2002) inside the lunidian increments of C. cortezi. However microincrements could also be the result of environmental variations, as illustrated by Yan et al. (2020) with the observations of microbands of different fluorescent intensity under confocal microscope in the giant clam Tridacna spp. Coupled with geochemical tools (in this case nanoSIMS), the authors revealed hourly changes of Fe/Ca ratios into shells, likely due to vertical mixing of iron in the water column during storm events. Further investigations on these short-term growth units and the search for biological clocks at higher frequencies in bivalves are required. Many studies pointed out ultradian endogenous timekeeping mechanisms in vertebrates that are meeting the temperature compensation and the auto-entertainment assumptions but no genes involved in the central molecular oscillator has been proposed yet (Ono et al., 2015; Ghenim et al., 2021).
Further Research Directions
The unit of time in shell, the increment, is well described and often used to infer a time frame to observations made in biomaterials. Unfortunately, the environmental drivers leading to the periodic formation of an increment are still poorly understood. The question is difficult to elucidate as there is probably not only one environmental cue driving the process but rather a combination of several factors among which a hierarchy likely exists. Biological clocks might be involved in increments formation but the regulation between clock and biomineralisation genes, and the production of the resulting growth increment, still need to be deciphered. A gap between the distinct scientific communities working on biomaterials can now be bridged through interdisciplinary researches across molecular biology, ecology and earth sciences. Observations made in this review could be used as some research trajectories.
Thanks to the development of molecular approaches, recent advances have been made to characterise biological clocks and how they affect the physiology of bivalves. A further step has to be taken in order to incorporate the shell response in chronobiological studies. This gap is actually shorter than expected as both chronobiologists and sclerochronologists usually work on similar biological models (e.g., oysters, pectens, mussels). These species are found in a wide spatial repartition and live in different types of habitat (e.g., estuaries, lagoons, coastal marine habitats) which correspond to ideal conditions to study such integrative processes. Both in the field and in aquaria, experiments need to be conducted using different environmental settings, with parameters recorded or controlled, using combined approaches of gene expressions (i.e., the ones involved in clocks and biomineralisation) together with shell growth patterns analysis.
In addition to classical zeitgebers observed in chronobiology (i.e., photoperiod or temperature), food availability and/or composition could be another important environmental driver of the internal biological clock system, particularly in the control of the shell growth. Valve activity responds to the presence of the energy source of the bivalve (e.g., concentration in chlorophyll-a) as illustrated for A. islandica (Ballesta-Artero et al., 2017). In the absence of food, valves remain closed which could lead to pH decrease in the EPF leading to decalcification. The integration of this environmental cue by a clock is however still unclear. The biological clock is an evolutionary innovation that aims to adjust the rhythm of the organism to its environment in order to optimise its energetic balance. In other terms, it allows the organism to be ready to incorporate nutriments when available, and then use the energy when present. The effect of food availability on endogenous rhythms has been extensively studied on mice and is associated to the peripherical clock system (Escobar et al., 2009). Experiments on suprachiasmatic nucleus ablated rats shown that starved individuals continue to exhibit a circadian food-anticipatory activity (FAA) (Boulos and Terman, 1980; Mistlberger, 2009). The notion of FAA and food-entertainment are different but linked together. The importance of the influence of food as time giver on the FAA is still under discussion [see the review of Mistlberger (2009)]. In bivalves, a study on the intertidal filter-feeder clam Austrovenus stutchburyi suggests that the FAA is linked to a food-entertained oscillator (Williams and Pilditch, 1997). This possible role of food in shell growth rhythms should be considered in future studies using biological archives for (paleo)environmental reconstructions.
Biomineralisation should be seen as a dynamical process that punctually slows down or even stops. Biomineralisation activity throughout time can be followed directly using the analysis of changes in the composition of the extrapallial fluid (EPF) over time. This technique uses microsensors able to be placed in the extrapallial cavity or by suction of the EPF (Misogianes and Chasteen, 1979; Stemmer et al., 2019). Using this last methods, observations of Ca2+, dissolved inorganic carbon (DIC) and pH variations in the fluid suggest that calcification occurs by waves, which is consistent with the observation of increments in the shell (Stemmer et al., 2019). Therefore, this method can be used to visualise directly the effect of an environmental variable on the biomineralisation kinetics.The inorganic fraction of the shell is not the only one present in the EPF as organic compounds are key components in the biomineralisation process due to their role of backbone for the deposition of inorganic material (Marin and Luquet, 2004). Proteomic approaches can thus be used on EPF samples taken at regular time intervals. A part of organic components of shells are the direct output of the transcription and translation of genes involved in biomineralisation. Their transcription is not a random process and is the result of molecular pathways reacting to environmental cues. A better understanding of the molecular machinery behind biomineralisation (organic and inorganic compounds) and the reconstitution of the molecular pathways are essential to decipher how the process is activated or repressed (see review Clark, 2020). Molecular characterisation might indicate if there are pathways between the biomineralisation process and the biological clock machinery. Direct response of bivalve behaviour and shell growth to environmental variations can be monitored using a combined approach of valvometry and sclerochronology on the same specimens. This can be done even for short-term experiments as chemical staining such as calcein or manganese have been demonstrated to be rapidly incorporated and visible (< 30 min) during the shell biomineralisation (Langlet et al., 2006; Lartaud et al., 2010; Mahé et al., 2010).Importantly, the community has to keep in mind that periodicities observed in shells are not necessarily the same during the whole life of a bivalve, which can be at the origin of bias when inferring past and present environments from the study of shell growth patterns. Owen et al. (2002) reported changes of patterns throughout the year in scallops. One daily increment in the juvenile Pecten maximus shell is observed during summer and less than one increment per day in winter, when growth rate is slower. Missing increments has been reported for the young oysters M. gigas (<one year), forming less than one increment per day instead of bi-daily tidal-influenced growth periodicity for older specimens, while reaching high growth rates during this period (Huyghe et al., 2019). This is in opposition with usual observations. Interestingly, those authors also reported occasional arrhythmicity in juveniles part with the formation of up to five increments per day on the youngest part of the shell without any relationship with environmental parameters. As uncommon periodicities are often observed in juveniles, a better understanding of the sensory organs of bivalves and their ontogenesis might give some responses to the integration of environmental drivers throughout the organism life. The larval shell production might be more deeply investigated as this period of life is recognised as particularly sensitive to anthropogenic stressful conditions, such as ocean acidification and global warming (Gobler et al., 2014; Wang et al., 2017).
Conclusion
In conclusion, although widely used to reconstruct past environments and estimate the potential effect of climate change on shellfish production, the dynamic process of bivalves biomineralisation is still not fully understood and the origins of the periodicities preserved in shells remains unclear. In this review different research axes have been proposed. A better comprehension of the integration of the environment in bivalve shells, driven or not by biological clocks, is crucial in order to refine models notably used to derive growth rate from geochemical profiles in paleoclimatology. Also, the characterisation of ultradian microincrements observed within the daily or tidal increment, is promising to improve the monitoring of bivalve physiological response to its environment and/or propose a temporal resolution never reached for past environmental studies. Bivalve are known to have a high ecological value as they are recognised as carbon sink and engineering species, forming refuges for biodiversity, their reaction to climate change will have a huge impact on many other species. A better understanding of the relation between the bivalve and its environment will additionally help to forecast the effect of climate changes on those communities.
Author Contributions
This review is the product of the PhD thesis of VL. following completion of research supervised by FL and LB. VL wrote the original draft. FL and LB edited and revised the manuscript. VL produced the artwork within this article and FL and LB revised it. All authors contributed to the article and approved the submitted version.
Funding
This project has received the financial support from the CNRS through the 80|Prime - MITI interdisciplinary program “TEMPO” and the MITI interdisciplinary program “ARCHIVE”.
Conflict of Interest
The authors declare that the research was conducted in the absence of any commercial or financial relationships that could be construed as a potential conflict of interest.
Publisher’s Note
All claims expressed in this article are solely those of the authors and do not necessarily represent those of their affiliated organizations, or those of the publisher, the editors and the reviewers. Any product that may be evaluated in this article, or claim that may be made by its manufacturer, is not guaranteed or endorsed by the publisher.
Acknowledgments
Authors are grateful to Mélanie Dussenne for checking the spelling.
References
Allemand D., Tambutté É., Zoccola D., Tambutté S. (2011). “Coral Calcification, Cells to Reefs,” in Coral Reefs: An Ecosystem in Transition (Dordrecht: Springer), 119–150. doi: 10.1007/978-94-007-0114-4_9
Alves M. G., Oliveira P. F. (2013). Effects of non-Steroidal Estrogen Diethylstilbestrol on pH and Ion Transport in the Mantle Epithelium of a Bivalve Anodonta Cygnea. Ecotoxicol. Environ. Saf. 97, 230–235. doi: 10.1016/j.ecoenv.2013.07.024
Andrade H., Massabuau J.-C., Cochrane S., Ciret P., Tran D., Sow M., et al. (2016). High Frequency Non-Invasive (HFNI) Bio-Sensors As a Potential Tool for Marine Monitoring and Assessments. Front. Mar. Sci. 3. doi: 10.3389/FMARS.2016.00187
Andrisoa A., Lartaud F., Rodellas V., Neveu I. (2019). Enhanced Growth Rates of the Mediterranean Mussel in a Coastal Lagoon Driven by Groundwater Inflow. Front. Mar. Sci. 6. doi: 10.3389/fmars.2019.00753
Anestis A., Lazou A., Pörtner H. O., Michaelidis B. (2007). Behavioral, Metabolic, and Molecular Stress Responses of Marine Bivalve Mytilus Galloprovincialis During Long-Term Acclimation at Increasing Ambient Temperature. Am. J. Physiol. - Regul. Integr. Comp. Physiol. 293, 911–921. doi: 10.1152/ajpregu.00124.2007
Ansell A. D. (1968). The Rate of Growth of the Hard Clam Mercenaria Mercenaria (L) Throughout the Geographical Range. ICES. J. Mar. Sci. 31, 364–409. doi: 10.1093/icesjms/31.3.364
Aschoff J. (1981). “Freerunning and Entrained Circadian Rhythms,” in Biological Rhythms ( Boston, MA:Springer US), 81–93. doi: 10.1007/978-1-4615-6552-9_6
Ballesta-Artero I., Witbaard R., Carroll M. L., van der Meer J. (2017). Environmental Factors Regulating Gaping Activity of the Bivalve Arctica Islandica in Northern Norway. Mar. Biol. 164, 116. doi: 10.1007/s00227-017-3144-7
Banni M., Negri A., Mignone F., Boussetta H., Viarengo A., Dondero F. (2011). Gene Expression Rhythms in the Mussel Mytilus Galloprovincialis (Lam.) Across an Annual Cycle. PloS One 6, e18904. doi: 10.1371/journal.pone.0018904
Bartness T. J., Albers H. E. (2000). “Activity Patterns and the Biological Clock in Mammals,” in Activity Patterns in Small Mammals (Berlin, Heidelberg: Springer), 23–47. doi: 10.1007/978-3-642-18264-8_3
Beckvar N. (1981). Cultivation, Spawning, and Growth of the Giant Clams Tridacna Gigas, T. Derasa, and T. Squamosa in Palau, Caroline Islands. Aquaculture 24, 21–30. doi: 10.1016/0044-8486(81)90040-5
Boo M. V., Hiong K. C., Choo C. Y. L., Cao-Pham A. H., Wong W. P., Chew S. F., et al. (2017). The Inner Mantle of the Giant Clam, Tridacna Squamosa, Expresses a Basolateral Na+/K+-ATPase α-Subunit, Which Displays Light-Dependent Gene and Protein Expression Along the Shell-Facing Epithelium. PloS One 12, e0186865. doi: 10.1371/journal.pone.0186865
Boulos Z., Terman M. (1980). Food Availability and Daily Biological Rhythms. Neurosci. Biobehav. Rev. 4, 119–131. doi: 10.1016/0149-7634(80)90010-X
Brahmi C., Kopp C., Domart-Coulon I., Stolarski J., Meibom A. (2012). Skeletal Growth Dynamics Linked to Trace-Element Composition in the Scleractinian Coral Pocillopora Damicornis. Geochim. Cosmochim. Acta 99, 146–158. doi: 10.1016/j.gca.2012.09.031
Butler P. G., Schöne B. R. (2017). New Research in the Methods and Applications of Sclerochronology. Palaeogeogr. Palaeoclimatol. Palaeoecol. 465, 295–299. doi: 10.1016/J.PALAEO.2016.11.013
Cao-Pham A. H., Hiong K. C., Boo M. V., Choo C. Y. L., Wong W. P., Chew S. F., et al. (2019). Calcium Absorption in the Fluted Giant Clam, Tridacna Squamosa, may Involve a Homolog of Voltage-Gated Calcium Channel Subunit α1 (CACNA1) That has an Apical Localization and Displays Light-Enhanced Protein Expression in the Ctenidium. J. Comp. Physiol. B. Biochem. Syst. Environ. Physiol. 189, 693–706. doi: 10.1007/s00360-019-01238-4
Carré M., Bentaleb I., Bruguier O., Ordinola E., Barrett N. T., Fontugne M. (2006). Calcification Rate Influence on Trace Element Concentrations in Aragonitic Bivalve Shells: Evidences and Mechanisms. Geochim. Cosmochim. Acta 70, 4906–4920. doi: 10.1016/j.gca.2006.07.019
Chapman E. C., Bonsor B. J., Parsons D. R., Rotchell J. M. (2020). Influence of Light and Temperature Cycles on the Expression of Circadian Clock Genes in the Mussel Mytilus Edulis. Mar. Environ. Res. 159, 104960. doi: 10.1016/j.marenvres.2020.104960
Checa A. G. (2018). Physical and Biological Determinants of the Fabrication of Molluscan Shell Microstructures. Front. Mar. Sci. 5. doi: 10.3389/fmars.2018.00353
Checa A. G., Esteban-Delgado F. J., Rodríguez-Navarro A. B. (2007). Crystallographic Structure of the Foliated Calcite of Bivalves. J. Struct. Biol. 157, 393–402. doi: 10.1016/j.jsb.2006.09.005
Checa A. G., Macías-Sánchez E., Harper E. M., Cartwright J. H. E. (2016). Organic Membranes Determine the Pattern of the Columnar Prismatic Layer of Mollusc Shells. Proc. R. Soc B. Biol. Sci. 283, 20160032. doi: 10.1098/rspb.2016.0032
Checa A. G., Okamoto T., Ramírez J. (2006). Organization Pattern of Nacre in Pteriidae (Bivalvia: Mollusca) Explained by Crystal Competition. Proc. R. Soc B. Biol. Sci. 273, 1329–1337. doi: 10.1098/rspb.2005.3460
Checa A. G., Rodríguez-Navarro A. B., Esteban-Delgado F. J. (2005). The Nature and Formation of Calcitic Columnar Prismatic Shell Layers in Pteriomorphian Bivalves. Biomaterials 26, 6404–6414. doi: 10.1016/j.biomaterials.2005.04.016
Chew S. F., Koh C. Z. Y., Hiong K. C., Choo C. Y. L., Wong W. P., Neo M. L., et al. (2019). Light-Enhanced Expression of Carbonic Anhydrase 4-Like Supports Shell Formation in the Fluted Giant Clam Tridacna Squamosa. Gene 683, 101–112. doi: 10.1016/j.gene.2018.10.023
Clark G. R. (2005). Daily Growth Lines in Some Living Pectens (Mollusca: Bivalvia), and Some Applications in a Fossil Relative: Time and Tide Will Tell. Palaeogeogr. Palaeoclimatol. Palaeoecol. 228, 26–42. doi: 10.1016/j.palaeo.2005.03.044
Clark M. S. (2020). Molecular Mechanisms of Biomineralization in Marine Invertebrates. J. Exp. Biol. 223, jeb206961. doi: 10.1242/jeb.206961
Coen L. D., Brumbaugh R. D., Bushek D., Grizzle R., Luckenbach M. W., Posey M. H., et al. (2007). Ecosystem Services Related to Oyster Restoration. Mar. Ecol. Prog. Ser. 341, 303–307. doi: 10.3354/meps341303
Coimbra J., Machado J., Fernandes P. L., Ferreira H. G., Ferreira K. G. (1988). Electrophysiology of the Mantle of Anodonta Cygnea. J. Exp. Biol. 140, 65–88. doi: 10.1242/JEB.140.1.65
Comeau L. A., Babarro J. M. F., Longa A., Padin X. A. (2018). Valve-Gaping Behavior of Raft-Cultivated Mussels in the Ría De Arousa, Spain. Aquac. Rep. 9, 68–73. doi: 10.1016/J.AQREP.2017.12.005
Connor K. M., Gracey A. Y. (2011). Circadian Cycles are the Dominant Transcriptional Rhythm in the Intertidal Mussel Mytilus Californianus. Pnas 108, 16110–16115. doi: 10.1073/pnas.1111076108
Crenshaw A. (1972). The Inorganic Composition of Molluscan Extrapallial Fluid. Biol. Bull. 143, 506–512. doi: 10.2307/1540180
Crone T. J., Wilcock W. S. D. (2005). Modeling the Effects of Tidal Loading on Mid-Ocean Ridge Hydrothermal Systems. Geochem. Geophys. Geosys. 6, Q07001. doi: 10.1029/2004GC000905
Dauphin Y., Cuif J. P., Doucet J., Salomé M., Susini J., Williams C. T. (2003). In Situ Mapping of Growth Lines in the Calcitic Prismatic Layers of Mollusc Shells Using X-Ray Absorption Near-Edge Structure (XANES) Spectroscopy at the Sulphur K-Edge. Mar. Biol. 142, 299–304. doi: 10.1007/s00227-002-0950-2
de Winter N. J., Goderis S., Van Malderen S. J. M., Sinnesael M., Vansteenberge S., Snoeck C., et al. (2020). Subdaily-Scale Chemical Variability in a Torreites Sanchezi Rudist Shell: Implications for Rudist Paleobiology and the Cretaceous Day-Night Cycle. Paleoceanogr. Paleoclimatology 35, e2019PA003723. doi: 10.1029/2019PA003723
Ditty J. L., Williams S. B., Golden S. S. (2003). A Cyanobacterial Circadian Timing Mechanism. Annu. Rev. Genet. 37, 513–543. doi: 10.1146/annurev.genet.37.110801.142716
Dodd J. R. (1965). Environmental Control of Strontium and Magnesium in Mytilus. Geochim. Cosmochim. Acta 29, 385–398. doi: 10.1016/0016-7037(65)90035-9
Dunlap J. C. (1999). Molecular Bases for Circadian Clocks. Cell 96, 271–290. doi: 10.1016/s0092-8674(00)80566-8
Duperron S. (2010). “The Diversity of Deep-Sea Mussels and Their Bacterial Symbioses,” in The Vent and Seep Biota - Aspect From Microbes to Ecosystems (Dordrecht: Springer), 137–167. doi: 10.1007/978-90-481-9572-5_6
Dupraz C., Reid R. P., Braissant O., Decho A. W., Norman R. S., Visscher P. T. (2009). Processes of Carbonate Precipitation in Modern Microbial Mats. Earth-Science. Rev. 96, 141–162. doi: 10.1016/j.earscirev.2008.10.005
Enright J. T. (1976). Plasticity in an Isopod’s Clockworks: Shaking Shapes Form and Affects Phase and Frequency. J. Comp. Physiol. 107, 13–37. doi: 10.1007/BF00663916
Epstein S., Buchsbaum R., Lowenstam H. A., Urey H. C. (1953). Revised Carbonate-Water Isotopic Temperature Scale. Geol. Soc Am. Bull. 64, 1315–1326. doi: 10.1130/0016-7606(1953)64[1315:RCITS]2.0.CO;2
Escobar C., Cailotto C., Angeles-Castellanos M., Delgado R. S., Buijs R. M. (2009). Peripheral Oscillators: The Driving Force for Food-Anticipatory Activity. Eur. J. Neurosci. 30, 1665–1675. doi: 10.1111/j.1460-9568.2009.06972.x
Figueroa F. L., Niell F. X., Figueiras F. G., Villarino M. L. (1998). Diel Migration of Phytoplankton and Spectral Light Field in the Ría De Vigo (NW Spain). Mar. Biol. 130, 491–499. doi: 10.1007/s002270050269
Gannon M. E., Pérez-Huerta A., Aharon P., Street S. C. (2017). A Biomineralization Study of the Indo-Pacific Giant Clam Tridacna Gigas. Coral. Reefs. 36, 503–517. doi: 10.1007/s00338-016-1538-5
Gao J., Chen Y., Yang Y., Liang J., Xie J., Liu J., et al. (2016). The Transcription Factor Pf-POU3F4 Regulates Expression of the Matrix Protein Genes Aspein and Prismalin-14 in Pearl Oyster ( Pinctada Fucata ). FEBS J. 283, 1962–1978. doi: 10.1111/febs.13716
García-March J. R., Sanchís Solsona M.Á., García-Carrascosa A. M. (2008). Shell Gaping Behaviour of Pinna Nobilis L. 1758: Circadian and Circalunar Rhythms Revealed by in Situ Monitoring. Mar. Biol. 153, 689–698. doi: 10.1007/s00227-007-0842-6
Ghenim L., Allier C., Obeid P., Hervé L., Fortin J. Y., Balakirev M., et al. (2021). A New Ultradian Rhythm in Mammalian Cell Dry Mass Observed by Holography. Sci. Rep. 2021. 11, 1290. doi: 10.1038/s41598-020-79661-9
Giuffre A. J., Hamm L. M., Han N., De Yoreo J. J., Dove P. M. (2013). Polysaccharide Chemistry Regulates Kinetics of Calcite Nucleation Through Competition of Interfacial Energies. Proc. Natl. Acad. Sci. U. S. A. 110, 9261–9266. doi: 10.1073/pnas.1222162110
Gobler C. J., DePasquale E. L., Griffith A. W., Baumann H. (2014). Hypoxia and Acidification Have Additive and Synergistic Negative Effects on the Growth, Survival, and Metamorphosis of Early Life Stage Bivalves. PloS One 9, e83648. doi: 10.1371/JOURNAL.PONE.0083648
Hallmann N., Burchell M., Schöne B. R., Irvine G. V., Maxwell D. (2009). High-Resolution Sclerochronological Analysis of the Bivalve Mollusk Saxidomus Gigantea From Alaska and British Columbia: Techniques for Revealing Environmental Archives and Archaeological Seasonality. J. Archaeol. Sci. 36, 2353–2364. doi: 10.1016/j.jas.2009.06.018
Hartmann J. T., Beggel S., Auerswald K., Geist J. (2016). Determination of the Most Suitable Adhesive for Tagging Freshwater Mussels and its Use in an Experimental Study of Filtration Behaviour and Biological Rhythm. J. Molluscan. Stud. 82, 415–421. doi: 10.1093/mollus/eyw003
Hawkins A. J. S., Klumpp D. W. (1995). Nutrition of the Giant Clam Tridacna Gigas (L.). II. Relative Contributions of Filter-Feeding and the Ammonium-Nitrogen Acquired and Recycled by Symbiotic Alga Towards Total Nitrogen Requirements for Tissue Growth and Metabolism. J. Exp. Mar. Bio. Ecol. 190, 263–290. doi: 10.1016/0022-0981(95)00044-R
Hiong K. C., Cao-Pham A. H., Choo C. Y. L., Boo M. V., Wong W. P., Chew S. F., et al. (2017). Light-Dependent Expression of a Na + /H + Exchanger 3-Like Transporter in the Ctenidium of the Giant Clam, Tridacna Squamosa, can be Related to Increased H + Excretion During Light-Enhanced Calcification. Physiol. Rep. 5, e13209. doi: 10.14814/phy2.13209
Huyghe D., Rafelis M., Ropert M., Mouchi V., Emmanuel L., Renard M. (2019). New Insights Into Oyster High − Resolution Hinge Growth Patterns. Mar. Biol. 166, 48. doi: 10.1007/s00227-019-3496-2
Ip Y. K., Ching B., Hiong K. C., Choo C. Y. L., Boo M. V., Wong W. P., et al. (2015). Light Induces Changes in Activities of Na+/K+-ATPase, H+/K+-ATPase and Glutamine Synthetase in Tissues Involved Directly or Indirectly in Light-Enhanced Calcification in the Giant Clam, Tridacna Squamosa. Front. Physiol. 6. doi: 10.3389/fphys.2015.00068
Ip Y. K., Hiong K. C., Lim L. J. Y., Choo C. Y. L., Boo M. V., Wong W. P., et al. (2018). Molecular Characterization, Light-Dependent Expression, and Cellular Localization of a Host Vacuolar-Type H+-ATPase (VHA) Subunit A in the Giant Clam, Tridacna Squamosa, Indicate the Involvement of the Host VHA in the Uptake of Inorganic Carbon and. Gene 659, 137–148. doi: 10.1016/j.gene.2018.03.054
Ip Y. K., Koh C. Z. Y., Hiong K. C., Choo C. Y. L., Boo M. V., Wong W. P., et al. (2017). Carbonic Anhydrase 2-Like in the Giant Clam, Tridacna Squamosa : Characterization, Localization, Response to Light, and Possible Role in the Transport of Inorganic Carbon From the Host to its Symbionts. Physiol. Rep. 5, e13494. doi: 10.14814/phy2.13494
Jones D. S. (1980). Annual Cycle of Shell Growth Increment Formation in Two Continental Shelf Bivalves and its Paleoecologic Significance. Paleobiology 3, 331–340. doi: 10.1017/S0094837300006837
Karney G. B., Butler P. G., Speller S., Scourse J. D., Richardson C. A., Schrder M., et al. (2012). Characterizing the Microstructure of Arctica Islandica Shells Using NanoSIMS and EBSD. Geochem. Geophys. Geosys. 13, Q04002. doi: 10.1029/2011GC003961
Kennish M. J., Olsson R. K. (1975). Effects of Thermal Discharges on the Microstructural Growth of Mercenaria Mercenaria. Environ. Geol. 1, 41–64. doi: 10.1007/BF02426940
Killam D. E., Clapham M. E. (2018). Identifying the Ticks of Bivalve Shell Clocks: Seasonal Growth in Relation to Temperature and Food Supply. Palaios 33, 228–236. doi: 10.2110/palo.2017.072
Klumpp D. W., Griffiths C. L. (1994). Contributions of Phototrophic and Heterotrophic Nutrition to the Metabolic and Growth Requirements of Four Species of Giant Clam (Tridacnidae. Mar. Ecol. Prog. Ser.)115, 103–115. doi: 10.3354/meps115103
Langlet D., Alunno-Bruscia M., Rafélis M., Renard M., Roux M., Schein E., et al. (2006). Experimental and Natural Cathodoluminescence in the Shell of Crassostrea Gigas From Thau Lagoon (France): Ecological and Environmental Implications. Mar. Ecol. Prog. Ser. 317, 143–156. doi: 10.3354/meps317143
Lartaud F., Chauvaud L., Richard J., Toulot A., Bollinger C., Testut L., et al. (2010). Experimental Growth Pattern Calibration of Antarctic Scallop Shells (Adamussium Colbecki, Smith 1902) to Provide a Biogenic Archive of High-Resolution Records of Environmental and Climatic Changes. J. Exp. Mar. Bio. Ecol. 393, 158–167. doi: 10.1016/j.jembe.2010.07.016
Li S., Liu Y., Huang J., Zhan A., Xie L., Zhang R. (2017). The Receptor Genes PfBMPR1B and PfBAMBI are Involved in Regulating Shell Biomineralization in the Pearl Oyster Pinctada Fucata. Sci. Rep. 7, 9219. doi: 10.1038/s41598-017-10011-y
Littlewood D. T. J., Young R. E. (1994). The Effect of Air-Gaping Behaviour on Extrapallial Fluid pH in the Tropical Oyster Crassostrea Rhizophorae. Comp. Biochem. Physiol. – Part A Physiol. 107, 1–6. doi: 10.1016/0300-9629(94)90264-X
L, loyd D., Murray D. B. (2005). Ultradian Metronome: Timekeeper for Orchestration of Cellular Coherence. Trends Biochem. Sci. 30, 373–377. doi: 10.1016/j.tibs.2005.05.005
Lutz R. A., Rhoads D. C. (1977). Anaerobiosis and a Theory of Growth Line Formation. Sci. (80-. ). 198, 1222–1227. doi: 10.1126/science.198.4323.1222
Mahé K., Bellamy E., Lartaud F., Rafélis M. (2010). Calcein and Manganese Experiments for Marking the Shell of the Common Cockle ( Cerastoderma Edule ): Tidal Rhythm Validation of Increments Formation. Aquatic Living Res. 245, 239–245. doi: 10.1051/alr/2010025
Marin F. (2020). Mollusc Shellomes: Past, Present and Future. J. Struct. Biol. 212, 107583. doi: 10.1016/j.jsb.2020.107583
Marin F., Luquet G. (2004). Molluscan Shell Proteins. Comptes. Rendus. - Palevol. 3, 469–492. doi: 10.1016/j.crpv.2004.07.009
Marin F., Luquet G., Marie B., Medakovic D. (2007). Molluscan Shell Proteins: Primary Structure, Origin, and Evolution. Curr. Top. Dev. Biol. 80, 209–276. doi: 10.1016/S0070-2153(07)80006-8
Marin F., Roy N., Marie B. (2012). The Formation and Mineralization of Mollusk. Front. Biosci. 4,1099–1125. doi: 10.2741/S321
Mat A. M., Massabuau J. C., Ciret P., Tran D. (2012). Evidence for a Plastic Dual Circadian Rhythm in the Oyster Crassostrea Gigas. Chronobiol. Int. 29, 857–867. doi: 10.3109/07420528.2012.699126
Mat A. M., Sarrazin J., Markov G. V., Apremont V., Dubreuil C., Eché C., et al. (2020). Biological Rhythms in the Deep-Sea Hydrothermal Mussel Bathymodiolus Azoricus. Nat. Commun. 11, 3454. doi: 10.1038/s41467-020-17284-4
Miglioli A., Dumollard R., Balbi T., Besnardeau L., Canesi L. (2019). Characterization of the Main Steps in First Shell Formation in Mytilus Galloprovincialis: Possible Role of Tyrosinase. Proc. R. Soc B. Biol. Sci. 286, 20192043. doi: 10.1098/rspb.2019.2043
Misogianes M. J., Chasteen N. D. (1979). A Chemical and Spectral Characterization of the Extrapallial Fluid of Mytilus Edulis. Anal. Biochem. 100, 324–334. doi: 10.1016/0003-2697(79)90236-7
Mistlberger R. E. (2009). Food-Anticipatory Circadian Rhythms: Concepts and Methods. Eur. J. Neurosci. 30, 1718–1729. doi: 10.1111/j.1460-9568.2009.06965.x
Miyazaki Y., Usui T., Kajikawa A., Hishiyama H., Matsuzawa N., Nishida T., et al. (2008). Daily Oscillation of Gene Expression Associated With Nacreous Layer Formation. Front. Mater. Sci. China 2, 162–166. doi: 10.1007/s11706-008-0027-3
Moberg F., Folke C. (1999). Ecological Goods and Services of Coral Reef Ecosystems. Ecol. Econ. 29, 215–233. doi: 10.1016/S0921-8009(99)00009-9
Murdock D. J. E. (2020). The ‘Biomineralization Toolkit’ and the Origin of Animal Skeletons. Biol. Rev. 95, 1372–1392. doi: 10.1111/brv.12614
Nagai K., Honjo T., Go J., Yamashita H., Oh S. J. (2006). Detecting the Shellfish Killer Heterocapsa Circularisquama (Dinophyceae) by Measuring Bivalve Valve Activity With a Hall Element Sensor. Aquaculture 255, 395–401. doi: 10.1016/j.aquaculture.2005.12.018
Naylor E. (1958). Tidal and Diurnal Rhythms of Locomotory Activity in Carcinus Maenas (L.). J. Exp. Biol. 35, 602–610. doi: 10.1242/jeb.35.3.602
Nedoncelle K., Lartaud F., de Rafelis M., Boulila S., Le Bris N. (2013). A New Method for High-Resolution Bivalve Growth Rate Studies in Hydrothermal Environments. Mar. Biol. 160, 1427–1439. doi: 10.1007/s00227-013-2195-7
Nedoncelle K., Lartaud F., Pereira L. C., Yücel M., Thurnherr A. M., Mullineaux L., et al. (2015). Deep-Sea Research I Bathymodiolus Growth Dynamics in Relation to Environmental Fluctuations in Vent Habitats. Deep. Res. Part I 106, 183–193. doi: 10.1016/j.dsr.2015.10.003
Nedoncelle K., Le Bris N., de Rafélis M., Labourdette N., Lartaud F. (2014). Non-Equilibrium Fractionation of Stable Carbon Isotopes in Chemosynthetic Mussels. Chem. Geol. 387, 35–46. doi: 10.1016/J.CHEMGEO.2014.08.002
Ono D., Honma K. I., Honma S. (2015). Circadian and Ultradian Rhythms of Clock Gene Expression in the Suprachiasmatic Nucleus of Freely Moving Mice. Sci. Rep. 5, 12310. doi: 10.1038/srep12310
Ono R., Koike N., Inokawa H., Tsuchiya Y., Umemura Y., Yamamoto T., et al. (2019). Incremental Growth Lines in Mouse Molar Dentin Represent 8-Hr Ultradian Rhythm. Acta Histochem. Cytochem. 52, 93–99. doi: 10.1267/AHC.19017
Owen R., Richardson C. A., Kennedy H. (2002). The Influence of Shell Growth Rate on Striae Deposition in the Scallop Pecten Maximus. J. Mar. Biol. Assoc. Unite. Kingdom. 82, 621–623. doi: 10.1017/S0025315402005969
Pairett A. N., Serb J. M. (2013). De Novo Assembly and Characterization of Two Transcriptomes Reveal Multiple Light-Mediated Functions in the Scallop Eye (Bivalvia: Pectinidae). PloS One 8, e69852. doi: 10.1371/JOURNAL.PONE.0069852
Palmer J. D., Williams B. G. (1986). Comparative Studies of Tidal Rhythms. II. The Dual Clock Control of the Locomotor Rhythms of Two Decapod Crustaceans. Mar. Behav. Physiol. 12, 269–278. doi: 10.1080/10236248609378653
Pannella G., MacClintock C. (1968). Biological and Environmental Rhythms Reflected in Molluscan Shell Growth. J. Paleontol. 2, 64–80. doi: 10.1017/S0022336000061655
Partch C. L., Green C. B., Takahashi J. S. (2014). Molecular Architecture of the Mammalian Circadian Clock. Trends Cell Biol. 24, 90–99. doi: 10.1016/J.TCB.2013.07.002
Peharda M., Schöne B. R., Black B. A., Corrège T. (2021). Advances of Sclerochronology Research in the Last Decade. Paleoceanogr. Paleoclimatology,Paleoecol. 570, 110371. doi: 10.1016/j.palaeo.2021.110371
Perrigault M., Andrade H., Bellec L., Ballantine C., Camus L., Tran D. (2020). Rhythms During the Polar Night: Evidence of Clock-Gene Oscillations in the Arctic Scallop Chlamys Islandica. Proc. Biol. Sci. 287, 20201001. doi: 10.1098/rspb.2020.1001
Perrigault M., Tran D. (2017). Identification of the Molecular Clockwork of the Oyster Crassostrea Gigas. PloS One 12, e0169790. doi: 10.1371/journal.pone.0169790
Poitevin P., Chauvaud L., Pécheyran C., Lazure P., Jolivet A., Thébault J. (2020). Does Trace Element Composition of Bivalve Shells Record Utra-High Frequency Environmental Variations? Mar. Environ. Res. 158, 104943. doi: 10.1016/j.marenvres.2020.104943
Poulain C., Lorrain A., Amice E., Morize E., Paulet Y. (2011). An Environmentally Induced Tidal Periodicity of Microgrowth Increment Formation in Subtidal Populations of the Clam Ruditapes Philippinarum. J. Exp. Mar. Bio. Ecol. 397, 58–64. doi: 10.1016/j.jembe.2010.11.001
Réaumur R.-A. (1709). De la formation et de l’accroissement des coquilles des animaux tant terrestres qu’aquatiques, soit de mer, soit de rivière. Hist. Acad. Roy, Sci. Mem. Paris, 364–400.
Rensing L., Ruoff P. (2002). Temperature Effect on Entrainment, Phase Shifting, and Amplitude of Circadian Clocks and its Molecular Bases. Chronobiol. Int. 19, 807–864. doi: 10.1081/CBI-120014569
Richardson C. A. (1987). Microgrowth Patterns in the Shell of the Malaysian Cockle Anadara Granosa (L.) and Their Use in Age Determination. J. Exp. Mar. Bio. Ecol. 111, 77–98. doi: 10.1016/0022-0981(87)90021-9
Richardson C. A. (1988). Exogenous and Endogenous Rhythms of Band Formation in the Shell of the Clam Tapes Philippinarum (Adams Et Reeve 1850). J. Exp. Mar. Bio. Ecol. 122, 105–126. doi: 10.1016/0022-0981(88)90179-7
Richardson C. A. (1989). An Analysis of the Microgrowth Bands in the Shell of the Common Mussel Mytilus Edulis. J. Mar. Biol. Assoc. Unite. Kingdom. 69, 477–491. doi: 10.1017/S0025315400029544
Richardson C. A., Crisp D. J., Runham N. W. (1979). Tidally Deposited Growth Bands in the Shell of the Common Cockle, Cerastoderma Edule. Malacologia 18, 277–290.
Richardson C. A., Crisp D. J., Runham N. W. (1980). An Endogenous Rhythm in Shell Deposition in Cerastoderma Edule. J. Mar. Biol. Assoc. Unite. Kingdom. 60, 991–1004. doi: 10.1017/S0025315400042041
Richardson C. A., Runham N. W., Crisp D. J. (1981). A Histological and Ultrastructural Study of the Cells of the Mantle Edge of a Marine Bivalve, Cerastoderma Edule. Tissue Cell 13, 715–730. doi: 10.1016/S0040-8166(81)80008-0
Richardson C. A., Seed R., Naylor E. (1990). Use of Internal Growth Bands for Measuring Individual and Population Growth Rates in Mytilus Edulis From Offshore Production Platforms. Mar. Ecol. Prog. Ser. 66, 259–265. doi: 10.3354/meps066259
Rodland D. L., Schöne B. R., Helama S., Nielsen J. K., Baier S. (2006). A Clockwork Mollusc : Ultradian Rhythms in Bivalve Activity Revealed by Digital Photography . J. Exp. Biol. Ecol. 334, 316–323. doi: 10.1016/j.jembe.2006.02.012
Rodríguez-Tovar F. J. (2014). Orbital Climate Cycles in the Fossil Record: From Semidiurnal to Million-Year Biotic Responses. Annu. Rev. Earth Planet. Sci. 42, 69–102. doi: 10.1146/annurev-earth-120412-145922
Rosbash M. (2009). The Implications of Multiple Circadian Clock Origins. PloS Biol. 7, e1000062. doi: 10.1371/journal.pbio.1000062
Scheirer D. S., Shank T. M., Fornari D. J. (2006). Temperature Variations at Diffuse and Focused Flow Hydrothermal Vent Sites Along the Northern East Pacific Rise. Geochem. Geophys. Geosys. 7, Q03002. doi: 10.1029/2005GC001094
Schöne B. R. (2008). The Curse of Physiology — Challenges and Opportunities in the Interpretation of Geochemical Data From Mollusk Shells. Geo-Mar. Lett. 28, 269–285. doi: 10.1007/s00367-008-0114-6
Schöne B. R. (2013). Arctica Islandica (Bivalvia): A Unique Paleoenvironmental Archive of the Northern North Atlantic Ocean. Glob. Planet. Change 111, 199–225. doi: 10.1016/j.gloplacha.2013.09.013
Schöne B. R., Giere O. (2005). Growth Increments and Stable Isotope Variation in Shells of the Deep-Sea Hydrothermal Vent Bivalve Mollusk Bathymodiolus Brevior From the North Fiji Basin, Pacific Ocean. Deep. Res. Part I Oceanogr. Res. Pap. 52, 1896–1910. doi: 10.1016/j.dsr.2005.06.003
Schöne B. R., Krause R. A. (2016). Retrospective Environmental Biomonitoring – Mussel Watch Expanded. Glob. Planet. Change 144, 228–251. doi: 10.1016/j.gloplacha.2016.08.002
Schöne B. R., Lega J., Flessa K. W., Goodwin D. H., Dettman D. L. (2002). Reconstructing Daily Temperatures From Growth Rates of the Intertidal Bivalve Mollusk Chione Cortezi (Northern Gulf of California, Mexico). Palaeogeogr. Palaeoclimatol. Palaeoecol. 184, 131–146. doi: 10.1016/S0031-0182(02)00252-3
Schöne B. R., Oschmann W., Rössler J., Freyre Castro A. D., Houk S. D., Kröncke I., et al. (2003a). North Atlantic Oscillation Dynamics Recorded in Shells of a Long-Lived Bivalve Mollusk. Geology 31, 1037–1040. doi: 10.1130/G20013.1
Schöne B. R., Page N. A., Rodland D. L., Fiebig J., Baier S., Helama S. O., et al. (2007). ENSO-Coupled Precipitation Records, (1959-2004) Based on Shells of Freshwater Bivalve Mollusks (Margaritifera Falcata) From British Columbia. Int. J. Earth Sci. 96, 525–540. doi: 10.1007/s00531-006-0109-3
Schöne B. R., Pfeiffer M., Pohlmann T., Siegismund F. (2005). A Seasonally Resolved Bottom-Water Temperature Record for the Period AD 1866-2002 Based on Shells of Arctica Islandica (Mollusca, North Sea). Int. J. Climatol. 25, 947–962. doi: 10.1002/joc.1174
Schöne B. R., Tanabe K., Dettman D., Sato S. (2003b). Environmental Controls on Shell Growth Rates and D 18 O of the Shallow-Marine Bivalve Mollusk Phacosoma Japonicum in Japan. Mar. Biol. 142, 473–485. doi: 10.1007/s00227-002-0970-y
Schwartzmann C., Durrieu G., Sow M., Ciret P., Lazareth C. E., Massabuaua J. C. (2011). In Situ Giant Clam Growth Rate Behavior in Relation to Temperature: A One-Year Coupled Study of High-Frequency Noninvasive Valvometry and Sclerochronology. Limnol. Oceanogr. 56, 1940–1951. doi: 10.4319/lo.2011.56.5.1940
Skinner H. C., Jahren A. H. (2003). “Biomineralization,” in Trearise on Geochemistry ( Elsevier Ltd), 117–184. doi: 10.1016/B0-08-043751-6/08128-7
Sleight V. A., Antczak P., Falciani F., Clark M. S., Cowen L. (2020). Computationally Predicted Gene Regulatory Networks in Molluscan Biomineralization Identify Extracellular Matrix Production and Ion Transportation Pathways. Bioinformatics 36, 1326–1332. doi: 10.1093/bioinformatics/btz754
Steinhardt J., Butler P. G., Carroll M. L., Hartley J. (2016). The Application of Long-Lived Bivalve Sclerochronology in Environmental Baseline Monitoring. Front. Mar. Sci. 3. doi: 10.3389/fmars.2016.00176
Stemmer K., Brey T., Gutbrod M. S. (2019). In Situ Measurements of Ph, CA2+, and Dic Dynamics Within the Extrapallial Fluid of the Ocean Quahog Arctica Islandica. J. Shellfish. Res. 38, 71–78. doi: 10.2983/035.038.0107
Sun J., Xu G., Wang Z., Li Q., Cui Y., Xie L., et al. (2015). The Effect of NF-κb Signalling Pathway on Expression and Regulation of Nacrein in Pearl Oyster, Pinctada Fucata. PloS One 10, e0131711. doi: 10.1371/journal.pone.0131711
Sun X. J., Zhou L. Q., Tian J. T., Liu Z. H., Wu B., Dong Y. H., et al. (2016). Transcriptome Survey of Phototransduction and Clock Genes in Marine Bivalves. Genet. Mol. Res. 15, gmr15048726. doi: 10.4238/gmr15048726
Tan Y., Merrow M., Roenneberg T. (2004). Photoperiodism in Neurospora Crassa. J. Biol. Rhythm. 19, 135–143. doi: 10.1177/0748730404263015
Thébault J., Chauvaud L., Clavier J., Fichez R., Morize E. (2006). Evidence of a 2-Day Periodicity of Striae Formation in the Tropical Scallop Comptopallium Radula Using Calcein Marking. Mar. Biol. 149, 257–267. doi: 10.1007/s00227-005-0198-8
Tong H., Hu J., Ma W., Zhong G., Yao S., Cao N. (2002). In Situ Analysis of the Organic Framework in the Prismatic Layer of Mollusc Shell. Biomaterials 23, 2593–2598. doi: 10.1016/S0142-9612(01)00397-0
Tran D., Nadau A., Durrieu G., Ciret P., Parisot J. P., Massabuau J. C. (2011). Field Chronobiology of a Molluscan Bivalve: How the Moon and Sun Cycles Interact to Drive Oyster Activity Rhythms. Chronobiol. Int. 28, 307–317. doi: 10.3109/07420528.2011.565897
Tran D., Perrigault M., Ciret P., Payton L. (2020). Bivalve Mollusc Circadian Clock Genes can Run at Tidal Frequency. Proc. R. Soc B. 287, 20192440. doi: 10.1098/rspb.2019.2440
Trofimova T., Alexandroff S. J., Mette M. J., Tray E., Butler P. G., Campana S. E., et al. (2020). Fundamental Questions and Applications of Sclerochronology: Community-Defined Research Priorities. Estuar. Coast. Shelf. Sci. 245, 106977. doi: 10.1016/j.ecss.2020.106977
Verrecchia E. P. (2005). “Multiresolution Analysis of Shell Growth Increments to Detect Variations in Natural Cycles,” in Image Analysis, Sediments and Paleoenvironments (Dordrecht: Springer), 273–293. doi: 10.1007/1-4020-2122-4_14
von Hessling T. (1859). Die Perlmuscheln Und Ihre Perlen Naturwissenschaftlich Und Geschichtlich; Mit Berücksichtigung Der Perlengewässer Bayerns. Ed. Leipzig W.E. Leipzig: Engelmann.
Wang X., Wang M., Jia Z., Song X., Wang L., Song L. (2017). A Shell-Formation Related Carbonic Anhydrase in Crassostrea Gigas Modulates Intracellular Calcium Against CO2 Exposure: Implication for Impacts of Ocean Acidification on Mollusk Calcification. Aquat. Toxicol. 189, 216–228. doi: 10.1016/J.AQUATOX.2017.06.009
Warter V., Erez J., Müller W. (2018). Environmental and Physiological Controls on Daily Trace Element Incorporation in Tridacna Crocea From Combined Laboratory Culturing and Ultra-High Resolution LA-ICP-MS Analysis. Palaeogeogr. Palaeoclimatol. Palaeoecol. 496, 32–47. doi: 10.1016/j.palaeo.2017.12.038
Weiner S., Dove P. M. (2003). “An Overview of Biomineralization Processes and the Problem of the Vital Effect,” in Biomineralization. Eds. Dove P. M., Yoreo J.J.De, Weiner S. (Washington: The mineralogical society of America), 1–24.
Wilbur K. M., Saleuddin A. S. M. (1983). “Shell Formation,” The Mollusca: Physiology Part. 1. (New York:Academic Press Inc.), 235–287. doi: 10.1016/b978-0-12-751404-8.50014-1
Williams B. G., Pilditch C. A. (1997). The Entrainment of Persistent Tidal Rhythmicity in a Filter-Feeding Bivalve Using Cycles of Food Availability. J. Biol. Rhythm. 12, 173–181. doi: 10.1177/074873049701200208
Yamaguchi K., Seto K., Takayasu K., Aizaki M. (2006). Shell Layers and Structures in the Brackish Water Bivalve, Corbicula Japonica. Quat. Res. 45, 317–331. doi: 10.4116/jaqua.45.317
Yan H., Liu C., An Z., Yang W., Yang Y., Huang P., et al. (2020). Extreme Weather Events Recorded by Daily to Hourly Resolution Biogeochemical Proxies of Marine Giant Clam Shells. Proc. Natl. Acad. Sci. U. S. A. 117, 7038–7043. doi: 10.1073/pnas.1916784117
Zhao M., He M., Huang X., Wang Q. (2014). A Homeodomain Transcription Factor Gene, PfMSX, Activates Expression of Pif Gene in the Pearl Oyster Pinctada Fucata. PloS One 9, e103830. doi: 10.1371/journal.pone.0103830
Zhao L., Shirai K., Tanaka K., Milano S., Higuchi T., Murakami-Sugihara N., et al. (2020). A Review of Transgenerational Effects of Ocean Acidification on Marine Bivalves and Their Implications for Sclerochronology. Estuar. Coast. Shelf. Sci. 235, 106620. doi: 10.1016/j.ecss.2020.106620
Keywords: shell biomineralisation, sclerochronology, biological clock, bivalve, environmental archive
Citation: Louis V, Besseau L and Lartaud F (2022) Step in Time: Biomineralisation of Bivalve’s Shell. Front. Mar. Sci. 9:906085. doi: 10.3389/fmars.2022.906085
Received: 28 March 2022; Accepted: 31 May 2022;
Published: 12 July 2022.
Edited by:
Menghong Hu, Shanghai Ocean University, ChinaReviewed by:
Liqiang Zhao, Guangdong Ocean University, ChinaIuliana Motrescu, Iasi University of Life Sciences, Romania
Copyright © 2022 Louis, Besseau and Lartaud. This is an open-access article distributed under the terms of the Creative Commons Attribution License (CC BY). The use, distribution or reproduction in other forums is permitted, provided the original author(s) and the copyright owner(s) are credited and that the original publication in this journal is cited, in accordance with accepted academic practice. No use, distribution or reproduction is permitted which does not comply with these terms.
*Correspondence: Franck Lartaud, ZnJhbmNrLmxhcnRhdWRAb2JzLWJhbnl1bHMuZnI=