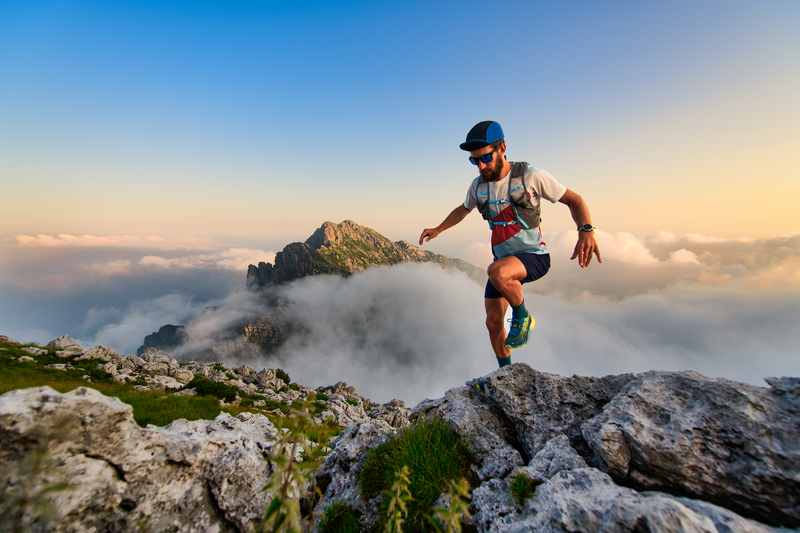
94% of researchers rate our articles as excellent or good
Learn more about the work of our research integrity team to safeguard the quality of each article we publish.
Find out more
ORIGINAL RESEARCH article
Front. Mar. Sci. , 15 September 2022
Sec. Marine Biogeochemistry
Volume 9 - 2022 | https://doi.org/10.3389/fmars.2022.903918
This article is part of the Research Topic The effects of early diagenesis in various marine environments on the stable isotope records of environmental conditions and biogeochemical processes View all 7 articles
Microbial iron (Fe) reduction by naturally abundant iron minerals has been observed in many anoxic aquatic sediments in the sulfidic and methanic zones, deeper than it is expected based on its energetic yield. However, the potential consequence of this “deep” iron reduction on microbial elemental cycles is still unclear in sediments where diffusion is the dominant transport process. In this contribution, we experimentally quantify the impact of iron oxides on sulfate-driven anaerobic oxidation of methane (S-AOM) within the sulfate methane transition zone (SMTZ) of marine diffusive controlled sediments. Sediments were collected from the oligotrophic Southeastern (SE) Mediterranean continental shelf and were incubated with 13C-labeled methane. We followed the conversion of 13C-labeled methane as a proxy of S-AOM and monitored the sediment response to hematite addition. Our study shows microbial hematite reduction as a significant process in the SMTZ, which appears to be co-occurring with S-AOM. Based on combined evidence from sulfur and carbon isotopes and functional gene analysis, the reduction of hematite seems to slow down S-AOM. This contrasts with methane seep environments, where iron oxides appear to stimulate S-AOM and hence attenuate the release of the greenhouse gas methane from the sediments. In the deep methanic zone, the addition of iron oxides inhibits the methanogenesis process and hence methane gas production. The inhibition effect deeper in the sediment is not related to Fe-AOM as a competing process on the methane substrate, since Fe-AOM was not observed throughout the methanic sediments with several iron oxides additions.
Organic matter degradation in sediments is coupled to the microbial reduction of electron acceptors along a ladder of decreasing free energy yield. The ladder progresses from oxygen to nitrate, then manganese and iron oxides, followed by sulfate. Fermentation of organic matter or the reduction of carbon dioxide by hydrogen to methane via methanogenesis is the last energy-yielding reaction (Froelich et al., 1979). Due to the high abundance of sulfate in modern oceans, microbial sulfate reduction coupled to organic matter oxidation (organoclastic sulfate reduction) consumes a significant portion of the organic matter in anoxic sediments (Kasten and Jørgensen, 2000).
While organoclastic sulfate reduction occurs throughout the sulfate zone (Eq. 1, (Jørgensen, 2000), it is often the case that sulfate diffusing into marine sediments will react at the sulfate-methane transition zone (SMTZ) by reacting with the upwards-diffusing flux of methane (e.g., (Niewöhner et al., 1998; Aharon, 2000; Boetius et al., 2000; Sivan et al., 2007), as presented in Eq. 2 (Hoehler et al., 1994). This process of sulfate reduction by anaerobic oxidation of methane (S-AOM) consumes up to 90% of the produced methane in marine sediment and limits its release to the water column and atmosphere (Valentine, 2002).
The S-AOM process is usually catalyzed by archaeal ANaerobic MEthanotrophs (ANMEs) with syntrophic sulfate-reducing bacterial (SRB) partners (Knittel and Boetius, 2009). The metabolic process used by ANMEs is the “reverse-methanogenesis” pathway (Hallam et al., 2004), while methyl-coenzyme M reductase (Mcr) catalyzes the initial step and the gene encoding the alpha subunit of the enzyme (mcrA gene) is usually used as a marker gene for methane metabolism (Hallam et al., 2003; Wang et al., 2019). Chemical species that were found to limit the S-AOM process in sediments, besides sulfate and methane, are some of the trace metals that are required for the enzymes involved in S-AOM such as Ni, Co, Mo/W and Zn (Glass and Orphan, 2012).
Organic carbon degradation below the sulfate depletion zone is controlled by methanogenesis. However, depending on the geological setting, the depositional history and the state of biogeochemical cycling, in many circumstances some iron-oxide mineral pools may remain and can be respired in the methanic zone, resulting in elevation of dissolved ferrous iron concentrations in porewater (Sivan et al., 2007; März et al., 2008; Crowe et al., 2011; Sivan et al., 2011; Norði et al., 2013; Riedinger et al., 2014; Bar-Or et al., 2017; Egger et al., 2017; Vigderovich et al., 2019; Amiel et al., 2020). This phenomenon largely exists in continental shelf and margin sediments (Aromokeye et al., 2020). Particularly, in our former work on the SE Mediterranean continental shelf, we identified microbially mediated iron reduction in the methanic zone of the sediment (Vigderovich et al., 2019). Microbial iron reduction in methanic marine sediments may result from: 1) Successful competition of iron reducing bacteria over methanogens for substrates such as acetate and hydrogen, as was shown near the surface of sediments (Lovley and Phillips, 1987; Conrad, 1999; Roden and Wetzel, 2003); 2) A shift of methanogens or syntrophic cultures from methanogenesis to iron reduction, as was shown in culture experiments (Bond and Lovley, 2002; Van Bodegom et al., 2004; Zhang et al., 2012; Zhang et al., 2013; Yamada et al., 2014; Sivan et al., 2016; Bar-Or et al., 2017); 3) Anaerobic oxidation of methane (Fe-AOM) (Sivan et al., 2007; Riedinger et al., 2014; Treude et al., 2014; Egger et al., 2017; Liang et al., 2019; Aromokeye et al., 2020); 4) Cryptic cycling, between iron oxides and reduced sulfur species (Holmkvist et al., 2011; Treude et al., 2014; Egger et al., 2017) or ammonium (Feammox) (Li et al., 2018).
The question remains whether in some stratigraphic or geochemical settings, a deep iron oxide pool that remains unreacted at or below the SMTZ can influence S-AOM and the makeup of the microbial community. In lake sediment, incubations showed that S-AOM is supported by reduction of iron oxides via oxidation of reduced sulfur species to sulfate under sulfate-starved conditions (Su et al., 2019), a mechanism that was also proposed in marine sediments below the SMTZ (Holmkvist et al., 2011; Pellerin et al., 2018). In incubations of methane seep sediments, iron oxide reduction seemed to stimulate S-AOM and serve as a cycling mechanism that can increase methane oxidation (Sivan et al., 2014). In addition, in the presence of Fe(III) complexes (ferric citrate and ferric-EDTA), even without sulfate, stimulation of AOM activity was observed in ANME seep cultures (Scheller et al., 2016). However, the potential involvement and the specific mechanism in which iron reduction relates to S-AOM have not been shown experimentally in diffusive non-seep marine sediments.
We hypothesized that iron reduction should be significant and influence S-AOM in a variety of depositional environments that are diffusion-dominated and where iron is abundant enough. This was tested by incubating marine sediments from the SE Mediterranean shelf. Sediments were incubated from the sulfate zone as well as the deep methanic zone, where sulfate is completely depleted. Similarly to the approach used in seeps incubations (Sivan et al., 2014), 13C-labeled methane and hematite were added. Hematite is a crystalline iron oxide of relatively low reactivity that can persist in the sediment over long timescales (Canfield, 1989). A previous study (Vigderovich et al., 2019) identified hematite as the most abundant “reactive” Fe oxide (together with goethite and akaganeite) at and below the SMTZ of the SE Mediterranean continental shelf, where iron reduction was recorded in the methanogenic zone. Hematite was thus added to mimic the natural sediments and test its effect on S-AOM without shifting the system to iron reduction. Amorphous iron, a more reactive iron oxide (Poulton and Canfield, 2005), was added to the experiment from the main methanic zone to test the maximum iron-reduction potential on the AOM process.
The field work was performed in the oligotrophic Levantine Basin of SE Mediterranean shelf. The shelf margin of Israel is built mainly of Pliocene-Quaternary Nile-derived sediments. The bottom seawater across the continental shelf is well oxygenated and the sulfate concentration in the water-sediment interface is ~30 mmol L-1 (Sela-Adler et al., 2015). The sediments are mostly clay silts between 30-50 m water depth, while the clay fraction dominates at >50 m water depth (Nir, 1984; Sandler and Herut, 2000). The organic carbon content in the central and eastern regions of the Levantine Basin is relatively low (∼0.1–1.4%; (Almogi-Labin et al., 2009; Sela-Adler et al., 2015; Astrahan et al., 2017). However, biogenic methane was found at some locations in shallow sediments (Sela-Adler et al., 2015).
Sediment cores (~5 - 6 m long) were collected using a Benthos 2175 piston corer, from the undisturbed seafloor sediments of the SE Mediterranean continental shelf at water depths of 85 m from Station SG-1 (32°57.82’ N 34°55.30’ E) (Figure S1). This study site was chosen due to the shallow distinct SMTZ with sulfate driven AOM (Sela-Adler et al., 2015; Wurgaft et al., 2019) and the observed intensive iron reduction in the methanic zone (Vigderovich et al., 2019; Amiel et al., 2020). In this station there is biogenic methane and the total organic carbon content is ~1% throughout the sediment (Sela-Adler et al., 2015). The cores were sliced on board into ~30 cm segments within minutes of retrieval from the seafloor. Sediments were collected immediately from the edge of each section using cut end syringes to pre-flushed vials (for porewater extraction) and bottles (for methane measurement). Porewater was extracted by centrifugation to produce the dissolved geochemical profiles and the sediment in the segment was used for the incubation experiments. The measured porosity in this site was about 0.5 along the cores in all campaigns.
A set of incubation experiments, called Mediterranean SMTZ (MS), was designed to test the influence of iron oxide addition on S-AOM, as detailed in Figure 1. The experiment consisted of sediments retrieved by six-meter piston coring at Station SG-1 in January 2017. Sediments were incubated from the SMTZ in this site (~132-142 cm below the seafloor), as indicated by the sulfate and methane concentration gradients. Within a day of core collection, pre-incubated slurries were prepared under anaerobic conditions in sterile bottles with 1:1 ratio of sediment to sterile artificial seawater containing 10 mM sulfate (details in Table S1). After nine months of pre-incubation at room temperature (20°C) in the dark, at atmospheric pressure, the slurry was transferred to several bottles at the same temperature and pressure conditions. To each N2 pre-flushed 60 mL sterile serum bottle, 22 g of slurry was transferred, and artificial seawater was added for the final 3:1 artificial seawater to sediment ratio (and final concentrations of sulfate of 8 mM). 2 mL of gaseous methane was added to the headspace of the bottle, as well as 0.5 mL of 99% 13C-labeled methane. One of the treatments (2 bottles) consisted of amending with 10 mM of 13C-labeled bicarbonate (H13CO3-) instead of 13C-labeled methane. Two of the bottles were sterilized by autoclaving and treated as a killed control. Hematite powder was added to all bottles besides two (other than the killed), which were used as the control. After 475 days, 16 mL of artificial seawater and sodium molybdate (Na2MoO4) (final concentration of 1 mM), which inhibits sulfate reduction, were added (except for the two bottles with the addition of labelled bicarbonate) to examine the influence of sulfate reduction inhibition on AOM. Sediment samples from each bottle were taken for qPCR analysis before the molybdate addition.
Figure 1 Experimental design. Treatments for SMTZ sediments of SE Mediterranean Sea (MS) shelf; Treatments for deep iron-rich sediments of SE Mediterranean Sea (MD); Yellow bottles represent amendments of 13CCH4 (natural controls); dark blue represents amendments of 13CCH4 and 10 mM of hematite; green represents 13CHCO3, and 10 mM of hematite amendments; light blue represents 13CCH4 and 10 mM of amorphous iron; and red (marked*) the killed controls.
To investigate the effect of iron reduction on the methane cycle in the main methanic zone, another set of experiments was conducted (Figure 1). A sediment core was collected from the same site (SG-1) in November 2017, and sediment from the methanic zone was used for the experiment, where high methane and dissolved Fe2+ were observed (400-435 cm sediment depth, Figure 2). The sediment was pre-incubated for three months in sterilized crimp-sealed glass bottles under anaerobic conditions with a 1:1 ratio of sediment to artificial seawater free of sulfate (details in Table S1). The slurry was then divided into six pre-flushed 60 mL sterile serum bottles, with final sediment to artificial seawater ratio of 1:3. About 0.5 ml of 13C-labeled methane was added to all bottles to follow methane oxidation. To test the maximum potential of these sediments to perform iron reduction, we added highly reactive Fe(III) hydroxide (amorphous iron) to the incubation bottles. Amorphous iron (Fe(OH)3) was prepared and added to 4 bottles (2 killed and 2 non killed) to a final concentration of 10 mM in order to examine the influence of the addition of a highly reactive iron pool on methanogenesis. The controls were treatments without iron oxide addition (natural controls), as well as the autoclaved treatments as killed control with iron oxide addition.
Figure 2 Depth profiles of dissolved geochemical species from SG-1 station from January and November 2017. (A) Sulfate concentrations. (B) Methane concentrations. (C) DIC concentrations. (D) δ13CDIC. (E) dissolved Fe2+ concentrations. The locations of the sediments taken for the incubations are marked in red: The SMTZ in January 2017 (for experiment MS) and the deep methanic iron-rich zone in November 2017 (for experiment MD). Both duplicates are presented when measured. The analytical error is marked if larger than the symbol.
Sediments were used for headspace methane concentration measurements on a gas chromatograph equipped with a flammable ionization detector (FID) at a precision of 2 µmol CH4 L−1. Extracted porewater was filtered through a 0.22 µm filter. A subsample was measured for dissolved sulfate using a Dionex DX500 high-performance liquid chromatograph (HPLC) with an error of 3%. Dissolved Fe2+ concentrations were measured using the ferrozine method (Stookey, 1970) by a spectrophotometer at 562 nm wavelength with a detection limit of 1 µmol L−1. Dissolved sulfide was measured by addition of zinc acetate to the porewater and quantification of the precipitated ZnS colorimetry (Cline, 1969). Another subsample of filtered porewater was transferred into a He-flushed vial containing 50 µl of concentrated H3PO4 that released all DIC to the headspace as CO2. Measurements of δ13CDIC were done using a conventional isotopic ratio mass spectrometer (IRMS, DeltaV Advantage, Thermo) with a precision of ±0.1‰, and the results are reported versus Vienna Pee Dee Belemnite (VPDB) standard. In this measurement the DIC concentration was calculated as well from the peak height, using a calibration curve (by standard samples prepared from NaHCO3 in different concentrations) and an error of ±0.2 mM. For δ34SSO4 analysis, ~1 ml of filtered subsample was transferred into a vial containing 20% zinc acetate to precipitate and remove sulfides as a precaution and standard procedure for this analysis (even though no free sulfide was measured). The tubes were centrifuged at 5000 RPM for 15 minutes in order to separate any ZnS that might have formed (Pellerin et al., 2018). Then, the supernatant was transferred to a new falcon tube and BaCl2 was added to precipitate barite (BaSO4). After three rinsing steps and drying at 100°C overnight, the barite was weighed into tin capsules and combusted at 1030 °C in a Flash Element Analyzer (EA), and the resulting sulfur dioxide (SO2) was measured for its δ34S composition by continuous helium flow on a GS-IRMS (Thermo Finnegan Delta V Plus Godwin Laboratory, University of Cambridge) with a precision of ±0.3‰.
Microbial DNA was extracted from the sediment samples of Experiment MS using a Power Soil DNA Kit (MoBio Laboratories, Inc., Carlsbad, CA, USA) following the manufacturer’s instructions. Copy numbers of selected genes were estimated with quantitative PCR (qPCR) as described previously (Niu et al., 2017) using specific primers: Uni519f/Arc908R and bac341f/519r for archaeal and bacterial 16S rRNA genes, respectively, and mlas/mcrA-rev for the mcrA gene. The amplification efficiency was 92.4%, 105.3%, and 94.2% for the archaeal 16S rRNA gene, bacterial 16S rRNA gene and the mcrA gene, respectively (the respective R2 of the standard curve was 0.996, 0.998, and 0.991).
The geochemical depth profiles along the sediment cores collected at SG-1 for the incubations are from January 2017 (for the experiment in the SMTZ) and from November 2017 (for the deep methanic experiment), and presented in Figure 2. On both dates sulfate decreased from seawater values at the upper sediments to near-complete depletion below 200 cm (Figure 2A), as in our other campaigns to this station (Vigderovich et al., 2019). We suggest that detectable concentrations of sulfate within the methanic zone on November 2017 are an artifact of on board methane gas release, which may result in slight advective water transport at the edge of the core segments in some cases. This may compromise sulfur isotope analyses [e.g. (Pellerin et al., 2018)] but is less crucial in this study. Sulfide was detected neither analytically (< 5 µM) nor by smell during on-board handling (as in previous campaigns to the shallow Eastern Mediterranean; Vigderovich et al., 2019), and we suggest that this is due to iron sulfide mineral precipitation.
Below the SMTZ methane started to accumulate (Figure 2B), reaching a maximum around 400 cm depth. It should be noted again that methane measurements below the potential depth of methane saturation are not accurate, due to pressure release during core collection and degassing. Therefore, the variations that we measure in the methanic zone may not reflect real concentrations but just the leftover methane in the sediment, as shown nicely by Beulig et al. (2019).
Both profiles show high (though different) concentrations of DIC along the entire profile, (Figure 2C). The δ13CDIC values (Figure 2D) in January 2017 showed dramatic decrease to a minimum value of about -25 ‰ at the SMTZ (~140cm) and then a gradual increase. The δ13CDIC values in November 2017 showed a minimum of about -16 ‰ at around 190 cm sediment depth at the SMTZ due to S-AOM and then an increase in the methanic zone (with another decrease at 650 cm depth). Dissolved Fe2+ concentrations (Figure 2E) were generally low, showing 3 peaks in the methanic zone (in the upper zone around 250 cm, in the middle at around 400 cm and in the deep part at about 620 cm depth.
Experiment MS consisted of sediment slurry collected from the SMTZ (132-142 cm depth) in January 2017. Experiment MS tested whether the addition of hematite to sediment slurries from the SMTZ of the SE Mediterranean continental shelf stimulates S-AOM, as had been shown in seep-sediments (Sivan et al., 2014). In addition to hematite, the slurries were amended with 13C-labeled methane. Sulfate concentrations were around 8 mM for all bottles at the onset of the experiment and did not vary among the different treatments throughout the incubation (Figure 3A). Since sulfate reduction imparts a large sulfur isotope fractionation, evidence for net sulfate reduction can be significantly imprinted in sulfate isotopes, as more sensitive tools than sulfate concentrations (e.g. (Sivan et al., 2014). Indeed, δ34SSO4 values in all non-killed bottles increased with time, indicating active sulfate reduction. Interestingly, in the sediments amended with hematite, the δ34SSO4 values increased by about 2 ‰ (from 5.4 ‰ to 7 ‰ and 7.5 ‰) in 440 days, while in the unamended sediment, δ34SSO4 values increased by about 3 ‰ from 5.3 ‰ to 8.1 ‰ (Figure 3B). The initial value of the dead control bottles was 5.6 ‰, which remained stable within 0.1‰ over the course of the experiment.
Figure 3 MS slurry incubations experiment. (A) SO42- concentrations; (B) δ34SSO4; (C) CH4 concentrations; (D) DIC concentrations; (E) δ13CDIC; (F) dissolved Fe2+ concentrations. In (E) the black arrow indicates molybdate addition after 475 days. Natural samples - yellow line; Addition of 10 mM Hematite - dark blue line; Labeled bicarbonate with Hematite addition - green line; Autoclaved + Hematite (killed control) - red line. Both duplicate bottles are shown, and the analytical error is marked if larger than the symbol.
Methane and DIC concentrations did not show any significant pattern in all treatments over 440 incubation days (Figures 3C, D). However, again, despite no substantial changes in concentrations, carbon isotopes were more sensitive and showed evidence for AOM by 13C transfer from the 13C-labeled methane to DIC. The δ13CDIC value was initially at c.a. -16 ‰ (except the dead control, Figure 3E), and then increased significantly throughout the incubation to +44.3 ‰ and +18.5 ‰ after 440 days in the hematite treatments (with large natural variability), and to +65.1 ‰ and +50.6‰ in the natural control bottles. Dead control bottles showed values between -4 ‰ and -10.5 ‰. Molybdate addition after 475 days of incubation stopped the δ13CDIC increase immediately, indicating that S-AOM was responsible for the transfer of the 13C label to the DIC pool. Combined C and S isotopes suggests direct effect of S-AOM with the addition of hematite, as discussed below.
In addition to the incubations described above, two additional bottles were amended with an aliquot of 13C-labeled bicarbonate to monitor the fate of the inorganic carbon pool. There was no amendment of 13C-methane in these bottles but of methane with a natural abundance of 13C (δ13C value of -70 ‰). A decrease in the δ13CDIC of the DIC pool from about ~1500 ‰ to about 1200 ‰ was observed over the 440 days incubation (Figure 3E). This indicates that the DIC pool must be turning over despite relatively stable DIC concentrations. Dissolved Fe2+ concentrations increased significantly in all the non-killed bottles immediately after the beginning of the experiments, indicating intensive microbial processes occurring naturally in the slurries in the beginning of the incubations. After 5 experimental days, dissolved Fe2+ concentrations remained relatively constant in all bottles at about ~55 µM (Figure 3F).
Quantification of archaeal 16S rRNA gene, bacterial 16S rRNA gene, and mcrA gene were conducted at the beginning (T0) and the end of Experiment MS as a tool to quantify the changes in the abundance of bacterial and archaeal biomass as well as methane metabolic gene. The qPCR of bacterial and archaeal 16S rRNA genes revealed that the abundances were 8.4×106 and 11×106 copies per gram wet sediment at the beginning of the experiment, respectively (Figure 4A). In the hematite treatment after 460 days, bacterial numbers increased slightly (9.5×106 and 1.3×107 copies per g wet sediment), and the numbers doubled for the archaea (2.2×107 and 2.6×107 copies per g wet sediment). In the natural control bottles after 460 days of incubation, the abundance of both bacteria and archaea increased dramatically (to 3.2×107 and 2.6×107 copies per g wet sediment for bacteria, and 5.5×107 and 4.9×107 copies per g wet sediment for archaea).
Figure 4 Functional gene copy counting per gram wet sediment on T0, natural and added hematite treatments from MS experiment. (A) Bacterial, archaeal and mcrA counts, which are shown in dotted, dashed and solid lines, respectively. (B) Only the mcrA counts.
At T0, the abundance of the mcrA gene was 3.3×105 copies per g wet sediment (Figure 4B). After 460 days, the abundance of the mcrA gene in the hematite treatment was similar to the initial abundance (3.7×105 and 7. 5×105 copies per g wet sediment), whereas the natural control bottles had one order of magnitude higher abundances of mcrA gene relative to the start (2.0×106 and 1.6×106 copies per g wet sediment). It can be seen that the microbial measurements support the geochemical data and suggest that the addition of iron oxides inhibited the growth of the S-AOM bacterial and archaeal community as well as methane metabolism indicated by the marker gene mcrA gene.
Experiment MD consisted of sediment slurry collected from the deep methanic zone (400-435 cm depth) in November 2017. This experiment examined the possible connection between iron reduction, methanogenesis and the potential for AOM in the deep sediments (depleted with sulfate). This was achieved by adding amorphous iron as the most reactive oxide and 13C- labeled methane over 490 days of observation (Figure S2). Dissolved Fe2+ concentrations (Figure S2A) showed gradual increase with time in all bottles from about 80 µM to 120 µM in those with the amorphous iron and to about 160 µM in the natural and killed bottles, maybe due to some precipitation of magnetite with the addition of amorphous iron. The DIC concentration was around 8 mM in the killed bottles and around 5 mM in the rest throughout the experiment (Figure S2B). The δ13CDIC initial values in all bottles were between -7.8 ‰ and -11 ‰ (Figure S2C). The δ13CDIC value in natural control bottles was stable for the first 165 days and then increased to a final value of up to -2.9 ‰, probably due to the natural carbon isotopic fractionation during methanogenesis. The δ13CDIC values in the dead control bottles and in the bottles containing amorphous remained within 1 ‰ of their initial value over the 490 days of the experiment, probably due to inhibition of the methanogenesis process.
The porewater profiles from the SE Mediterranean continental shelf show significant iron reduction below the expected zone in the upper sediment, into the sulfate reduction and methanic zone. In the SMTZ there is no buildup of porewater Fe2+, probably due to precipitation of iron with sulfide as FeS and FeS2, as we showed previously (Wurgaft et al., 2019). Below the SMTZ, where sulfide is absent, there is a large increase of dissolved Fe2+, most likely as a product of microbial iron reduction.
The observed Fe(II) in the non-killed sediment incubations also indicates significant microbial iron reduction in the SMTZ and in the methanic zone, even with the addition of hematite, which displays the slowest microbial reduction kinetics. The experimental design enabled not only observing this iron reduction, but also quantifying the influence of iron mineral assemblages on the microbial metabolisms, which is challenging based on the porewater geochemical profiles alone (Antler et al., 2014; Sela-Adler et al., 2015; Vigderovich et al., 2019; Wurgaft et al., 2019).
The observed dissolved Fe2+ in the non-killed sediment incubations suggest iron reduction co-exists with other processes, such as organoclastic sulfate reduction, S-AOM, and methanogenesis (Figure 3). This agrees with previous observations based solely on porewater geochemistry (e.g. Vigderovich et al., 2019). A significant AOM signal is shown in the SMTZ by the transfer of 13C-labeled methane to enriched 13C-DIC (Figure 3). The methane is likely oxidized by the process of S-AOM, which is evident by the inactivation of this process (indicated by δ13CDIC) with the addition of molybdate (Figure 3). This is also evident with the stop in sulfur isotopic fractionation (Figure 3), and the change in distribution of functional genes (Figure 4).
S-AOM reduces a large portion of the sulfate in the SE Mediterranean shelf sediments. Wurgaft et al. (2019) argued that about half of the sulfate reduction within the SMTZ is due to organoclastic sulfate reduction and the other half by S-AOM. No AOM was observed in the methanic zone below the sulfidic zone, at the deeper few meters of the core at SG-1. This can be seen in incubation experiment MD (Figure S2), where even after more than a year of incubation, the δ13CDIC value did not increase by more than 10 ‰ in the natural incubations, much less than the expected change due to any AOM activity with the addition of 13C-labeled methane. The slight increase in δ13CDIC is most likely due to the natural fractionation of methanogenesis. This is in contrast to lake methanic sediments, where, with an identical methodology and with the same order of magnitude of cells and 13C-methane labeling extremely high enrichments in 13C of the DIC pool were observed (Bar-Or et al., 2017; Vigderovich et al., 2021). These results suggest that below the SMTZ of SE Mediterranean shelf sediments, no evident AOM is taking place.
This lack of AOM activity in experiments from the deep methanic zone of diffusive marine sediments (with seawater salinity) is a significant observation but perplexing. It suggests that the iron reduction observed in the deep methanic zone of marine sediments that was previously attributed only to Fe-AOM in diagenetic models (Sivan et al., 2007; Riedinger et al., 2014; Treude et al., 2014; Egger et al., 2017) may be also related to other mechanisms. Another possibility is that Fe-AOM is present in natural conditions but not observed in long-term incubations. These results agree with another study in marine diffusive sediment, which found only slight 13C-labeled methane transfer to DIC, and hence a low AOM signal (Aromokeye et al., 2020). The low signal may relate to the rates attributed to Fe-AOM, which could be as low as 0.095 nmol cm−3 d−1 in North Sea sediment (Aromokeye et al., 2020). It may also be related to the doubling time of the microbes performing the AOM. In the case of S-AOM, the consortia doubling time was reported to be between 3 and 7 months (Yu et al., 2022). We propose thus that the significant iron reduction in the methanic zone could be mediated by alternative mechanisms, like a switch of methanogens to iron reduction due to some physiological advantages over other functional groups (such as the presence of methanophenazines in some methanogens) or due to other changes in this zone (Sivan et al., 2016; Vigderovich et al., 2019).
The incubation experiments show that the addition of iron oxides to sediment incubations from the SMTZ affects both the δ13CDIC and the δ34SSO4 and the microbial data, thus directly the S-AOM activity. It seems that the most likely explanation for the significantly lower enrichment in both 13C-DIC and in 34SSO4 is that the addition of the hematite slows down sulfate reduction by AOM. Previous studies have suggested that the lower values of δ34SSO4 with the addition of hematite than without its addition could be also explained by higher rates of S-AOM, lowering the isotopic discrimination imparted by microbial activity (Sivan et al., 2014) or by lowering sulfate concentrations (Habicht and Canfield, 1997; Brunner and Bernasconi, 2005). However, higher rates of S-AOM would lead to enrichment in 13C-DIC (from the labeled methane), as shown in seeps (Sivan et al., 2014) but this is not the case here. A decrease in the organoclastic sulfate reduction with the addition of iron oxides could also be due to adsorption of organic compounds onto iron oxides that lead to their preservation in sediments, interfering with the availability of degradable organic compounds for sulfate reduction (Lalonde et al., 2012). This, however, would also result in a smaller imprint of light carbon isotope from organoclastic sulfate reduction and thus more enrichment in 13C-DIC.
The explanation that the addition of the hematite slows down sulfate reduction by AOM is supported by the microbial data (Figure 4). We measured in the MS experiment the abundances (qPCR) of 16S rRNA gene of bacteria and archaea and the mcrA gene (diagnostic indicator for methyl coenzyme M reductase (Hallam et al., 2003)). All were significantly higher in samples of natural bottles compared to bottles containing hematite. This reinforces that the hematite addition inhibited the growth of both methanotrophic archaea and sulfate reducing bacteria. The abundances of bacteria and mcrA gene in samples from bottles containing hematite were very similar to those of T0 samples, but the number of 16S rRNA gene copies of archaea in bottles containing hematite was twice as high as that in T0. This may indicate that different archaea, which do not have methane-related metabolism, are surviving in the bottles containing hematite, maybe due to other respiratory processes.
A rough calculation suggests that the inhibition of S-AOM by iron oxides accounts for ~40% decrease in the AOM rates in the SE Mediterranean site. The calculation was based on changes in δ34SSO4, the initial concentration of sulfate in the slurries (8.2 mM), and a fractionation factor of ~20 ‰, estimated for S-AOM in this and other areas (Avrahamov et al., 2014; Deusner et al., 2014; Sivan et al., 2014). We used the Rayleigh equation for isotopic distillation (Eq. 3), and Eq. 4 to calculate how much sulfate reacted with methane in the different treatments. In the natural bottles, 1 mM of sulfate was consumed for AOM, while only 0.6 mM sulfate was consumed in bottles containing hematite.
An important finding of this study is that there appears to be a different interplay between AOM, organoclastic sulfate reduction and iron reduction in diffusion-controlled sediments than in marine seeps, where iron addition stimulated S-AOM (Sivan et al., 2014). Inhibition of AOM by iron oxides is not trivial. We questioned why the addition of iron oxides slows down S-AOM in marine diffusive sediments. Three possible mechanisms are suggested:
1) Iron oxide addition increases the oxidation state of the sediments, impacting the S-AOM process. Iron oxide addition can increase the oxidation state by reacting with several electron donors, thereby releasing dissolved oxidized species. For example, ammonium oxidation by iron reduction (Feammox) would lower the concentrations of ammonium and release nitrite to the porewater (Li et al., 2018), increasing the redox potential. This change in the oxidation state will influence S-AOM. S-AOM was shown to be performed through the syntrophic metabolism between ANME and SRB, and extracellular electron transfer was proposed to be adopted by ANME to transfer the electrons from methane to SRB partners (Chadwick et al., 2022; Yu et al., 2022). Various electron carriers/intermediates were suggested in S-AOM process, including redox active compounds like hydrogen, formate, acetate, methylsulfides, zero-valent sulfur as well as direct interspecies electron transfer by nanowires formed by cytochrome proteins or even 9,10-anthraquinone- 2,6-disulfonate (AQDS) (Nauhaus et al., 2005; Milucka et al., 2012; Wegener et al., 2015; Scheller et al., 2016). The detailed mechanisms and factors that could influence electron transfer between ANME and SRB still remain as open questions. Since the oxidation state of the substrates or electron carriers may control microbial electron transfer pathway and process (Levar et al., 2017; Zhang et al., 2020), the elevated oxidation state of the sediments would impact S-AOM.
2) Iron reducing microbes out-compete and utilize substrates that are needed for S-AOM. This includes methane itself, hydrogen, essential metals for assimilation that are needed for S-AOM or conductive compounds. It should be noted, however, that a previous study showed no need for hydrogen for sulfate reducers in the S-AOM process (Wegener et al., 2015). Also, our experimental results do not support any Fe-AOM in the SMTZ, as the addition of iron did not cause any increase of δ13CDIC over the natural controls without its addition (Figure 2). Fe-AOM was not observed also in the deep methanic zone (Figure S2), as no enriched 13C-DIC was detected, even with the addition of highly reactive amorphous iron.
3) Sulfate reducers switch to iron reduction when iron oxides are added, and the S-AOM is inhibited due to this microbial limitation. It is known that there are bacteria that can respire both iron oxides and sulfur species (Lovley et al., 2004). These includes Desulfobulbus (Holmes et al., 2004), which was found indeed in these sediments (Vigderovich et al., 2019). A shift from the reduction of sulfate to iron reduction can cause S-AOM inhibition in case of microbial stress. Our results indicate, as mentioned above that there is no significant AOM in the main methanic zone, as there is no more than 10 ‰ increase in the δ13CDIC value (incubation experiment MD). The addition of iron oxides probably inhibits the methanogenic activity in these deep sediments and thus the increase in the δ13CDIC. Methanogenesis yields much less energy than respiration of organic matter with terminal electron acceptors such as nitrate, iron oxides or sulfate. Therefore, methanogenesis usually only dominates the degradation pathway of organic matter at low availability of these electron acceptors. The addition of poorly ordered Fe(III) oxides to the sediment is a new source of advantageous electron acceptor that will effectively compete with methanogenesis. Thus, in certain natural conditions in the methanogenic zone hematite reduction can become more favorable than methanogenesis. Two different mechanisms can result in this inhibition of methanogenesis. First, inhibition can occur because of competition of Fe(III)-reducing bacteria and methanogens for the common substrates acetate and hydrogen (e.g. Achtnich et al., 1995). Second, methanogenesis can be directly inhibited by Fe(III) by the switch of methanogens to iron reduction (Van Bodegom et al., 2004). Some methanogens may have switched to iron reduction, but the presence of Fe(III) can by itself inhibit some parts of the metabolic pathway. It was not possible with the data acquired to identify the main driving force behind the reduction in methanogensis upon addition of poorly ordered Fe(III) oxides. Given that this is a natural sediment containing iron reducing metabolisms and methanogens, it is likely that both processes led to the inhibition that was observed.
Incubation experiments allow us to probe the mechanisms that control biogeochemical cycling in methanic sediments. In the SE Mediterranean, it seems like the depositional history and local environment exerts a strong control on the interplay between the Fe and S cycles. The combined sulfur and carbon isotopes and functional gene analyses indicate that the reduction of hematite slows down S-AOM. The plausible explanations are that iron oxide addition increases the oxidation state of the sediments, which causes a change in the potential S-AOM intermediates and/or that sulfate reducers switch to iron reduction and the S-AOM is inhibited due to this microbial limitation. Different conclusions are reached when the influence of Fe on S-AOM is investigated in dynamic seep environments, where iron oxides may enhance AOM. In the main methanic zone we did not observe any evidence for AOM of any type. There, the addition of iron oxides probably inhibited the methanogenic activity. Our study emphasizes the importance of Fe and S cycles for methane and organic carbon mineralization in marine sediments, and the need to continue to peer into the mechanistic controls that regulate the Fe and S cycles and therefore, the cycling of carbon.
The original contributions presented in the study are included in the article/Supplementary Material. Further inquiries can be directed to the corresponding author.
OY and OS designed the experiments; OY performed the research together with LL, OS, and BH (sampling and incubations); all authors analyzed the data; OY wrote the manuscript with the help of the co-authors. All authors contributed to the article and approved the submitted version.
This project was supported by the NSFC grant (41921006, 42141003) to FW, and the ISF grant (2561/16) and the H2020 European Research Council grant (818450) to OS.
The authors thank the crew of the R.V Bat-Galim for their assistance during field sampling and core handling. Many thanks to Gilad Antler for the sulfur isotope analysis. We also thank Valeria Boyko, Effie Eliani-Russak and Hanni Vigderovich for their help in laboratory work, and Gilad Antler, Alice Bosco Santos and Eyal Wurgaft for discussions and editing.
The authors declare that the research was conducted in the absence of any commercial or financial relationships that could be construed as a potential conflict of interest.
The handling editor IH declared a past collaboration with the author OS.
All claims expressed in this article are solely those of the authors and do not necessarily represent those of their affiliated organizations, or those of the publisher, the editors and the reviewers. Any product that may be evaluated in this article, or claim that may be made by its manufacturer, is not guaranteed or endorsed by the publisher.
The Supplementary Material for this article can be found online at: https://www.frontiersin.org/articles/10.3389/fmars.2022.903918/full#supplementary-material
Achtnich C., Bak F., Conrad R. (1995). Competition for electron donors among nitrate reducers, ferric iron reducers, sulfate reducers, and methanogens in anoxic paddy soil. Biol. Fertil. Soils 19, 65–72. doi: 10.1007/BF00336349
Aharon P. (2000). “Microbial processes and products fueled by hydrocarbons at submarine seeps,” in Microbial sediments (Berlin, Heidelberg:Springer), 270–281. doi: 10.1007/978-3-662-04036-2_29
Almogi-Labin A., Bar-Matthews M., Shriki D., Kolosovsky E., Paterne M., Schilman B., et al. (2009). Climatic variability during the last∼ 90 ka of the southern and northern levantine basin as evident from marine records and speleothems. Quaternary Sci. Rev. 28, 2882–2896. doi: 10.1016/j.quascirev.2009.07.017
Amiel N., Shaar R., Sivan O. (2020). The effect of early diagenesis in methanic sediments on sedimentary magnetic properties: Case study from the SE Mediterranean continental shelf. Front. Earth Sci. 8, 283. doi: 10.3389/feart.2020.00283
Antler G., Turchyn A. V., Herut B., Davies A., Rennie V. C., Sivan O. (2014). Sulfur and oxygen isotope tracing of sulfate driven anaerobic methane oxidation in estuarine sediments. Estuarine Coast. Shelf Sci. 142, 4–11. doi: 10.1016/j.ecss.2014.03.001
Aromokeye D. A., Kulkarni A. C., Elvert M., Wegener G., Henkel S., Coffinet S., et al. (2020). Rates and microbial players of iron-driven anaerobic oxidation of methane in methanic marine sediments. Front. Microbiol. 10, 3041. doi: 10.3389/fmicb.2019.03041
Astrahan P., Silverman J., Gertner Y., Herut B. (2017). Spatial distribution and sources of organic matter and pollutants in the SE Mediterranean (Levantine basin) deep water sediments. Mar. Pollut. Bull. 116, 521–527. doi: 10.1016/j.marpolbul.2017.01.006
Avrahamov N., Antler G., Yechieli Y., Gavrieli I., Joye S., Saxton M., et al. (2014). Anaerobic oxidation of methane by sulfate in hypersaline groundwater of the dead Sea aquifer. Geobiology 12, 511–528. doi: 10.1111/gbi.12095
Bar-Or I., Elvert M., Eckert W., Kushmaro A., Vigderovich H., Zhu Q., et al. (2017). Iron-coupled anaerobic oxidation of methane performed by a mixed bacterial-archaeal community based on poorly reactive minerals. Environ. Sci. Technol. 51, 12293–12301. doi: 10.1021/acs.est.7b03126
Beulig F., Roy H., McGlynn S. E., Jorgensen B. B. (2019). Cryptic CH4 cycling in the sulfate-methane transition of marine sediments apparently mediated by ANME-1 archaea. ISME J. 13, 250–262. doi: 10.1038/s41396-018-0273-z
Boetius A., Ferdelman T., Lochte K. (2000). Bacterial activity in sediments of the deep Arabian Sea in relation to vertical flux. Deep Sea Res. Part II: Topical Stud. Oceanogr. 47, 2835–2875. doi: 10.1016/S0967-0645(00)00051-5
Bond D. R., Lovley D. R. (2002). Reduction of fe (III) oxide by methanogens in the presence and absence of extracellular quinones. Environ. Microbiol. 4, 115–124. doi: 10.1046/j.1462-2920.2002.00279.x
Brunner B., Bernasconi S. M. (2005). A revised isotope fractionation model for dissimilatory sulfate reduction in sulfate reducing bacteria. Geochim. Cosmochim. Ac. 69, 4759–4771. doi: 10.1016/j.gca.2005.04.015
Canfield D. E. (1989). Reactive iron in marine sediments. Geochim. Cosmochim. Acta 53, 619–632. doi: 10.1016/0016-7037(89)90005-7
Chadwick G. L., Skennerton C. T., Laso-Perez R., Leu A. O., Speth D. R., Yu H., et al. (2022). Comparative genomics reveals electron transfer and syntrophic mechanisms differentiating methanotrophic and methanogenic archaea. PloS Biol. 20, e3001508. doi: 10.1371/journal.pbio.3001508
Cline J. D. (1969). Spectrophotometric determination of hydrogen sulfide in natural waters 1. Limnol. Oceanogr. 14, 454–458. doi: 10.4319/lo.1969.14.3.0454
Conrad R. (1999). Contribution of hydrogen to methane production and control of hydrogen concentrations in methanogenic soils and sediments. FEMS Microbiol. Ecol. 28, 193–202. doi: 10.1111/j.1574-6941.1999.tb00575.x
Crowe S. A., Katsev S., Leslie K., Sturm A., Magen C., Nomosatryo S., et al. (2011). The methane cycle in ferruginous lake matano. Geobiology 9, 61–78. doi: 10.1111/j.1472-4669.2010.00257.x
Deusner C., Holler T., Arnold G. L., Bernasconi S. M., Formolo M. J., Brunner B. (2014). Sulfur and oxygen isotope fractionation during sulfate reduction coupled to anaerobic oxidation of methane is dependent on methane concentration. Earth Planet. Sc. Lett. 399, 61–73. doi: 10.1016/j.epsl.2014.04.047
Egger M., Hagens M., Sapart C. J., Dijkstra N., van Helmond N. A. G. M., Mogollon J. M., et al. (2017). Iron oxide reduction in methane-rich deep Baltic Sea sediments. Geochim. Cosmochim. Ac. 207, 256–276. doi: 10.1016/j.gca.2017.03.019
Froelich P. N., Klinkhammer G., Bender M. L., Luedtke N., Heath G. R., Cullen D., et al. (1979). Early oxidation of organic matter in pelagic sediments of the eastern equatorial Atlantic: suboxic diagenesis. Geochim. Cosmochim. Ac. 43, 1075–1090. doi: 10.1016/0016-7037(79)90095-4
Glass J., Orphan V. (2012). Trace metal requirements for microbial enzymes involved in the production and consumption of methane and nitrous oxide. Front. Microbiol. 3. doi: 10.3389/fmicb.2012.00061
Habicht K. S., Canfield D. E. (1997). Sulfur isotope fractionation during bacterial sulfate reduction in organic-rich sediments. Geochim. Cosmochim. Ac. 61, 5351–5361. doi: 10.1016/S0016-7037(97)00311-6
Hallam S. J., Girguis P. R., Preston C. M., Richardson P. M., DeLong E. F. (2003). Identification of methyl coenzyme m reductase a (mcrA) genes associated with methane-oxidizing archaea. Appl. Environ. Microbiol. 69, 5483–5491. doi: 10.1128/AEM.69.9.5483-5491.2003
Hallam S. J., Putnam N., Preston C. M., Detter J. C., Rokhsar D., Richardson P. M., et al. (2004). Reverse methanogenesis: testing the hypothesis with environmental genomics. Science 305, 1457–1462. doi: 10.1126/science.1100025
Hoehler T. M., Alperin M. J., Albert D. B., Martens C. S. (1994). Field and laboratory studies of methane oxidation in an anoxic marine sediment: Evidence for a methanogen-sulfate reducer consortium. Global Biogeochem. Cycles 8, 451–463. doi: 10.1029/94GB01800
Holmes D. E., Bond D. R., Lovley D. R. (2004). Electron transfer by desulfobulbus propionicus to fe (III) and graphite electrodes. Appl. Environ. Microbiol. 70, 1234–1237. doi: 10.1128/AEM.70.2.1234-1237.2004
Holmkvist L., Ferdelman T. G., Jorgensen B. B. (2011). A cryptic sulfur cycle driven by iron in the methane zone of marine sediment (Aarhus bay, Denmark). Geochim. Cosmochim. Ac. 75, 3581–3599. doi: 10.1016/j.gca.2011.03.033
Jørgensen B. B. (2000). “Bacteria and marine biogeochemistry,” in Marine geochemistry (Berlin, Heidelberg: Springer), 173–207. doi: 10.1007/978-3-662-04242-7_5
Kasten S., Jørgensen B. B. (2000). “Sulfate reduction in marine sediments,” in Marine geochemistry ((Berlin, Heidelberg: Springer), pp. 263–281. doi: 10.1007/978-3-662-04242-7-8
Knittel K., Boetius A. (2009). Anaerobic oxidation of methane: progress with an unknown process. Annu. Rev. Microbiol. 63, 311–334. doi: 10.1146/annurev.micro.61.080706.093130
Lalonde K., Mucci A., Ouellet A., Gélinas Y. (2012). Preservation of organic matter in sediments promoted by iron. Nature 483, 198–200. doi: 10.1038/nature10855
Levar C. E., Hoffman C. L., Dunshee A. J., Toner B. M., Bond D. R. (2017). Redox potential as a master variable controlling pathways of metal reduction by geobacter sulfurreducens. ISME J. 11, 741–752. doi: 10.1038/ismej.2016.146
Liang L., Wang Y., Sivan O., Wang F. (2019). Metal-dependent anaerobic methane oxidation in marine sediment: Insights from marine settings and other systems. Sci. China Life Sci. 62, 1287–1295. doi: 10.1007/s11427-018-9554-5
Li X., Yuan Y., Huang Y., Liu H.-W., Bi Z., Yuan Y., et al. (2018). A novel method of simultaneous NH4+ and NO3– removal using fe cycling as a catalyst: Feammox coupled with NAFO. Sci. Total Environ. 631, 153–157. doi: 10.1016/j.scitotenv.2018.03.018
Lovley D. R., Holmes D. E., Nevin K. P. (2004). Dissimilatory fe (iii) and mn (iv) reduction. Adv. microbial. Physiol. 49, 219–286. doi: 10.1016/S0065-2911(04)49005-5
Lovley D. R., Phillips E. J. (1987). Competitive mechanisms for inhibition of sulfate reduction and methane production in the zone of ferric iron reduction in sediments. Appl. Environ. Microbiol. 53, 2636–2641. doi: 10.1128/aem.53.11.2636-2641.1987
März C., Hoffmann J., Bleil U., de Lange G. J., Kasten S. (2008). Diagenetic changes of magnetic and geochemical signals by anaerobic methane oxidation in sediments of the Zambezi deep-sea fan (SW Indian ocean). Mar. Geol. 255, 118–130. doi: 10.1016/j.margeo.2008.05.013
Milucka J., Ferdelman T. G., Polerecky L., Franzke D., Wegener G., Schmid M., et al. (2012). Zero-valent sulphur is a key intermediate in marine methane oxidation. Nature 491, 541–546. doi: 10.1038/nature11656
Nauhaus K., Treude T., Boetius A., Krüger M. (2005). Environmental regulation of the anaerobic oxidation of methane: a comparison of ANME-I and ANME-II communities. Environ. Microbiol. 7, 98–106. doi: 10.1111/j.1462-2920.2004.00669.x
Niewöhner C., Hensen C., Kasten S., Zabel M., Schulz H. (1998). Deep sulfate reduction completely mediated by anaerobic methane oxidation in sediments of the upwelling area off Namibia. Geochim. cosmochimica Acta 62, 455–464. doi: 10.1016/S0016-7037(98)00055-6
Niu M., Fan X., Zhuang G., Liang Q., Wang F (2017). Methane-metabolizing microbial communities in sediments of the Haima cold seep area , northwest slope of the South China Sea. FEMS Microbiol. Ecol. 93, 1–13. doi: 10.1093/femsec/fix101
Norði K.à., Thamdrup B., Schubert C. J. (2013). Anaerobic oxidation of methane in an iron-rich Danish freshwater lake sediment. Limnol. Oceanogr. 58, 546–554. doi: 10.4319/lo.2013.58.2.0546
Pellerin A., Antler G., Roy H., Findlay A., Beulig F., Scholze C., et al. (2018). The sulfur cycle below the sulfate-methane transition of marine sediments. Geochim. Cosmochim. Ac. 239, 74–89. doi: 10.1016/j.gca.2018.07.027
Poulton S. W., Canfield D. E. (2005). Development of a sequential extraction procedure for iron: implications for iron partitioning in continentally derived particulates. Chem. Geol. 214, 209–221. doi: 10.1016/j.chemgeo.2004.09.003
Riedinger N., Formolo M. J., Lyons T. W., Henkel S., Beck A., Kasten S. (2014). An inorganic geochemical argument for coupled anaerobic oxidation of methane and iron reduction in marine sediments. Geobiology 12, 172–181. doi: 10.1111/gbi.12077
Roden E. E., Wetzel R. (2003). Competition between fe (III)-reducing and methanogenic bacteria for acetate in iron-rich freshwater sediments. Microbial Ecol. 45, 252–258. doi: 10.1007/s00248-002-1037-9
Sandler A., Herut B. (2000). Composition of clays along the continental shelf off Israel: contribution of the Nile versus local sources. Mar. Geol. 167, 339–354. doi: 10.1016/S0025-3227(00)00021-9
Scheller S., Yu H., Chadwick G. L., McGlynn S. E., Orphan V. J. (2016). Artificial electron acceptors decouple archaeal methane oxidation from sulfate reduction. Science 351, 703–707. doi: 10.1126/science.aad7154
Sela-Adler M., Herut B., Bar-Or I., Antler G., Eliani-Russak E., Levy E., et al. (2015). Geochemical evidence for biogenic methane production and consumption in the shallow sediments of the SE Mediterranean shelf (Israel). Continental Shelf Res. 101, 117–124. doi: 10.1016/j.csr.2015.04.001
Sivan O., Adler M., Pearson A., Gelman F., Bar-Or I., John S. G., et al. (2011). Geochemical evidence for iron-mediated anaerobic oxidation of methane. Limnol. Oceanogr. 56, 1536–1544. doi: 10.4319/lo.2011.56.4.1536
Sivan O., Antler G., Turchyn A. V., Marlow J. J., Orphan V. J. (2014). Iron oxides stimulate sulfate-driven anaerobic methane oxidation in seeps. Proc. Natl. Acad. Sci. U.S.A. 111, E4139–E4147. doi: 10.1073/pnas.1412269111
Sivan O., Schrag D., Murray R. (2007). Rates of methanogenesis and methanotrophy in deep-sea sediments. Geobiology 5, 141–151. doi: 10.1111/j.1472-4669.2007.00098.x
Sivan O., Shusta S., Valentine D. (2016). Methanogens rapidly transition from methane production to iron reduction. Geobiology 14, 190–203. doi: 10.1111/gbi.12172
Stookey L. L. (1970). Ferrozine–a new spectrophotometric reagent for iron. Analytical Chem. 42, 779–781. doi: 10.1021/ac60289a016
Su G., Zopfi J., Yao H., Steinle L., Niemann H., Lehmann M. F. (2019). Manganese/iron-supported sulfate-dependent anaerobic oxidation of methane by archaea in lake sediments. Limnol. Oceanogr. 65, 863–875. doi: 10.1002/lno.11354
Treude T., Krause S., Maltby J., Dale A. W., Coffin R., Hamdan L. J. (2014). Sulfate reduction and methane oxidation activity below the sulfate-methane transition zone in alaskan Beaufort Sea continental margin sediments: Implications for deep sulfur cycling. Geochim. Cosmochimica Acta 144, 217–237. doi: 10.1016/j.gca.2014.08.018
Valentine D. L. (2002). Biogeochemistry and microbial ecology of methane oxidation in anoxic environments: a review. Antonie Van Leeuwenhoek 81, 271–282. doi: 10.1023/A:1020587206351
Van Bodegom P. M., Scholten J. C., Stams A. J. (2004). Direct inhibition of methanogenesis by ferric iron. FEMS Microbiol. Ecol. 49, 261–268. doi: 10.1016/j.femsec.2004.03.017
Vigderovich H., Eckert W., Rubin-Blum M., Elul M., Elvert M., Sivan O. (2021). Potential electron acceptors for anaerobic methane oxidation during long-term incubations of lake sediments. Goldschmidt. doi: 10.7185/gold2021.5157
Vigderovich H., Liang L. W., Herut B., Wang F. P., Wurgaft E., Rubin-Blum M., et al. (2019). Evidence for microbial iron reduction in the methanic sediments of the oligotrophic southeastern Mediterranean continental shelf. Biogeosciences 16, 3165–3181. doi: 10.5194/bg-16-3165-2019
Wang Y., Wegener G., Hou J., Wang F., Xiao X. (2019). Expanding anaerobic alkane metabolism in the domain of archaea. Nat. Microbiol. 4, 595–602. doi: 10.1038/s41564-019-0364-2
Wegener G., Krukenberg V., Riedel D., Tegetmeyer H. E., Boetius A. (2015). Intercellular wiring enables electron transfer between methanotrophic archaea and bacteria. Nature 526, 587–590. doi: 10.1038/nature15733
Wurgaft E., Findlay A. J., Vigderovich H., Herut B., Sivan O. (2019). Sulfate reduction rates in the sediments of the Mediterranean continental shelf inferred from combined dissolved inorganic carbon and total alkalinity profiles. Mar. Chem. 211, 64–74. doi: 10.1016/j.marchem.2019.03.004
Yamada C., Kato S., Kimura S., Ishii M., Igarashi Y. (2014). Reduction of fe (III) oxides by phylogenetically and physiologically diverse thermophilic methanogens. FEMS Microbiol. Ecol. 89, 637–645. doi: 10.1111/1574-6941.12365
Yu H., Skennerton C. T., Chadwick G. L., Leu A. O., Aoki M., Tyson G. W., et al. (2022). Sulfate differentially stimulates but is not respired by diverse anaerobic methanotrophic archaea. ISME J. 16, 168–177. doi: 10.1038/s41396-021-01047-0
Zhang J., Dong H., Liu D., Agrawal A. (2013). Microbial reduction of fe (III) in smectite minerals by thermophilic methanogen methanothermobacter thermautotrophicus. Geochim. Cosmochim. Acta 106, 203–215. doi: 10.1016/j.gca.2012.12.031
Zhang J., Dong H., Liu D., Fischer T. B., Wang S., Huang L. (2012). Microbial reduction of fe (III) in illite–smectite minerals by methanogen methanosarcina mazei. Chem. Geol. 292, 35–44. doi: 10.1016/j.chemgeo.2011.11.003
Zhang X., Fan W. Y., Yao M. C., Yang C. W., Sheng G. P. (2020). Redox state of microbial extracellular polymeric substances regulates reduction of selenite to elemental selenium accompanying with enhancing microbial detoxification in aquatic environments. Water Res. 172, 115538. doi: 10.1016/j.watres.2020.115538
Keywords: AOM, iron, sulfate, sediments, S isotopes
Citation: Yorshansky O, Liang L, Pellerin A, Wang F, Herut B and Sivan O (2022) Iron oxides impact sulfate-driven anaerobic oxidation of methane in diffusion-dominated marine sediments. Front. Mar. Sci. 9:903918. doi: 10.3389/fmars.2022.903918
Received: 24 March 2022; Accepted: 16 August 2022;
Published: 15 September 2022.
Edited by:
Itay Halevy, Weizmann Institute of Science, IsraelReviewed by:
Peter Kraal, Royal Netherlands Institute for Sea Research (NIOZ), NetherlandsCopyright © 2022 Yorshansky, Liang, Pellerin, Wang, Herut and Sivan. This is an open-access article distributed under the terms of the Creative Commons Attribution License (CC BY). The use, distribution or reproduction in other forums is permitted, provided the original author(s) and the copyright owner(s) are credited and that the original publication in this journal is cited, in accordance with accepted academic practice. No use, distribution or reproduction is permitted which does not comply with these terms.
*Correspondence: Orit Sivan, b3JpdHNpQGJndS5hYy5pbA==
Disclaimer: All claims expressed in this article are solely those of the authors and do not necessarily represent those of their affiliated organizations, or those of the publisher, the editors and the reviewers. Any product that may be evaluated in this article or claim that may be made by its manufacturer is not guaranteed or endorsed by the publisher.
Research integrity at Frontiers
Learn more about the work of our research integrity team to safeguard the quality of each article we publish.