- Univ Brest, Ifremer, CNRS, Unité Biologie des Environnements Extrêmes marins Profonds, F-29280, Plouzané, France
The shrimp Rimicaris exoculata and Rimicaris chacei are visually dominant fauna co-occurring at deep-sea hydrothermal sites of the Mid-Atlantic Ridge (MAR). Their co-existence was related to contrasted life-history traits, including differences in their diet and reliance on chemoautotrophic symbionts at the adult stage. Both species of shrimp are colonized by diversified chemosynthetic symbiotic microbial communities in their cephalothoracic cavity. Symbiotic association with bacteria was also evidenced in their digestive system, and the major lineages were identified through sequencing (with Mycoplasmatales in the foregut and Deferribacteres in the midgut) but their precise distribution within each host species was not assessed. For the first time, we used Fluorescence in situ Hybridization (FISH) to visualize these lineages and describe their association with digestive structures of their host. The aim of the study was to identify possible differences between host species that could be related to their different life-history traits. For this purpose, we developed new specific FISH probes targeting Deferribacteres and Mycoplasmatales lineages identified in the digestive system of these shrimp. Our FISH results showed a partitioning of the bacterial lineages according to the digestive organ corroborating sequencing data, and highlighted their association with specific anatomical structures. Despite morphological differences between the foreguts of R. exoculata and R. chacei that could be related to the adult diet, our FISH results showed overall similar distribution of digestive symbionts for the two host species. However, a more comprehensive study is needed with specimens at different life or molt stages to reveal potential host specific patterns. Such comparisons are now possible thanks to our newly designed FISH probes. The tools used in our study are valuable for tracking symbiont lineages in the environment, allowing a better understanding of their relationship with their host along its life cycle, including their acquisition mechanisms.
Introduction
Living communities thriving at deep-sea hydrothermal vents are fueled by chemosynthesis performed by microorganisms that are either free-living or forming associations with protists or metazoan hosts (Kouris et al., 2007; Dubilier et al., 2008; Sogin et al., 2021). These associations are called holobionts (Zilber-Rosenberg and Rosenberg, 2008) and dominate vent biomass near fluid exits, such as Rimicaris caridean shrimp along the Mid-Atlantic Ridge (MAR).
Rimicaris exoculata and Rimicaris chacei (Williams and Rona, 1986) co-occur at the active vents of many MAR sites (Zbinden and Cambon Bonavita, 2020) (Figure 1A). R. exoculata live in dense aggregations near the emitted fluids, and are therefore exposed to high temperatures and high concentrations of toxic compounds such as heavy metals or minerals (Schmidt et al., 2008a; Schmidt et al., 2008b) (Figure 1B). Juveniles of R. exoculata also occur within these aggregations or adjacent to them (Hernández-Ávila et al., 2021; Methou et al., 2022). R. chacei shrimp live nearby R. exoculata aggregations, sometimes observed hiding in crevices under rocks, or behind mussels (Methou et al., 2022). They can occasionally form small aggregations. However, R. chacei adults are much less abundant than R. exoculata adults, while numerous juveniles of R. chacei live in nurseries at TAG and Snake Pit (Methou et al., 2020; Hernández-Ávila et al., 2021) suggesting that the relatively low adult number results from a population collapse during the recruitment process of this species (Methou et al., 2022). Such a drop in abundance between life stages does not seem to exist for R. exoculata, and raises questions on the possible underlying mechanisms. The acquisition of symbiotic communities throughout each species’s recruitment process may play a major role in holobiont fitness (Methou et al., 2022).
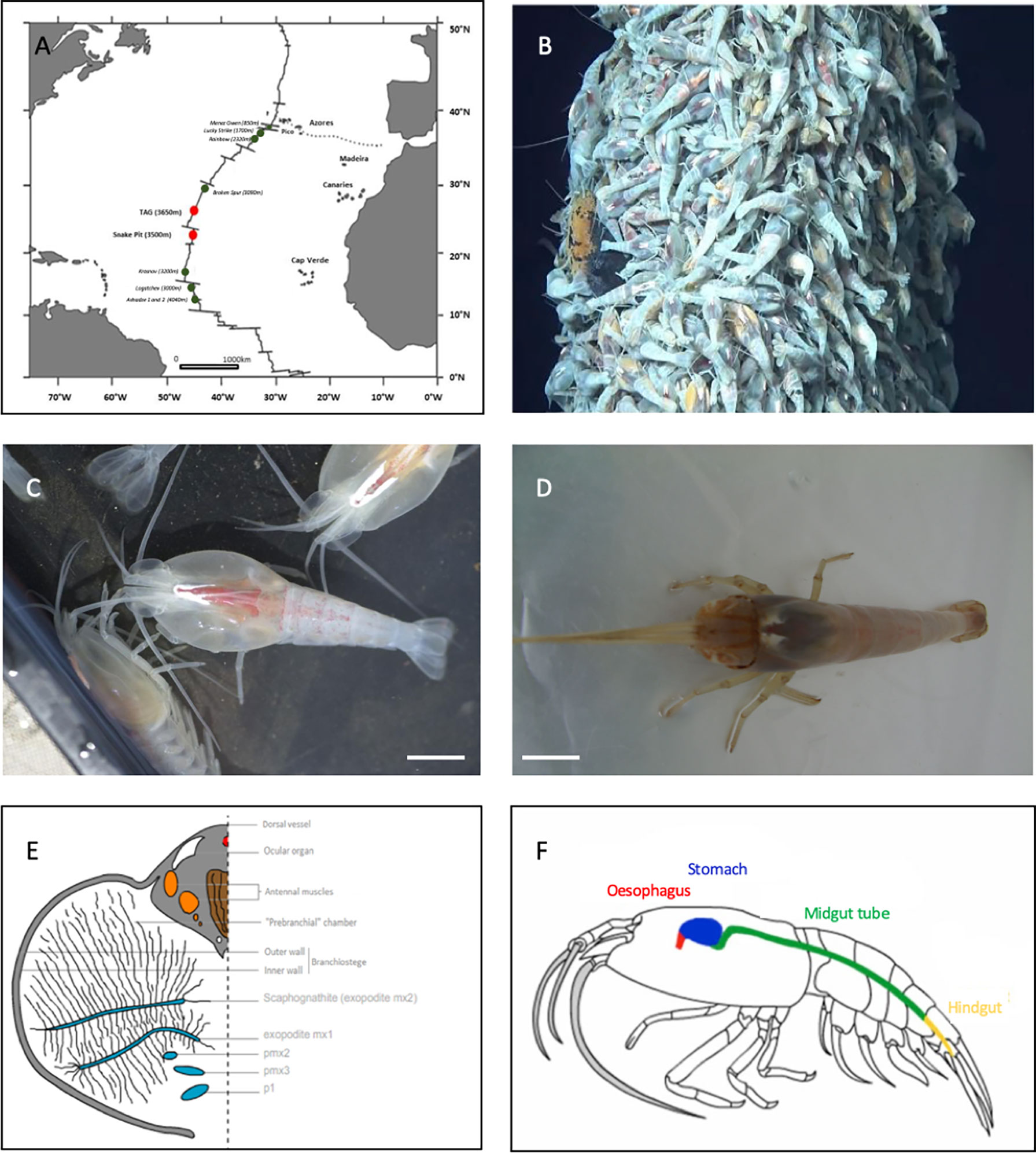
Figure 1 Study models. (A) Two main study sites on the MAR: TAG and Snake Pit. (B) Rimicaris exoculata aggregations on a hydrothermal vent at the TAG site (Nautile-BICOSE2, 2018). (C) Adult Rimicaris exoculata. (D) Adult Rimicaris chacei. (E) Transversal section of half of the cephalothoracic cavity of R. exoculata (adapted from Segonzac et al., 1993). (F) Digestive system of Rimicaris spp. (adapted from Durand et al., 2009).
In adulthood, R. exoculata stands out from other vent shrimp through its enlarged cephalothoracic cavity (Figure 1C), enclosing hypertrophied mouthparts (scaphognathites and exopodites) (Van Dover et al., 1988; Segonzac et al., 1993; Komaï and Segonzac, 2008) (Figures 1C, E). In contrast, R. chacei have a narrower cephalothoracic cavity with the first two pairs of chelipeds remaining visible and functional (Casanova et al., 1993; Segonzac et al., 1993) as well as less hypertrophied mouthparts than R. exoculata (Apremont et al., 2018) (Figure 1D). These morphological differences may be related to the level of development of the symbiotic bacterial communities, where more abundant communities are observed along with hypertrophied head organs of R. exoculata (Segonzac et al., 1993). This cephalothoracic community is dominated by Campylobacteria and Gammaproteobacteria together with other lineages (between 10-30% depending on specimens) (for review see Zbinden and Cambon, 2020).
These microbial mats are cleared, together with the minerals and the cuticle at each molt event (every 10 days for R. exoculata – Corbari et al., 2008). In R. exoculata, these symbiotic communities play a major trophic role through direct transcuticular transfer of chemosynthetic organic matter to their host (Ponsard et al., 2013). However, chelipeds of R. chacei are functional which, together with isotopic data, suggest a mixotrophic regime based on bacteriotrophy, scavenging and symbiosis (Casanova et al., 1993; Apremont et al., 2018).
Rimicaris shrimp also host microbial communities in their digestive system, but the lack of detailed anatomical knowledge prevents a good understanding of the morphological and functional relationships between microbes and their host. The description of the digestive system anatomy has to be better documented. As in other decapods (Vogt, 2021), the digestive system of R. exoculata and R. chacei comprises three regions: the foregut, the midgut and the hindgut (Figure 1F). In farmed shrimp (such as Penaeus monodon orMacrobrachium carcinus), the foregut is a complex organ because of its numerous internal features allowing crushing and filtration of digested particles (Ceccaldi, 1989; Lima et al., 2016; Štrus et al., 2019). The foregut comprises the œsophagus and the stomach, while the hindgut is the terminal excretion zone located at the end of the abdomen (Vogt, 2021). These two regions have an ectodermic origin and are lined by a cuticle, which is exuviated during the molt. The third region linking the two others is the midgut, comprising the hepatopancreas and the midgut tube (Vogt, 2021). Contrary to the hindgut or the foregut, the midgut is devoid of a cuticle due to its endodermic origin, and therefore does not molt.
The digestive system of R. exoculata seems modified in comparison to other crustaceans (Ceccaldi, 1989; Komai and Segonzac, 2008; Lima et al., 2016). The foregut and hindgut are reduced (the stomach being a single and rather small cavity), while the midgut tube is long (Segonzac et al., 1993; Komaï and Segonzac, 2008; Durand et al., 2009) (Figure 1F). In contrast, the stomach of R. chacei is larger and looks similar to that of other caridean shrimp (Segonzac et al., 1993; Komai and Segonzac, 2008; Apremont et al., 2018). This is in agreement with a mixotrophic diet involving both symbiosis and scavenging (Casanova et al., 1993; Segonzac et al., 1993; Gebruk et al., 1997; Gebruk et al., 2000; Apremont et al., 2018; Methou et al., 2020). The alimentary bolus of R. exoculata, i.e. particles ingested by the mouth, passing through the foregut and midgut tube and expulsed by the hindgut as feces after digestion processes, is mainly composed of minerals: iron sulphides and oxides, phosphate and calcium sulphate at different stages of oxidation, as well as some cuticle debris from molts (Segonzac et al., 1993). The alimentary bolus of R. chacei seems to differ: in addition to minerals and cuticle debris, organic waste and debris can be found (Casanova et al., 1993; Apremont et al., 2018).
Using 16S rDNA approaches (PCR-cloning), two microbial communities were identified in the digestive system of both shrimp species (Figure 1F) (Durand et al., 2009; Durand et al., 2015; Apremont et al., 2018). The foregut community is mainly composed of Mycoplasmatales (Firmicutes-Bacilli) while Deferribacteres are housed in the midgut tube. Fluorescent in situ Hybridization (FISH), Scanning Electron Microscopy (SEM) and Transmission Electron Microscopy (TEM) approaches have been used to observe the symbiont lineages. In the midgut tube, long thin “spaghetti-like” bacterial cells inserted between the microvilli of epithelial cells were observed but could not be affiliated to any bacterial lineages identified through sequencing as only the universal bacterial probe Eub338 gave a positive FISH signal (Durand et al., 2009; Durand et al., 2015; Apremont et al., 2018). Morphologically, they do not resemble described Deferribacteres species, which are usually small curved rods (Garrity et al., 2001). The closest relative of Deferribacteres lineage from the midgut tube of Rimicaris spp. is Mucispirillum schaedleri, isolated from rodent digestive mucus layer (Robertson et al., 2005). The location of the Mycoplasmatales is still unclear. Mycoplasmatales are heterotrophic wall-less bacterial cells usually having a reduced genome (Tully et al., 1993). The closest relatives of Rimicaris spp. Mycoplasmatales were identified in the hepatopancreas of the terrestrial isopod Porcellio scaber, appearing mostly as amorphous coccoid cells (Wang et al., 2004). In R. exoculata, the different microbial lineages retrieved in the digestive system seem to be resident symbionts still present after 72 hours of fasting, which empties the digestive system (Durand et al., 2009). However, microbial communities in the foregut must be cleared out regularly due to molt, contrary to those in the midgut tube. Inter-molt and inter-generation transmission of lineages affiliated to Mycoplasmatales and Deferribacteres remain enigmatic, as are their potential roles. Only one Mycoplasmatales affiliated OTU, but no Deferribacteres (Hügler et al., 2010; Flores et al., 2011; Durand et al., 2015; Cowart et al., 2017) was identified from the shrimp’s environment. The level of similarity between Deferribacteres 16S rDNA gene sequences, regardless of sampling site, is higher than 99%, suggesting a single phylotype, which suggests a vertical transmission (Durand et al., 2015). However, this lineage was not found in microbial communities associated with broods (Guri et al., 2012; Cowart et al., 2017; Methou et al., 2019), which would then rather indicate horizontal transmission.
The key questions related with the acquisition of symbionts at the juvenile stage and after each molt (transmission mode, key moments of acquisition, symbiotic evolution, relation between symbiosis and recruitment…), as well as the overall functioning of the digestive system and symbiont niche distribution in Rimicaris spp. are debated. To study acquisition and to infer their potential roles in the overall functioning of holobionts, it is first necessary to decipher symbiont niche distribution in the digestive system of adult shrimp. For this purpose, we first described Rimicaris spp. foregut anatomy through binocular microscopy and Scanning Electronic Microscopy (SEM). Then, we used fluorescent in situ hybridization (FISH) approaches with newly designed molecular probes targeting specific rRNA sequences of these digestive symbiotic lineages. These approaches allow both unaltered symbiotic cell visualization (morphology and localization on host organ/cells) and phylogenetic identification (Amann et al., 1990; Amann et al., 2001). To date, in situ observations of Deferribacteres and Mycoplasmatales lineages in Rimicaris spp. tissues have failed, as no specific molecular probe except the universal Eub338 bacterial probe has ever given a positive signal (Durand et al., 2009; Durand et al., 2015).
The combination of FISH approaches with SEM imaging of the digestive structures of both shrimp species was then used to answer the following questions: 1) What is the distribution and abundance of digestive symbiotic lineages in each Rimicaris species? 2) Is each symbiont lineage associated with a specific digestive structure in each host, possibly reflecting organ partitioning? 3) Can symbiont distribution and abundance in their respective host be related to specific ecological traits of the holobionts (e.g. nutrition type)?
Materials and Methods
Sampling and Specimen Processing on Board
Samples were collected at two vent fields along the MAR: TAG (26°8 “N-44°50 “W, 3650 m depth) and Snake Pit (23°22 ‘‘N; 44°57’’W, 3460 m depth) during the BICOSE2 cruise (26 January to 10 March 2018, DOI http://dx.doi.org/10.17600/18000004). The specimens were caught in shrimp aggregations using the suction sampler of the HOV (Human Operated Vehicle) Nautile operated from the R/V Pourquoi pas?
Once on board, shrimp were dissected under sterile conditions to recover different anatomical parts: the branchiostegites (LB) and the scaphognathites (Sc) in the cephalothoracic cavity, and the digestive system comprising the foregut and the midgut tube. The different organs were immediately fixed for FISH studies in a 3% formalin seawater solution for 3 hours to keep cell integrity. Samples were then rinsed three times with a phosphate buffered saline solution (PBS) and stored in a PBS/Ethanol (1:1) solution at -20°C (Durand et al., 2009). Some digestive tissues were fixed in a 2.5% glutaraldehyde solution (16 hours at 4°C), and then rinsed and stored at 4°C in a buffered solution containing a biocide to avoid bacterial development [filtrated seawater with 0,44 g/L of NaN3 at pH 7.4] until use for scanning electron microscopy (SEM) observations. In addition, whole shrimp specimens were frozen at -80°C for later dissections at the laboratory (Table 1).

Table 1 Samples selected for the different approaches: SEM, FISH and dissection under binocular microscope.
In Silico Design and Validation of New FISH Specific Probes
The FISH method is based on the use of specific molecular probes linked to a fluorescent dye, which are complementary to a region of the 16S or 23S rRNA target molecule, directly inside the native ribosomes of fixed microbial cells. We developed new probes that specifically target Mycoplasmatales symbiont lineages and the single Deferribacteres Rimicaris symbiont lineage found in Rimicaris (Durand et al., 2009; Durand et al., 2015). These probes were first designed in silico, based on the Rimicaris symbiont 16S rDNA sequences, obtained by PCR cloning approaches (Durand et al., 2009; Durand et al., 2015; Apremont et al., 2018). These sequences were aligned with the MUSCLE (MUltiple Sequence Comparison by Log- Expectation) algorithm (Edgar, 2004) in Geneious software v9 (Kearse et al., 2012). This software can be used to highlight homology and evolutionary relationships between sequences and thus, to select rRNA molecule regions specific to the digestive symbiont lineages. Literature data (Behrens et al., 2003) were used to select 16S rRNA molecule regions known to be accessible for probe hybridization. First, the 2D conformation of the rRNA was used to select potential zones for probe hybridization and to assess the potential for co-hybridization between two probes. The potential accessible regions are chosen to avoid 3D in situ conformations of the rRNA molecule or regions showing interactions with ribosomal proteins. Then, the physical properties of the designed probes were estimated to avoid hairpin structure formation, cross-hybridization, self-dimer formation, and to check their melting temperatures (TM), using the Geneious Primer Design tool and the Oligo Calc software (Kibbe, 2007). Finally, the complementarity of these designed probes with rRNA gene sequences of non-targeted microorganisms was evaluated more broadly using BLAST (Altschul et al., 1990) with the Silva138 database (test probes v3.0).
Sample Preparation for Fluorescence In Situ Hybridization Procedures
Tissue sections were prepared by embedding dissected organs (foregut, midgut tube and scaphognathites) in polyethylene glycol distearate-1-hexadecanol (9: 1) resin (Sigma, St., Louis, MO) after progressive dehydration and immersion series (PBS – ethanol series at ambient temperature and ethanol – resin series at 40°C) (Duperron et al., 2007). After resin polymerization, blocks containing organs were stored at -20°C until trimming. Depending on the sample, 8-10 µm transversal tissue sections were obtained with a RM 2255 microtome (Leica Biosystems, Nussloch, Germany) and placed on slides (Menzel-Gläser Superfrost® Plus, USA). Before hybridization, resin was removed with ethanol (3x5min in 96% ethanol) and tissues were rehydrated (5 min in 70% ethanol).
Determination of Optimal Hybridization Conditions for New FISH Probes
After in silico validation, optimal probe hybridization conditions were determined using host tissues known to harbour (e.g. digestive) or lack (e.g. scaphognathites) these target symbiont lineages (Table 1). GAM42a targeting Gammaproteobacteria (Manz et al., 1992) and Epsy549 targeting Campylobacteria (Lin et al., 2006) were used as positive controls on scaphognathites (Table 2). The universal probe Eub338-I targeting most Eubacteria (Amann et al., 1990) was used as a general positive co-hybridization control (Table 2). All probes were synthesized by Eurofins Genomics (Ebersberg, Germany) and were labelled with either Cyanine 3 or Cyanine 5 dyes (Table 2).
Appropriate stringency conditions were determined using various combinations of 1) formamide concentrations in the hybridization buffer and 2) hybridization and washing temperatures, both of which act to denature, without altering, the ribosome structure, enabling effective probe hybridization. Sections were immersed in reaction mix containing probes (each at 0.5µM final concentration) in a hybridization buffer [0.9M NaCl, 0.02M Tris-HCl [pH 7.5], 0.01% [w/v] sodium dodecyl sulphate (SDS), 20%, 30%, 35%, 40%, 45% or 50% deionized formamide (Table 3)], and incubated for 3 hours at 46°C or 48°C (Table 3). Sections were briefly pre-rinsed and then were washed for 15 min or 30 min, at a slightly higher temperature (48°C or 50°C) than the hybridization temperature applied (Table 3), in a washing buffer adapted to the formamide concentrations [0.215M, 0.102M, 0.07M, 0.046M, 0.03M or 0.018M NaCl respectively for 20%, 30%, 35%, 40%, 45% or 50% formamide, 0.02M Tris-HCl [pH 7.5], 0.005M EDTA [pH 8] and 0.01% [w/v] SDS]. Then, they were briefly rinsed twice with distilled water, once at washing temperature then at room temperature. Finally, sections were mounted on slides with SlowFade™ Gold antifade reagent with DAPI (Invitrogen). Observations on hybridized tissues were made using a Zeiss Imager.Z2 microscope equipped with the Apotome.2® sliding module and Colibri.7 light technology (Zeiss, Oberkochen, Germany). The micrographs were analyzed using the Zen software (Zeiss). Differential Interference contrast (DIC) was used to better visualize the host tissues.
Scanning Electron Microscopy
Samples fixed in glutaraldehyde were first dehydrated in ethanol series (10% to 100% in 8 steps). Dehydrated samples were placed in a perforated box, critical-point dried (Leica EM CPD300), affixed to a stub using carbon glue and then coated by gold-sputtering (60% gold/40% Palladium, Quorum Technologies SC7640). SEM observations were performed using a Quanta 200 MK microscope (FEI, Hillsboro, OR) and images were taken with the SCANDIUM acquisition program (Soft Imaging System, Munster, Germany).
Results
Probe Specificity and Optimal Hybridization
Three probes targeting Mycoplasmatales lineages of the two Rimicaris species and one probe targeting the Deferribacteres lineage were designed. To ensure their specificity, stringency tests (variation of the formamide percentage in the hybridization buffer, variation of hybridization and washing temperatures) were performed on specific organs: foreguts, midgut tube and scaphognathites of R. exoculata adults. Scaphognathites were used as a control for non-specific hybridization, as Deferribacteres or Mycoplasmatales were never detected on these structures using DNA sequencing approaches.
According to these tests, one specific probe covering the diversity of Mycoplasmatales lineages was retained: Myco378-1 (5’GTGGAAAATTCCCTACTGCTG’3) (see Supplementary Material; Supplementary Figures 1, 2). The optimal conditions to ensure its best specificity are: hybridization at 46°C for 3 hours with a buffer hybridization containing 45% formamide, washing at 48°C for 30 minutes (see Supplementary Material; Supplementary Table 1). The probe Def1229 (5’GCCCTCTGTATAGTCCATTG’3) targeting Deferribacteres (see Supplementary Material; Supplementary Figures 3–6) optimal conditions of use are: hybridization at 46°C for 3 hours with a buffer hybridization containing 30% formamide, washing at 48°C for 15 minutes (see Supplementary Material; Supplementary Table 2).
Description of Foregut Structure
In R. exoculata and R. chacei, the stomach is surrounded with the hepatopancreas that appears as an amorphous and oily gland, cream/orange in colour. In our observations, the digestive system differed in size between our two species. In R. exoculata, the midgut tube was very long, while the foregut was small and quite flexible. The œsophagus was very short. The stomach appeared as a single cavity that could be mostly black-colored when containing minerals. In contrast, R. chacei adults had a more rigid and voluminous foregut, closer to that of other shrimp (Vogt, 2021), appearing mostly brown in colour. The midgut tube of R. chacei was also relatively shorter than that of R. exoculata. To date, the foregut of these two species have only been partially described (Segonzac et al., 1993; Apremont et al., 2018), and a more detailed analysis is required prior to characterizing niche colonization by microorganisms. Due to sample limitation, a complete description is given for R. exoculata adults only (Figure 2), done by comparison with recent descriptions in the caridean shrimp Macrobrachium carnicus and other crustaceans (Lima et al., 2016; Vogt, 2021):
A) Global structure (Figure 2A): The foregut is located dorsally in the cephalothorax (Figure 1F). Like that of M. carcinus (Lima et al., 2016), the overall structure seems reduced to a single cavity (Figure 2B). The dorsal side presents a bulge, while the ventral side is convex lengthwise. The anterior part of the stomach is connected to the œsophagus. Posteriorly, the stomach connects to the midgut tube, which dips ventrally slightly before rising to reach the dorsal side of the abdomen. The foregut is sometimes difficult to locate because of its small size and concealment in the glandular mass of the hepatopancreas. The hepatopancreas is connected to the stomach ventrally, surrounding the posterior-ventral part of the stomach and ascending towards its dorsal side.
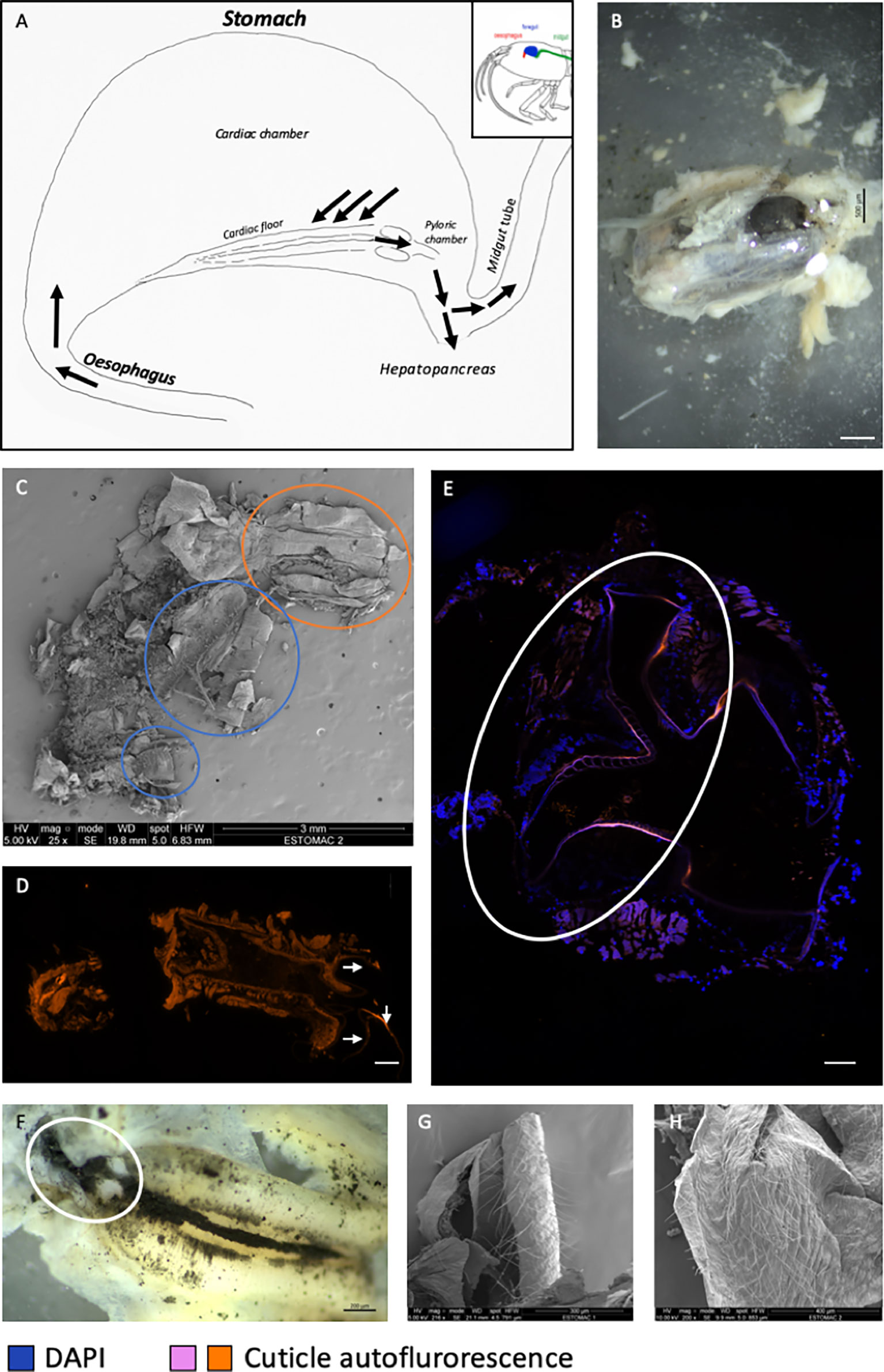
Figure 2 General anatomy of the foregut of a R. exoculata adult. (A) Drawing of the general shape of a foregut of R. exoculata adult (see Figure 1F). The black arrows indicate the path of the ingested materials through the stomach. (B) Stomach (black colored) dissected from the cephalothorax surrounded by hepatopancreas (white colored) of R. exoculata (binocular microscope, scale bar = 500 µm). (C) Opened critical-point dried foregut revealing its content (SEM, scale bar = 3 mm). The orange circle shows the œsophagus and the blue circles show the cardiac floor sieve, which is broken in two parts. (D) Photography of the transversal section of an œsophagus observed by autofluorescence in orange with 548 wavelength (image mosaic from 5734 x 11264 µm sections, scale bar = 20 µm). The white arrows show the ossicles where setae are located. (E) Transversal section of the pyloric chamber observed by autofluorescence in pink with 548 wavelength, image mosaic from 7577 x 7577 µm sections, blue DAPI staining showing host cell nucleus (image mosaic from DIC, Apotome®, scale bar = 20 µm). The circle encloses the different plates and setae – view in transverse section- present in the pyloric chamber. (F) Cardiac floor sieve, with the different setae and minerals (binocular microscope, scale bar = 200 µm). The circle corresponds to the probable reduced pyloric chamber. (G, H) Inner part of the cardiac chamber showing thin and long setae (SEM). Scale bar = 300 µm (E); Scale bar = 400 µm (F).
B) Oesophagus (Figure 2A): This structure is located anteriorly of the stomach. It is a short, curved and overall narrow structure, which dips slightly from the anterior part of the stomach to the shrimp’s mouth (Figures 2C, D). The œsophagus is lined with a thin cuticle and is surrounded with muscles. The internal cuticle of the ventral side is covered with numerous long, thin setae (here called “needles”).
C) Internal structure (Figure 2A): The stomach is composed of two chambers - appearing mostly like those of the shrimp M. carcinus - called the “cardiac sac or chamber”, and the “pyloric chamber” (Figures 2C–F). The cardiac chamber is the larger of the two. It extends laterally towards the posterior part of the stomach and covers the entire pyloric chamber. The latter, which is much less voluminous and hardly visible, is located behind the cardiac floor of the cardiac chamber, in the curved ventral zone of the stomach.
D) Cardiac chamber (Figure 2A): This is a “simple” structure of the stomach of R. exoculata, which actually comprises most its volume (Figure 2B). Its walls are made of striated muscles lined with a cuticle appearing thinner than usually observed in other shrimp. This cuticle is covered with spicules and long thin setae (Figures 2G, H). This chamber contains the shrimp’s alimentary bolus consisting of food items coming directly from the œsophagus (Figure 2C). The cardiac chamber contracts and relaxes thanks to its numerous individual muscles that allow movements of the gastric mill and press plates of the pyloric chamber (Vogt, 2021).
E) Cardiac floor (Figure 2A): The cardiac floor is the ventral part of the cardiac chamber. It is easily recognizable because of its “bulbous” shape when viewed dorsally (Figure 2F). This floor is made of a combination of ossicles and setae, which give it a curved and thick shape, forming the cardiac floor sieve. The different structures that form the cardiac floor (according to the nomenclature of Lima et al., 2016) are the following (Figure 3) (starting from the outermost to the innermost part of the structure).
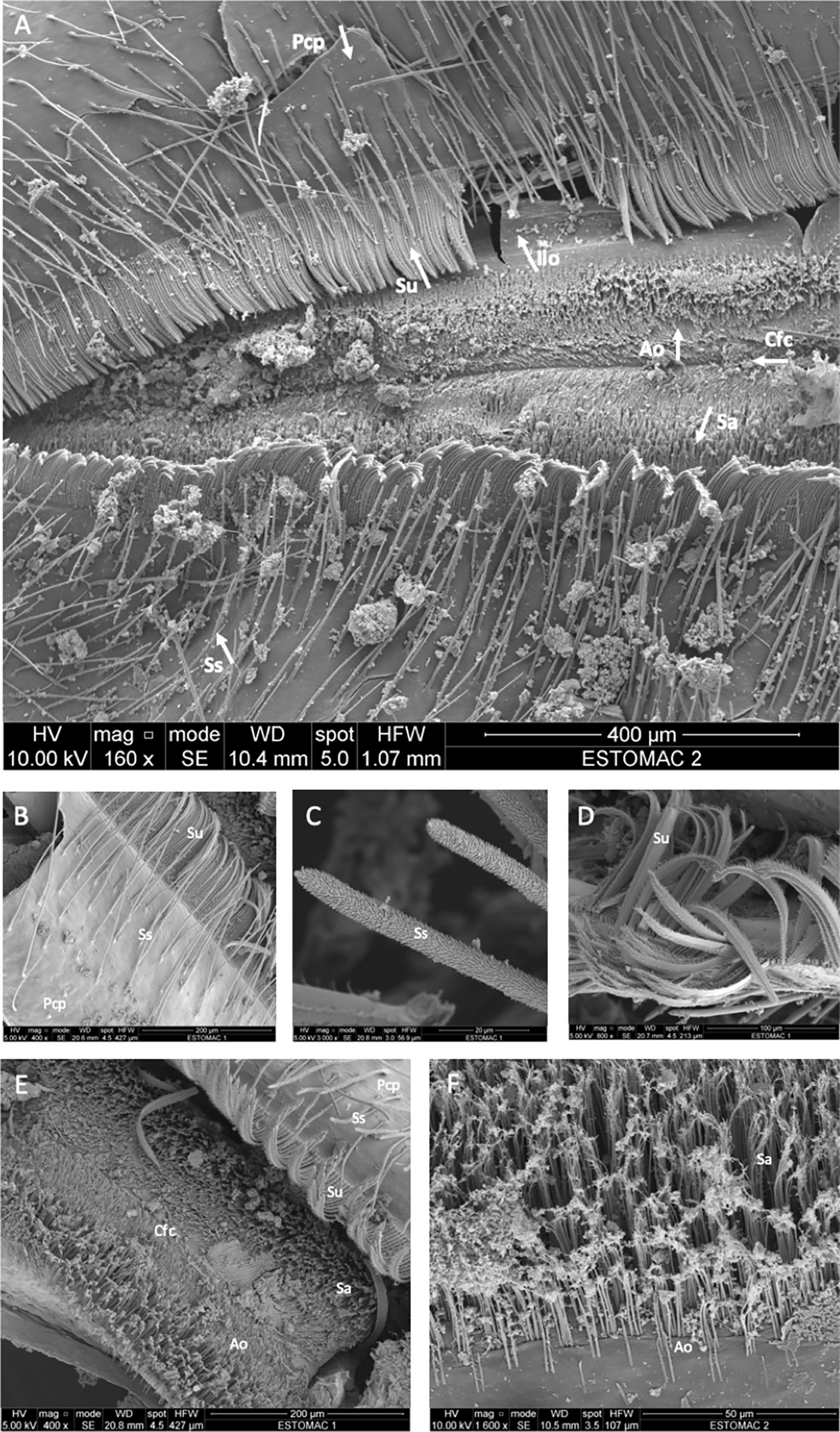
Figure 3 Structure of the cardiac floor of a R. exoculata adult observed with scanning electon microscopy. (A) Dorsal view of the cardiac floor sieve showing the different ossicles and setae (scale bar = 400 µm). (B) The posterior cardiac plate with simple and serrulate setae (scale bar = 200 µm). (C) Simple setae of the posterior cardiac plate (scale bar = 20 µm). (D) Serrulate setae of the posterior cardiac plate (scale bar = 100 µm). (E) Closer view of the anterior ossicle with its setae, minerals along the surface and the cardiac floor crest on the center (scale bar = 200 µm). (F) The different setae of the anterior ossicle (scale bar = 50 µm). Pcp, Posterior cardiac plate; Ss, Simple setae; Su, Serrulate setae; Ilo, Inferior lateral ossicle; Ao, Anterior ossicle; Sa, Setae of the anterior ossicle with minerals; Cfc, cardiac floor crest.
A pair of posterior cardiac plates (Figures 3A, B). On these two plates, long setae are visible. These are simple, thick setae, but not dense (Figures 3A–C). Along the central edge of these two plates, numerous serrulate setae (Figures 3A, B) form a thick barrier (called sieve) between the internal structure of the cardiac floor and the two plates. These setae are thick, curved and are themselves covered with small, thin setae (Figure 3D). By comparison, these serrulate setae are reminiscent of the setae of the scaphognathites and exopodites of R. exoculata (Figure 3D). These setae cover the paired inferior lateral ossicles (Figures 3A, E) identified as the cardio-pyloric valve (Lima et al., 2016), and may have a role in filtering and transporting resources to the midgut (hepatopancreas and midgut tube).
-The unpaired anterior ossicle (of the cardio-pyloric valve (Lima et al., 2016)) is located between the pair of inferior lateral ossicles, and is visible between the serrulate setae of the posterior cardiac plates. This structure is a thin triangular calcified plate, covered with simple setae (Figures 3A, E, F). These setae are very thin and much shorter than those found on posterior cardiac plates. They are covered with the minerals and materials contained in the shrimp’s alimentary bolus, suggesting a role in filtration, which sometimes makes them difficult to distinguish (Figure 3F).
-In the center of the anterior ossicle, a longitudinal groove is visible (Figures 3A, E). This is the cardiac floor crest, in which the residues of the alimentary bolus accumulate. The residues are brought to the pyloric chamber through this groove. The various and numerous setae allow the passage of nutrient and mineral residues to the pyloric chamber and then to the midgut (hepatopancreas and midgut tube).
F) Pyloric chamber (Figure 2A; Supplementary Figure 7): This structure is considerably reduced and hardly visible in the stomach of adult R. exoculata. It is a very small chamber that connects the cardiac floor to the midgut tube and is probably also connected to the hepatopancreas (Figures 2A, E, F), but this last connection was not properly observed here due to sample limitation. By analogy to what is described for omnivorous shrimp, and according to anatomical similarities, the cardiac floor sieve separates the cardiac sac and the pyloric chamber. The cuticle of the pyloric chamber appears to be very curved and covered with dense setae that are folded on themselves (Figure 2E; Supplementary Figure 7). These setae are probably involved in filtration activity (see Štrus et al., 2019).
The structure of the foregut of R. exoculata strongly resembles that of M. carcinus shrimp (Lima et al., 2016) but is much smaller. In comparison, the foregut of the single available R. chacei specimen observed under the binocular microscope is larger than that of R. exoculata, with a more developed pyloric chamber, but full description is needed with new specimens.
Distribution of Mycoplasmatales and Deferribacteres in the Digestive Tract of Rimicaris spp.
Mycoplasmatales in the Foregut of R. Exoculata and R. Chacei
Mycoplasmatales are wall-less bacterial cells with a wide morphological spectrum (from rod to coccoid) (Tully et al., 1993; Wang et al., 2004) that make their visual identification challenging. The use of the Myco378-1 probe revealed for the first time their morphology in both Rimicaris spp. with FISH approaches (Figures 4A–F). To improve symbiont description in terms of both morphology and distribution on host tissues, many foregut sections were examined from the œsophagus to the posterior end. Depending on the foregut sample, section location in the organ, fixation quality (directly on board or fixed at the laboratory after freezing), or molt stage (cuticle being more or less present and thick), the Mycoplasmatales were not always clearly observable (Figure 4C).
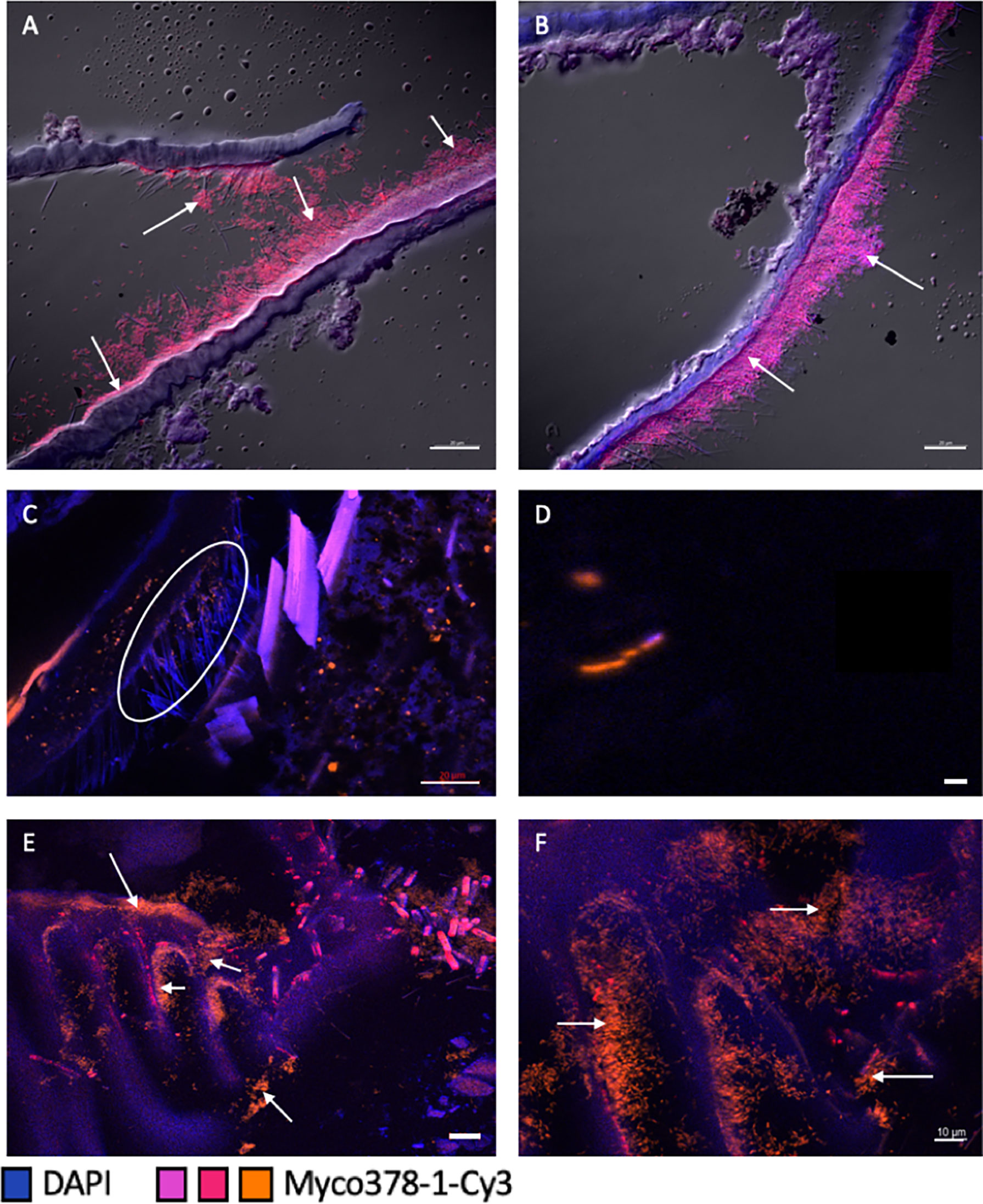
Figure 4 Presence of Mycoplasmatales in the foregut of R. exoculata and R. chacei adults. (A–D) represent sections through the foregut [œsophagus for (A, B), pyloric chamber for (C, D)] of a R. exoculata adult hybridized with Myco378-1-Cy3 (pink in (A, B) or orange in (C, D). Tissue cell nuclei are labeled with DAPI (blue). Formamide concentration in hybridization buffer was 45% (A–D). Images (A–D) were taken with Apotome®, and DIC for (A, B). The white circle (C) highlights the Mycoplasmatales hidden by the setae. (E, F) represent sections through the foregut (pyloric chamber) of a R. chacei adult hybridized with Myco378-1-Cy3 (orange). Tissue cell nuclei are labeled with DAPI (blue). White arrows are targeting some Mycoplasmatales observed on the different structures. Formamide concentration in hybridization buffer was 45%. Pictures (E, F) were taken with Apotome®. Scale bars = 20 µm (A–C, E); Scale bar = 1 µm (D); Scale bar = 10 µm (F).
For both holobionts, Mycoplasmatales were observed as rod-shaped cells. Many divided rod-cells were observed, suggesting cell division and therefore an active community (Figure 4D). In the different specimens available (two R. chacei and eighteen R. exoculata), symbionts were observed in several areas of the foregut. First, many Mycoplasmatales were found in the œsophagus (Figures 4A, B) where symbiont accumulated as thick mats along the thin setae. The same rod morphologies were observed in the pyloric chamber in dense colonies for both Rimicaris spp. (Figures 4C, E, F). Notably, Mycoplasmatales colonies were much denser in the two R. chacei specimens, mostly on setae in the pyloric chamber (Figures 4E, F). In contrast, no Mycoplasmatales was observed in the alimentary bolus, nor along the walls of the cardiac sac (Figures 4C, E, F). Furthermore, none was evidenced at the entrance to the midgut tube. Only one adult R. exoculata empty foregut (i.e. without minerals) could be observed. In this specimen, Mycoplasmatales were visible on the setae of the pyloric chamber and in in the lumen of the stomach (probably detached from their original place). Myco378-1 gave no positive signal on any of the midgut tube sections of adult R. exoculata. No fluorescence hybridization signal was observed on “spaghetti-like” filaments or in the alimentary bolus (Supplementary Table 2).
Deferribacteres in the Midgut Tube of R. Exoculata and R. Chacei
Based on previous DNA sequencing data, Deferribacteres were expected to be localized in the midgut tube of R. exoculata and R. chacei (Durand et al., 2009; Durand et al., 2015; Apremont et al., 2018). FISH observations of the midgut tube sections using the specific probe Def1229 allowed us to validate the presence of these bacteria and to identify them clearly. Labeled bacteria appeared with the same morphology as previously observed with FISH using the general Eub338 probe (Durand et al., 2009) (Figure 5A), confirmed by the use of slides from Durand et al., 2015. Rimicaris spp. Deferribacteres are long and thin single cells, inserted between the microvilli of the midgut tube epithelial cells. They are visible in the lumen of the midgut tube, located between the intestinal epithelium and the peritrophic membrane, called the ectoperitrophic space (Figures 5A–C). The Deferribacteres are present all along the midgut tube (Figures 5A–C). In most observations, the bacteria were so long that they curled up on themselves, forming massive clusters on the epithelium of the midgut tube (Figures 5B, C).
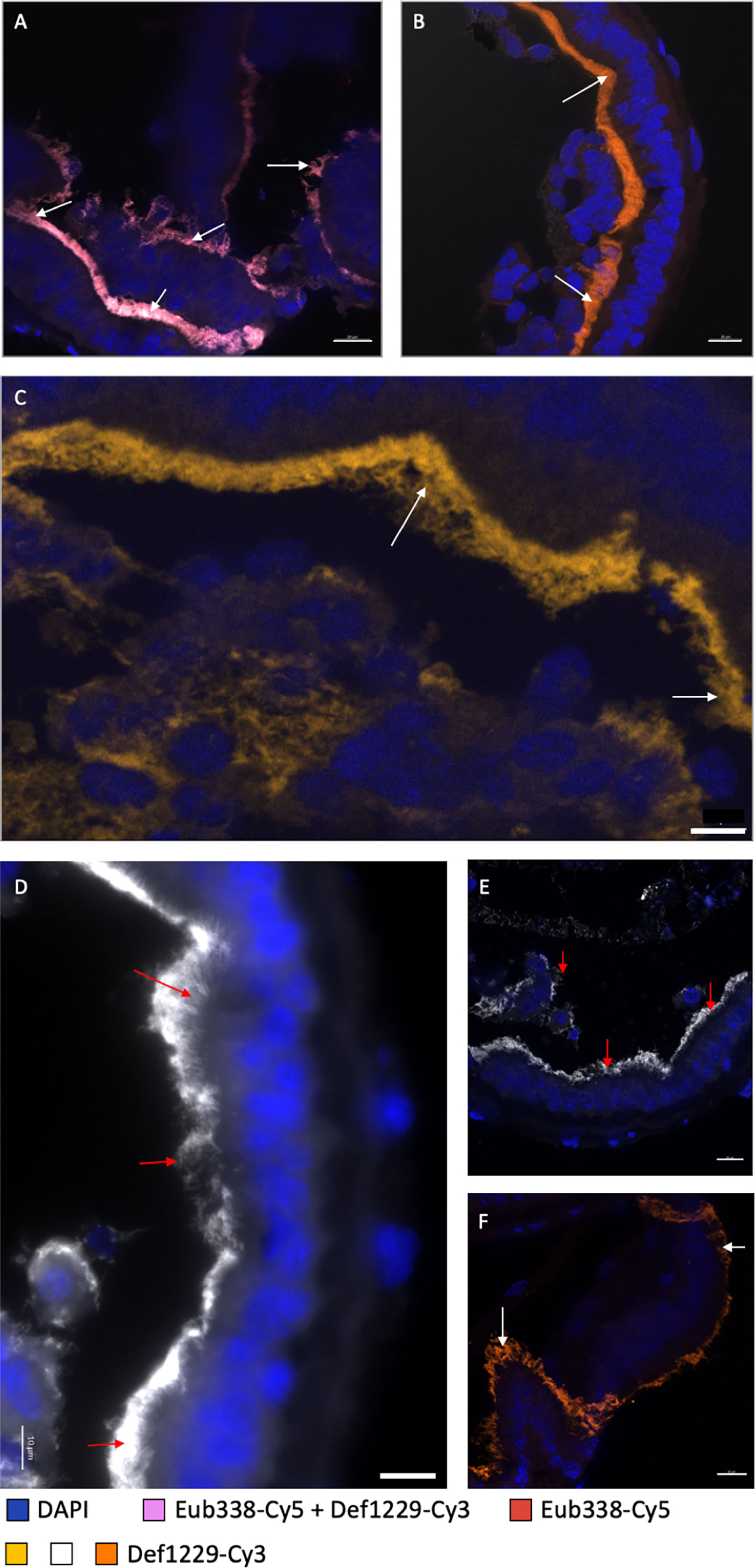
Figure 5 Observation of Deferribacteres in different midgut tube sections of a R. exoculata and a R. chacei adults. (A–C) represent sections through the midgut tube of R. exoculata adult hybridized with Eub338-Cy5 (red)/Def1229-Cy3 (white) (A) where double hybridization appeared in pink, or with Def1229-Cy3 (orange in (B) and yellow-brown in (C). Tissue cell nuclei are labeled with DAPI (blue). Formamide concentration in hybridization buffer was 30%. Pictures in (A–C) were taken with Apotome®, and with Z-stack and DIC for B only. (D–F) represent sections through the midgut tube of R. chacei adult hybridized with Def1229-Cy3 (white in (D, E) and orange in (F). Tissue cell nuclei are labeled with DAPI (blue). Formamide concentration in hybridization buffer was 30%. White and red arrows are targeting some Deferribacteres observed on different part of the midgut tube. Pictures (E, F) were taken with Apotome®. Scale bars (A–C, E, F) = 20 µm; Scale bar (D) = 10 µm.
FISH observations revealed no Deferribacteres in the alimentary bolus, which was rich in minerals and fragments of cuticle - probably from an ingested molt. In fact, the only bacteria (few rods and coccoids) visible in the alimentary bolus hybridized only with Epsy549 and GAM42a probes. Upon dissection, the midgut tube appeared more or less transparent (i.e. more or less empty). FISH observations confirmed that when the alimentary bolus was absent (transparent midgut tube), Deferribacteres were still observable while rods and coccoids from the alimentary bolus were not. No Deferribacteres signal was ever revealed on the scaphognathites nor in the foregut sections, suggesting restriction to the midgut tube.
R. chacei midgut tube sections from two specimens were also hybridized with the Def1229 probe and results were comparable to that of R. exoculata (Figures 5D–F). As for R. exoculata, the foregut was devoid of Deferribacteres, contrasting with the midgut tube (Figures 5D–F). Campylobacteria and Gammaproteobacteria cells were present only in the alimentary bolus of the midgut tube, showing coccoid and bacilli shapes. Minerals were less abundant in the alimentary bolus of both R. chacei specimens. The bolus was filled with cuticle fragments, more common than in R. exoculata. Of note, the Deferribacteres seemed longer on both R. chacei specimens, compared to R. exoculata ones.
To sum up, according to our observations on Rimicaris spp. adult specimens, Deferribacteres symbionts are long single-cell filaments. They are located in the ectoperitrophic space of the midgut tube, inserted between host epithelial cell microvilli, and are not washed out with the alimentary bolus (Figure 6).
Discussion
Foregut Functioning and Symbiosis
In omnivorous shrimp, the foregut is composed of two chambers, covered with teeth, setae and spicules allowing food processing and filtration of nutriments. The alimentary bolus contained in the cardiac chamber is directed towards the pyloric chamber by means of setose lateral ridges and lateral valves preventing regurgitation. After passing through sieves, the smallest particles within the alimentary bolus (less than 1µm) enter the hepatopancreas (a major digestive and storage gland in crustaceans) and larger ones are directed to the midgut tube, embedded in the peritrophic membrane. Thus, the stomach triturates food and acts as a filter, while the hepatopancreas and midgut tube are involved in nutrient absorption and digestion (Pattarayingsakul et al., 2019; Štrus et al., 2019). Our anatomical study of the stomach of Rimicaris spp. also revealed complex filtering structures. In R. exoculata, the very small size of the foregut and its thin cuticle reinforce the idea of a weak mechanical action (Segonzac et al., 1993; Durand et al., 2009), reminiscent of that described for the shrimp Macrobrachium carcinus (Lima et al., 2016). In M. carcinus, the pyloric and cardiac chambers form what appears to be a single cavity (Lima et al., 2016), with almost no grinding appendages – no teeth in the œsophagus, nor in the cardiac chamber, as in other crustaceans (Vogt, 2021). In this species, food trituration by the gastric mill is possible thanks to the large quantity of sand ingested by the shrimp (Lima et al., 2016). By analogy, in R. exoculata, ingested minerals may play the role of the grinding appendages, which are absent in this species (lacking teeth) and the alimentary bolus would then be directed toward the midgut through the pyloric sieve. In R. chacei, the foregut is almost twice as large as that of R. exoculata, with a thicker cuticle. This, together with the presence of functional chelipeds (Casanova et al., 1993), numerous pieces of cuticle and organic material in the digestive tract, strengthen the mixotrophic diet hypothesis (Apremont et al., 2018; Methou et al., 2020), with a more important foregut mechanical digestive activity.
Despite these anatomical differences, both species exhibit similar bacterial colonization of their foregut, including the œsophagus and stomach, with a higher density of bacteria observed in the two R. chacei specimens. Mycoplasmatales colonize specific areas inside the foregut of both Rimicaris spp. We observed them in dense clusters on the setae at the entrance of the foregut (œsophagus) or on setae of the pyloric chamber for R. exoculata, and in high density close to the junction with the midgut tube for R. chacei. Mycoplasmatales were sometimes difficult to observe in the pyloric chamber of R. exoculata. It can be hypothesized that they were hidden by the cuticle and setae that occupy most of the space in the pyloric chamber (Figure 4C), or that signal observation may have been impaired due to ingested minerals. However, the morphology of the foregut filtering structures probably depends on the molt cycle stage and the host species (denser and thicker in the mixotrophic R. chacei). In the same way, the symbiont colonization may be subjected to molt events and the associated loss of cuticle, including setae. Generally, Mycoplasmatales seemed to colonize preferentially areas with dense setae, which is reminiscent of bacterial colonization in the cephalothorax. However, additional observations on a higher number of specimens would be required to better understand the distribution of Mycoplasmatales within the Rimicaris foregut, and identify common patterns or variations related to life stage, molt stage, nutritional status or host species.
Being present in high abundance across all specimens, Mycoplasmatales may play an essential role in the digestion processes of Rimicaris spp. Where associated with thin setae in the anterior-most end of the foregut (œsophagus), they may be involved in processing minerals, food items or fragments of pieces. They are also observed on setae forming sieves of the pyloric chamber through which crushed food passes. Their role may then be comparable to that of the Bg1 and Bg2 Mycoplasmatales relatives found in the foregut of the marine isopods Bathynomus sp. (Wang et al., 2016). These lineages are close to ‘Candidatus Hepatoplasma crinochetorum’ found in the hepatopancreas of terrestrial isopods (Wang et al., 2004; Wang et al., 2007). In the case of Bathynomus sp., Bg1 and Bg2 Mycoplasmatales are found in the foregut, and enhance host development in nutrient-poor environments (Wang et al., 2016). In the same way, in terrestrial isopods, Mycoplasmatales are involved in food digestion through complementation of the host’s diet, which is based on leaves (Wang et al., 2004; Wang et al., 2007). In terrestrial isopods, digestion occurs in the hepatopancreas, after ingested material is crushed in the foregut, and the midgut tube is almost absent. This differs from marine isopods and was proposed to be an adaptation to terrestrial conditions (Wang et al., 2004). Mycoplasmatales may play the same role in the hepatopancreas of terrestrial isopods and in the foregut of marine crustaceans. In deep-sea hydrothermal environments, most nutrition, but not all, is fueled by symbiotic chemosynthesis. This may also lead to unbalanced diet, with regards to nutrients required to support chitin synthesis for example, as hydrothermal shrimp have a very short molt cycle duration compared to coastal shrimp (10 days for R. exoculata against 21 days fort Penaeus japonicus and 41 to 98 days for Macrobrachium rosenbergii) (Corbari et al., 2008). We can hypothesize that Mycoplasmatales help the host degrade chitin within the cuticle, as described for Porcellio scaber (Bouchon et al., 2016). This hypothesis is supported by the occurrence of genes coding for enzymes involved in chitin metabolism in the metagenomes of the Mycoplasmatales symbionts (Aubé, Cambon-Bonavita et al., submitted). Overall, these observations suggest that the Mycoplasmatales colonization is directed toward specific location on specific structures, probably related to their role and function. Neither the host nor the organ (hepatopancreas vs foregut) would have an impact on their colonization capacity.
Rimicaris spp. Midgut Tube Houses a Tight and Specific Symbiosis
Unaffiliated single-cell bacteria were previously observed using Transmission Electron Microscopy (TEM) and revealed by FISH with the universal probe Eub338 in the midgut tube of R. exoculata adults (Durand et al., 2009; Durand et al., 2015). These preliminary tests confirmed the presence of bacteria in the midgut tube, but did not allow their identification. The present study documents for the first time the main lineage of the midgut tube bacterial community with a specific probe targeting Deferribacteres in both R. exoculata and R. chacei (Figure 5). Deferribacteres colonize the full length of the midgut tube, even in specimens subjected to prolonged fasting [(Durand et al., 2009); courtesy of Durand slides]. The microorganisms are inserted between the microvilli of midgut tube epithelium cells and are thus separated from the alimentary bolus by the peritrophic membrane. Then, Deferribacteres may not participate in the digestive processes of the shrimp (Durand et al., 2009; Apremont et al., 2018; Zbinden and Cambon Bonavita, 2020), contrasting with gut symbionts known in other crustaceans (Martin et al., 2020). Unlike in the foregut or cephalothoracic cavity, there is no exuviation in the midgut tube, (Štrus et al., 2019). Thus, it is not subject to bacterial turnover with (re)colonization at each molt every 10 days (Corbari et al., 2008). The colonization of the midgut tube by Deferribacteres appears to be a relatively long-term association within the shrimp’s life cycle. Sequencing data obtained after a 72-hour fast in adults (Durand et al., 2009) as well as our FISH observations on full or empty midgut tubes confirmed this. Indeed, regardless of the nutritional state of the host, Deferribacteres were attached to the microvilli of the epithelium cell and can be described as resident, rather than transient, in nature.
Filamentous bacteria are usually composed of several subunits resulting from cell division, as observed for Campylobacteria or Gammaproteobacteria lineages in the cephalothoracic cavity of Rimicaris shrimp. TEM observations showed a different morphology for Deferribacteres observed in the midgut tube of both hosts (Durand et al., 2009; Apremont et al., 2018). These microorganisms do not undergo cell division, although they appear highly active according to FISH results. As the FISH procedure is based on probe hybridization of rRNA molecules located in the ribosomes, inactive or poorly active cells are expected to have a lower ribosome content and so, would give weak or even no signal, which is not the case here. The morphology of Deferribacteres in the midgut tube of our shrimp is reminiscent of that in bacteria experiencing stress, such as pressure or antibiotic inhibitors (Spratt, 1975; Welch et al., 1993). Indeed, a metagenomic study (Aubé, Cambon-Bonavita et al., submitted) showed that Deferribacteres have the genetic machinery for cell division, which is somehow inhibited. Such inhibition could result from a significant control of the host. Control of symbiont cell division by the host has already been suggested for the symbiont Vesicomyosocius okutanii of Calyptogena clams (Kuwahara et al., 2007; Duperron, 2017). Our FISH results in co-hybridization with Def1229 and Eub338 probes showed that this community is almost exclusively composed of Deferribacteres. This further suggests a control by the host, and/or tight recognition mechanisms preventing colonization by other lineages. In the same way, Vibrio fischeri is the only symbiont able to colonize the host thanks to complex recognition mechanisms (McFall-Ngai, 2014; Duperron, 2017).
Our FISH observations showed differences between Deferribacteres in our two host species: they appear as relatively longer cells in the two observed R. chacei specimens. However, since a low number of individuals were examined here, the variation in Deferribacteres cell length may not be attributable to the host species only, but perhaps also to intraspecific variability related to the host life stage. Additional observations including a larger number of specimens are necessary to evaluate the respective influence of host diet (mixotrophic in R. chacei vs symbiotrophic in R. exoculata), life stage, molt stage, individual nutritional status, etc … on the Deferribacteres lineages within the midgut tube.
According to our observations, Deferribacteres cells are in direct contact with their host, located in the ectoperitrophic space (Figure 6). This contradicts previous reports on shrimp and crustaceans more generally (Martin et al., 2020; Vogt, 2021). In Artemia salina, Daphnia magna, Gammarus spp., Homarus americanus, Tigriopus californicus and Sicyonia ingentis, microorganisms are in the midgut tube and hindgut, located inside the alimentary bolus within the endoperitrophic space. The peritrophic membrane that separates the endoperitrophic space and the ectoperitrophic space usually protects the epithelium against pathogens and more generally prevents any bacterial colonization (Martin et al., 2020). For the six above-mentioned species, microorganisms are ingested during feeding and evacuated with the feces, and are not considered essential for host nutrition and digestion (Martin et al., 2020). Our results show that Deferribacteres are located within the ectoperitrophic space, which is then not “sterile”, contrasting with observations made in many other crustaceans (Martin et al., 2020). This exceptional localization strengthens the hypothesis that the Deferribacteres play a specific role in both Rimicaris holobiont.
The role of Deferribacteres in the midgut tube remains enigmatic; metagenomics study recently suggested that this lineage would be involved in host nutrition and defense (genes coding for fumarate reductase, genes required for biotin and riboflavin biosynthesis, immunity systems, Aubé, Cambon-Bonavita et al., submitted). Their high abundance in both Rimicaris spp. may provide protection against proliferation of deleterious bacteria. This is the case of Deferribacteres found in mammals, particularly in rodents (Lee, 1985). In rats and mice, Deferribacteres relatives (Mucispirillum schaedleri (Robertson et al., 2005)) are located in the mucus layer covering and protecting the gastrointestinal epithelial cells (Lee, 1980). This mucus harbors other spiral bacteria belonging to the genus Helicobacter and Campylobacter (Lee et al., 1968; Davis et al., 1972), as well as Salmonella enterica serovar Typhimurium causing non-typhoidal colitis in hosts (Herp et al., 2019). There, Deferribacteres play a key protective role in the symbiosis of the digestive system as they outcompete pathogenic Salmonella spp. (Herp et al., 2019). Similarly, in Rimicaris shrimp, Deferribacteres may act as a barrier against deleterious microorganisms and play two roles: protection of the host through competitive interactions with other bacteria, and host nutrition. They may also play a role in detoxification of minerals contained in the alimentary bolus. In fact, Deferribacteres are usually described as involved in the use of minerals such as iron, similarly to bacteria of the cephalothoracic cavity that may be involved in mineral detoxification (for review see Zbinden and Cambon Bonavita, 2020).
A Complex Symbiosis Linked to the Diet of Both Rimicaris spp.
Similarly to the cephalothoracic cavity of the two Rimicaris spp., their digestive system differs in morphology. Our detailed observations confirm that the foregut of Rimicaris spp. is a filtering structure as in other shrimp. The œsophagus is reduced to a simple structure with filtering elements. The stomach is deprived of a grinding appendage but exhibits many setae and a sieve to filter nutrients and minerals. This stomach is highly reduced in R. exoculata but not the midgut tube. In contrast, the digestive system of the few R. chacei observed (FISH, binocular microscope) showed significant morphological differences: the stomach is much more voluminous compared to that of R. exoculata, with a thicker cuticle, and a larger pyloric chamber. We hypothesize that the comparably more developed internal structure with grinding appendages and free mandibles facilitate food uptake and digestion in this species. Digestive system size and structure may therefore be related to diet, which is predominantly chemosynthetic in R. exoculata and mixotrophic in R. chacei. To draw stronger conclusions with regards to anatomical adaptation to nutritional mode in these shrimp, further observations of R. chacei specimens are required, across life stages and molt cycles.
The digestive symbioses of both Rimicaris spp. share similarities with other species such as mammals for the midgut tube and other terrestrial, freshwater or marine crustaceans for the foregut. These symbioses may allow the host to adapt to hostile and nutrient-poor or unbalanced environments, and in return allow the symbionts to find shelter and colonize favorable niches. Hydrothermal shrimp show commonalities with isopods in the functioning of their foregut and with mice for the midgut tube functioning. This suggests a somewhat “universal” evolution of digestive symbiosis. The specific location of the various microorganisms in the digestive system allows us to propose hypothesis about their role and function. The Deferribacteres could be mainly protective for the host, while the Mycoplasmatales may support host development. Their function has yet to be further studied through metagenomics and in vivo studies.
Knowledge gaps persist regarding the transmission of Deferribacteres and Mycoplasmatales lineages along Rimicaris life cycle and molt events. Because the foregut is covered with cuticle, which is lost during exuviation, the hypothesis of horizontal transmission is often proposed for Mycoplasmatales. On the contrary, the control of Deferribacteres cell division and colonization by the host, together with the quasi absence of their free-living relatives in water surrounding shrimp (Hügler et al., 2010; Flores et al., 2011; Durand et al., 2015; Cowart et al., 2017) suggests specific symbiont recognition and potential vertical transmission. However, no symbiont was observed on the envelope of brooded eggs (Methou et al., 2019), raising questions about potential mechanisms allowing such vertical transmission for Deferribacteres. Another mode of transmission may be proposed, as for terrestrial isopods, where juveniles eat the feces of the adults when ingesting food on litter (Wang et al., 2007). This may be the case for the R. exoculata where juveniles are recruited close to adult aggregations (Methou et al., 2022). In R. chacei, however, juveniles are mainly recruited in isolated patches, with few or no adults (Methou et al., 2022). In theory, recruitment away from adults could hinder the acquisition of digestive symbionts, leading to lower holobiont fitness, and may be a partial explanation of the observed population collapse of R. chacei. Still, more studies are required especially on juveniles to establish whether symbionts are acquired during recruitment. Understanding the mode of acquisition of these symbionts (horizontal, vertical and/or mixed transmission) would help to understand the development of both hosts and their relation to their symbionts. The study of juveniles and larvae (Guéganton et al., in prep) is essential to better understand symbiont transmission, localization and functions in these shrimp.
Data Availability Statement
The original contributions presented in the study are included in the article/Supplementary Material. Further inquiries can be directed to the corresponding author.
Author Contributions
MG contributed to data acquisition and analysis, wrote the first draft of the manuscript, review and editing of this manuscript. LD and VC-G contributed for methodology for biology work. OR and LD contributed for FISH microscopy data acquisition and review of this manuscript. NG contributed for electron microscopy data acquisition. FP and M-AC-B contributed to the conception and the design of this study, review and editing of the manuscript, and the supervision of the project. All authors contributed to the article and approved the submitted version.
Funding
Funding was provided by Ifremer REMIMA program, and Ifremer and Région Bretagne doctoral grant.
Conflict of Interest
The authors declare that the research was conducted in the absence of any commercial or financial relationships that could be construed as a potential conflict of interest.
Publisher’s Note
All claims expressed in this article are solely those of the authors and do not necessarily represent those of their affiliated organizations, or those of the publisher, the editors and the reviewers. Any product that may be evaluated in this article, or claim that may be made by its manufacturer, is not guaranteed or endorsed by the publisher.
Acknowledgments
We thank cruise chief scientist of BICOSE2 2018 cruise (M-AC-B) and the captain and crew of R/V Pourquoi pas? and Nautile for logistic assistance in collecting samples.
Supplementary Material
The Supplementary Material for this article can be found online at: https://www.frontiersin.org/articles/10.3389/fmars.2022.903748/full#supplementary-material
References
Altschul S. F., Gish W., Miller W., Myers E. W., Lipman D. J. (1990). Basic Local Alignment Search Tool. J. Mol. Biol. 215, 403–410. doi: 10.1016/S0022-2836(05)80360-2
Amann R. I., Binder B. J., Olson R. J., Chisholm S. W., Devereux R., Stahl D. A. (1990). Combination of 16s Ribosomal-Rna-Targeted Oligonucleotide Probes With Flow-Cytometry for Analyzing Mixed Microbial-Populations. Appl. Environ. Microbiol. 56, 1919–1925. doi: 10.1128/aem.56.6.1919-1925.1990
Amann R., Fuchs B., Behrens S. (2001). The Identification of Microorganisms by Fluorescence in Situ Hybridisation. Curr. Opin. Biotechnol. 12, 231–236. doi: 10.1016/S0958-1669(00)00204-4
Apremont V., Cambon-Bonavita M.-A., Cueff-Gauchard V., François D., Pradillon F., Corbari L., et al. (2018). Gill Chamber and Gut Microbial Communities of the Hydrothermal Shrimp Rimicaris Chacei Williams and Rona 1986: A Possible Symbiosis. PloS One 13, e0206084. doi: 10.1371/journal.pone.0206084
Aubé J., Cambon-Bonavita M.-A., Velo-Suárez L., Cueff-Gauchard V., Lesongeur F., Guéganton M., et al. A Novel and Dual Digestive Symbiosis Scales Up the Nutrition and Immune System of the Holobiont Rimicaris Exoculata. Submitted to Microbiome. doi: 10.21203/rs.3.rs-1584541/v1
Behrens S., Fuchs B. M., Mueller F., Amann R. (2003). Is the In Situ Accessibility of the 16S rRNA of Escherichia Coli for Cy3-Labeled Oligonucleotide Probes Predicted by a Three-Dimensional Structure Model of the 30S Ribosomal Subunit? Appl. Environ. Microbiol. 69, 4935–4941. doi: 10.1128/AEM.69.8.4935-4941.2003
Behrens S., Rühland C., Inácio J., Huber H., Fonseca Á., Spencer-Martins I., et al. (2003). In Situ Acessibility of Small-Subunit rRNA of Members of the Domains Bacteria, Archea, and Eucarya to Cy3-Labeled Oligonucleotide Probes. Appl. Environ. Microbiol. 69, 1748–1758. doi: 10.1128/AEM.69.3.1748–1758.2003
Bouchon D., Zimmer M., Dittmer J. (2016). The Terrestrial Isopod Microbiome: An All-In-One Toolbox for Animal–Microbe Interactions of Ecological Relevance. Front. Microbiol. 7. doi: 10.3389/fmicb.2016.01472
Casanova B., Brunet M., Segonzac M. (1993). Impact of Bacterial Epibiosis on Functional Morphology of Shrimp Associated With the Mid-Atlantic Hydrothermal Conditions. Cahiers Biologie Mar. 34, 573–588. doi: 10.21411/CBM.A.E587F68C
Ceccaldi H. (1989). “Anatomy and Physiology of Digestive Tract of Crustaceans Decapods Reared in Aquaculture,” in Advances in Tropical Aquaculture, vol. 9. (Tahiti, French Polynesia: Actes de colloques Ifremer), 243–259. Available at: https://archimer.ifremer.fr/doc/00000/1486/.
Corbari L., Zbinden M., Cambon-Bonavita M.-A., Gaill F., Compere P. (2008). Bacterial Symbionts and Mineral Deposits in the Branchial Chamber of the Hydrothermal Vent Shrimp Rimicaris Exoculata: Relationship to Moult Cycle. Aquat. Biol. 1, 225–238. doi: 10.3354/ab00024
Cowart D. A., Durand L., Cambon-Bonavita M. A., Arnaud-Haond S. (2017). Investigation of Bacterial Communities Within the Digestive Organs of the Hydrothermal Vent Shrimp Rimicaris Exoculata Provide Insights Into Holobiont Geographic Clustering. PloS One 12, 1–22. doi: 10.1371/journal.pone.0172543
Davis C. P., Mulcahy D., Takeuchi A., Savage D. C. (1972). Location and Description of Spiral-Shaped Microorganisms in the Normal Rat Cecum. Infect. Immunity 2 (6), 184–192. doi: 10.1128/iai.6.2.184-192.1972
Dubilier N., Bergin C., Lott C. (2008). Symbiotic Diversity in Marine Animals: The Art of Harnessing Chemosynthesis. Nat. Rev. Microbiol. 6, 725–740. doi: 10.1038/nrmicro1992
Duperron S., Sibuet M., MacGregor B. J., Kuypers M. M. M., Fisher C. R., Dubilier N. (2007). Diversity, Relative Abundance and Metabolic Potential of Bacterial Endosymbionts in Three Bathymodiolus Mussel Species From Cold Seeps in the Gulf of Mexico. Environ. Microbiol. 9, 1423–1438. doi: 10.1111/j.1462-2920.2007.01259.x
Durand L., Roumagnac M., Cueff-Gauchard V., Jan C., Guri M., Tessier C., et al. (2015). Biogeographical Distribution of Rimicaris Exoculata Resident Gut Epibiont Communities Along the Mid-Atlantic Ridge Hydrothermal Vent Sites. FEMS Microbiol. Ecol. 91, 1–15. doi: 10.1093/femsec/fiv101
Durand L., Zbinden M., Cueff-Gauchard V., Duperron S., Roussel E. G., Shillito B., et al. (2009). Microbial Diversity Associated With the Hydrothermal Shrimp Rimicaris Exoculata Gut and Occurrence of a Resident Microbial Community. FEMS Microbiol. Ecol. 71, 291–303. doi: 10.1111/j.1574-6941.2009.00806.x
Edgar R. C. (2004). MUSCLE: Multiple Sequence Alignment With High Accuracy and High Throughput. Nucleic Acids Res. 32, 1792–1797. doi: 10.1093/nar/gkh340
Flores G. E., Campbell J. H., Kirshtein J. D., Meneghin J., Podar M., Steinberg J. I., et al. (2011). Microbial Community Structure of Hydrothermal Deposits From Geochemically Different Vent Fields Along the Mid-Atlantic Ridge. Environ. Microbiol. 13, 2158–2171. doi: 10.1111/j.1462-2920.2011.02463.x
Garrity G. M., Holt J. M., Huber H., Karl O. S., Greene A. C., Patel B. K. C., et al. (2001). Pylum BIX. Deferribacteres Phy. Nov. Bergey’s Manual® Systematic Bacteriol. 1, 465–471. doi: 10.1007/978-0-387-21609-6_26
Gebruk A. V., Chevaldonne P., Shank T., Lutz R. A., Vrijenhoek R. C. (2000). Deep-Sea Hydrothermal Vent Communities of the Logatchev Area (14 Degrees 45’n, Mid-Atlantic Ridge): Diverse Biotopes and High Biomass. J. Mar. Biol. Assoc. United Kingdom 80, 383–393. doi: 10.1017/S0025315499002088
Gebruk A. V., Galkin S. V., Vereshchaka A. L., Moskalev L. I., Southward A. J. (1997). Ecology and Biogeography of the Hydrothermal Vent Fauna of the Mid-Atlantic Ridge. Adv. Mar. Biol. 32, 93–144. doi: 10.1016/S0065-2881(08)60016-4
Guri M., Durand L., Cueff-Gauchard V., Zbinden M., Crassous P., Shillito B., et al. (2012). Acquisition of Epibiotic Bacteria Along the Life Cycle of the Hydrothermal Shrimp Rimicaris Exoculata. ISME J. 6, 597–609. doi: 10.1038/ismej.2011.133
Hernández-Ávila I., Cambon-Bonavita M.-A., Sarrazin J., Pradillon F. (2021). Population Structure and Reproduction of the Alvinocaridid Shrimp Rimicaris Exoculata on the Mid-Atlantic Ridge: Variations Between Habitats and Vent Fields. bioRxiv 06.27, 450066. doi: 10.1101/2021.06.27.450066
Herp S., Brugiroux S., Garzetti D., Ring D., Jochum L. M., Beutler M., et al. (2019). Mucispirillum Schaedleri Antagonizes Salmonella Virulence to Protect Mice Against Colitis. Cell Host Microbe 25, 681–694.e8. doi: 10.1016/j.chom.2019.03.004
Hügler M., Gärtner A., Imhoff J. F. (2010). Functional Genes as Markers for Sulfur Cycling and CO2 Fixation in Microbial Communities of Hydrothermal Vents of the Logatchev Field. FEMS Microbiol. Ecol. 73, 526–537. doi: 10.1111/j.1574-6941.2010.00919.x
Kearse M., Moir R., Wilson A., Stones-Havas S., Cheung M., Sturrock S., et al. (2012). Geneious Basic: An Integrated and Extendable Desktop Software Platform for the Organization and Analysis of Sequence Data. Bioinformatics 28, 1647–1649. doi: 10.1093/bioinformatics/bts199
Kibbe W. A. (2007). OligoCalc: An Online Oligonucleotide Properties Calculator. Nucleic Acids Res. 35, W43–W46. doi: 10.1093/nar/gkm234
Komai T., Segonzac M. (2008). Taxonomic Review of the Hydrothermal Vent Shrimp Genera Rimicaris Williams & Rona and Chorocaris Martin & Hessler (Crustacea: Decapoda: Caridea: Alvinocarididae). J. Shellfish Res. 27, 21–41. doi: 10.2983/0730-8000(2008)27[21:trothv]2.0.co;2
Kouris A., Juniper S. K., Frébourg G., Gail F. (2007). Protozoan-Bacterial Symbiosis in a Deep-Sea Hydrothermal Vent Folliculinid Ciliate (Folliculinopsis Sp.) From the Juan De Fuca Ridge. Mar. Ecol. 28, 63–71. doi: 10.1111/j.1439-0485.2006.00118.x
Kuwahara H., Yoshida T., Takaki Y., Shimamura S., Nishi S., Harada M., et al. (2007). Reduced Genome of the Thioautotrophic Intracellular Symbiont in Deep-Sea Clam, Calyptogena Okutanii. Curr. Biol. 17, 881–886. doi: 10.1016/j.cub.2007.04.039
Lee A. (1980). “Normal Flora of Animal Intestinal Surfaces,” in Adsorption of Microorganisms to Surfaces (New York: Wiley), 145–173.
Lee A. (1985). “Neglected Niches,” in Advances in Microbial Ecology: Volume 8 Advances in Microbial Ecology. Ed. Marshall K. C. (Boston, MA: Springer US), 115–162. doi: 10.1007/978-1-4615-9412-3_3
Lee A., Gordon J., Dubos R. (1968). Enumeration of the Oxygen Sensitive Bacteria Usually Present in the Intestine of Healthy Mice. Nature 220, 1137–1139. doi: 10.1038/2201137a0
Lima J.de F., Garcia J.da S., Tavares M. (2016). Foregut Morphology of Macrobrachium Carcinus (Crustacea, Decapoda, Palaemonidae). Acta Amaz. 46, 209–218. doi: 10.1590/1809-4392201501214
Lin X. J., Wakeham S. G., Putnam I. F., Astor Y. M., Scranton M. I., Chistoserdov A. Y., et al. (2006). Comparison of Vertical Distributions of Prokaryotic Assemblages in the Anoxic Cariaco Basin and Black Sea by Use of Fluorescence in Situ Hybridization. Appl. And Environ. Microbiol. 72, 2679–2690. doi: 10.1128/aem.72.4.2679-2690.2006
Manz W., Amann R., Ludwig W., Wagner M., Schleifer K. H. (1992). Phylogenetic Oligodeoxynucleotide Probes for the Major Subclasses of Proteobacteria - Problems and Solutions. Systematic Appl. Microbiol. 15, 593–600. doi: 10.1016/S0723-2020(11)80121-9
Martin G. G., Natha Z., Henderson N., Bang S., Hendry H., Loera Y. (2020). Absence of a Microbiome in the Midgut Trunk of Six Representative Crustacea. J. Crustacean Biol. 40, 122–130. doi: 10.1093/jcbiol/ruz087
McFall-Ngai M. J. (2014). The Importance of Microbes in Animal Development: Lessons From the Squid-Vibrio Symbiosis. Annu. Rev. Microbiol. 68, 177–184. doi: 10.1146/annurev-micro-091313-103654
Methou P., Hernández-Ávila I., Aube J., Cueff-Gauchard V., Gayet N., Amand L., et al. (2019). Is It First the Egg or the Shrimp? – Diversity and Variation in Microbial Communities Colonizing Broods of the Vent Shrimp Rimicaris Exoculata During Embryonic Development. Front. In Microbiol. 10. doi: 10.3389/fmicb.2019.00808
Methou P., Hernández-Ávila I., Cathalot C., Cambon-Bonavita M.-A., Pradillon F. (2022). Population Structure and Environmental Niches of Rimicaris Shrimp From the Mid-Atlantic Ridge. Mar. Ecol. Prog. Ser. 684, 1–20. doi: 10.3354/meps13986
Methou P., Michel L., Segonzac M., Cambon-Bonavita M.-A., Pradillon F. (2020). Integrative Taxonomy Revisits the Ontogeny and Trophic Niches of Rimicaris Vent Shrimp. R. Soc. Open Sci. 7. doi: 10.1098/rsos.200837
Pattarayingsakul W., Pudgerd A., Munkongwongsiri N., Vanichviriyakit R., Chaijarasphong T., Thitamadee S., et al. (2019). The Gastric Sieve of Penaeid Shrimp Species Is a Sub-Micron Nutrient Filter. J. Exp. Biol. 222, jeb.199638. doi: 10.1242/jeb.199638
Ponsard J., Cambon-Bonavita M.-A., Zbinden M., Lepoint G., Joassin A., Corbari L., et al. (2013). Inorganic Carbon Fixation by Chemosynthetic Ectosymbionts and Nutritional Transfers to the Hydrothermal Vent Host-Shrimp Rimicaris Exoculata. ISME J. 7, 96–109. doi: 10.1038/ismej.2012.87
Robertson B. R., O’ Rourke J. L., Neilan B. A., Vandamme P., On S. L. W., Fox J. G., et al. (2005). Mucispirillum Schaedleri Gen. Nov., Sp. Nov., a Spiral-Shaped Bacterium Colonizing the Mucus Layer of the Gastrointestinal Tract of Laboratory Rodents. Int. J. Systematic Evolutionary Microbiol. 55, 1199–1204. doi: 10.1099/ijs.0.63472-0
Schmidt C., Le Bris N., Gaill F. (2008a). Interactions of Deep-Sea Vent Invertebrates With Their Environment: The Case of Rimicaris Exoculata. J. Shellfish Res. 27, 79–90. doi: 10.2983/0730-8000(2008)27[79:IODVIW]2.0.CO;2
Schmidt C., Vuillemin R., Le Gall C., Gaill F., Le Bris N. (2008b). Geochemical Energy Sources for Microbial Primary Production in the Environment of Hydrothermal Vent Shrimp. Mar. Chem. 108, 18–31. doi: 10.1016/j.marchem.2007.09.009
Segonzac M., de Saint Laurent M., Casanova B. (1993). L’énigme Du Comportement Trophique Des Crevettes Alvinocarididae Des Sites Hydrothermaux De La Dorsale Médio-Atlantique. Cahiers Biologie Mar. 34, 535–571. doi: 10.21411/CBM.A.B3683E29
Sogin E. M., Kleiner M., Borowski C., Gruber-Vodicka H. R., Dubilier N. (2021). Life in the Dark: Phylogenetic and Physiological Diversity of Chemosynthetic Symbioses. Annu. Rev. Microbiol. 75, 695–718. doi: 10.1146/annurev-micro-051021-123130
Spratt B. G. (1975). Distinct Penicillin Binding Proteins Involved in the Division, Elongation, and Shape of Escherichia Coli K12. Proc. Nat. Acad. Sci. U.S.A. 72, 2999–3003. doi: 10.1073/pnas.72.8.2999
Štrus J., Žnidaršič N., Mrak P., Bogataj U., Vogt G. (2019). Structure, Function and Development of the Digestive System in Malacostracan Crustaceans and Adaptation to Different Lifestyles. Cell Tissue Res. 377, 415–443. doi: 10.1007/s00441-019-03056-0
Tully J. G., Bove J. M., Laigret F., Whitcomb R. F. (1993). Revised Taxonomy of the Class Mollicutes: Proposed Elevation of a Monophyletic Cluster of Arthropod-Associated Mollicutes to Ordinal Rank (Entomoplasmatales Ord. Nov.), With Provision for Familial Rank To Separate Species With Nonhelical Morphology (Entomoplasmataceace Fam. Nov.) From Helical Species (Spiroplasmataceae), and Emended Descriptions of the Order Mycoplasmatales, Family Mycoplasmataceae. Int. J. Systematic Bacteriol. 43, 378–385. doi: 10.1099/00207713-43-2-378
Van Dover C. L., Fry B., Grassle J. F., Humphris S., Rona P. A. (1988). Feeding Biology of the Shrimp Rimicaris Exoculata at Hydrothermal Vents on the Mid-Atlantic Ridge. Mar. Biol. 98, 209–216. doi: 10.1007/BF00391196
Vogt G. (2021). Synthesis of Digestive Enzymes, Food Processing, and Nutrient Absorption in Decapod Crustaceans: A Comparison to the Mammalian Model of Digestion. Zoology 147, 125945. doi: 10.1016/j.zool.2021.125945
Wang Y., Brune A., Zimmer M. (2007). Bacterial Symbionts in the Hepatopancreas of Isopods: Diversity and Environmental Transmission. FEMS Microbiol. Ecol. 61, 141–152. doi: 10.1111/j.1574-6941.2007.00329.x
Wang Y., Huang J.-M., Wang S.-L., Gao Z.-M., Zhang A.-Q., Danchin A., et al. (2016). Genomic Characterization of Symbiotic Mycoplasmas From the Stomach of Deep-Sea Isopod Bathynomus Sp. Environ. Microbiol. 18, 2646–2659. doi: 10.1111/1462-2920.13411
Wang Y., Stingl U., Anton-Erxleben F., Geisler S., Brune A., Zimmer M. (2004). “Candidatus Hepatoplasma Crinochetorum”, A New, Stalk-Forming Lineage of Mollicutes Colonizing the Midgut Glands of a Terrestrial Isopod. Appl. Environ. Microbiol. 70, 6166–6172. doi: 10.1128/AEM.70.10.6166-6172.2004
Welch T. J., Farewell A., Neidhardt F. C., Bartlett D. H. (1993). Stress Response of Escherichia Coli to Elevated Hydrostatic Pressure. J. Bacteriol. 175, 7170–7177. doi: 10.1128/jb.175.22.7170-7177.1993
Williams A. B., Rona P. A. (1986). Two New Caridean Shrimp (Bresiliidae) From a Hydrothermal Field on the Mid-Atlantic Ridge. J. Crustacean Biol. 6, 446–462. doi: 10.1163/193724086X00299
Zbinden M., Cambon Bonavita M.-A. (2020). Rimicaris Exoculata: Biology and Ecology of a Shrimp From Deep-Sea Hydrothermal Vents Associated With Ectosymbiotic Bacteria. Mar. Ecology-Progress Ser. 652, 187–222. doi: 10.3354/meps13467
Keywords: Rimicaris, digestive-symbiosis, Deferribacteres, Mycoplasmatales, microscopy, FISH-probes, foregut, midgut
Citation: Guéganton M, Rouxel O, Durand L, Cueff-Gauchard V, Gayet N, Pradillon F and Cambon-Bonavita M-A (2022) Anatomy and Symbiosis of the Digestive System of the Vent Shrimp Rimicaris Exoculata and Rimicaris Chacei Revealed Through Imaging Approaches. Front. Mar. Sci. 9:903748. doi: 10.3389/fmars.2022.903748
Received: 24 March 2022; Accepted: 23 May 2022;
Published: 20 June 2022.
Edited by:
Pei-Yuan Qian, Hong Kong University of Science and Technology, Hong Kong, SAR ChinaReviewed by:
Maeva Perez, Université de Montréal, MontrealSuzanne Dufour, Memorial University of Newfoundland, Canada
Copyright © 2022 Guéganton, Rouxel, Durand, Cueff-Gauchard, Gayet, Pradillon and Cambon-Bonavita. This is an open-access article distributed under the terms of the Creative Commons Attribution License (CC BY). The use, distribution or reproduction in other forums is permitted, provided the original author(s) and the copyright owner(s) are credited and that the original publication in this journal is cited, in accordance with accepted academic practice. No use, distribution or reproduction is permitted which does not comply with these terms.
*Correspondence: Marie-Anne Cambon-Bonavita, TWFyaWUuQW5uZS5DYW1ib25AaWZyZW1lci5mcg==